- 1International Pacific Research Center, School of Ocean and Earth Science and Technology, University of Hawaii, Honolulu, HI, United States
- 2European Space Research and Technology Centre, European Space Agency, Noordwijk, Netherlands
- 3Sea Education Association, Falmouth, MA, United States
- 4Institute for Marine and Atmospheric Research, Utrecht University, Utrecht, Netherlands
- 5Marine Sensor Systems, Institute for Chemistry and Biology of the Marine Environment, University of Oldenburg, Oldenburg, Germany
- 6National Oceanography Centre, Southampton, United Kingdom
- 7Département Océanographie et Dynamique des Écosystémes, Institut Français de Recherche pour l'Exploitation de la Mer, Bastia, France
- 8Plymouth Marine Laboratory, Plymouth, United Kingdom
- 9Environmental Research Institute, University of the Highlands and Islands, Thurso, United Kingdom
- 10Deltares, Delft, Netherlands
- 11University of Plymouth, Plymouth, United Kingdom
- 12Laboratoire d'Océanographie Physique et Spatiale, Institute of Research for Development, Brest, France
- 13Remote Sensing Solutions, Los Angeles, CA, United States
- 14Department of Biology, Faculty of Science, University of Southern Denmark, Odense, Denmark
- 15European Commission, Joint Research Centre (JRC), Ispra, Italy
- 16Hawai‘i Wildlife Fund, Paia, HI, United States
- 17Scripps Institution of Oceanography, University of California, San Diego, La Jolla, CA, United States
- 18Department of Geosciences and Natural Resource Management (IGN), University of Copenhagen, Copenhagen, Denmark
- 19Atlantic Oceanographic and Meteorological Laboratory (NOAA), Miami, FL, United States
- 20Sezione di Oceanografia, Istituto Nazionale di Oceanografia e di Geofisica Sperimentale, Trieste, Italy
- 21Department of Oceanography and Fisheries, University of the Azores, Ponta Delgada, Portugal
- 22Research Institute for Applied Mechanics, Kyushu University, Fukuoka, Japan
- 23Applied Research Laboratory, Hawaii Institute of Geophysics and Planetology, University of Hawaii, Honolulu, HI, United States
- 24P.P. Shirshov Institute of Oceanology, Russian Academy of Sciences, Moscow, Russia
- 25NASA Jet Propulsion Laboratory, California Institute of Technology, Pasadena, CA, United States
- 26CNR Institute of Marine Sciences, Lerici, Italy
- 27Argans, Plymouth, United Kingdom
- 28Center for Global Discovery and Conservation Science, Arizona State University, Tempe, AZ, United States
- 29Mystic Seaport Program, Williams College, Mystic, CT, United States
- 30Environmental Protection Agency (EPA), San Francisco, CA, United States
- 31School of Ocean and Earth Science, National Oceanography Centre, University of Southampton, Southampton, United Kingdom
- 32College of Life and Environmental Science, University of Exeter, Exeter, United Kingdom
- 33eOdyn, Plouzané, France
- 34Smithsonian Environmental Research Center, Edgewater, MD, United States
- 35CSIRO Oceans and Atmosphere, Hobart, TAS, Australia
- 36International Council for the Exploration of the Sea, Copenhagen, Denmark
- 37The Ocean Cleanup, Delft, Netherlands
- 38Department of Environment and Health, Vrije Universiteit Amsterdam, Amsterdam, Netherlands
- 39Algalita Marine Research and Education, Long Beach, CA, United States
- 40Mace Geospatial, LLC, Menasha, WI, United States
- 41Freestone Environmental Services, Richland, WA, United States
- 42National Oceanic and Atmospheric Administration, Seattle, WA, United States
- 43Carl Sagan Center, SETI Institute, Mountain View, CA, United States
- 44COISPA Tecnologia and Ricerca, Bari, Italy
- 45Facultad Ciencias del Mar, Universidad Católica del Norte, Coquimbo, Chile
- 46Oceanographic Institute, Universidade de São Paulo, São Paulo, Brazil
Plastics and other artificial materials pose new risks to the health of the ocean. Anthropogenic debris travels across large distances and is ubiquitous in the water and on shorelines, yet, observations of its sources, composition, pathways, and distributions in the ocean are very sparse and inaccurate. Total amounts of plastics and other man-made debris in the ocean and on the shore, temporal trends in these amounts under exponentially increasing production, as well as degradation processes, vertical fluxes, and time scales are largely unknown. Present ocean circulation models are not able to accurately simulate drift of debris because of its complex hydrodynamics. In this paper we discuss the structure of the future integrated marine debris observing system (IMDOS) that is required to provide long-term monitoring of the state of this anthropogenic pollution and support operational activities to mitigate impacts on the ecosystem and on the safety of maritime activity. The proposed observing system integrates remote sensing and in situ observations. Also, models are used to optimize the design of the system and, in turn, they will be gradually improved using the products of the system. Remote sensing technologies will provide spatially coherent coverage and consistent surveying time series at local to global scale. Optical sensors, including high-resolution imaging, multi- and hyperspectral, fluorescence, and Raman technologies, as well as SAR will be used to measure different types of debris. They will be implemented in a variety of platforms, from hand-held tools to ship-, buoy-, aircraft-, and satellite-based sensors. A network of in situ observations, including reports from volunteers, citizen scientists and ships of opportunity, will be developed to provide data for calibration/validation of remote sensors and to monitor the spread of plastic pollution and other marine debris. IMDOS will interact with other observing systems monitoring physical, chemical, and biological processes in the ocean and on shorelines as well as the state of the ecosystem, maritime activities and safety, drift of sea ice, etc. The synthesized data will support innovative multi-disciplinary research and serve a diverse community of users.
Introduction
In this paper, serving as a Community White Paper contributed to the OceanObs19 conference1, we present the concept of an Integrated Marine Debris Observing System (IMDOS) that will provide global coverage and accuracy, required for understanding the dynamics of marine debris and monitoring of changes in its distribution and effectiveness of mitigation of its impacts. IMDOS builds on previous initiatives (e.g., MSFD, 2013; GESAMP, 2019) to include into consideration a broad variety of debris and its complete life cycle in the marine environment, and aims to stimulate the establishment of best practices as well as optimization and expansion of the existing observational networks. We review the properties and impacts of different types of marine debris, as well as observation techniques and technologies that are used or could potentially be used in the next decade and beyond, and we share our vision of how direct observation, remote sensing, and numerical modeling can be integrated to compose a global observing system.
The World Ocean plays an integral role in connecting remote areas by transporting substances and materials over large distances and in between continents. This is not limited to transport of heat and chemical compounds that shape the climate of the planet, but also includes solid objects and particulate matter floating on or near the ocean surface or suspended in the water column, including dispersal of many forms of living organisms. This way, over millions of years, floating seeds have populated new volcanic islands, and kelp species have spread over large areas. Land-derived plant material, entering the ocean, provides a substrate and food for marine life, and sinking particles in turn support the diverse ecosystems on the ocean floor (Dupont et al., 2009). Introduction of durable synthetic materials, especially plastics, has dramatically increased the amount of marine debris. This increase has co-occurred with other natural and anthropogenic changes in the ocean, such as warming, sea level rise, acidification, and loss of biodiversity; and plastic pollution is one particular, and very visible, example of the lack of sustainability in our actions.
The advent of commercial mass production of plastics in the mid-twentieth-century, and the subsequent exponential rise in consumption to an estimated 407 million metric tons in 2015 (Geyer et al., 2017), resulted in 4.8–12.7 million metric tons of plastic waste entering the oceans in 2010 from land and an estimated 120 million tons cumulative total (Jambeck et al., 2015). Plastics comprise the majority of marine debris and represent its most durable and persistent part (e.g., 88% of samples analyzed by Cózar et al., 2014 were composed of plastics). In the absence of comprehensive international law regulating marine pollution, MARPOL Annex V2 was introduced in 1988 and has been signed by more than 150 countries to prevent ocean pollution with garbage from ships. However, it does not extend to land-based sources.
Now, as part of the Decade of Ocean Science for Sustainable Development (2021–2030)3, proclaimed by the United Nations, the Sustainable Development Goal (SDG) Target 14.14 aims to prevent and significantly reduce marine pollution of all kinds by 2025, including marine debris (or marine litter). Marine litter is also addressed in the voluntary Global Programme of Action (GPA) through the Global Partnership on Marine Litter5 (GPML), promoting the implementation of the Honolulu Strategy6 from 2011, and through the UN Environment Clean Seas7 campaign launched in 2017. These initiatives engage governments, the general public, civil society and the private sector to transform habits, practices, standards and policies in the fight against marine plastic litter. Sessions and resolutions of the United Nations Environment Assembly (UNEA, which encompass GPA) highlighted marine plastic debris and microplastics amongst the issues of global importance and moved to act toward the negotiation of a new international legally binding instrument.
The Implementation of the G7 Action Plan on Marine Litter8 mainstreams the work of the Regional Seas Programmes and includes capacity building and sharing of best practices, ongoing coordination with European Regional Seas conventions, strengthening the collaboration with GPA and GPML, and a contribution to the G20 Action Plan on Marine Litter9. The latter will be implemented through a voluntary Global Network of the Committed (GNC). The actions focus on the promotion of the socio-economic benefits of establishing policies on marine litter prevention, waste reduction and resource efficiency, waste/stormwater management, public awareness, education and research, remediation action and the promotion of stakeholder engagement. Under the presidency of Canada in 2018, the G7 countries agreed on a short list of seven main challenges in the areas of health and environment, and committed to move toward a more resource-efficient and sustainable approach to the management of marine plastic litter, working with industries toward 100% reusable, recycled, or recovered plastics by 2040. In support of these plans, the International Association for the Physical Sciences of the Oceans (IAPSO), the International Council for Science (ICSU), the International Union of Geodesy and Geophysics (IUGG), and the Scientific Committee on Oceanic Research (SCOR) published a document, providing a scientific perspective on these issues (Thompson and Maximenko, 2016).
To be effective, new regulations must be based on solid knowledge, and changes resulting from their implementation must be monitored comprehensively, accurately, and in a standardized way. Decades of scientific research have addressed many important aspects of the abundance, composition, and dynamics of marine debris, and plastic pollution in particular. The earliest scientific reports documented a variety of items in many marine habitats: industrial resin pellets and millimeter-sized fragments (Carpenter and Smith, 1972) and plastic bottles (Venrick et al., 1973) floating at the sea surface in the open ocean; synthetic fibers derived from marine netting and rope in filtered coastal water column samples (Buchanan, 1971); and items derived from food and beverage consumption, and fishing and boating activities on a beach near recreational waters (Cundell, 1973). A systematic chronology of marine debris research was well-described by Ryan (2015).
At the same time many questions remain unanswered. There are many reasons why measuring and understanding marine debris is difficult, including: (i) variety of object sizes (from tens of meters to microns) and shapes (e.g., spherical pellets, packaging films, fibers, and composite objects), (ii) complexity of chemical composition (e.g., different polymers, metals, glass, and organic materials), (iii) unknown sources and sinks, as well as (iv) pathways and (v) decay processes. Also, because different laboratories and groups use different methodologies to study marine litter, local observations are often hard to generalize into a global picture. For example, estimates of the amount of microplastic floating at the sea surface vary between 6,350 and 236,000 metric tons (Cózar et al., 2014; Eriksen et al., 2014; van Sebille et al., 2015). Note that even the highest estimate constitutes only 0.1% of the estimated total amount of plastic added to the ocean from land-based sources. Inclusion of estimates of other debris types (184,000 metric tons of larger plastic debris floating on the ocean surface; Eriksen et al., 2014), sinks (8,000 metric tons removed annually from the shorelines by the cleanup groups), and reservoirs (seabed, buried in beaches, water column, ingested by marine life, etc.) still leaves the mass balance of plastic debris open, with the fate of at least 90% of it unknown.
Impacts of Marine Debris and Importance of Their Global Monitoring
Marine debris has numerous impacts on the environment and society. Those influenced by these impacts, managing them professionally, or responding voluntarily are the potential users of the IMDOS. The global extent and growing magnitude of the impacts justifies the importance and urgency of the global observing system. It is also expected that increased knowledge of marine debris will reveal new impacts that are currently unrecognized. Most impacts are specific to the type of marine debris. People's activities increase the amount of natural material entering the ocean (such as driftwood from logging) and also introduce artificial materials that pose new threats to the environment.
Microplastics and Biological Interactions
Consumption of oil grows exponentially, with about 10% used to produce plastic, a large fraction of which is intended for single-use applications and/or packaging. Jambeck et al. (2015) estimated that trends in plastic input entering the ocean from land-based sources follow the trends in oil consumption. These human-made polymers can last in the marine environment for a significant time; during their lifespan plastic items can travel over large distances and accumulate in some areas in high concentrations. Weakened by ultraviolet light and broken apart by storms, larger objects gradually fragment into smaller and smaller pieces (Figure 1) that become increasingly accessible to different marine species. Pre-production pellets and microbeads are deliberately manufactured in small sizes. The abundance and impacts of the smallest plastic particles, nanoplastics, are not well-studied, but their demonstrated ability to penetrate cell membranes and accumulate in organisms raises great concern (c.f., Koelmans et al., 2015). Fish, seabirds, turtles, and other marine animals, mistaking microplastics for food, ingest them, which may cause physical injury, starvation and even death. After entering the food web, plastics may travel to upper trophic levels, resulting in bioaccumulation and biomagnification, but such trophic transfer is not yet well-studied (c.f., Provencher et al., 2018).
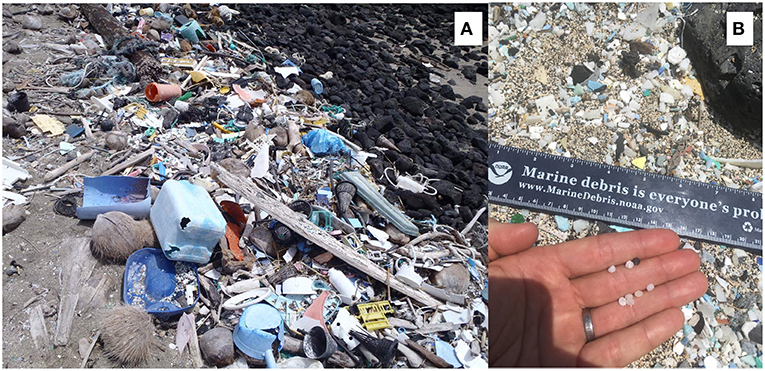
Figure 1. Plastic debris accumulation at Kamilo Point, Island of Hawai‘i. Large items (A) are often still identifiable, but gradually break into small fragments (microplastics, B). Photos courtesy of the 2011 IPRC project (A) and Hawai‘i Wildlife Fund (B) (Maximenko holds copyright on 1A and Lamson holds copyright on 1B).
Additives incorporated during production (such as colorants, plasticizers, stabilizers, antioxidants, flame retardants, UV absorbers, antistatic agents, etc.) make some plastics toxic; if ingested by organisms, these toxins may enter the food chain, posing a threat to ecosystem structure and function and possibly even to human health. Leaching of additives to the seawater may also pose ecotoxicological threats to the marine biodiversity. Toxins can be also adsorbed by the plastic when it moves through areas contaminated with industrial pollution or via natural events (e.g., such as “red tides”10). Because of their large relative surface area, nanoplastics may be most efficient transporters of these added or adsorbed chemicals (c.f., Galloway, 2015).
Derelict Fishing Gear
One of most abundant and conspicuous types of marine debris is derelict fishing gear. Used in large numbers, fishing nets are often lost during storms. According to Lebreton et al. (2018), they comprise 46% of the plastic mass floating in the upper ocean in the North Pacific Subtropical Gyre. The lifespan of these “ghost nets” is not known but as long as they float they continue killing not only fish, but also sea turtles, seabirds and other marine animals (Figure 2A). Washed ashore, partly buried in sand or stuck on rocks, nets are difficult to remove. Every year, fishing nets damage large areas of coral reef ecosystems: the Northwestern Hawaiian Islands alone collect on average an estimated 52 tons per year11, with 80 tons removed in 201812.
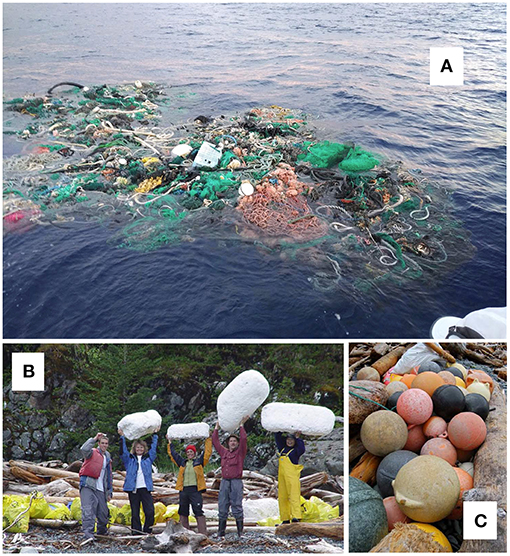
Figure 2. Derelict fishing nets (A) floating in the eastern North Pacific. Photo courtesy of Raymond McCormack, who tagged the net with a satellite tracker during the Ocean Voyages Institute's projects. Styrofoam (B) and plastic (C) fishing buoys, removed from the shoreline of Alaska. Photos courtesy of Christopher Pallister, Gulf of Alaska Keeper15 (Maximenko has permissions from McCormack and Pallister to use the photos).
While concentration of heavy floating fishing nets tend to be higher in the subtropical “garbage patches” or in other frontal convergence regions of the ocean, light floats or buoys separating from these nets or lost during other fishing or aquaculture activities are blown by the wind onto selected shorelines. For example, plastic and styrofoam buoys, used for fishing and in aquafarms in the western Pacific and Chinese seas do not only pollute Asian shoreline (Lee et al., 2013) but reach in large numbers the coast of Alaska (Figures 2B,C), where inaccessible terrain, sparse populations, short summers, and dangerous wildlife make cleanup very difficult. Winter storms break debris into tiny pieces and blow them inland, contaminating large areas of land. Similarly, crab and lobster tags arrive from North America on the coasts of Europe, and Styrofoam is one of major types of plastic debris in coastal waters of Chile (Hinojosa and Thiel, 2009).
Large Debris and Maritime Safety
In addition to small items that sneak through our waste management systems, marine debris also includes large items that carry a threat to maritime activity. The “All is Lost” movie that starts with a sailboat collision with a marine container is not a completely unrealistic story. The World Shipping Council estimated that in 2008-2016 there were on average 56813 containers lost at sea each year during normal operational activity (Figure 3A). This is a tiny fraction of millions of containers delivered safely to sustain the World's economy, but even one 40-foot object drifting without control can be tremendously dangerous. Its path and associated risks depend on the cargo inside the container, making predictions virtually impossible.
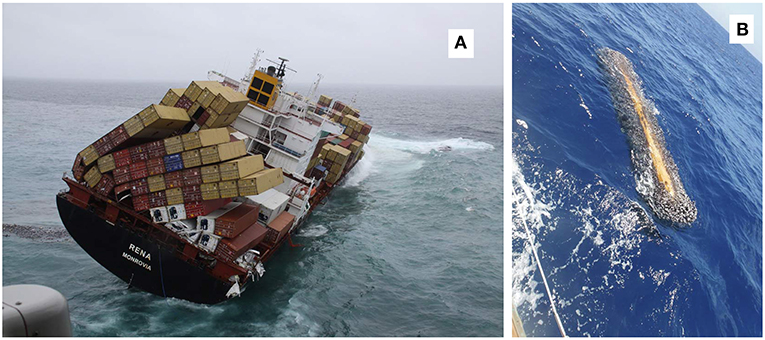
Figure 3. (A) The MV Rena lost an estimated 900 containers when it ran aground and broke up off the coast of New Zealand in October 2011 (Photo courtesy of Maritime New Zealand). (B) Heavily fouled driftwood, floating in the North Pacific (courtesy of Randal Reeves) (Maximenko has permissions from Maritime New Zealand and Reeves to use the photos).
Smaller objects, including large natural debris, are hard to document. For example, driftwood is abundant on many shores, but extensive data on its amounts in the open ocean are lacking. However, individual observations (Figure 3B) and collision reports14 indicate that the risk is real. In some regions encounters with fishing nets and entanglement of ship propellers also poses significant threat to navigation (Hong et al., 2017).
Debris on the Ocean Floor
The ocean floor is one of the main destinations of marine debris. Many materials are denser than water and sink immediately after entering the ocean. For example, the seabed near big cities, ports, and river mouths are often densely covered with whole PET bottles and other presumably recent debris (Figure 4) of local origin. Other types of debris or their parts float initially, but gradually lose their buoyancy due to biofouling and degradation, processes that may remove debris from the sea surface. Wooden debris, by being exposed to water may saturate and increase its density and sink. The ocean floor is difficult to study (section Direct Observations of Marine Debris provides more information about benthic observations). However, because the benthic zone is a very important and vulnerable part of the marine ecosystem, it is exposed to critical impacts of natural and artificial marine debris (c.f., Galgani et al., 2015).
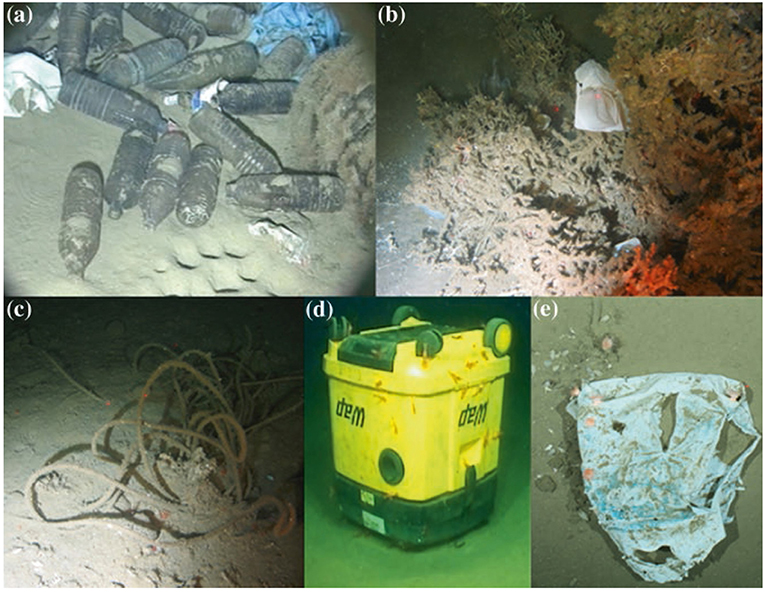
Figure 4. Litter on the deep seafloor. (a) Plastic bags and bottles dumped 20 km off the French Mediterranean coast at 1,000 m in close vicinity to burrow holes; (b) food package entrapped at 1,058 m in deep-water coral colony; (c) rope at 1,041 m depth, both from Darwin Mounds; (d) waste disposal bin or a vacuum cleaner with prawns on the seafloor off Mauritania at 1,312 m depth; (e) plastic carrier bag found at ~2,500 m depth at the HAUSGARTEN observatory (Arctic) colonized by hormathiid anemones and surrounded by dead tests of irregular sea urchins (adopted from Galgani et al., 2015 Galgani holds copyright of this figure).
Over centuries, a large number of shipwrecks, lost in accidents and combat or sunk intentionally, as well as dumped munitions and containers with chemicals, including toxic and radioactive materials16 have been also deposited on the ocean floor. These wrecks and dumpsites will sooner or later start leaking contaminants into the ocean; their retrieval or conservation and close monitoring are essential for the health of the deep ocean.
Biological Dispersal
“Floating macroalgae, wood and volcanic pumice have been part of the natural flotsam assemblage of the oceans for millions of years” (Kiessling et al., 2015). They provide not only a substratum for the pelagic ocean, but also facilitate the spread of new life to volcanic islands. Together with fouled ship hulls and ballast water, artificial marine debris opens new opportunities for long-distance travel and elevates risks of invasions of alien species, which are considered a major threat to coastal ecosystems (Molnar et al., 2008). Additionally, changing climate and ocean currents open up novel pathways for rafters (Miller, 2018).
Catastrophic Events
Natural disasters such as hurricanes, floods, and tsunamis, along with accidents created by human activity, can greatly increase inputs of all kinds of natural and artificial debris overviewed above. For example, when catastrophic events are considered, the number of marine containers lost at sea in 2008–2016 increased three times to 1,582 per year on average. According to the Japan Ministry of the Environment. (2012), the 2011 tsunami in Japan washed about 5 million tons of debris into the ocean within hours. Of this amount, 3.5 million tons sank on the shelf, severely damaging the benthic ecosystem and, together with the radioactive spill from the Fukushima nuclear plant, badly affecting the local fishing industry. The remaining 1.5 million tons (an amount close to a full–year input of land-based plastic debris for the entire North Pacific) became flotsam and a fraction of this drifted to North America and Hawai‘i. Four 20-meter floating docks, about 1,000 vessels (Maximenko et al., 2018), and other large objects posed threats to navigation and remote coastlines. At least 289 documented Japanese species (Carlton et al., 2017) were also transported over large distances due to this event, some of which are known as past invaders. Many near-shore mariculture farms were set afloat and produced large numbers of floats. The composition of tsunami debris was very complex: according to Murray et al. (2018), counts of all categories of debris, monitored on Washington state beaches, increased in 2012 by a factor of 10 compared to pre-tsunami levels.
Disasters and accidents often occur under conditions when limited resources, difficult access and emergency priorities do not allow for accurate documentation of the amounts of marine debris generated. For example, little is known about flotsam from the 2004 tsunami in Indonesia that caused 20 times more casualties than the tsunami in Japan. On a similar note, the Malaysian Airlines Flight 370 that disappeared in 2014 is still missing even though satellite engineers determined the approximate location of the crash, verified fragments were found on Reunion Island, Madagascar, and in Africa, their drift was studied in research projects (Trinanes et al., 2016) and 100 million Australian dollars were invested in the search.
Economics
Plastic plays an important role in the economy: every member of society benefits from this inexpensive material. Yet, the market price of the virgin plastics is only based on the low cost of plastic production and does not take into account the potentially much higher cost of its end-of-life processing and mitigation of its leakage into the environment. The consequences are often hard to estimate because they are coupled with other factors, and impacts are indirect, unproven, or even unknown. Newman et al. (2015) overviewed published studies that revealed high or significant costs of marine litter impacts on tourism and recreation, shipping and yachting, fisheries, and aquaculture. Generally, the data available for consolidation are rare. In the UK, the cost of professional litter removal is estimated at €7,000 per km per year (Mouat et al., 2010). Jang et al. (2014) linked more than 20 million dollars lost revenue in 2011 (compared to 2010) due to increased litter on Goeje Island beaches. Leggett et al. (2014) estimated that a 75% reduction of marine debris at six popular beaches of Orange County, California generated 40 million dollars benefit in just 3 months. Fisheries, shipping industries, navy, and aquaculture lose time and money by cleaning marine debris from their nets, ship propellers, cooling intakes, and farms.
Much higher risks and expenses are associated with potential future degradation of ecosystems, reduction of food production, and human health issues. The role of marine debris in these impacts is yet to be quantified. IMDOS will provide such data, without which such quantification is impossible. A recent study by Royer et al. (2018) also suggests that aging plastics release trace gases that contribute to the greenhouse effect. This impact may be significant because of its delayed effect and because it can be localized to areas (such as the seabed) where other sources are weak. Plastic contamination is one of the multiple stressors on the ocean that is projected to increase as the human population grows17.
IMDOS Stakeholders
IMDOS will provide data to individuals, organizations, and governments dealing with the surveillance, impact management and mitigation of marine debris, including but not limited to the following groups:
• Policy and decision makers. The long-term resolution of the problems of anthropogenic debris will likely be achieved through integrated local, national, and international laws, balancing rewards and enforcement. IMDOS will provide knowledge for their development and tools to monitor their implementation. Such a system will have a strategic role in supporting the international agenda on the oceans, including reporting to the SDG 14.1 at different but complimentary scales.
• Management of relevant marine environments. Local agencies and companies will have first-hand information on risks, “hot spots” and “extreme events” associated with marine debris spills, improving strategies to locate and remove litter from shorelines, diving sites and Marine Protected Areas.
• First responders. IMDOS will inform about abnormal amounts of marine debris generated by disasters and accidents, and determine risks and influenced areas. It will also track large objects and ensembles of debris that will help with search and rescue operations and prevention of subsequent collisions.
• Scientists. With the current general understanding of sources, pathways, processes, and impacts related to marine debris, quantitative description is very poor; amounts, fluxes, and densities, estimated in different studies, often disagree by orders of magnitude and fate of as much as 90% of plastic debris is not known. Marine physicists, biologists, ecologists, chemists, and climate scientists will use the IMDOS data in their research, to reduce these uncertainties to both understand marine litter dynamics and its interaction with other oceanic processes.
• Businesses. Industries affected by marine debris (such as fisheries, tourism, etc.) will use IMDOS to plan preparedness and mitigation of potential impacts. The marine debris problem also opens new opportunities for inventions and new technology development. Given the co-responsibility of the private sector in generating marine litter (e.g., plastic pellets), they will also be able to inspect the effectiveness of implemented mitigation measures (e.g., Operational Clean Sweep, Circular economy). IMDOS will help high-tech companies to use marine debris information as a resource and to monitor impacts of new technologies on the environment.
• Environmental activists and citizen scientists. Plastic pollution has mobilized millions of concerned citizens, who are working to raise awareness, push legislation and, when possible, to clean the most polluted areas. Using IMDOS will strengthen their arguments, avoid biases and misunderstandings, and optimize their field work. In addition, IMDOS will offer a platform to consolidate and share validated marine litter data produced by citizen scientists.
• Educators. IMDOS will help to promote a more holistic view of our planet by raising population awareness of the consequences of our actions as a civilization and our responsibilities for personal and collective decisions.
Structure of the Integrated Marine Debris Observing System
An effective global IMDOS will make use of a variety of components, collecting in situ observations and sensing the environment remotely. It will be augmented by numerical models and theoretical analyses.
The structure of the IMDOS will be designed to provide best possible accurate estimates of variables required by the users, such as the SDG 14.1 indicators and sub-indicators under development at UN Environment (GESAMP, 2019). The list of variables includes, but is not limited to: concentration, composition, origin and pathways of marine debris. Concentration (abundance and mass) data will highlight patterns of debris distribution, identify areas of accumulation, and monitor temporal variability and trends. IMDOS will help identify main sources (i.e., varying both geographically and possibly in terms of human activities) and quantify inputs and ways debris enters the marine environment, estimate total amounts residing in different reservoirs [such as upper ocean, water column, seabed, shoreline, etc. (Hardesty et al., 2017; GESAMP, 2019)], close the gaps in the budget of marine litter as a system, and characterize connectivity between different regions. Distinct types of debris come from different sources, are transported along different paths and have different impacts on the environment and people's activity (e.g., Pieper et al., 2019). Data on the composition of debris will help scientists choose the right set of instruments for its monitoring, optimized for a given region.
A key goal of IMDOS is to monitor and assess the risk posed by marine debris. This risk can be distilled as a superposition of: (1) exposure or concentration; and (2) vulnerability of—or harms to—the system. Large knowledge gaps exist for both of these risk-determining variables. For example, in terms of exposure, it is still not clear among the scientific community how precise a measurement should be such as to accurately estimate the amount of debris in a region, and to calculate the fluxes of debris in and out of that region. All of these demand more scientific studies, but once risks of environmental impact of marine debris can be computed in IMDOS, these combined with data from other observing systems (e.g., systems monitoring the ecosystem, biodiversity, climate and/or maritime activity, and safety), can allow for the diagnosis of extreme situations, can support the mitigation and/or response to these risks, and can help to assess the effectiveness of those responses.
Direct observations of marine debris will provide “ground-truth” information and will be the most critical component of IMDOS. Different instruments will be used to measure distinct variables and characteristics of debris types. Abundance of debris will be monitored in all parts of the Earth hydrological system, including surface, water-column and seafloor compartments of the open ocean, coastal areas, shoreline, sea ice, lakes, rivers, streams and watersheds. To monitor changes in debris density, spatial patterns, and composition, IMDOS will include a set of permanent sites and repeat sections in the main areas of debris accumulation. IMDOS will have a global spatial coverage and resolution sufficient to describe large-scale patterns of debris distribution. While one-time observational and regional campaigns may also provide important insights, the core of IMDOS will be designed for multi-year functionality, with gradual enhancement driven by national monitoring capacities, developing technologies, gained knowledge and changes in the ocean circulation, climate, and ecosystem. To provide data for calibration/validation of satellite sensors, a part of in situ observations will be located along satellite tracks and maintained for the lifetime of these satellite missions. The regional structure of in situ observations will be adjusted to best cover regional sources of debris as well as the areas most affected by local and remote marine debris. To increase the volume of high-quality observational data, new instruments and techniques will be developed and used in collaboration with governmental organizations, citizen scientists, volunteers, and ships and aircraft of opportunity. Crowdsourcing will be used to establish better connections to various groups worldwide and to collect reports on extreme events, such as accidental spills or unusual floating objects. Techniques will be developed to validate and synthesize low-level data (such as photographs or even verbal reports) with products of IMDOS. This in situ observational component of IMDOS is discussed in more detail in section Direct Observations of Marine Debris.
Remote sensing will fill gaps between sparse in situ observations (an example of the present coverage is shown in Figure 5A) and provide nearly uniform, nearly global coverage over long time scales. Spatiotemporal scales, resolved by IMDOS, will include scales of important sources and accumulation zones of marine debris and their variability as well as scales of physical processes controlling the dynamics of debris (such as oceanic gyres, fronts, eddies, seasonal cycle, El Nino—Southern Oscillation, Pacific Decadal Oscillation, etc.). Remote sensing will extend into such remote, unpopulated areas as the Arctic and Southern Oceans, as well as coastlines of uninhabited islands (Lavers and Bond, 2017). Calibration/validation of remote sensors will be conducted through comparison with data from a network of dedicated in situ observations and specialized models. To enhance regional monitoring, with higher spatiotemporal resolution, remote technologies will be implemented on suborbital missions using drones and small aircraft. Portable, robust, automated instruments, using remote sensors, will be used on ships and airplanes of opportunity as well as by citizen scientists and volunteers to sustain a cost-effective web of additional observations. The remote sensing component of IMDOS is discussed in more detail in section Remote Sensing of Marine Debris.
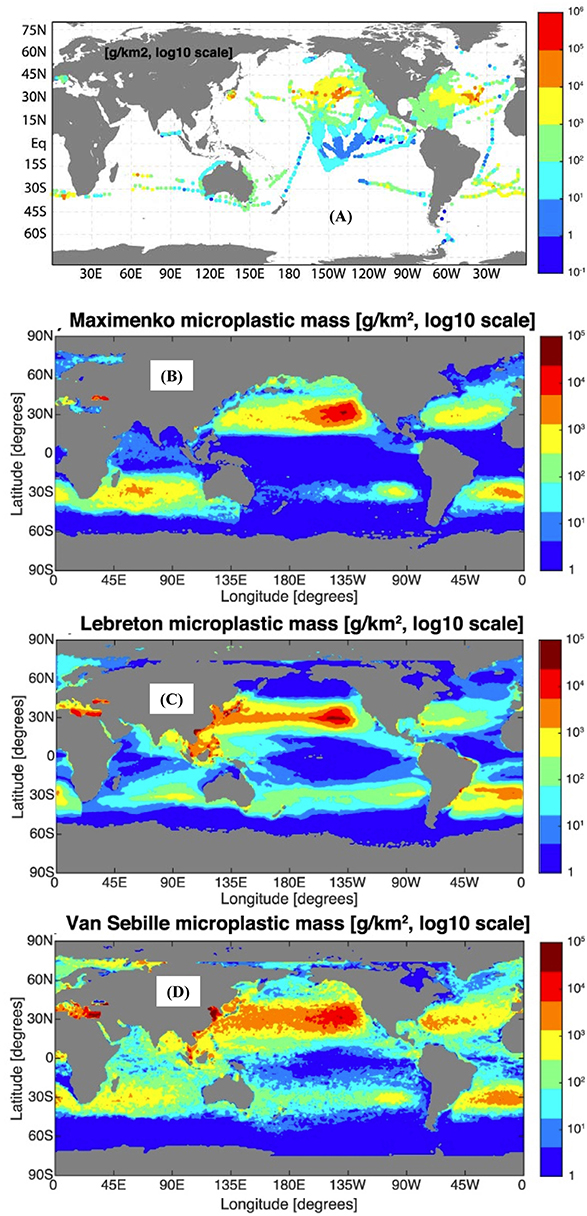
Figure 5. (A) Density of microplastics near the ocean surface in the global dataset and (B–D) three model simulations [adopted from (van Sebille et al., 2015)] (Van Sebille holds copyright on this figure).
Like any effective observing system, scientific analysis and numerical modeling will play important roles in all stages of IMDOS design, implementation, and data analysis. Among the advantages of numerical modeling is that dynamical models can provide a global perspective on extremely sparse observations and fill the regions between the observations with an “educated guess” (e.g., Figures 5B–D; also, Hardesty et al., 2017). This extrapolation will be especially relevant in areas where direct observations are difficult to make, such as the high Arctic region (Cózar et al., 2017; Peeken et al., 2018).
Moreover, dynamical models can be used to better understand the processes that affect transport, fate and distribution of marine debris. For example, the hypothesized sinking and rising of plastic particles due to biofouling and possible subsequent remineralization at depth (Kooi et al., 2017) will be extremely difficult to observe, but its large-scale effect on the horizontal distribution of marine plastic can be modeled.
A possibly even more important task for modeling is to underpin Observing System Simulation Experiments (OSSEs), where the design of the large-scale structure of the IMDOS can be fine-tuned with the aid of simulations. OSSEs are a mature and proven methodology for effective design of observing systems given a realistic “nature run,” but in the case of marine debris the difficulty will be that the simulated distribution of debris can be orders of magnitude different between models (van Sebille et al., 2015). How to deal with this large inter-model uncertainty in the scope of OSSEs will require further research.
Over the last decade or so, a number of different models for marine debris have been developed. These range from statistical observation-driven Markov models (Maximenko et al., 2012; van Sebille et al., 2012), to highly idealized mathematical models (e.g., Koelmans et al., 2017), to full-blown two- and three-dimensional particle tracking models (Lebreton et al., 2012; Robinson et al., 2017).
While designing an IMDOS with uncertain models will be challenging, the fact that these models are so poorly constrained is a primary motivation for the IMDOS in itself. The high sensitivity to unknown (or vaguely known) parameters in sets of model experiments will be used as guidance for upgrading the initial IMDOS with additional, targeted observations. Examples of these include fragmentation, biofouling, and sinking rates of marine plastic particles.
Once operational, the models will then be used to estimate the overall performance and efficiency of the observing system as well as to indicate the degree to which the whole dynamics of marine debris are understood and monitored. As such, the planning and operation of the IMDOS go hand in hand with the development of the models.
Specialized studies may be necessary to understand poorly-known processes responsible for changes in debris properties, degradation, and interaction with the ecosystem, economics, and other factors. These studies will be designed to improve the products but will not become a permanent part of the IMDOS.
Some model uncertainties reflect gaps in the current state of knowledge or in critical ocean observations. For example, surface currents are one of the most complex components of the ocean dynamics, presently not measured by satellites directly, but derived from other remotely sensed variables (sea level, winds, temperature, etc.), using either relatively coarse ocean global circulation models (OGCMs) or simplified mixed layer models18. The development of IMDOS, with its emphasis on debris drift on or near the ocean surface, will motivate development and improvement of technologies of observations of ocean currents, waves and surface winds and their effects on movement of debris (e.g., Fraser et al., 2018; Putman et al., 2018), while objects of dimensions upwards of a few cm are subject to inertial forces that scale with mass of the object (e.g., Brooks et al., 2019).
The overall structure and operation of IMDOS will be intrinsically based on the scientific analysis of existing observations and models. The efficiency of IMDOS will be achieved by focusing on variables demanded by end-users of the data products. Because (based on our experience) the majority of users have local and regional interests, the observing system will have an important focus on regional products while maintaining global coverage.
IMDOS will build on the positive experience of such successful programs as the Global Ocean Observing System (GOOS)19 and, after a setup period, may become a part of the GOOS. International groups of experts will maintain the list of Essential Ocean Variables and discuss the effective methodologies. Data centers will be set up for producing, managing, and distributing quality-controlled data and near real-time products. These data centers will work together to develop and implement observational methods and techniques, providing uniform format and accuracy of observations. They will also work with satellite mission science teams and modelers to fully use the wealth of information into gridded products useful for various applications. Synthesis of heterogeneous data (such as counts, images, and coarse satellite indices) will require development of new advanced methods such as those using machine learning. Interaction with users will be improved by involving them in the voluntary data collection process as boats of opportunity or coastal observers.
Direct Observations of Marine Debris
In order to characterize the abundance of different types of marine debris, a variety of observing platforms are required. Each of these have advantages and disadvantages in terms of the level of temporal and spatial resolution they can provide, the sensors and samplers they can carry, debris types and size ranges they can cover, and the physical parts of the marine environment from which they acquire data. Until appropriate sensors become available and provide sufficient temporal and spatial coverage, samplers will be broadly used for “point” observations. At the same time, only samplers provide “ground truthing” in its entirety and complexity. It was the collection of samples that raised concern for the trends of plastic debris in the marine environment. Samples are also irreplaceable in process studies investigating biological interactions, chemical degradation, and changes in the chemical composition of debris over time.
Platforms
The following platforms are used for marine debris observations (Figure 6).
• Satellites, Aircraft, and Drones. Development of advanced sensors makes the boundary between “direct” and “remote” observations somewhat fuzzy. Presently, aerial surveys and remote technologies (described in section Remote Sensing of Marine Debris) provide rich information on debris at the ocean or shoreline surface (e.g., Lecke-Mitchell and Mullin, 1992). As the major part of marine debris is submerged in the ocean or buried in marine sediments (e.g., van Sebille et al., 2015; Koelmans et al., 2017; Chubarenko et al., 2018), direct measurements remain critical for comprehensive monitoring. In a comprehensive discussion of sensors applicable in monitoring of hazardous materials and organisms in the marine environment Zielinski et al. (2009) present how different measurement platforms can contribute to the depth of knowledge at varying geo-spatial and temporal scales.
• Ships. Ships are traditionally used to collect data on marine debris floating on or near the surface. Ship observations are sparse, vulnerable to the weather conditions, and often sensitive to the type of ship and expertise of the operator. Nevertheless, they provide a platform from which a broad variety of sensors and samplers can be used for comprehensive study of the entire water column, from seabed to the surface. With the small number of research vessels, ships of opportunity have a great potential to greatly improve coverage by increasing the number of visual observations and using ship-borne autonomous systems (e.g., Ferrybox)20.
• Autonomous platforms. Floats, gliders (both seagliders and wavegliders), and Autonomous Surface and Underwater Vehicles (ASVs and AUVs), equipped with adequate sensors can provide unique measurements in hard-to-reach parts of the ocean. As a rule these platforms have insufficient space for samplers and are often not recovered. The expanding Animal Telemetry Network21 can be also used for marine debris data collection as well as to monitor the interaction of debris with marine life. Lagrangian platforms may play an important role in tracking marine debris and understanding its pathways. The Global Drifter Program22 (Niiler, 2001) maintains a network of ~1,400 active satellite-tracked drifting buoys reporting hourly (Centurioni, 2018), and an archive of more than 30,000 drifter-years of historical trajectories, used in many studies (e.g., Lumpkin et al., 2012; Maximenko et al., 2012; van Sebille et al., 2012). Satellite trackers, attached to large debris (such as fishing net in Figure 2A) facilitate their retrieval from the ocean and reduction of associated hazards for maritime activities and coastal businesses. Specialized Lagrangian tools are developed to study drift of debris and other pollutions in focused regional projects. For example, Meyerjürgens et al. (2019) built compact surface drifters and used them on the southern North Sea shelf to study the transport patterns in the nearshore zones and the beaching-refloating dynamics.
• Fixed Point Observatories. These are important and efficient platforms to monitor temporal variability and, in particular, long-term trends of the problem. Moored platforms have the great advantage of being able to carry sensors and samplers. At present, there are about 120 open ocean observatories (OceanSITES)23 and even higher numbers of coastal and shelf observatories that can be used for marine debris observations. Some of the observatories are cabled24 and can produce a large volume of real-time data. Choice of locations of sites optimal for marine debris trend observations as well as interpretation of the time series, contaminated with the spatial signal will require coordination with satellite observations and numerical models.
• Benthic landers and crawlers. A range of devices have been developed which remain on the seabed for protracted periods of time some of which photograph the seabed repeatedly (e.g., Lampitt and Burnham, 1983; Lampitt, 1985) or can take time series sediment samples when crawling over the seabed (Purser et al., 2013). These provide unique information about the arrival of debris on the seabed and the interaction with this rather different benthic ecological community.
• Shoreline monitoring and Beachcombing. The highest known concentrations of marine debris are reported from selected shorelines, including both anthropogenic debris25 (Figure 1) and natural materials such as driftwood (e.g., in the background of Figure 2B). Societal concern has promoted a massive beachcombing activity. In 2017, the International Coastal Cleanup26, including more than 0.5 million people worldwide, removed more than 8,000 tons of artificial debris. Despite patchy geographical distribution and sparse timetables, cleanups have great potential of crowdsourcing qualitative and quantitative marine debris data through coordinated surveys using approved protocols [e.g., NOAA's Marine Debris Tracker27, Litterati28, and JRC Floating Litter App (González-Fernández and Hanke, 2017)]. Easy-to-access shorelines facilitate citizen science initiatives (GESAMP, 2019), can be used for sampling and monitored with beach cameras (Kako et al., 2010).
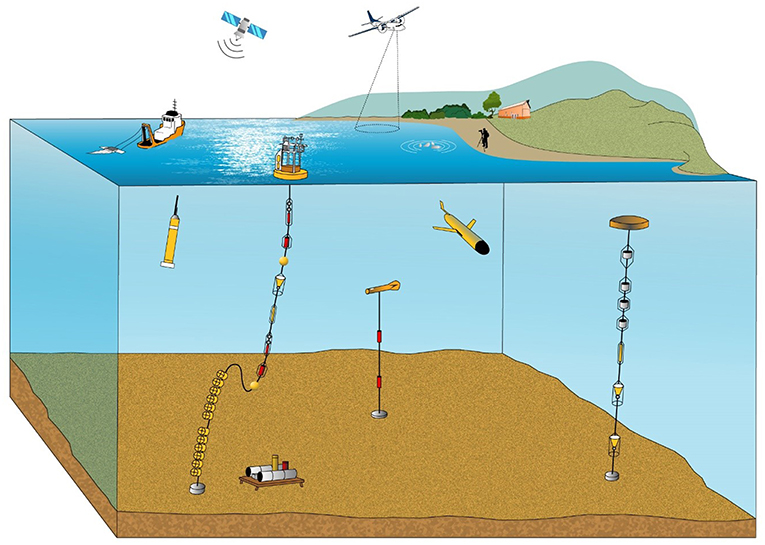
Figure 6. Schematic of the various observation platforms currently in use and on which a variety of sensors and samplers can be carried (Courtesy Kate Davis) (Lampitt has permission to use this figure).
Sensors and Samplers
A combination of samplers and sensors is used for direct observations of marine debris.
Sensors
While items at the sea and land surface can be detected and classified using remote sensing (as discussed in section Remote Sensing of Marine Debris), the development of autonomous and field deployable sensors for marine debris including microplastics remains a significant research challenge. Some field-deployed deep capable systems carry sensors and combine them with sampling and post-retrieval analysis (Wang and Wang, 2018): the sensors in this case are only used to trigger sampling and to provide environmental context (Edson and Patterson, 2015). Other imaging systems developed for macrofauna or microorganism studies can also be used to study plastic debris. For example, a towed camera and human assisted semi-automated image analysis (BIIGLE–Bio-Image Indexing and Graphical Labeling Environment–database) has been used to track increases in debris 2004–2014 in the eastern Fram Strait (Bergmann and Klages, 2012; Tekman et al., 2017). For microplastics, many of the techniques used in the lab (e.g., see Wang and Wang, 2018) are being semi-automated and can be applied in situ after developing the necessary engineering / instrumentation. For example, staining with the dye Nile Red (Shim et al., 2016) results in discrimination of plastics from other particles using fluorescence excitation and emission in the visible range (Maes et al., 2017): a spectral range that can be implemented in situ. Whilst chitin and some other organic matter is also stained, these particles may be discriminated using other means, e.g., by density (Maes et al., 2017) or digestion (Erni-Cassola et al., 2017). This technique has been semi-automated using image analysis software (Erni-Cassola et al., 2017). Full automation will require in situ filtration and image capture and likely separation or digestions steps which though onerous are not beyond the capabilities of in situ instrumentation (e.g., Scholin et al., 2017). Popular and powerful direct hyperspectral/FT-IR techniques used on filtered samples (e.g., Karlsson et al., 2016) and being increasingly automated may be problematic in situ due to the optical absorption of water in preferred spectral ranges (e.g., 1,000–2,500 nm, Karlsson et al., 2016). However, Raman spectroscopy has previously been applied in situ for other applications (Kirkwood et al., 2013; Peltzer et al., 2016; Guo et al., 2017; Jing et al., 2018; Li et al., 2018), and for microplastics can be operated in a spectral range with low absorption in water (e.g., 785 nm laser, Frere et al., 2016). Imaging (Nelson et al., 2018) and flow cytometry (Sgier et al., 2016; Long et al., 2017) can also be used, currently in the lab to analyze small plastic particles. Indeed cytometry is calibrated using plastic microbeads. In situ flow and imaging cytometers have been deployed (Dubelaar and Jonker, 2000; Dubelaar and Geerders, 2004; Lambert et al., 2017; Olson et al., 2017; Sosik, 2017), and an intriguing possibility is the reduction in size and power of deployed systems using microcytometers (Benazzi et al., 2007; Barat et al., 2012; Spencer et al., 2014, 2016; McGrath et al., 2017). Focused and significant effort is required to turn these opportunities into mature sensor technologies that can address operational metrology of marine debris across a wide size range in the marine environment.
Samplers and Subsequent Analytics
Beach litter surveys, using standardized protocols, are the simplest sampling technique, in which all analytical methods can be applied in order to deduce debris characteristics, including composition and possibly even origin. For smaller particles of debris found in the water, plankton nets of various types have frequently been used, some focusing on the surface layer (Neuston nets, Eriksen et al., 2018), and all usually with mesh sizes >250–300 μm. These techniques are relatively inexpensive and easy to operate but they may be missing a significant mass fraction of plastic debris associated with smaller particles. Direct measurements are needed to derive the size distribution to assess the missing mass. Presently, smaller particles have been sampled using in situ and deck-mounted filters with pore sizes down to 0.5 μm, which addresses the smaller size classes very effectively. However, such techniques are expensive demanding specialist equipment and operators.
Depending on their composition and degree of biofouling, many common microplastics are less dense than seawater so tend to float at or near the ocean surface, and as such may exhibit high concentrations in the upper ocean relative to deeper water. Manta trawls are used near the surface and a number of methods are available for sampling in the 1–1,000 μm surface microlayer, including rapid and facile glass plate sampling methods, which collect samples that contain relatively small amounts of sediment and biogenic material, facilitating subsequent microplastics identification (Anderson et al., 2018).
Sediments are typically sampled using corers such as the multicorer, which retains the sediment/water interface (Barnett et al., 1984). The separation of anthropogenic debris from naturally occurring material is a challenging task and significant research is still required to perfect techniques, particularly for micro-debris.
Biota is an additional and relevant source of information to estimate litter distribution and exposure to biodiversity and humans (GESAMP, 2019). It may also be used to validate models since the greater amount of micro and nano-particles in the tissues reflects a more chronic instead of episodic contamination in the surrounding environment. Particles in the digestive tract are also highly informative on the risks of trophic transfer and impacts in the ecosystem. However, as sediment samples, biotic sample processing and analysis still demand improved inter-calibration between laboratories.
Sample Contamination and Intercompatibility
In terms of sample analyses, a range of techniques has been used often with unknown precision and specificity, which frequently leads to data of unknown quality. A key aspiration for the coming decade is that rigorous and standardized analytical techniques are developed so that data are intercomparable between laboratories and in order to determine temporal and spatial variability.
Sampling of the smaller size categories of debris and particularly nanoplastics and fibers (due to the relative ubiquity of microparticles in clothing, buildings, etc.), has to date been frequently characterized by poor protocols which will have generated large numbers of contaminated samples. In order to obtain adequate data on which to base decisions, the coming decade must be characterized by high principles of “best practice.”
Remote Sensing of Marine Debris
The remote sensing of marine debris, in particular plastic pollution, is in its infancy (Maximenko et al., 2016a,b; Garaba et al., 2018). Despite encouraging results of first experiments with detection of large floating items (e.g., Acuña-Ruz et al., 2018; Garaba et al., 2018; Topouzelis et al., 2019) overall marine debris monitoring represents a significant technological challenge. Successful remote sensing can fill gaps between sparse in situ observations and provide uniform coverage over large areas and long time periods. Quantifying how much and where debris enters the marine environment over time is critical to formulate and evaluate proper responses but on a global scale has, so far, only been roughly estimated (Jambeck et al., 2015). Global coverage is particularly important because some artificial marine debris such as plastic can travel over long distances and accumulate over time. Satellites can also help to survey remote, hard-to-reach areas, in which direct observations are sparse and difficult. Because of the huge diversity of chemistry and geometry of different types of debris, no single sensor can see it all. A list of instruments is presented to highlight those currently available on in situ, air- and space-borne remote sensing platforms that have potential applications relevant to the aims of IMDOS (Table 1). A number of these sensors have already been tested in past missions but each sensor has observational limitations related to spectral resolution, spectral range, sensitivity, revisit time, geo-spatial resolution and coverage. To this end, an integrated sensor system combining sensors of different capabilities on different platforms is needed to advance future operational remote sensing efforts of marine debris especially in remote areas of the global oceans (Zielinski et al., 2009; Garaba and Dierssen, 2018).
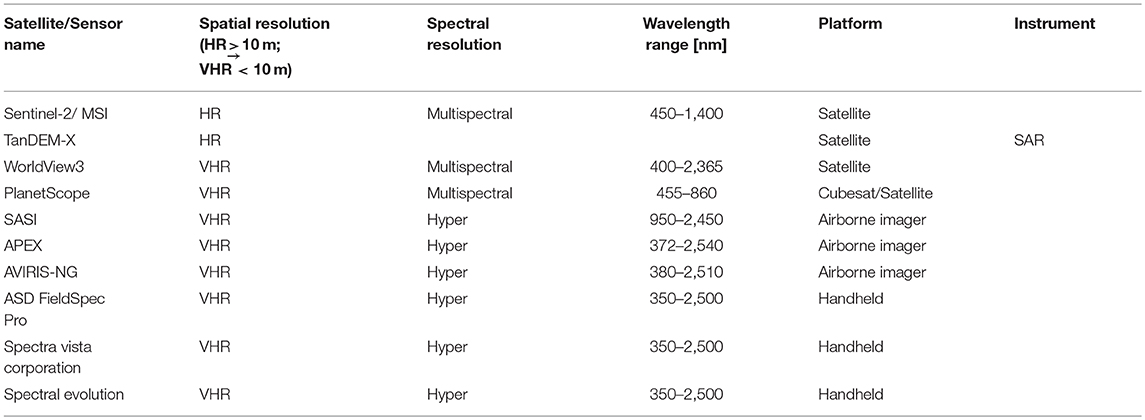
Table 1. Examples of actual sensors already used for detection and/or polymer identification of plastic debris.
High Spatial Resolution Imaging
Washed ashore, floating and slightly submerged marine debris has been monitored using high-resolution cameras on fixed platforms (Kako et al., 2018), shipborne (Hanke and Gonzalez-Fernandez, 2014), airborne (e.g., Veenstra and Churnside, 2012; Kataoka et al., 2017; Garaba et al., 2018; Martin et al., 2018; Moy et al., 2018) and satellites (e.g., Matthews et al., 2017; Topouzelis et al., 2019). Generally this technique is applied to the visible spectrum (400–700 nm) by making true color RGB composite images. Visible images have been used, for example, to study the dynamics of rafts of marine debris generated by the March 11, 2011 tsunami that devastated the coastline of eastern Japan (Figure 7, Matthews et al., 2017). The key requirement for the imaging technique is high spatial resolution sufficient to not only detect flotsam but desirably also identify it as a particular type of debris or specific object. While military satellite technology is capable of providing higher resolution, commercial products are limited to 25–50 cm, restricting their utility to several-meters-sized objects. Identification of smaller objects is feasible in suborbital missions.
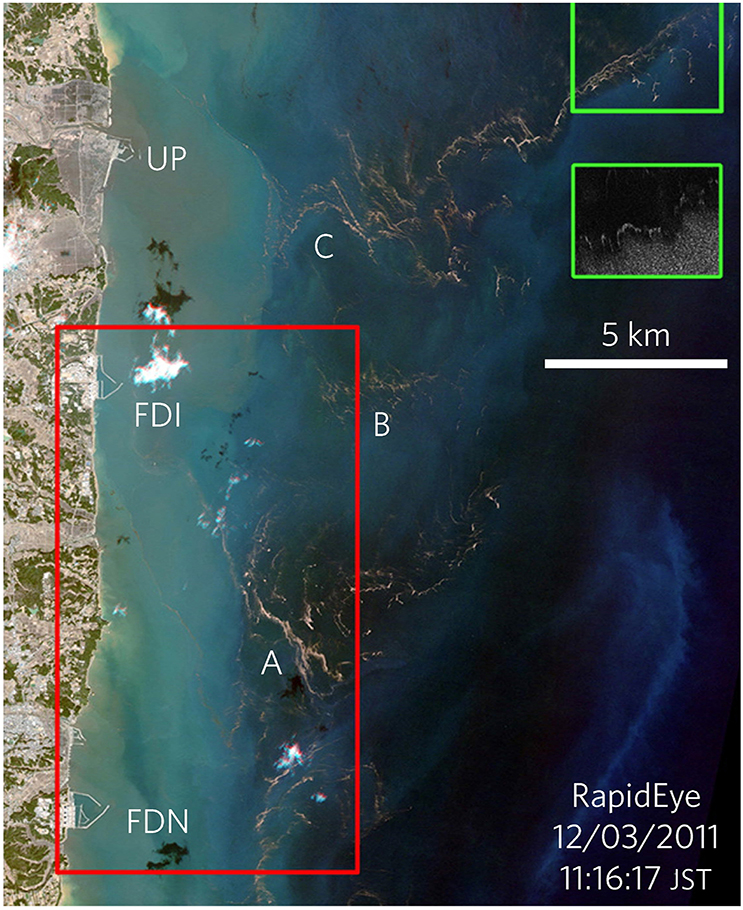
Figure 7. RapidEye image of debris, floating east of Japan 1 day after the March 11, 2011 tsunami showing Ukedo Port (Port), Fukushima Daiichi (FDI) nuclear power station and Fukushima Daini (FDN), floating litter A–C visible on 12 March 2011 [Adopted from (Matthews et al., 2017)] (Maximenko has permission from Nature Geosciences to use this figure).
Analysis of visible images requires advanced interpretation techniques to eliminate environmental perturbations from ocean bright targets (breaking waves, white caps, sea foam, surface reflected glint), clouds and cloud shadow (Matthews et al., 2017; Garaba and Dierssen, 2018). The unwanted effects can be mitigated by capturing a series of images with intervals of several seconds and at an optimal viewing geometry. True color RGB images provide crucial complementary information in monitoring marine debris about the apparent color and shape of litter that can be used to, for example, discriminate man-made objects from marine organisms, such as kelp or whales. However, the RGB images do not provide information on the physical and chemical composition of the litter. The potential of new “machine learning” techniques, combined with hyperspectral information, demonstrated recently by Acuña-Ruz et al. (2018) in their pilot study on the shoreline, will increase the value of high-resolution visible imaging in the future. Costs related to managing “big data” might be an operational challenge for such very high temporal and geo-spatial resolution RGB imagery. Efficiency of visual observations can be optimized through interaction with other components of IMDOS, used to identify “hot spots,” and in concert with other observing ocean systems, such as weather forecast and disaster/accident warning systems.
Optical Spectro-Radiometric Techniques
Spectro-radiometric analysis from the ultraviolet to the far infrared spectrum has opened new avenues for detection and characterization of plastic and other types of marine debris. Garaba and Dierssen (2018) demonstrated the existence of unique spectral absorption features in the near infrared (NIR) and short-wave infrared (SWIR) spectrum using washed ashore macroplastics harvested from the west coast of the USA (Figure 8). These absorption features were found to be insensitive to the object size (even in the marine-harvested microplastics from the North Atlantic and Pacific waters), apparent color or polymer type of plastic particles, suggesting that these features have potential applications in remote detection of ocean plastics under various backgrounds, including vegetation. Spectral information from multi- and hyperspectral optical sensors can be used to infer the abundance of plastic objects of a subpixel size. In the infrared spectrum plastics have identifying spectral signatures with a higher reflectance signal when compared to the ocean, which is a dark target with a very low nearly flat reflectance. However, this approach should take into account recent substantial increases in the quantity of pelagic Sargassum (e.g., since 2011 in the tropical Atlantic, Gower et al., 2013) also characterized by high reflectance in the NIR. It is therefore possible to detect the reflectance of floating ocean plastics, but this will depend on sensor capability as even shallowly submerged objects might be completely masked by water, a strong absorber of light in the SWIR spectrum. Although the relationship between degree of submersion and reflectance properties of plastics has not been fully investigated, it has been shown spectral reflectance shape of the samples was retained but the magnitude of the reflectance was lower for wet samples compared to dry samples (Garaba and Dierssen, 2018). A combination of drones and satellite imagery was recently experimented with to detect typical household items as floating plastic targets. Topouzelis et al. (2019) confirmed that floating plastics are seen from space as bright objects and demonstrated the benefits of using very high (~0.02 m) geo-spatial resolution imagery from drones to improve geo-referencing of Sentinel 2 data, resampled to 10 m resolution.
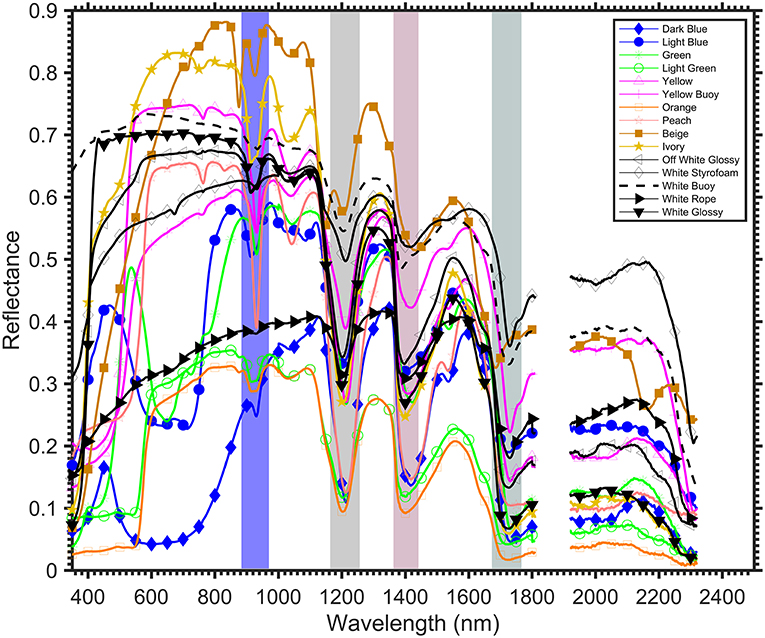
Figure 8. Reflectance of washed ashore macroplastics from beaches along the west coast of the USA reveals spectral absorption features at ~931, 1,215, 1,417, and 1,732 nm (shaded) that are fairly consistent across the variety of plastic objects (adopted from Garaba and Dierssen, 2018) (Garaba holds copyright on this figure).
Spectral observations provide a wealth of information that can be applied to algorithms that are key to inferring abundance of marine debris, polymer types and degree of weathering or degradation relating this to the possible age of debris. To become useful, large spectral libraries of different types of debris as well as inorganic substrates and biological environment need to be established (e.g., Garaba and Dierssen, 2017; Kokaly et al., 2017). Because marine debris attracts all kind of organisms, from bacteria and algae to fishes, separation of the signals may be challenging but, if successful, will provide a very important characterization of the impact on the ecosystem. Additionally, atmospheric correctional approaches (such as the “black pixel assumption” approach which assumes that in the open ocean the signal in the NIR is negligible since the ocean is a dark target) for satellite and airborne observations need to be revised to account for the contribution of floating plastics to the bulk water leaving signal. More recently, the use of SWIR at-sensor radiance was presented showing promising results in the airborne detection, quantification and characterization of floating ocean plastics (Garaba et al., 2018). Goddijn-Murphy and Dufaur (2018) and Goddijn-Murphy et al. (2018) developed and verified an optical model of sunlight hitting a water surface, littered with floating plastic, that describes how inherent optical properties of plastic items (such as transparency) affect reflectance in the visible to SWIR spectrum.
A prospective technique to observe submerged debris is active remote sensing using a light detection and ranging system (LIDAR) that can measure the onboard laser lights backscattered from the ocean. Alternative approaches with potential application are based on fluorescence and Raman spectroscopy although the latter operates with a low signal that is presently challenging to detect from current satellites missions.
Radar Sensors
Radars are active sensors that transmit an electromagnetic pulse and measure the signal as scattered by the scene, in this case the water surface. Radar sensors are broadly used to measure such essential ocean variables as sea surface topography (and thereby geostrophic currents), wind-speed and direction, waves as well as whitecaps and sea ice coverage and for oceanic surveillance.
With respect to marine debris, one of most promising radar technologies is synthetic aperture radar (SAR), whereby the forward movement of the platform (aircraft or satellite) is exploited to synthesize a large aperture and thereby greatly increase the spatial resolution, possibly even to sub-meter resolution depending on sensor design and scene properties. Figure 9 shows an example of SAR image exhibiting many oceanic features. These data were collected from the NASA JPL AirSAR system and employed a technique called along-track interferometry to enable measurement of the ocean radial surface velocity (indicated by the color) in addition to traditional backscatter contrast (indicated by brightness). Employing various techniques and capabilities one can exploit interferometry, frequency and polarization diversity to measure not only the signal reflected from marine debris but also its profile above the sea surface, the wake generated by its interaction with ambient surface waves, and velocity of its drift relative to the surrounding area. Combined with remotely sensed currents and wind, SAR has the potential to provide a comprehensive description of the dynamics of the floating object.

Figure 9. High-resolution sea surface map off Santa Barbara, California, derived from suborbital C-band SAR (Moller, 2016)35.
Other radars presently used in satellite missions, such as coarse-resolution altimeters and scatterometers, provide data that are used to derive mesoscale surface currents29, 30 (Maximenko and Hafner, 2010) that play an important role in the transport of marine debris, other pollution (such as oil spills), as well as biological rafting and climate systems. At the same time, model studies (McWilliams, 2016), supported by high-resolution images of the sea surface temperature and oil spills suggest that the strongest convergences on the ocean surface occur on the submesoscale (1–10 km). These local convergences, whose signatures are seen in Figure 9, play a very important role in the dynamics of floating marine debris. Such missions as SKIM31 (Sea Surface KInematics Multiscale monitoring satellite mission; Ardhuin et al., 2018, 2019), SWOT32 (Surface Water and Ocean Topography), SEASTAR33, and WACM34 (Wind And Currents Mission) are expected to give insight into submesoscale dynamics. SAR observations, embedded in these missions, or used as complementing projects, will significantly enrich our knowledge of marine debris sources, sinks, patterns, and pathways. Passive microwave radiometers, broadly used in satellite missions, may also be helpful in tracking marine debris, however, their capability is yet to be demonstrated.
As a part of IMDOS, remote sensing will require adequate calibration and validation, based on an observational in situ component, described in section Direct Observations of Marine Debris. Analysis of the influence of subsurface ocean processes (such as the vertical shear of currents) on debris drift will also require close interaction with other components of the Global Ocean Observing System (see Centurioni et al., 2019).
While satellites are important to cover the largest scales, the same or similar remote sensing technologies will be used from suborbital platforms [High Altitude Pseudo Satellites (HAPS), aircraft, drones] ships and as portable devices to provide focus on important scales and priority regions as required by the users.
Using Existing and Planned Satellite Missions to Measure Marine Debris
While remote sensing is needed to provide a global view of the marine litter concentration in the oceans, dedicated satellite missions are expensive, no single sensor responds to all needs of IMDOS, and none of the currently orbiting instruments were specifically designed to detect plastic marine litter. Neither are “marine debris” satellites in the short-term plans of leading space agencies (e.g., recommendation on the Decadal Strategy for Earth Observation from Space from the US National Academies, ESAS201736). At the same time, the scope and capabilities of some flying or coming soon missions do overlap with the properties and dynamics of marine debris37. With the significant increase of the number of satellite launches foreseen, including commercial launches (Euroconsult, 2017), this provides new opportunities for remote sensing of marine debris. For example, the ESAS2017 list of designated (top priority) targeted variables includes Surface Biology and Geology, studied with hyperspectral imagery in the visible and SWIR, multi- or hyperspectral imagery in the thermal IR. As described in previous sections, the same sensors can be used for monitoring marine debris. Similarly, other missions will provide data on submesoscale currents that will advance models, simulating marine debris drift.
The PRISMA satellite, carrying a hyperspectral instrument operating in the 400–2500 nm range, with spectral and spatial resolution of 12 nm and 30 m, respectively, has been launched in March 2019 by Italian Space Agency (ASI). The Plankton, Aerosol, Cloud, ocean Ecosystem mission (PACE, NASA), expected to be launched late 2022, will include a hyperspectral Ocean Color Instrument (OCI), and Environmental Mapping and Analysis Program (EnMAP, DLR) will carry a hyperspectral “pushbroom” imager with high spectral (6.5–10 nm) and spatial (30 m) resolutions. More examples of future missions with varying spectral and spatial resolution capabilities relevant to marine debris remote sensing are summarized below and in Table 2. To identify characteristics (spectral bands, spectral resolution, signal-to-noise ratio, minimum and maximum ground sampling distance, etc.) of orbiting optical sensors optimal for detection of plastic marine debris, the European Space Agency (ESA) sponsored two parallel ongoing projects (OPTIMAL and RESMALI) focusing on passive optical spectro-radiometric remote sensing.
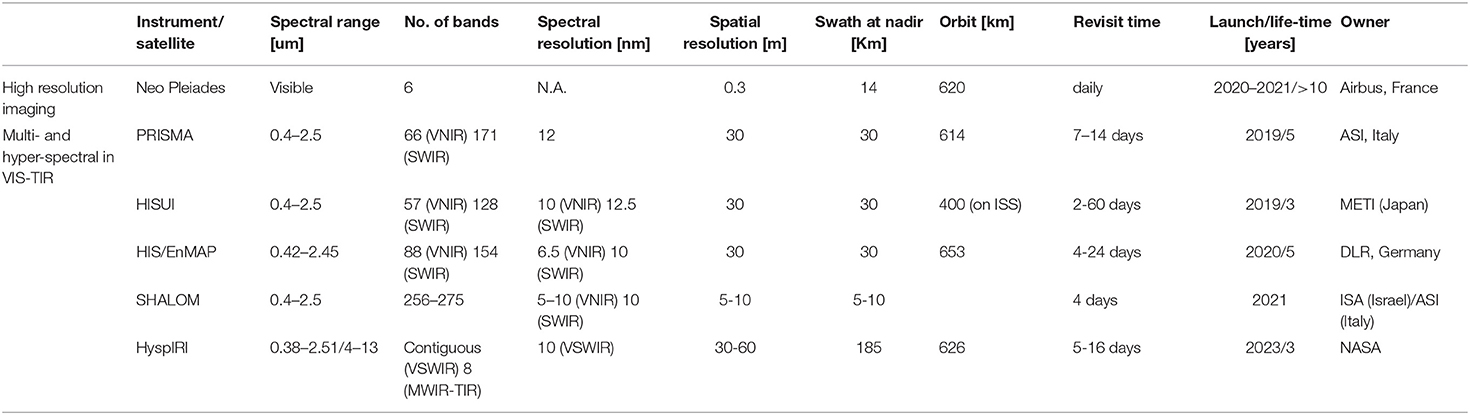
Table 2. Examples of future satellite missions and instruments with the potential of plastic marine litter detection.
Expansion of the satellite mission tasks to include marine debris will require collaborative work of marine debris scientists, data users, and mission teams to demonstrate the presence of significant debris signal in the sensor data and develop methodologies for its extraction and calibration-validation efforts. In some cases, this may require modification of standard data processing procedures or adjustment of the sensor parameters (e.g., Garaba and Dierssen, 2018). It is critical that researchers, focusing on the dynamics and impacts of marine debris, penetrate mission science teams and gain influence on the team decisions. Backed by the growing interest of society in the marine debris problem, the activity of these scientists can accelerate development of new sensors or modification of sensors on some planned missions and inclusion of marine debris applications in the mission goals. Furthermore, small satellites (such as Cubesat)38 offer an affordable solution for deployment of experimental sensors or their constellations but require development of compact space systems. Monitoring marine pollution is addressed as a potential application in several small-satellite initiatives proposed recently.
Networking
Consolidation of distributed platforms and their groups into an integrated system requires active networking. Presently, there are several platforms that monitor marine litter on a large scale by assembling local observations (GESAMP, 2019). These platforms include national (run by such governmental agencies as NOAA, CSIRO, SOA, etc.) and regional (such as OSPAR39 in the Northeast Atlantic or UNEP/MED40 in the Mediterranean Sea) programs as well as such coordinated efforts as MSFD (European Parliament and Council of the European Union, 2008; European Commission, 2017). Some of the contributing observations are collected on a regular basis while some other are opportunistic or acquired in the course of short-time projects, experiments, or initiatives. Several data-collecting systems, often managed by non-governmental organizations (NGOs), rely on coordinated crowdsourcing (e.g., The Trawlshare Program41, The International Coastal Cleanup42).
With the fast-growing number of local initiatives and datasets, their assembling into larger products and databases for joint analyses requires unified definitions, standards and formats, complemented by well-developed infrastructure for data flow and storage. For example, division on such categories as mega-, meso-, macro-, micro-, and nano-plastics should be based on clear size ranges (e.g., Frias and Nash, 2019; GESAMP, 2019), and accepted by all contributors and users of the observing system. While precise boundaries of the observed range of marine debris are hard to enforce with respect to observational tools and methods that are currently used, ambiguity of the analysis should be avoided by carefully following unified methodologies. This also includes harmonization of indicators and sampling strategies, as recently proposed by GESAMP (2019).
A good example of successful regional partnership is the European Marine Observation and Data Network (EMODnet). In Europe, the Marine Strategy Framework Directive (MSFD) establishes principles and rules for a monitoring network of environmental data and marine litter (Galgani et al., 2013). This monitoring is based on the measurements of stranded litter, litter at sea, microplastics, and litter ingested by animals (like birds or sea turtles). Also, in some cases, entanglement of marine organisms is rated on a regular basis. The data acquired by EU Member States are used for harmonized assessments and aims at maintaining or progressing toward Good Environmental Status. The MSFD Technical Group on Marine Litter43 is working toward consolidated approaches in order to derive compatible data (see also GESAMP, 2019). EMODnet was launched by the European Commission to promote and support the large-scale collection and harmonization of environmental data in European Seas, including (as defined by the UNEP) the North Atlantic Ocean (OSPAR) and Baltic (HELCOM)44, Mediterranean (UNEP MAP), and Black (Black Sea Commission)45 seas that are currently at different degrees of maturity. The aim is to provide reliable information to set targets and baselines for policy decisions (Addamo et al., 2018).
The EMODnet Chemistry46 represents one of the seven thematic data portals, each being related to a different area of expertise. It was created in 2009 by a wide consortium of European research institutes and environmental agencies involved in the collection, standardization, aggregation, and sharing of data related to eutrophication (oxygen, chlorophyll, phosphate, nitrogen and silicate compounds), and contaminants (hydrocarbons, metals, pesticides, radionuclides). The scope has been recently extended to marine debris with specific foci on beach litter, seafloor litter (collected by fish trawl surveys) and micro-litter (microplastics).
The overall structure of the management of litter information in Europe is heterogeneous. There are different levels of development and harmonization of formats and protocols for different compartments (beach and seafloor), particle size (macro and micro-litter) and among geographic scales (regional, national, etc.). The EMODnet Chemistry plans to deal with this heterogeneity by adopting consolidated data formats (when available) and adapting them as needed (Galgani et al., 2017, 2018). Specific approaches have been proposed, based on the best available reference documents, to address the task at European scale.
The management of beach litter is based on OSPAR, with the possibility to report data using OSPAR, MSFD, UNEP/MAP, or UNEP–Marlin protocols. The EMODnet Chemistry beach litter format is designed as an Excel spreadsheet with four worksheets that handle separately individual topics: beach metadata, survey metadata, animals and litter data.
The first pan-European beach litter database has been populated with all relevant datasets available in the European seas. It has been developed in synergy with the European Commission Joint Research Center (JRC), OSPAR, HELCOM, and the MSFD Technical Group on Marine Litter. Presently, 518 beaches and 4,772 surveys from 29 countries have been imported in this database (Addamo et al., 2018). Figures 10–12 show distribution of data of beach litter, seafloor litter and micro-litter, respectively.
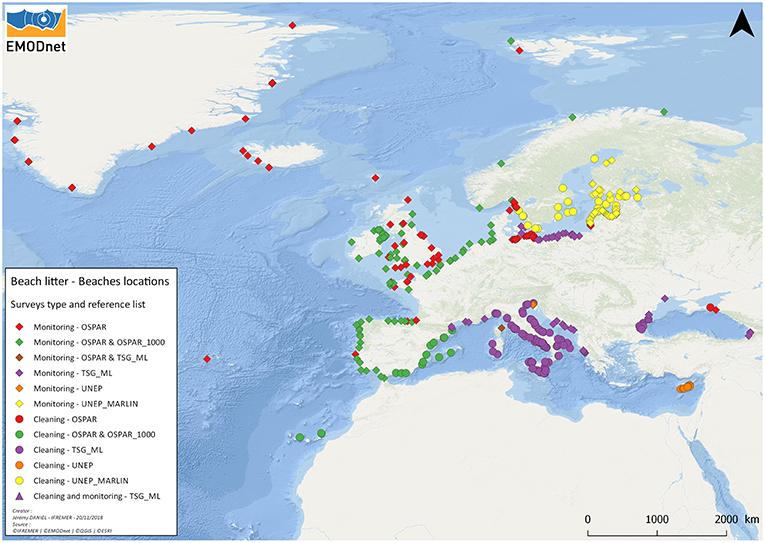
Figure 10. Spatial distribution of surveyed beaches, and reference lists used for litter items in EMODnet and cleaning/monitoring sites (The EMODnet Chemistry project, whose PIs are among the authors of this paper, holds the copyright on this figure).
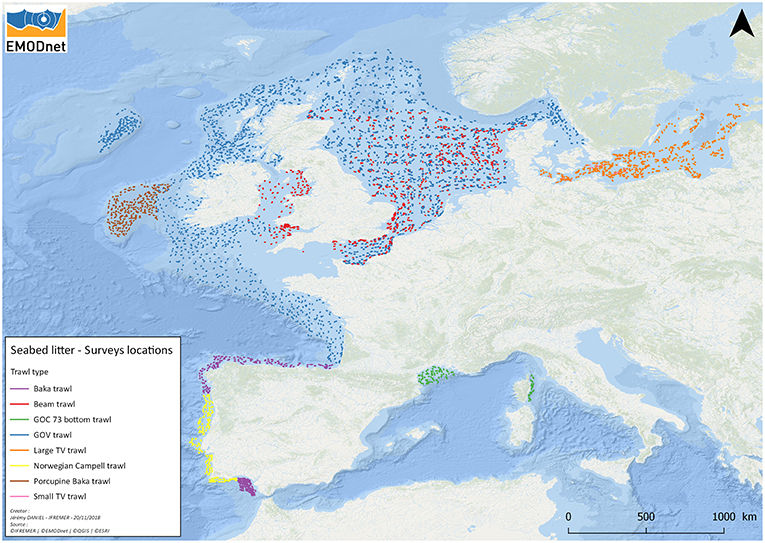
Figure 11. Spatial distribution of seafloor litter survey transects and gear types used for sampling (the EMODnet Chemistry project, whose PIs are among the authors of this paper, holds the copyright on this figure).
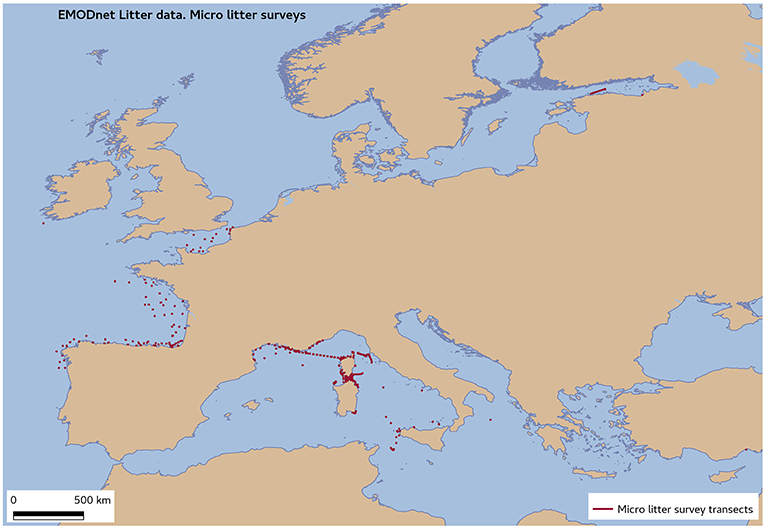
Figure 12. Spatial distribution of micro-litter survey transects in EMODnet (The EMODnet Chemistry project, whose PIs are among the authors of this paper, holds the copyright on this figure).
For the bottom trawls litter, the database used the experience of ICES DATRAS47 but added reports from some of the International Bottom Trawl Surveys in the Mediterranean (MEDITS)48 as well as general data submitted according to the Marine Strategy Framework Directive (MSFD49.)
For floating marine microplastics, the SeaDataNet50 metadata and data formats have been adopted after some adaptation to address the diversity of information from other European sources. With the development of autonomous instruments and sensors, the observing network system will also benefit from the use of platforms of opportunity, such as ships, airplanes, and coastal structures, from which they can be operated either remotely or with minimum intervention of operators.
Data of beach, floating micro and (soon) also seafloor litter can be accessed through the dedicated discovery and access service in the EMODnet Chemistry portal51.
Depending on the interests and agenda of the international community, the EMODnet could serve as a template for networks developing in other regions and/or expand beyond the European Seas. In any case, priorities of marine litter indicators and associated environmental variables may differ among regions and space scales and the work successfully performed in Europe needs to be redone elsewhere, with economical, political, ecological, and cultural differences accounted for.
The global governance scheme for marine debris data acquisition, streaming, quality control, and distribution to users is still to be designed and built. This infrastructure is critical for IMDOS and it will develop in collaboration with UN Environment, assuming the coordinating role in international harmonization, and all other stakeholders, described in section IMDOS Stakeholders.
Building IMDOS
The implementation of IMDOS is difficult to plan. The marine debris community is still under construction, observations are sparse, many impacts of marine debris are poorly documented or unstudied, numerical models do not capture all relevant processes, different groups follow different methodologies, international regulations are vague, national plans are incomplete and often missing, and overall funding to develop the observing system is not secured.
This is exactly why we believe that this paper, presenting the concept of the “ideal” observing system and identifying gaps and problems as current and future opportunities, is very timely. The concept of IMDOS aligns efforts of very different entities (from international to individuals) in a coherent way, maximizing the efficacy of use of available resources. This includes geographical expansion of the experience of leading countries and groups, exemplified in previous sections, and interaction with other observing systems. For example, just as neuston nets are used to sample microplastics and plankton, some other instruments can collect both debris and non-debris data simultaneously, so that adding debris measurements to existing protocols will be beneficial to both observing systems.
With the growing international concern and momentum toward resolution of the problem of artificial pollutions, the present states of local, regional and national marine debris programs are very heterogeneous. Generally, developed countries have resources and information systems to better address the problem but this leaves large geographical areas unattended, preventing a global action. In the next decade we expect increased activity on all scales that will lead to development of new national initiatives, unification and/or harmonization of approaches, standards, instruments and formats and consolidation of individual components into an integrated observing system. Exchange of the data, their processing, archiving and serving to the users will require construction of efficient global informational infrastructure. This is a tremendously difficult task but examples of such European programs as EMODnet and Copernicus Marine Environment Monitoring Service (MEMS52) are inspiring.
On a global scale, GOOS exemplifies the evolution of ocean science and technology in recent decades from exploration and understanding the basic dynamics to monitoring and practical applications. The governance structure of GOOS as a “collaborative system of ocean observations, encompassing in situ networks, satellite systems, governments, UN agencies and individual scientists,” combining sustainability with evolution, can be adopted when constructing IMDOS. The problem of marine debris is interdisciplinary by its nature and close collaboration between IMDOS and GOOS and other global observing systems as well as the diversity of actors and institutions, monitoring the environment and society, is imperative for its effective control and successful resolution.
Author Contributions
This review paper is produced by the members of five working groups that proposed Community White Papers relevant to the dynamics and impacts of marine debris and merged on advice of the OceanObs19 organizers. NM developed the structure of the paper and coordinated work on individual sections. KL, RT, and NM drafted section Introduction. NM and KL drafted section Impacts and CM, CL, AA, MRL, NP, MV, GH, ME, JC, and A-ER provided critical revision of this section. EV and NM drafted section Structure of IMDOS and JV, CM, and MA provided critical revision. Section Direct Measurements was drafted by RSL, KP, DM, MEM, AC, RT, and TG and critically revised by KL, CL, LC, AM, RL, CP, AI, GH, ME, IC, SA, JC, GR, LH, A-MC, TG, GG, BH, LL, HL, RM, EM, RO, BR, MT, AT, and CW. PC, VM-V, SG, and NM drafted section Remote Sensing and LG-M, DM, ER, GA, YC, YG, and TM provided critical revision of the section. MV, FG, AG, MJ, NH, AB, AA, GH, MS, and MLM drafted section Networking and PC, RSL, MRL, IM-S, MM, and AT provided critical revision. Section Interactions was drafted by PC, NM, and SG, and critically revised by BH, EV, and CW. Every part of the paper went through the full-team discussion. All authors approve the version to be published and agree to be accountable for all aspects of the work in ensuring that questions related to the accuracy or integrity of any part of the work are appropriately investigated and resolved.
Funding
NM, LC, GR, LH, and JC were partly funded by NASA Interdisciplinary Science through Grant 80NSSC17K0559 (FloatEco project). The SCOR WG 153 partners were partly supported by the national committees of the Scientific Committee on Oceanic Research (SCOR) and Grant OCE-1546580 to SCOR from the U.S. National Science Foundation. EMODnet Chemistry was supported by DG MARE under the Service Contract number EASME/EMFF/2016/1.3.1.2-Lot4/SI2.749773. SG was supported by Deutsche Forschungsgemeinschaft (DFG, German Research Foundation)-Projektnummer 417276871. CP was funded through an FCT fellowship (SFRH/BD/111757/2015). AM was supported through the EAPA_165/2016: iFADO: Innovation in the Framework of the Atlantic Deep Ocean, financed by the INTERREG | Atlantic Area. EV was supported through funding from the European Research Council (ERC) under the European Union's Horizon 2020 research and innovation programme (Grant Agreement No. 715386). IC was supported by Russian Foundation for Basic Research, grant number 18-55-76001. VM-V was supported through funding from the European Space Agency (ESA) (project No. 4000120879/17/NL/PS). NP was supported by the VILLUM FONDEN (grant number: 15397). RL was supported by NOAA/AOML and NOAA's Ocean Observation and Monitoring Division. AT was supported by the Chili National Council for Scientific and Technological Development (CNPq) through research grant 309697/2015-8. AI was supported by the Environmental Research and Technology Development Fund (SII-2) of the Japan Ministry of the Environment. TG was partly supported through UK NERC grant NE/S003975/1.
Disclaimer
The information in this paper reflects the views of the authors, and does not necessarily reflect the official positions or policies of NOAA, EPA, or Smithsonian Institution.
Conflict of Interest Statement
MA was employed by Argans. YG was employed by eOdyn. TM was employed by Mace Geospatial, LLC.
The remaining authors declare that the research was conducted in the absence of any commercial or financial relationships that could be construed as a potential conflict of interest.
Acknowledgments
The authors would like to thank the editor, reviewers, and Frontiers Production staff, whose excellent help allowed to significantly improve the paper.
Footnotes
1. ^http://www.oceanobs19.net/
2. ^http://www.imo.org/en/OurWork/Environment/PollutionPrevention/Garbage/Pages/Default.aspx
3. ^https://en.unesco.org/ocean-decade
4. ^https://sustainabledevelopment.un.org/sdg14
5. ^https://www.unenvironment.org/explore-topics/oceans-seas/what-we-do/addressing-land-based-pollution/global-partnership-marine
6. ^https://marinedebris.noaa.gov/sites/default/files/publications-files/Honolulu_Strategy.pdf
8. ^http://www.g8.utoronto.ca/science/G7_Science_2015-en.pdf
9. ^https://ieep.eu/news/g20-adopts-t20-recommendations-on-plastics-and-marine-litter
10. ^https://oceanservice.noaa.gov/facts/redtide.html
11. ^https://www.fisheries.noaa.gov/feature-story/removal-and-research-marine-debris-team-strikes-again
12. ^https://response.restoration.noaa.gov/over-80-tons-marine-debris-removed-northwestern-hawaiian-islands
13. ^http://www.worldshipping.org/industry-issues/safety/Containers_Lost_at_Sea_-_2017_Update_FINAL_July_10.pdf
14. ^https://2019.transpacyc.com/race-archives/news-article/2013/2013-honolulu/dismastings-debris-dazzling-speed-in-transpac
16. ^https://www.epa.gov/ocean-dumping/learn-about-ocean-dumping#Before
17. ^https://en.unesco.org/ocean-decade
18. ^http://www.globcurrent.org/
19. ^http://www.goosocean.org/
20. ^https://www.ferrybox.com/
21. ^https://ioos.noaa.gov/project/atn/
22. ^http://www.aoml.noaa.gov/phod/gdp/index.php
23. ^http://www.oceansites.org/
24. ^http://aco-ssds.soest.hawaii.edu/
25. ^https://www.axios.com/dominican-republic-garbage-plastic-waste-cover-beach-c16f8637-6002-476c-bcf5-1964fe38abc1.html
26. ^https://oceanconservancy.org/wp-content/uploads/2017/06/International-Coastal-Cleanup_2017-Report.pdf
27. ^https://marinedebris.noaa.gov/partnerships/marine-debris-tracker
28. ^https://www.litterati.org/
29. ^https://www.esr.org/research/oscar/oscar-surface-currents/
30. ^http://marine.copernicus.eu/services-portfolio/access-to-products/
31. ^https://www.umr-lops.fr/en/Projects/Active-projects/SKIM
32. ^https://swot.jpl.nasa.gov/
33. ^https://www.researchgate.net/publication/323629810_SEASTAR_A_mission_to_study_ocean_submesoscale_dynamics_and_small-scale_atmosphere-ocean_ processes_in_coastal_shelf_and_polar_seas
34. ^https://mdc.coaps.fsu.edu/scatterometry/meeting/docs/2016/Tue_PM/B_winds_update.pdf
35. ^http://iprc.soest.hawaii.edu/NASA_WS_MD2016/agenda.php
36. ^https://www.nap.edu/catalog/24938/thriving-on-our-changing-planet-a-decadal-strategy-for-earth
37. ^https://www.esa.int/Our_Activities/Preparing_for_the_Future/Discovery_and_Preparation/Cleaning_up_our_oceans; https://www.esa.int/Our_Activities/Space_Engineering_Technology/Seeking_innovative_ideas_space_for_the_oceans
40. ^http://web.unep.org/unepmap/
41. ^https://www.5gyres.org/trawlshare-application
42. ^https://oceanconservancy.org/trash-free-seas/international-coastal-cleanup/annual-data-release/
43. ^https://mcc.jrc.ec.europa.eu/main/dev.py?N=41&O=434&titre_chap=TG%2520Marine%2520Litter
45. ^http://www.blacksea-commission.org/
46. ^http://www.emodnet-chemistry.eu/welcome
47. ^http://www.ices.dk/marine-data/data-portals/Pages/DATRAS.aspx
48. ^http://www.sibm.it/SITO%20MEDITS/principaleprogramme.htm
49. ^https://eur-lex.europa.eu/legal-content/EN/TXT/?uri=CELEX:32008L0056
50. ^https://www.seadatanet.org/
References
Acuña-Ruz, T., Uribe, D., Taylor, R., Amézquita, L., Guzmán, M. C., Merrill, J., et al. (2018). Anthropogenic marine debris over beaches: spectral characterization for remote sensing applications. Remote Sens. Environ. 217, 309–322. doi: 10.1016/j.rse.2018.08.008
Addamo, A. M., Brosich, A., Chaves, M. D. M., Giorgetti, A., Hanke, G., Molina, E., et al. (2018). Marine Litter Database: Lessons Learned in Compiling the First Pan-European Beach Litter Database, EUR 29469 EN. Luxembourg: Publications Office of the European Union.
Anderson, Z. T., Cundy, A. B., Croudace, I. W., Warwick, P. E., Celis-Hernandez, O., and Stead, J. L. (2018). A rapid method for assessing the accumulation of microplastics in the sea surface microlayer (SML) of estuarine systems. Sci. Reports 8:9428. doi: 10.1038/s41598-018-27612-w
Ardhuin, F., Aksenov, Y., Benetazzo, A., Bertino, L., Brandt, P., Caubet, E., et al. (2018). Measuring currents, ice drift, and waves from space: the sea surface kinematics multiscale monitoring (SKIM) concept. Ocean Sci. 14, 337–354. doi: 10.5194/os-14-337-2018
Ardhuin, F., Brandt, P., Gaultier, L., Donlon, C., Battaglia, A., Boy, F., et al. (2019). SKIM, a candidate satellite mission exploring global ocean currents and waves. Front. Mar. Sci. 6:209. doi: 10.3389/fmars.2019.00209
Barat, D., Spencer, D., Benazzi, G., Mowlem, M. C., and Morgan, H. (2012). Simultaneous high speed optical and impedance analysis of single particles with a microfluidic cytometer. Lab Chip 12, 118–126. doi: 10.1039/C1LC20785G
Barnett, P. R. O., Watson, J., and Connelly, D. (1984). The multiple corer for taking virtually undisturbed samples from shelf, bathyal and abyssal sediments. Oceanol. Acta. 7, 399–408.
Benazzi, G., Holmes, D., Sun, T., Mowlem, M. C., and Morgan, H. (2007). Discrimination and analysis of phytoplankton using a microfluidic cytometer. Nanobiotechnology 1, 94–101. doi: 10.1049/iet-nbt:20070020
Bergmann, M., and Klages, M. (2012). Increase of litter at the Arctic deep-sea observatory Hausgarten. Mar. Poll. Bull. 64, 2734–2741. doi: 10.1016/j.marpolbul.2012.09.018
Brooks, M. T., Coles, V. J, and Coles, W. C. (2019). Inertia influences pelagic sargassum advection and distribution. Geophys. Res. Lett. 46, 2610–2618. doi: 10.1029/2018GL081489
Buchanan, J. B. (1971). Pollution by synthetic fibres. Marine Pollution Bulletin. 2:23. doi: 10.1016/0025-326X(71)90136-6
Carlton, J. T., Chapman, J. W., Geller, J. B., Miller, J. A., Carlton, D. A., McCuller, M. I., et al. (2017). Tsunami-driven rafting, transoceanic species dispersal and implications for marine biogeography. Science 357, 1402–1406. doi: 10.1126/science.aao1498
Carpenter, E. J., and Smith, K. L. Jr. (1972). Plastics on the Sargasso Sea surface. Science 175, 1240–1241. doi: 10.1126/science.175.4027.1240
Centurioni, L., Chen, Z., Lumpkin, R., Braasch, L., Brassington, G., Chao, Y., et al. (2019). Multidisciplinary global in-situ observations of essential climate and ocean variables at the air-sea interface in support of climate variability and change studies and to improve weather forecasting, pollution, hazard and maritime safety assessments. Front. Mar. Sci.
Centurioni, L. R. (2018). “Drifter technology and impacts for sea surface temperature, sea-level pressure, and ocean circulation studies,” in Observing the Oceans in Real Time, eds. R. Venkatesan, A. Tandon, E. D'Asaro, and M. A. Atmanand. 37–57. doi: 10.1007/978-3-319-66493-4_3
Chubarenko, I. P., Esiukova, E. E., Bagaev, A. V., Bagaeva, M. A., and Grave, A. N. (2018). Three-dimensional distribution of anthropogenic microparticles in the body of sandy beaches. Sci. Total Environ. 628–629, 1340–1351. doi: 10.1016/j.scitotenv.2018.02.167
Cózar, A., Echevarría, F., González-Gordillo, J. I., Irigoien, X., Úbeda, B., Hernández-León, S., et al. (2014). Plastic debris in the open ocean. Proc. Natl. Acad. Sci. U.S.A. 111, 10238–10244. doi: 10.1073/pnas.1314705111
Cózar, A., Martí, E., Duarte, J. C. M., García-de-Lomas, E. J., van Sebille, E., Ballatore, T. J., et al. (2017). The Arctic Ocean as a dead end for floating plastics in the North Atlantic branch of the thermohaline circulation. Sci. Adv. 3:e1600582. doi: 10.1126/sciadv.1600582
Cundell, A. M. (1973). Plastic materials accumulating in Narragansett Bay. Mar. Poll. Bull. 4, 187–188. doi: 10.1016/0025-326X(73)90226-9
Dubelaar, G. B. J., and Geerders, P. J. F. (2004). Innovative technologies to monitor plankton dynamics - Scanning flow cytometry, a new dimension in real-time, in-situ water quality monitoring. Sea Technol. 45, 15–21.
Dubelaar, G. B. J., and Jonker, R. R. (2000). Flow cytometry as a tool for the study of phytoplankton. Sci. Mar. 64, 135–156. doi: 10.3989/scimar.2000.64n2135
Dupont, J., Magnin, S., Rousseau, F., Zbinden, M., Frebourg, G., Samadi, S., et al. (2009). Molecular and ultrastructural characterization of two ascomycetes found on sunken wood off Vanuatu Islands in the deep Pacific Ocean. Mycol. Res. 113, 1351–1364. doi: 10.1016/j.mycres.2009.08.015
Edson, E. C., and Patterson, M. R. (2015). “MantaRay: a novel autonomous sampling instrument for in situ measurements of environmental microplastic particle concentrations,” in Oceans 2015 Conference (Washington, DC).
Eriksen, M., Lebreton, L., Carson, H., Thiel, M., Moore, C., Borerro, J., et al. (2014). Plastic pollution in the World's oceans: more than 5 trillion plastic pieces weighing over 250,000 Tons Afloat at sea. PLoS ONE 9:e111913. doi: 10.1371/journal.pone.0111913
Eriksen, M., Liboiron, M., Kiessling, T., Charron, L., Alling, A., Lebreton, L., et al. (2018). Microplastic sampling with the AVANI trawl compared to two neuston trawls in the Bay of Bengal and South Pacific. Environ. Poll. 232, 430–439. doi: 10.1016/j.envpol.2017.09.058
Erni-Cassola, G., Gibson, M. I., Thompson, R. C., and Christie-Oleza, J. A. (2017). Lost, but found with nile red: a novel method for detecting and quantifying small microplastics (1 mm to 20 mu m) in environmental samples. Environ. Sci. Technol. 51, 13641–13648. doi: 10.1021/acs.est.7b04512
Euroconsult (2017). Satellites to be Built and Launched by 2026. Analysis and Report. Available online at: http://www.euroconsult-ec.com/research/satellites-built-launched-by-2026-brochure.pdf (accessed August 16, 2019).
European Commission (2017). Laying Down Criteria and Methodological Standards on Good Environmental Status of Marine Waters and Specifications and Standardized Methods for Monitoring and Assessment, and Repealing Decision 2010/477/EU COMMISSION DECISION (EU) 2017/848. Journal of the European Union. Available online at: https://eur-lex.europa.eu/legal-content/EN/TXT/PDF/?uri=CELEX:32017D0848&from=EN
European Parliament and Council of the European Union (2008). Marine strategy framework directive, directive 2008/56/EC of the European parliament and of the council. J. Eur. Union 164, 19–40.
Fraser, C. I., Morrison, A. K., Hogg, A., Mc, C., Macaya, E. C., Van Sebille, E., et al. (2018). Antarctica's ecological isolation will be broken by storm-driven dispersal and warming. Nat. Clim. Change 8, 704–708. doi: 10.1038/s41558-018-0209-7
Frere, L., Paul-Pont, I., Moreau, J., Soudant, P., Lambert, C., Huvet, A., et al. (2016). A semi-automated Raman micro-spectroscopy method for morphological and chemical characterizations of microplastic litter. Mar. Poll. Bull. 113, 461–468. doi: 10.1016/j.marpolbul.2016.10.051
Frias, J. P. G. L., and Nash, R. (2019). Microplastics: finding a consensus on the definition. Mar. Poll. Bull. 138, 145–147. doi: 10.1016/j.marpolbul.2018.11.022
Galgani, F., Giorgetti, A., Le Moigne, M., Brosich, A., Vinci, M., Lipizer, M., et al. (2018). Guidelines and Forms for Gathering Marine Litter Data. European scale. 58. doi: 10.6092/8ce4e8b7-f42c-4683-9ece-c32559606dbd
Galgani, F., Giorgetti, A., Vinci, M., Le Moigne, M., Moncoiffe, G., Brosich, A., et al. (2017). Proposal for Gathering and Managing Data Sets on Marine Micro-Litter on a European Scale. 35.
Galgani, F., Hanke, G., and Maes, T. (2015). “Global distribution, composition and abundance of marine litter,”in Marine Anthropogenic Litter, eds M. Bergmann, L. Gutow, and M. Klages (Cham: Springer Open; Elsevier, 29–56.
Galgani, F., Hanke, G., Werner, S., and De Vrees, L. (2013). Marine litter within the european marine strategy framework directive. Ices J. Mar. Sci. 70, 1055–1064. doi: 10.1093/icesjms/fst122
Galloway, T. S. (2015). “Micro- and nano-plastics and human health” in Marine Anthropogenic Litter, eds M. Bergmann, L. Gutow, and M. Klages (Cham: Springer Open; Elsevier), 343–366. doi: 10.1007/978-3-319-16510-3_13
Garaba, S. P., Aitken, J., Slat, B., Dierssen, H. M., Lebreton, L., Zielinski, O., et al. (2018). Sensing ocean plastics with an airborne hyperspectral shortwave infrared imager. Environ. Sci. Technol. 52, 11699–11707. doi: 10.1021/acs.est.8b02855
Garaba, S. P., and Dierssen, H. M. (2017). Spectral Reference Library of 11 Types of Virgin Plastic Pellets Common in Marine Plastic Debris. The Ecological Spectral Information System (EcoSIS). Available online at: http://dx.doi.org/10.21232/C27H34 (accessed July 25, 2019).
Garaba, S. P., and Dierssen, H. M. (2018). An airborne remote sensing case study of synthetic hydrocarbon detection using short wave infrared absorption features identified from marine harvested macro- and microplastics. Rem. Sens. Environ. 205, 224–235. doi: 10.1016/j.rse.2017.11.023
GESAMP (2019). Guidelines or the Monitoring and Assessment of Plastic Litter and Microplastics in the Ocean, eds P. J. Kershaw, A. Turra, and F. Galgani, Rep. Stud. GESAMP No. 99 (IMO/FAO/UNESCO-IOC/UNIDO/WMO/IAEA/UN/UNEP/UNDP/ISA Joint Group of Experts on the Scientific Aspects of Marine Environmental Protection).
Geyer, R., Jambeck, J. R., and Law, K. L. (2017). Production, use, and fate of all plastics ever made. Sci. Adv. 3:e1700782. doi: 10.1126/sciadv.1700782
Goddijn-Murphy, L., Peters, S., Van Sebille, E., James, N. A., and Gibb, S. (2018). Concept for a hyperspectral remote sensing algorithm for floating macro plastics. Mar. Poll. Bull. 126:11. doi: 10.1016/j.marpolbul.2017.11.011
Goddijn-Murphy, L. M., and Dufaur, J. (2018). Proof of concept for a model of light reflectance of plastics floating on natural waters. Mar. Poll. Bull. 135, 1145–1157. doi: 10.1016/j.marpolbul.2018.08.044
González-Fernández, D., and Hanke, G. (2017). Toward a harmonized approach for monitoring of riverine floating macro litter inputs to the marine environment. Front. Mar. Sci. 4:86. doi: 10.3389/fmars.2017.00086
Gower, J., Young, E., and King, S. (2013), Satellite images suggest a new Sargassum source region in 2011. Remote Sens. Lett. 4, 764–773. doi: 10.1080/2150704X.2013.796433
Guo, J. J., Zhang, F., Liu, C. H., Li, Y., and Zheng, R. E. (2017). Raman-fluorescence spectroscopy for underwater in-situ application. Spectrosc. Spectr. Anal. 37, 3099–3102.
Hanke, G., and Gonzalez-Fernandez, D. (2014). “Long term deployment of the JRC Sealittercam on the Western Mediterranean Sea,” in Perseus 2nd Scientific Workshop. Marrakesh. Book of Abstracts. PERSEUS Project. Available online at: http://www.perseus-net.eu/assets/media/PDF/GA%20Marrakesh/3408.pdf
Hardesty, B. D., Harari, J., Isobe, A., Lebreton, L., Maximenko, N., Potemra, J., et al. (2017). Using numerical model simulations to improve the understanding of microplastic distribution and pathways in the marine environment. Front. Mar. Sci. 30, 1–9. doi: 10.3389/fmars.2017.00030
Hinojosa, I. A., and Thiel, M. (2009). Floating marine debris in fjords, gulfs and channels of southern Chile. Mar. Poll. Bull. 58, 341–350. doi: 10.1016/j.marpolbul.2008.10.020
Hong, S., Lee, J., and Lim, S. (2017). Navigational threats by derelict fishing gear to navy ships in the Korean seas. Mar. Poll. Bull. 119, 100–105 doi: 10.1016/j.marpolbul.2017.04.006
Jambeck, J. R., Geyer, R., Wilcox, C., Siegler, T. R., Perryman, M., Andrady, A., et al. (2015). Plastic waste inputs from land into the ocean. Science 347, 768–771. doi: 10.1126/science.1260352
Jang, Y. C., Hong, S., Lee, J., Lee, M. J., and Shim, W. J. (2014). Estimation of lost tourism revenue in Geoje Island from the 2011 marine debris pollution event in South Korea. Mar. Poll. Bull. 81, 49–54. doi: 10.1016/j.marpolbul.2014.02.021
Japan Ministry of the Environment. (2012). Estimated Total Amount of Debris Washed out by the Great East Japan Earthquake. Available online at: http://www.env.go.jp/en/focus/docs/files/20120901-57.pdf (accessed August 10).
Jing, X., Gou, H., Gong, Y., Su, X., Xu, L., Ji, Y., et al. (2018). Raman-activated cell sorting and metagenomic sequencing revealing carbon-fixing bacteria in the ocean. Environ. Microbiol. 20, 2241–2255. doi: 10.1111/1462-2920.14268
Kako, S., Isobe, A., Kataoka, T., Yufu, K., Sugizono, S., Plybon, C., et al. (2018). Sequential webcam monitoring and modeling of marine debris abundance. Mar. Poll. Bull. 132, 33–43. doi: 10.1016/j.marpolbul.2018.04.075
Kako, S. I., Isobe, A., and Magome, S. (2010). Sequential monitoring of beach litter using webcams. Mar. Poll. Bull. 60, 775–779. doi: 10.1016/j.marpolbul.2010.03.009
Karlsson, T. M., Grahn, H., van Bavel, B., and Geladi, P. (2016). Hyperspectral imaging and data analysis for detecting and determining plastic contamination in seawater filtrates. J. Infr. Spectros. 24, 141–149. doi: 10.1255/jnirs.1212
Kataoka, T., Murray, C. C., and Isobe, A. (2017). Quantification of marine macro-debris abundance around Vancouver Island, Canada, based on archived aerial photographs processed by projective transformation. Mar. Poll. Bull. 132, 44–51. doi: 10.1016/j.marpolbul.2017.08.060
Kiessling, T., Gutow, L., and Thiel, M. (2015). “Marine litter as habitat and dispersal vector,” in Marine Anthropogenic Litter, eds. M. Bergmann, L. Gutow, and M. Klages (Cham: Springer Open; Elsevier), 141–181. doi: 10.1007/978-3-319-16510-3_6
Kirkwood, W. J., Peltzer, E., and Brewer, P. G. (2013). Development and deployment of a deep-sea Raman instrumentation for in situ analysis. Abstr. Am. Chem. Soc. 245.
Koelmans, A. A., Besseling, E., and Shim, W. J. (2015). “Nanoplastics in the aquatic environment. Critical review,” in Marine Anthropogenic Litter, eds. M. Bergmann, L. Gutow, and M. Klages (Cham: Springer Open; Elsevier), 325–340. doi: 10.1007/978-3-319-16510-3_12
Koelmans, A. A., Kooi, M., Law, K. L., and van Sebille, E. (2017). All is not lost, deriving a top-down mass budget of plastic at sea. Environ. Res. Lett. 12:500. doi: 10.1088/1748-9326/aa9500
Kokaly, R. F., Clark, R. N., Swayze, G. A., Livo, K. E., Hoefen, T. M., Pearson, N. C., et al. (2017). USGS spectral library version 7, US. Geolog. Surv. Data Ser. 61, 1035. doi: 10.3133/ds1035
Kooi, M., Nes, E. H. V., Scheffer, M., and Koelmans, A. A. (2017). Ups and downs in the ocean, effects on biofouling on vertical transport of microplastics. Environ. Sci. Technol. 51, 7963–7971. doi: 10.1021/acs.est.6b04702
Lambert, B. S., Olson, R. J., and Sosik, H. M. (2017). A fluorescence-activated cell sorting subsystem for the imaging flowcytobot. Limnol. Oceanogr. Methods 15:145. doi: 10.1002/lom3.10145
Lampitt, R. S. (1985). Evidence for the seasonal deposition of detritus to the deep-sea floor and its subsequent resuspension. Deep Sea Res. 32, 885–897. doi: 10.1016/0198-0149(85)90034-2
Lampitt, R. S., and Burnham, M. P. (1983). A free fall time lapse camera and current meter system “Bathysnap” with notes on the foraging behaviour of a bathyal decapod shrimp. Deep Sea Res. 30. 1009–1017. doi: 10.1016/0198-0149(83)90055-9
Lavers, J. L., and Bond, A. L. (2017). Exceptional and rapid accumulation of anthropogenic debris on one of the world's most remote and pristine islands, Proc. Natl. Acad. Sci. U.S.A. 114, 6052–6055. doi: 10.1073/pnas.1619818114
Lebreton, L., Slat, B., Ferrari, F., Sainte-Rose, B., Aitken, J., Marthouse, R., et al. (2018). Evidence that the Great Pacific Garbage Patch is rapidly accumulating plastic. Sci. Reports. 8:4666. doi: 10.1038/s41598-018-22939-w
Lebreton, L. C.-M., Greer, S. D., and Borrero, J. C. (2012). Numerical modelling of floating debris in the world's oceans. Mar. Poll. Bull. 64, 653–661. doi: 10.1016/j.marpolbul.2011.10.027
Lecke-Mitchell, K. M., and Mullin, K. (1992). Distribution and abundance of large floating plastic in the north-central Gulf of Mexico. Mar. Poll. Bull. 24, 598–601. doi: 10.1016/0025-326X(92)90279-F
Lee, J., Hong, S., Song, Y. K., Hong, S. H., Jang, Y. C., Jang, M., et al. (2013). Relationships among the abundances of plastic debris in different size classes on beaches in South Korea. Mar. Poll. Bull. 77, 349–354. doi: 10.1016/j.marpolbul.2013.08.013
Leggett, C., Scherer, N., Curry, M., and Bailey, R. (2014). Assessing the Economic Benefits of Reductions in Marine Debris, A Pilot Study of Beach Recreation in Orange County, California. Final report, June 15, 2014, National Oceanic and Atmospheric Administration. Cambridge, MA. Available online at: http://marinedebris.noaa.gov/sites/default/files/MarineDebrisEconomicStudy.pdf
Li, L. F., Zhang, X., Luan, Z. D., Du, Z. F., Xi, S. C., Wang, B., et al. (2018). In situ quantitative raman detection of dissolved carbon dioxide and sulfate in deep-sea high-temperature hydrothermal vent fluids. Geochem. Geophys. Geosyst. 19:7445. doi: 10.1029/2018GC007445
Long, M., Paul-Pont, I., Hegaret, H., Moriceau, B., Lambert, C., Huvet, A., et al. (2017). Interactions between polystyrene microplastics and marine phytoplankton lead to species-specific hetero-aggregation. Environ. Poll. 2017:228. doi: 10.1016/j.envpol.2017.05.047
Lumpkin, R., Maximenko, N., and Pazos, M. (2012). Evaluating where and why drifters die. J. Atmos. Ocean. Techn. 29, 300–308. doi: 10.1175/JTECH-D-11-00100.1
Maes, T., Jessop, R., Wellner, N., Haupt, K., and Mayes, A. G. (2017). A rapid-screening approach to detect and quantify microplastics based on fluorescent tagging with Nile Red. Sci. Reports 2017:7. doi: 10.1038/srep44501
Martin, C., Parkes, S., Zhang, Q., Zhang, X., McCabe, M. F., and Duarte, C. M. (2018). Use of unmanned aerial vehicles for efficient beach litter monitoring. Mar. Poll. Bull. (2018) 131, 662–673. doi: 10.1016/j.marpolbul.2018.04.045
Matthews, J. P., Ostrovsky, L., Yoshikawa, Y., Komori, S., and Tamura, H. (2017). Dynamics and early post-tsunami evolution of floating marine debris near Fukushima Daiichi. Nat. Geosci. 10, 598–605. doi: 10.1038/ngeo2975
Maximenko, N., Arvesen, J., Asner, G., Carlton, J., Castrence, M., Centurioni, L., et al. (2016a) “Remote sensing of marine debris to study dynamics, balances trends,” in NASA Workshop on Mission Concepts for Marine Debris Sensing, 2016. (Hawai‘i: University of Hawai‘i at Manoa).
Maximenko, N., Arvesen, J., Asner, G., Carlton, J., Castrence, M., Centurioni, L., et al. (2016b). Remote Sensing of Marine Debris to Study Dynamics, Balances and Trends. White Paper, Decadal Survey for Earth Science and Applications from Space, 22.
Maximenko, N., and Hafner, J. (2010). SCUD, Surface Currents From Diagnostic Model, IPRC Technical Note No. 5. Available online at: http://iprc.soest.hawaii.edu/publications/tech_notes/SCUD_manual_02_17.pdf (accessed on February 16, 2010)
Maximenko, N., Hafner, J., and Niiler, P. (2012). Pathways of marine debris derived from trajectories of Lagrangian drifters. Mar. Poll. Bull. 65, 51–62. doi: 10.1016/j.marpolbul.2011.04.016
Maximenko, N. A., Hafner, J., Kamachi, M., and MacFadyen, A. (2018). Numerical simulations of debris drift from the 2011 tsunami in Japan, verified with boat reports. Mar. Pollut. Bull. 132, 5–25. doi: 10.1016/j.marpolbul.2018.03.056
McGrath, J. S., Honrado, C., Spencer, D., Horton, B., Bridle, H. L., and Morgan, H. (2017). Analysis of parasitic protozoa at the single-cell level using microfluidic impedance cytometry. Sci. Reports 2017:7. doi: 10.1038/s41598-017-02715-y
McWilliams, J. C. (2016). Submesoscale currents in the ocean. Proc. R. Soc. A 472:20160117. doi: 10.1098/rspa.2016.0117
Meyerjürgens, J., Badewien, T. H., Garaba, S. P., Wolff, J. O., and Zielinski, O. (2019). A state-of-the-art compact surface drifter reveals pathways of floating marine litter in the German bight. Front. Marine Sci. 6:58. doi: 10.3389/fmars.2019.00058
Miller, J. (2018). Antarctica is not protected from an influx of new species. Phys. Today 71, 14–16. doi: 10.1063/PT.3.4012
Moller, D. (2016). “SAR and IfSAR observational concepts for marine debris mapping and oceanic monitoring,” in Presentation at Workshop on Mission Concepts for Marine Debris Sensing (Honolulu, HI). Available online at: http://iprc.soest.hawaii.edu/NASA_WS_MD2016/pdf/Moller2016.pdf (accessed August 10, 2019).
Molnar, J. L., Gamboa, R. L., Revenga, C., and Spalding, M. D. (2008). Assessing the global threat of invasive species to marine biodiversity. Front. Ecol. Environ. 6, 485–492. doi: 10.1890/070064
Mouat, J., Lozano, R. L., and Bateson, H. (2010). Economic Impacts of Marine Litter. KIMO International. Retrieved from: http://www.kimointernational.org/wp/wp-content/uploads/2017/09/KIMO_Economic-Impacts-of-Marine-Litter.pdf (accessed on November 29, 2013).
Moy, K., Neilson, B., Chung, A., Meadows, A., Castrence, M., Ambagis, S., et al. (2018). Mapping coastal marine debris using aerial imagery and spatial analysis. Mar. Poll. Bull. 132, 52–59. doi: 10.1016/j.marpolbul.2017.11.045
MSFD (2013). Marine Strategy Framework Directive GES Technical Group on Marine Litter. Guidance on Monitoring of Marine Litter in European Seas, Luxembourg. Available online at: https://mcc.jrc.ec.europa.eu/documents/201702074014.pdf
Murray, C. C., Maximenko, N., and Lippiatt, S. (2018). The influx of marine debris from the Great Japan Tsunami of 2011 to North American shorelines. Mar. Pollut. Bull. 132, 26–32. doi: 10.1016/j.marpolbul.2018.01.004
Nelson, H., Woods, M., Lorenz, C., Gerdts, G., Fields, D., Matrai, P., et al. (2018). “Use of Imaging Flow Cytometry (FlowCam) in the Study of Microplastics,” in Ocean Sciences Meeting. (Portland, OR: Oregon Convention Center).
Newman, S., Watkins, E., Farmer, A., ten Brink, P. J., and Schweitzer, P. (2015). “The economics of marine litter,” in Marine Anthropogenic Litter, eds. M. Bergmann, L. Gutow, and M. Klages (Cham: Springer Open; Elsevier), 367–394. doi: 10.1007/978-3-319-16510-3_14
Niiler, P. P. (2001). “The world ocean surface circulation,” in Ocean Circulation and Climate. International Geophysics Series, Vol. 77, eds G. Siedler, J. Church, and J. Gould (New York, NY: Academic Press), 193–204. doi: 10.1016/S0074-6142(01)80119-4
Olson, R. J., Shalapyonok, A., Kalb, D. J., Graves, S. W., and Sosik, H. M. (2017). Imaging flowcytobot modified for high throughput by in-line acoustic focusing of sample particles. Limnol. Oceanogr. Methods 15:10. doi: 10.1002/lom3.10205
Peeken, I., Primpke, S., Beyer, B., Gütermann, J., Katlein, C., Krumpen, T., et al. (2018). Arctic sea ice is an important temporal sink and means of transport for microplastic. Nat Commun. 9:1505. doi: 10.1038/s41467-018-03825-5
Peltzer, E. T., Zhang, X., Walz, P. M., Luna, M., and Brewer, P. G. (2016). In situ Raman measurement of HS- and H2S in sediment pore waters and use of the HS-:H2S ratio as an indicator of pore water pH. Mar. Chem. 2016:184. doi: 10.1016/j.marchem.2016.05.006
Pieper, C., Amaral-Zettler, L., Law, K. L., Loureiro, C. M., and Martins, A. (2019). Application of matrix scoring techniques to evaluate marine debris sources in the remote islands of the azores archipelago. Environ. Poll. 249, 666–675. doi: 10.1016/j.envpol.2019.03.084
Provencher, J. F., Ammendolia, J., Rochman, C. M., and Mallory, M. L. (2018). Assessing plastic debris in aquatic food webs, what we know and don't know about uptake and trophic transfer. Environ. Rev. doi: 10.1139/er-2018-0079
Purser, A., Thomsen, L., Barnes, C., Best, M., Chapman, R., Hofbauer, M., et al. (2013). Temporal and spatial benthic data collection via an internet operated deep sea crawler. Methods Oceanogr. 5, 1–18. doi: 10.1016/j.mio.2013.07.001
Putman, N. F., Goni, G. J., Gramer, L. J., Hu, C., Johns, E. M., Trinanes, J. Wang, et al. (2018). Simulating transport pathways of pelagic sargassum from the Equatorial Atlantic into the Caribbean Sea. Progr. Oceanogr. 165, 205–214. doi: 10.1016/j.pocean.2018.06.009
Robinson, J., New, A. L., Popova, E. E., Srokosz, M. A., and Yool, A. (2017). Far-field connectivity of the UK's four largest marine protected areas, Four of a kind? Earth Fut. 5, 475–494. doi: 10.1002/2016EF000516
Royer, S. J., Ferrón, S., Wilson, S. T., and Karl, D. M. (2018). Production of methane and ethylene from plastic in the environment. PLoS ONE 13:e0200574. doi: 10.1371/journal.pone.0200574
Ryan, P. G. (2015). “A brief history of marine litter research,” in Marine Anthropogenic Litter, eds. M. Bergmann, L. Gutow, and M. Klages (Cham: Springer Open; Elsevier), 1–25. doi: 10.1007/978-3-319-16510-3_1
Scholin, C. A., Birch, J., Jensen, S., Marin, R., Massion, E., Pargett, D., et al. (2017).the quest to develop ecogenomic sensors a 25-year history of the environmental sample processor (esp) as a case study. Oceanography 30:427. doi: 10.5670/oceanog.2017.427
Sgier, L., Freimann, R., Zupanic, A., and Kroll, A. (2016). Flow cytometry combined with viSNE for the analysis of microbial biofilms and detection of microplastics. Nat. Commun. 7:11587. doi: 10.1038/ncomms11587
Shim, W. J., Song, Y. K., Hong, S. H., and Jang, M. (2016). Identification and quantification of microplastics using Nile Red staining. Mar. Pollu. Bull. 113:49. doi: 10.1016/j.marpolbul.2016.10.049
Sosik, H. M. (2017). Life in the plankton, stories from automated submersible microscopy and flow cytometry. Integr. Compar. Biol. 57. Available online at: http://www.sicb.org/meetings/2017/schedule/abstractdetails.php?id=1946
Spencer, D., Caselli, F., Bisegna, P., and Morgan, H. (2016). High accuracy particle analysis using sheathless microfluidic impedance cytometry. Lab Chip. 16:13. doi: 10.1039/C6LC00339G
Spencer, D., Elliott, G., and Morgan, H. (2014). A sheath-less combined optical and impedance micro-cytometer. Lab Chip 14, 3064–3073. doi: 10.1039/C4LC00224E
Tekman, M. B., Krumpen, T., and Bergmann, M. (2017). Marine litter on deep Arctic seafloor continues to increase and spreads to the North at the HAUSGARTEN observatory. Deep Sea Res. I Oceanogr. Res. Papers 120:11. doi: 10.1016/j.dsr.2016.12.011
Thompson, R. C., and Maximenko, N. (2016). “Plastic pollution in the marine environment,” in Future of the Ocean and Its Seas, a Non-Governmental Scientific Perspective on Seven Marine Research Issues of G7 Interest, eds. P. Williamson, D. Smythe-Wright, and P. Burkill (Paris: ICSU-IAPSO-IUGG-SCOR), 12–18.
Topouzelis, K., Papakonstantinou, A., and Garaba, S. P. (2019). Detection of floating plastics from satellite and unmanned aerial systems (plastic litter project 2018). Int. J. Appl. Earth Observ. Geoinform. 79, 175–183. doi: 10.1016/j.jag.2019.03.011
Trinanes, J. A., Olascoaga, M. J., Goni, G. J., Maximenko, N. A., Griffin, D. A., and Hafner, J. (2016). Analysis of potential MH370 debris trajectories using ocean observations and numerical model results. J. Oper. Oceanogr. 9, 126–138. doi: 10.1080/1755876X.2016.1248149
van Sebille, E., England, M. H., and Froyland, G. (2012). Origin, dynamics and evolution of ocean garbage patches from observed surface drifters. Environ. Res. Lett. 7:044040. doi: 10.1088/1748-9326/7/4/044040
van Sebille, E., Wilcox, C., Lebreton, L., Maximenko, N. A., Hardesty, B. D., van Franeker, J. A., et al. (2015). A global inventory of small floating plastic debris. Environ. Res. Lett. 10:124006. doi: 10.1088/1748-9326/10/12/124006
Veenstra, T. S., and Churnside, J. H. (2012). Airborne sensors for detecting large marine debris at sea. Mar. Pollut. Bull. 65, 1–3. doi: 10.1016/j.marpolbul.2010.11.018
Venrick, E. L., Backman, T., Bartram, W. C., Platt, C. J., Thornhill, M. S., and Yates, R. E. (1973). Man-made objects on the surface of the Central North Pacific ocean. Nature 241, 271–271. doi: 10.1038/241271a0
Wang, W., and Wang, J. (2018). Investigation of microplastics in aquatic environments, An overview of the methods used, from field sampling to laboratory analysis. Trends Anal. Chem. 108:26. doi: 10.1016/j.trac.2018.08.026
Keywords: plastics, marine debris, sensor development, observing network, ecosystem stressors, maritime safety
Citation: Maximenko N, Corradi P, Law KL, Van Sebille E, Garaba SP, Lampitt RS, Galgani F, Martinez-Vicente V, Goddijn-Murphy L, Veiga JM, Thompson RC, Maes C, Moller D, Löscher CR, Addamo AM, Lamson MR, Centurioni LR, Posth NR, Lumpkin R, Vinci M, Martins AM, Pieper CD, Isobe A, Hanke G, Edwards M, Chubarenko IP, Rodriguez E, Aliani S, Arias M, Asner GP, Brosich A, Carlton JT, Chao Y, Cook A-M, Cundy AB, Galloway TS, Giorgetti A, Goni GJ, Guichoux Y, Haram LE, Hardesty BD, Holdsworth N, Lebreton L, Leslie HA, Macadam-Somer I, Mace T, Manuel M, Marsh R, Martinez E, Mayor DJ, Le Moigne M, Molina Jack ME, Mowlem MC, Obbard RW, Pabortsava K, Robberson B, Rotaru A-E, Ruiz GM, Spedicato MT, Thiel M, Turra A and Wilcox C (2019) Toward the Integrated Marine Debris Observing System. Front. Mar. Sci. 6:447. doi: 10.3389/fmars.2019.00447
Received: 21 November 2018; Accepted: 05 July 2019;
Published: 28 August 2019.
Edited by:
Sanae Chiba, Japan Agency for Marine-Earth Science and Technology, JapanReviewed by:
Hans-Peter Plag, Old Dominion University, United StatesRene Garello, IMT Atlantique Bretagne-Pays de la Loire, France
At least a portion of this work is authored by Rick Lumpkin, Anna-Marie Cook, Gustavo Goni, Bill Robberson, and Gregory Ruiz on behalf of the U.S. Government and, as regards Dr. Lumpkin, Mrs. Cook, Dr. Goni, Mr. Robberson, Dr. Ruiz, and the U.S. Government, is not subject to copyright protection in the United States. Foreign and other copyrights may apply. This is an open-access article distributed under the terms of the Creative Commons Attribution License (CC BY). The use, distribution or reproduction in other forums is permitted, provided the original author(s) and the copyright owner(s) are credited and that the original publication in this journal is cited, in accordance with accepted academic practice. No use, distribution or reproduction is permitted which does not comply with these terms.
*Correspondence: Nikolai Maximenko, bWF4aW1lbmtAaGF3YWlpLmVkdQ==