- Phytoplankton and Microorganisms Laboratory, Institute of Oceanography, Federal University of Rio Grande, Rio Grande, Brazil
Harmful blooms of the cyanobacterium Nodularia spumigena occur in several parts of the world, and this species is notorious for its ability to produce cyanotoxins, such as nodularin. This species is also able to perform akinete differentiation, thus favoring the success of their populations under adverse conditions. In southern Brazil, N. spumigena has developed large blooms in experimental shrimp production farms. In this study, we implemented an experiment to evaluate the influence of salinity and temperature on several physiological attributes of a Brazilian strain of N. spumigena: cell growth, nodularin production, the ability to form akinetes and germination potential. A factorial experiment (3 × 4) was conducted to test the effects of temperature (15, 23, and 30°C) and salinity (1, 7, 15, and 30 ppm) on the growth, production of nodularin and the differentiation of akinetes. The germination potential of the akinetes was tested after incubation for 30, 60, 90, 120, 180, and 360 days at 4°C in the dark. N. spumigena grew under a wide range of salinity and temperatures, but salinity had a greater influence. The highest cell densities at the temperatures tested were observed at salinity 7 ppm and the lowest at salinity 1 ppm. The toxin nodularin was produced in all treatments, but there was an inverse relationship between the content of nodularin per cell and cell density. The akinetes were also differentiated in all the treatments, with the exception of S1T30. However, the largest proportion of akinetes (65% of the cells) was observed in the treatment with low cell growth at salinity 15 and 15°C, possibly indicating an effort of the population to survive over the long term. The akinetes germinated in all treatments, but those cultivated at salinity 7 and 15 ppm (except S15T30) showed a higher percentage of germination and, in contrast, the lowest germination rate was observed in the treatments with salinity 1 ppm. Thus, the low salinity (1) at all the temperatures tested has the potential to inhibit N. spumigena blooms, since it hampers cell growth and the formation of akinetes.
Introduction
The planktonic cyanobacterium Nodularia spumigena Mertens ex Bornet and Flahault develops important blooms in several areas in the world (Blackburn et al., 1996; Van Buynder et al., 2001; Rakko and Seppälä, 2014). The first toxic bloom of N. spumigena was reported in southern Australia in the lakes that form the Murray estuary, in particular, Alexandrina Lake (Francis, 1878). In Brazil, blooms of this species were recently documented for the first time (Costa et al., 2013), reaching very high abundances in nursery ponds of the shrimp Penaeus vannamei Boone, 1931, in which they caused a reduction in the growth and survival rates. The identification of N. spumigena was confirmed using molecular methods and ultrastructural analysis (Popin et al., 2016; Silveira et al., 2017).
Among the common characteristics to the Order Nostocales, N. spumigena can differentiate its cells in heterocytes (molecular nitrogen fixation cells) and akinetes (resistance spores) (Komárek, 2013; Silveira et al., 2017). Both kinds of cells provide important advantages. Heterocytes favor the growth of N. spumigena under nitrogen low concentrations, while the akinetes remain viable for long periods in the sediment and can germinate in response to changes in environmental conditions (Hansson, 1993, 1996; Myers et al., 2010). The germinated akinetes may function as inoculum for the initiation of a harmful algal bloom and thus, represent an important mechanism of population propagation (Kim et al., 2005). In addition, this species can produce the toxin nodularin, a hepatotoxic cyclic pentapeptide (Rinehart et al., 1988), which accumulates in the animal liver and can act as a carcinogen in mammals (Carmichael, 1992; Ohta et al., 1994). Nodularin also causes the enzymatic inhibition of the eukaryotic proteins phosphatase 1 and 2A (Honkanen et al., 1991). N. spumigena can also produce other bioactive compounds, such as spumigins, aeruginosins, pseudoaeruginosins, and anabaenopeptins (Mazur-Marzec et al., 2016).
Important environmental factors that govern the distribution and bloom development of N. spumigena are salinity and temperature, light quality and intensity, and nutrient concentration. Salinity is considered to be a controlling factor of cyanobacteria in general (Kononen, 1992; Lehtimäki et al., 1994), affecting photosynthesis, the functioning of the plasma membrane (Singh et al., 2002) and therefore, cell growth (Moisander et al., 2002). In addition, the cyanobacterial morphology and life cycle vary in response to fluctuations in salinity (Iranshahi et al., 2014), as well as the turgor pressure on the cell (Ladas and Papageorgiou, 2000). It has been demonstrated that N. spumigena tolerates a large variation in water salinity (0–35 ppm), but its optimal growth differs between strains analyzed in the Baltic Sea and lakes and lagoons in Australia (Blackburn et al., 1996).
It is widely known that the temperature is directly related to the growth rate of cyanobacteria (Mackey et al., 2013) and that this relationship varies among species (Reynolds, 1984). The temperature also influences the toxin production of N. spumigena. It was shown that toxin production was favored at lower temperatures than cellular growth. Thus, a higher concentration of the intracellular toxin was observed in cells grown at 10°C, while the maximum biomass production was observed at 20–30°C (Hobson and Fallowfield, 2003). Nodularia strains isolated in Brazilian shrimp ponds were tested for their growth and toxin production under controlled conditions of salinity (7 ppm) and temperature (23°C) (Silveira et al., 2017). However, the optimal environmental conditions for the Brazilian strain and how they compare to strains from other sites in the world are not yet known. Phylogenetic analyses based on its 16S rRNA gene indicated that the Brazilian strain is closely related to the strains from Australia (Popin, 2017; Silveira et al., 2017). Alternatively, based on the analysis of genome, 31 proteins of this same Brazilian strain were grouped with the Baltic Sea strain (Popin, 2017). Currently, there is a lack of accessible data on the NCBI GenBank with only one Baltic Sea N. spumigena strain available for comparison, and thus, it is important to provide information on the strains from other geographical regions.
It is known that salinity and temperature influence the growth of N. spumigena. However, different strains respond differently to environmental variables. In this study, the effects of salinity and temperature on the growth, production of the nodularin toxin, and akinete differentiation were evaluated, as well as the germination potential of a strain of N. spumigena isolated from a shrimp production pond in southern Brazil. The results of this study will improve the understanding of the physiology and management of recurrent N. spumigena blooms in the environment and in aquaculture ponds, in which they cause great damage.
Materials and Methods
Species Isolation and Stock Culture
Water samples containing Nodularia trichomes were collected on December 5, 2013 in production ponds of the marine shrimp P. vannamei at the Marine Aquaculture Station (EMA) of the Federal University of Rio Grande-FURG, Brazil. The isolated trichome gave rise to the NODSPU1 strain, which was identified as N. spumigena (Silveira et al., 2017). The strain was cultivated in f/2 medium (Guillard, 1975) at salinity 7 ppm in incubators with a light cycle control (12:12 h light-dark), at 80 μmol m-2 s-1 of light intensity supplied by white light fluorescent lamps (OSRAM, 20 W) and constant temperature (23 ± 1°C). The culture temperature and salinity values corresponded to those registered in the ponds on the sampling day.
Acclimation and Experimental Design
The conditions were gradually modified for the acclimation process every 7 days using the stock culture as the starting point. The cultures were acclimated at the test temperatures (15, 23, and 30°C) and salinities (1, 7, 15, and 30 ppm) through at least three transfers.
A factorial experiment (3 × 4) was conducted to concomitantly test the effect of temperature and salinity on the growth of N. spumigena using the f/2 medium and a light intensity of 80 μmol m-2 s-1. Cultures for the experiments (three replicates) were maintained in incubators with a light cycle control (12:12 h light: dark) provided by white light fluorescent lamps (OSRAM, 20 W). The light intensity in the incubators was measured using a LI-COR (LI-1400) spherical sensor. All the cultures were prepared in 500 ml of f/2 culture medium. To standardize the initial cell concentration, the volume of inoculum added corresponded to an optical density of 0.008 determined at absorbance 680 nm in a spectrophotometer (Varian Cary 1E) (Ma et al., 2006). The experiment lasted 40 days.
Cell Density, Growth, and Cyanotoxin Production
The growth was determined daily during the first four culture days based on the cell density, optical density and chlorophyll-a (Chla) content and subsequently every 48 and 72 h until the end of the experiments. The Chla content was determined in culture samples (5–10 mL) by retaining cells in glass fiber filters (Whatman GF/F, 25 mm) using a vacuum pump. The filters were stored in darkened flasks and kept in absolute methanol for 24 h in a freezer. The Chla concentration (μg L-1) was determined by measuring the absorbance at 665 and 750 nm as described by Mackinney (1941). Mean concentration of chlorophyll-a per cell (pg cell-1) at the start, middle and end of growth curves was performed by dividing the value of chlorophyll-a by cell density. At the end of the logarithmic growth phase, the biomass was also determined using the dry weight (DW) and ash-free dry weight (AFDW) as described by Zhu and Lee (1997).
The correlation between cell density and optical density was higher (r = 0.93) compared to the correlation between cell density and Chla concentration (r = 0.84) and between Chla concentration and optical density (r = 0.87), but all correlations were significant (Pearson correlation, Figure 1). We presented the growth curves based solely on cell density for clarity. The growth rate (μ) was calculated as the slope of the linear regression between the time and cell density (Ln scale) during the exponential phase (Wood et al., 2005).
The density of the trichomes, vegetative cells and akinetes were estimated in samples fixed with acetic Lugol’s solution in Sedgewick Rafter chambers using an inverted microscope (Olympus IX51; 200×). To estimate the number of vegetative cells per trichome, the total size of each trichome was measured, discounting the average size of the heterocysts and akinetes present, and divided by the average size of the vegetative cells. The counting error was maintained at a maximum of 20% and verified by estimating the coefficient of variation between the fields counted. The size of the vegetative cells (n = 50) and the akinetes (n = 30) were determined using an inverted microscope (Olympus IX71; 400×), and the cell volume was calculated assuming the geometric shape of a cylinder (Sun and Liu, 2003).
The toxin concentration was determined at each experimental treatment at the beginning of the stationary phase using an immunoassay ELISA plate kit for nodularin (Beacon Analytical Systems, Inc.). The concentration of nodularin (μg L-1) was determined using the absorbance (450 nm) in a plate photometer (Quick ELISA Drake Instruments) as described by the manufacturer.
Akinete Isolation
Cultures of N. spumigena were maintained until the stationary/decline phase in salinity 15 ppm and temperature 15°C (S15T15). This condition was shown to favor the akinete differentiation as described above. The akinetes produced were separated from the vegetative cells as described by Sukenik et al. (2007). Cultures of N. spumigena with akinetes were harvested by suction, suspended and washed in Tris buffer (3×; 50 mM, pH 7.5, containing 10 mM EDTA and 1 mM Mg Cl2). The biomass was washed in EDTA-free Tris buffer (50 mM pH 7.5, 1 mM MgCl2) containing 5 mg/mL lysozyme, incubated (2 h, 37 ± 1°C) and centrifuged (3 min, 2000 rpm). The pellet was washed three times with EDTA-free Tris buffer to remove the cell debris, sonicated (3×, 10 s, 30 kHz), and the sample was examined under an optical microscope to confirm the separation of the akinetes. The material was centrifuged (10 min, 3000 rpm), and the pellet was suspended in ultrapure water and stored in a refrigerator (4°C) in the dark (Myers et al., 2010).
Germination tests were performed after incubation of the akinetes at 30, 60, 90, 120, 180, and 360 days maintained in the dark, salinity 15 and temperature 15°C.
Germination Tests
After incubation in the dark for different time periods, 100 akinetes for each treatment (in triplicate) were inoculated in 2.5 mL f/2 culture medium in cell culture plates under the microscope and maintained in incubators (light intensity 80 μmol m-2 s-1). The effects of both temperature (15, 23 and 30°C) and salinity (1, 7, 15, and 30 ppm) were tested on the germination potential of the akinetes. The akinetes were exposed to the experimental conditions for 72 and 192 h, and the number of germinated akinetes was determined in the cell culture plates using an optical microscope (200×).
Statistical Analysis
To determine the cell density differences between treatments, a two-way ANOVA was applied and the Tukey’s a posteriori test to specify the differences at 95% significance level (Zar, 2010). The data of the percentage of akinetes, cell volume, DW and AFDW, Chla L-1, Chla cell-1, toxin concentration and akinete germination were analyzed using a one-way ANOVA and Tukey’s a posteriori test to specify the differences at 95% significance level. All data were tested for their normality and homoscedasticity (Cochran C., Hartley e Bartlett). The cell density, akinete percentage, cell volume of the vegetative cells and Chla cell-1 were transformed using logarithmic transformations and the square root for the cell volume of the akinetes to ensure normal distributions.
Results
Cell Density and Growth
The comparison between the N. spumigena growth curves shows that both the time to reach the maximum cell density and the maximum values differed among the treatments (Figure 2; ANOVA p < 0.001, F 435.3; Supplementary Table 1). The highest value (1.9 × 106 cells mL-1) was recorded at the highest values of salinity and temperature (S30T30) (Figure 2D), followed by treatments with salinity 7 ppm at the three temperature values (S7T30, S7T15, S7T23) (Figure 2B). At the lowest salinity level (S1) (Figure 2A), the cultures showed practically no growth and either collapsed within a week (S1T30) or remained at low density for approximately 1 month (S1T23, S1T15). At salinity 15 ppm (Figure 2C), the cultures persisted for approximately 1 month at low density (S15T15, S15T23) or died within 2 weeks (S15T30). The growth rates (μ) varied between 0.2 (S1T23) and 0.4 d-1 (S7T30, S30T30).
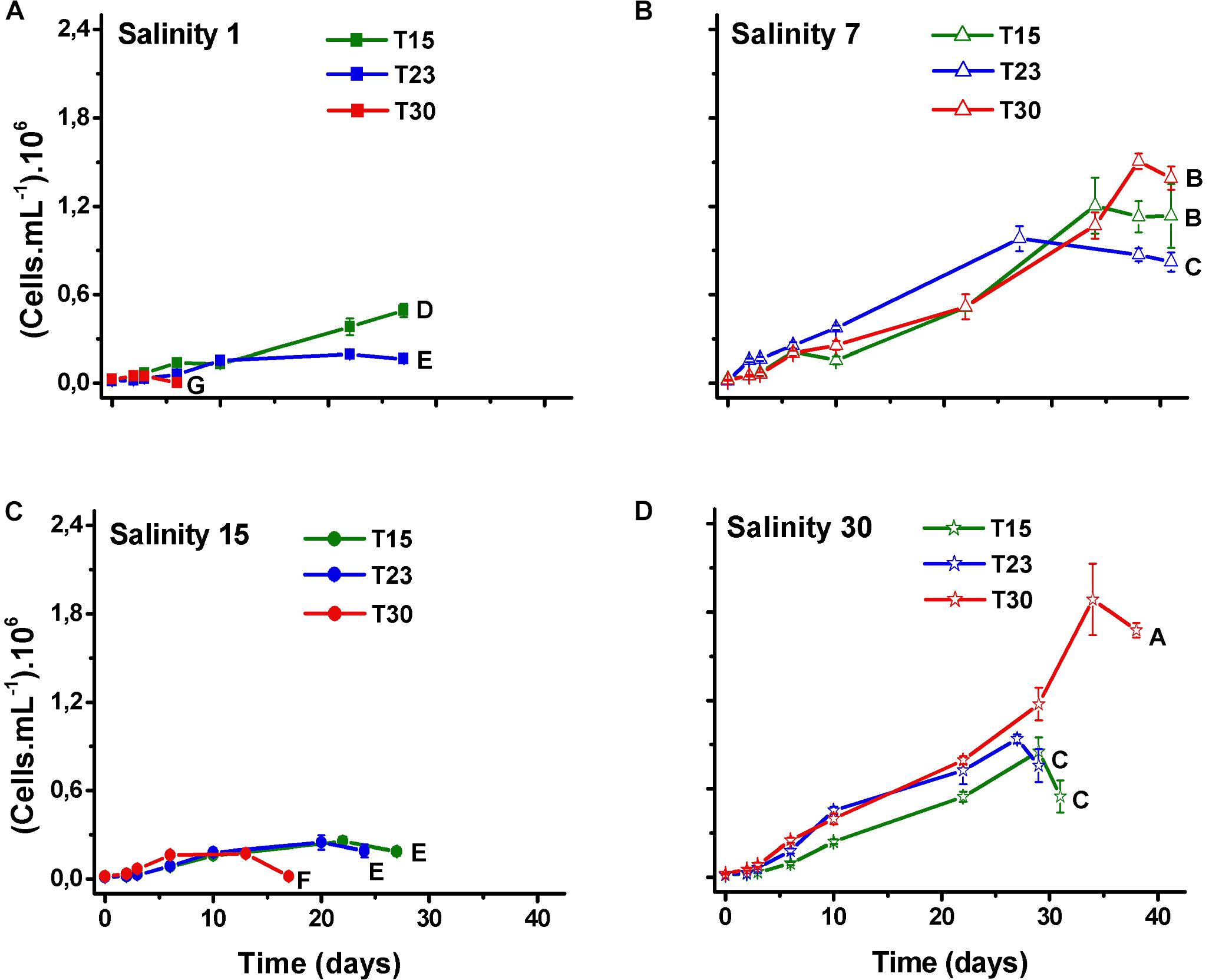
Figure 2. Mean values of cell density (±SD; n = 3) of N. spumigena (NODSPU1) observed along the time in the factorial experiment, which analyzed the influence of temperature (T15, T23, T30°C) and salinity S1 (A), S7 (B), S15 (C), and S30 (D). Significant difference between treatments is indicated by letters (ANOVA, Tukey’s p < 0.05).
As expected, the number of trichomes also increased over time in all the treatments, except in those treatments that rapidly died (S1T30; S15T30; Figure 3). The number of cells per trichome remained relatively constant or decreased, indicating that the trichome length was reduced over time with some variation among treatments and with time. The treatment with high salinity and temperature (S30T30), which presented the largest cell number (Figure 2) also presented the longest trichomes at the end of the experiment with 140 cells per trichome (Figure 4).
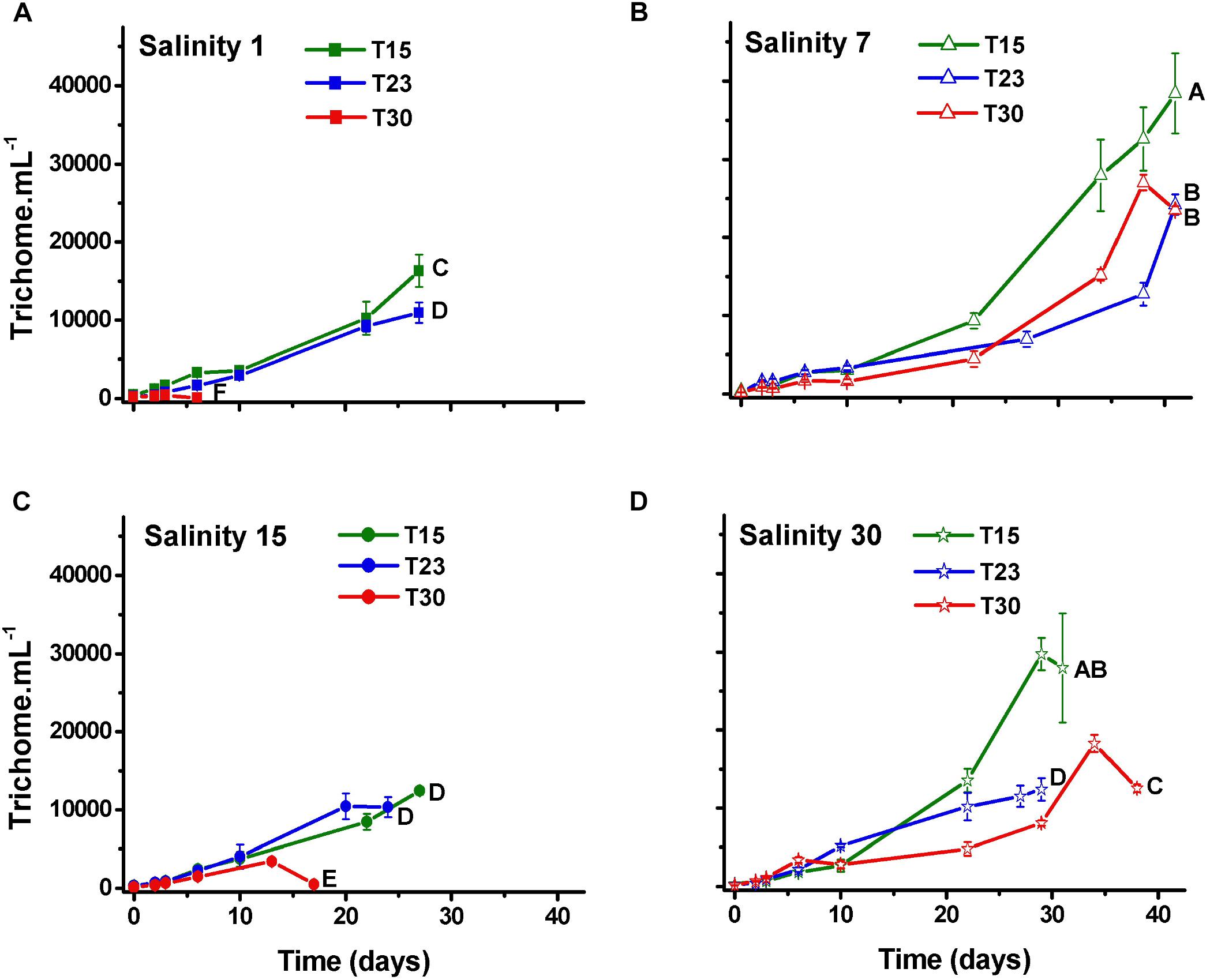
Figure 3. Trichome density (±SD; n = 3) of N. spumigena (NODSPU1) observed along the time in the factorial experiment, which analyzed the influence of temperature (T15, T23, T30°C) and salinity S1 (A), S7 (B), S15 (C), and S30 (D). Significant difference between treatments is indicated by letters (ANOVA, Tukey’s p < 0.05).
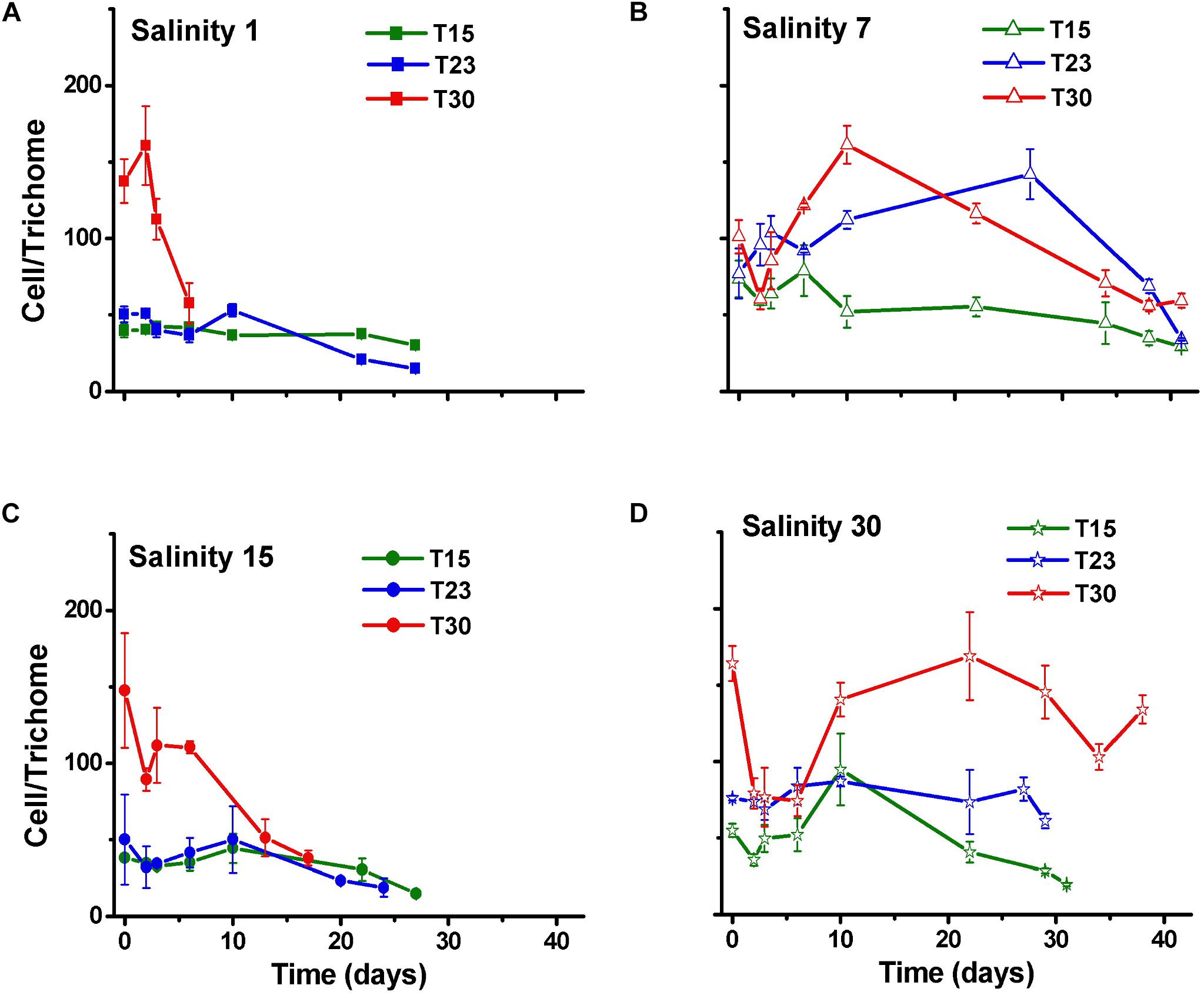
Figure 4. Cell number per trichome (±SD; n = 3) of N. spumigena (NODSPU1) observed along the time in the factorial experiment, which analyzed the influence of temperature (T15, T23, T30°C) and salinity S1 (A), S7 (B), S15 (C), and S30 (D).
The percentage of akinetes in relation to the number of cells (0.01–65.2%) increased over time, particularly in the S15T15 treatment but also in S7T15, S30T15 and S30T23. Other treatments showed low percentages of akinetes (<5%), except for S1T30, in which no akinetes were observed (Figure 5).
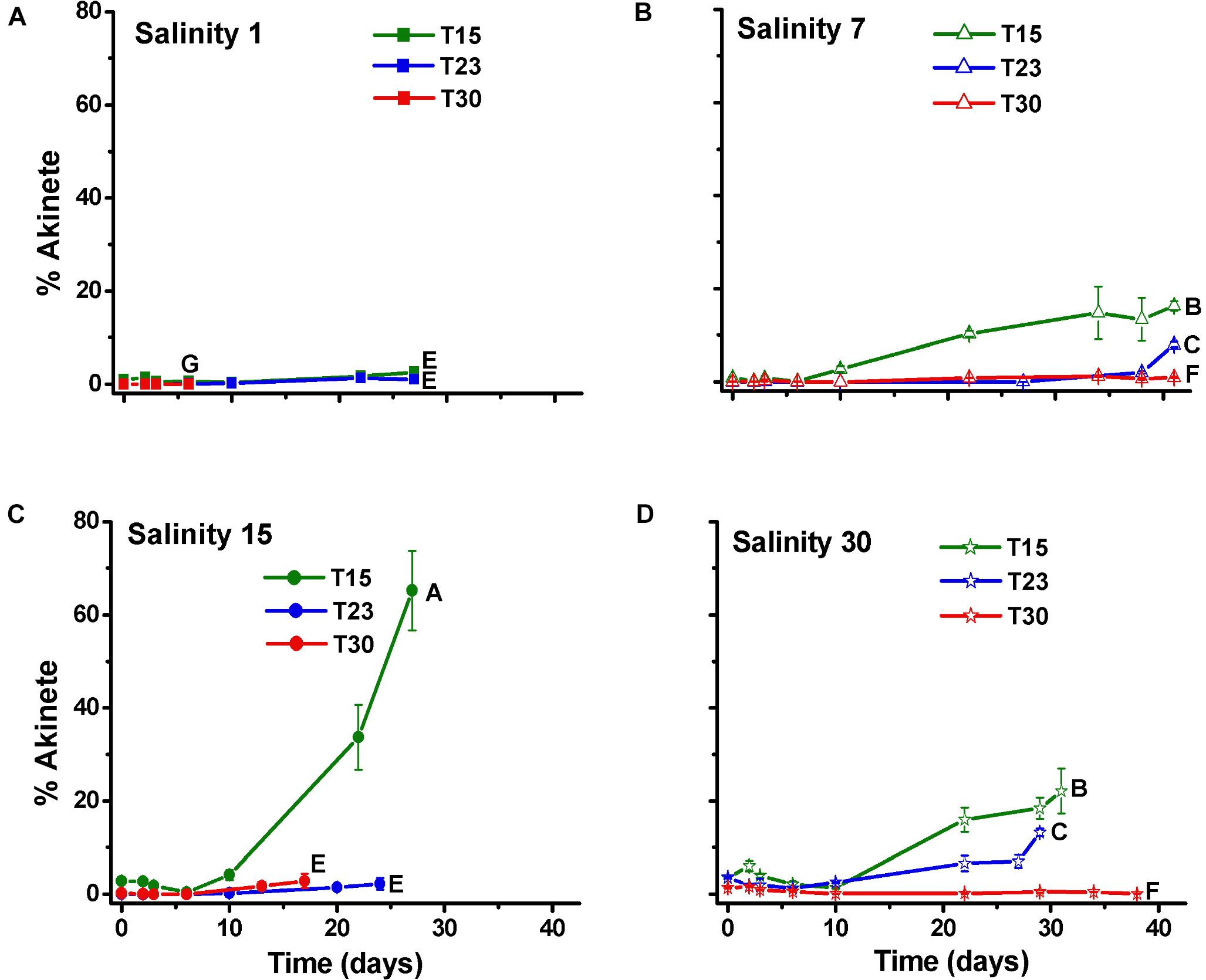
Figure 5. Percentage of akinete per cell (±SD; n = 3) of N. spumigena (NODSPU1) observed along the time in the factorial experiment, which analyzed the influence of temperature (T15, T23, T30°C) and salinity S1 (A), S7 (B), S15 (C), and S30 (D). Significant difference between treatments is indicated by letters (ANOVA, Tukey’s p < 0.05).
Biomass
The biomass values determined by both the DW and AFDW (Figure 6A) and the Chla content (Figure 6B) were generally consistent with the cell density results. All were higher in the treatments with salinity 7 and lower in salinity 1. However, the S30T30 treatment, which demonstrated the highest cell density and largest trichomes, did not correspond to the largest DW, AFDW and Chla. The lowest values of DW, AFDW and Chla were observed in high temperature treatments at salinity 1 and 15 ppm (S1T30; S15T30) in treatments in which no growth was observed. In these treatments, the Chla cell-1 (Figure 7A) was higher at the end of the experiment, as opposed to the general decreasing trend from the beginning to the end of the experiment.
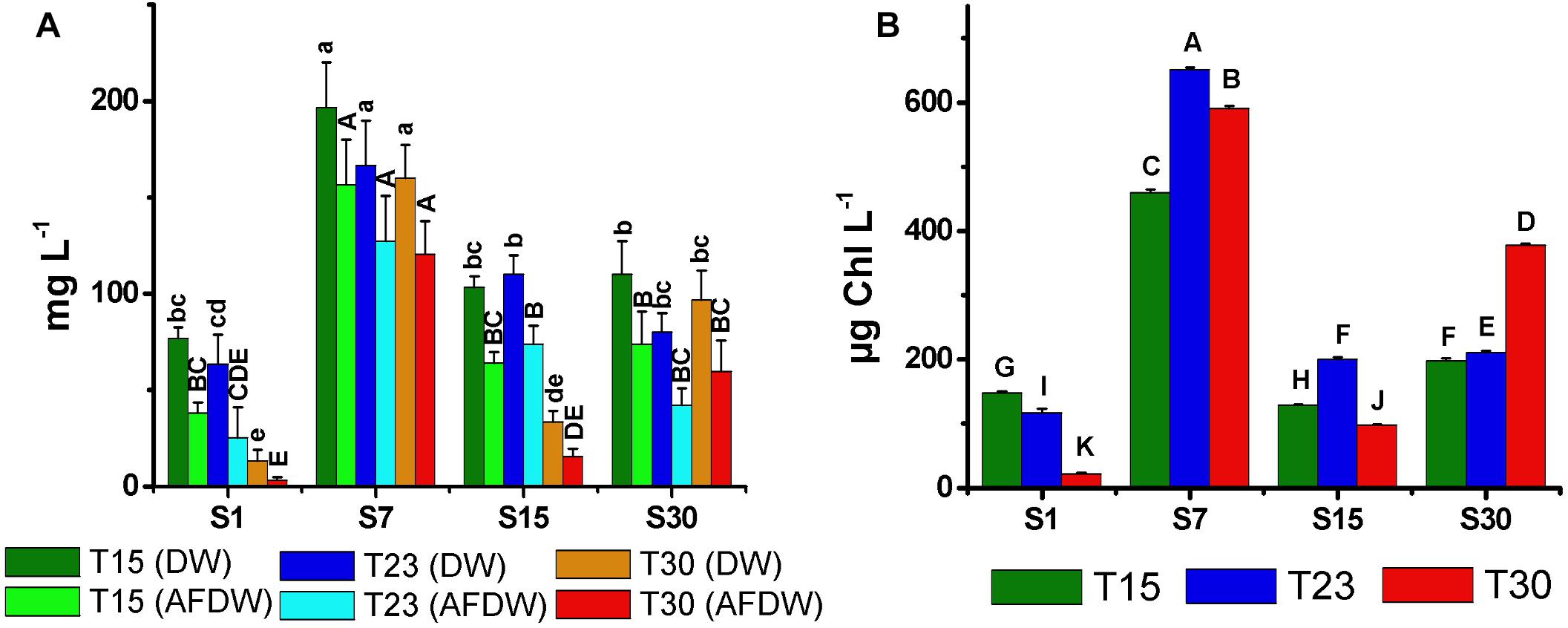
Figure 6. Total biomass dry weight (DW), ash free dry weight (AFDW) (A), and mean concentration of chlorophyll-a (B) of N. spumigena (NODSPU1) (±SD; n = 3). Significant difference between treatments is indicated by letters (ANOVA, Tukey’s p < 0.05). Lower case for DW and upper case for AFDW.
The mean volume of the vegetative cells (116–342 μm3) and akinetes (520–1054 μm3) varied without a clear pattern (Figure 7B). However, it is noteworthy that the size of vegetative cells in the salinity 30 ppm treatment decreased with increasing temperature, being largest at 15°C. This trend was inverse to the cell density, as smallest vegetative cells at temperature 30°C coincided with highest cell density (Figure 2). The mean volume of the akinetes was approximately three times larger than that of the vegetative cells and, unlike these, the largest akinetes were observed in treatments with low cell numbers (S1T23, S15T15). The smallest akinetes were observed in the high salinity and temperature treatment (S30T30) (Figure 7B).
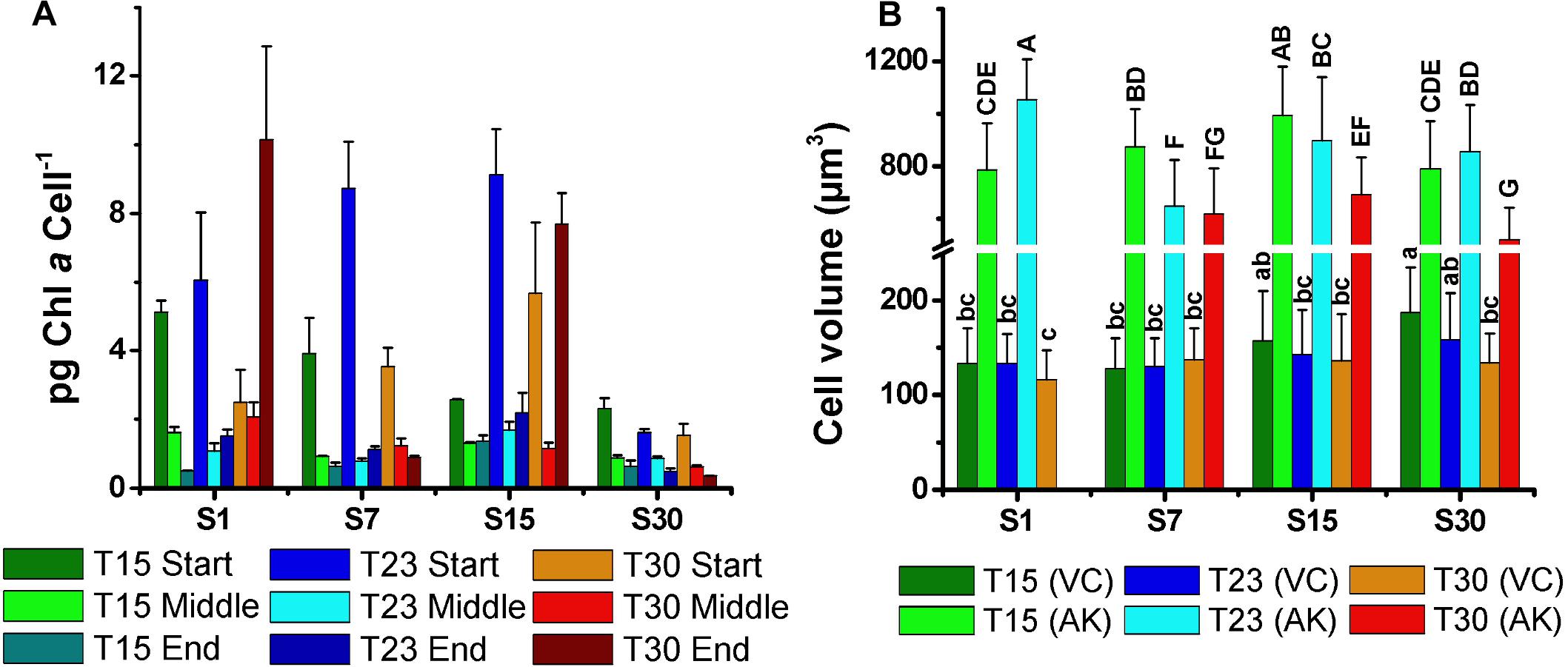
Figure 7. Mean concentration of chlorophyll-a per cell (pg cell-1) at the start, middle, and end of growth curves (A), and (B) mean cell volume of the vegetative cells (VC) and akinetes (AK) of N. spumigena (NODSPU1) (±SD; n = 3). Significant difference is indicated by letter (ANOVA, Tukey’s p < 0.05). Lower case for VC and upper case for AK.
Toxin Concentration
All the cultures of N. spumigena produced the nodularin toxin at the beginning of the stationary phase, ranging between 1.3 μg L-1 (S1T30) and 6.0 μg L-1 (S7T23) (Figure 8A). High values were observed in salinity 7 ppm (S7T15, S7T23, S7T30) associated with large cell density but also in treatments with low cell density and low salinity (S1T15, S1T23) and intermediate (S15T15). The toxin concentration per cell (2.8 × 10-9 to 3.4 × 10-7 pg cell-1) (Figure 8B) was generally lower in the treatments of salinity 7 and 30 ppm, i.e., in the treatments with higher cell density. The highest values of toxin per cell were observed at salinity 1 and 15 ppm, particularly in S1T30 (3.4 × 10-7 pg cell-1) and S15T30 (2.0 × 10-7 pg cell-1), i.e., in the treatments with low cell density.
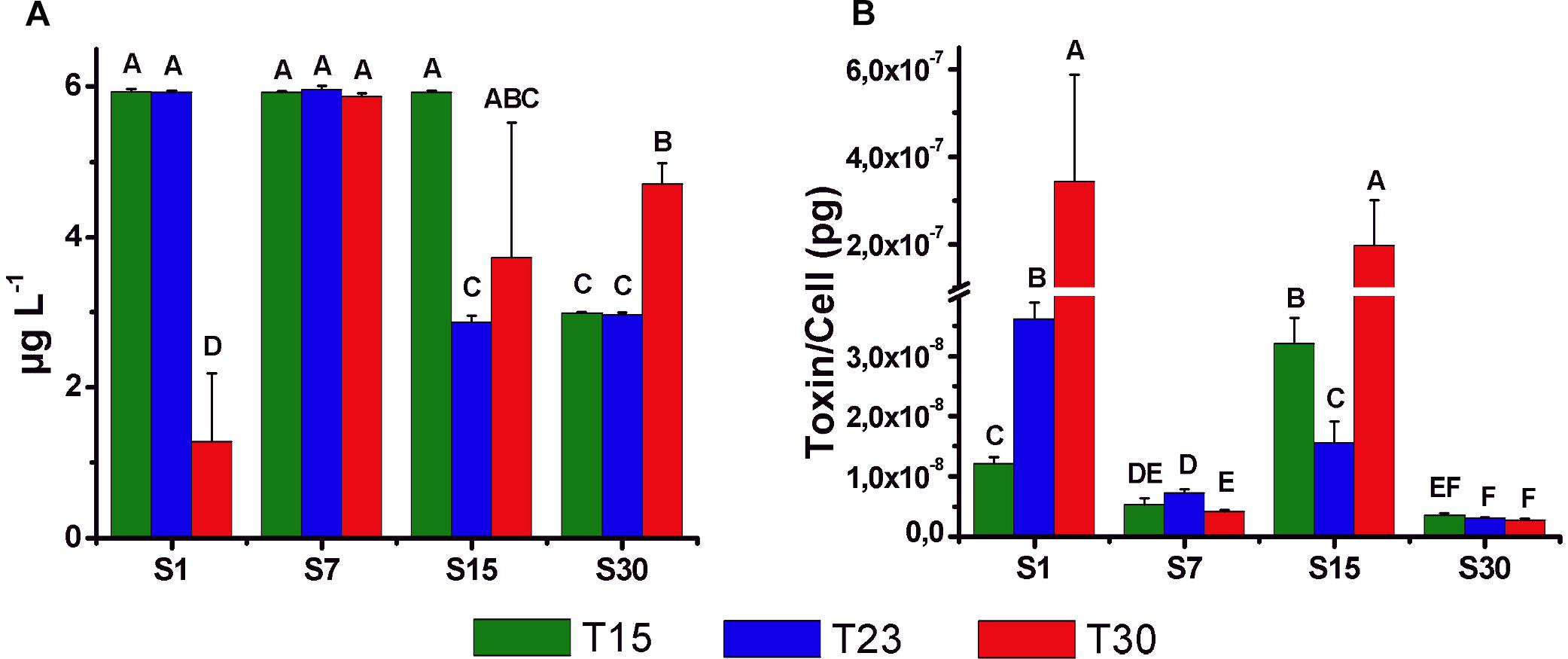
Figure 8. Mean concentration (±SD; n = 3) of intracellular nodularin (μg l-1) (A) and toxin content per cell (pg cell-1) (B) at the beginning of the stationary phase of the growth curves for different treatments. Significant differences are indicated by letters (ANOVA, Tukey’s p < 0.05).
Akinete Germination
After incubation in the dark for 30, 60, 90, 120, 180, and 360 days, akinetes exposed to light at the respective treatments germinated in a relatively high percentage (8–53%) in all the treatments (Figure 9). The same germination pattern was observed among the treatments in all the tests. The lowest percentage of germination was always observed in the treatments of salinity 1 ppm, particularly in S1T30 with the lowest germination rate. The highest germination rate was observed in treatments with salinity 7 and 15 ppm (S7T15; S7T23; S7T30; S15T15; S15T23), with the exception of S15T30. These differences between the treatments became more significant after 120 days of akinete incubation in the dark when the germination rate was the highest. The time of exposure to light (72 or 192 h) after the dark incubation did not appear to influence the germination rate.
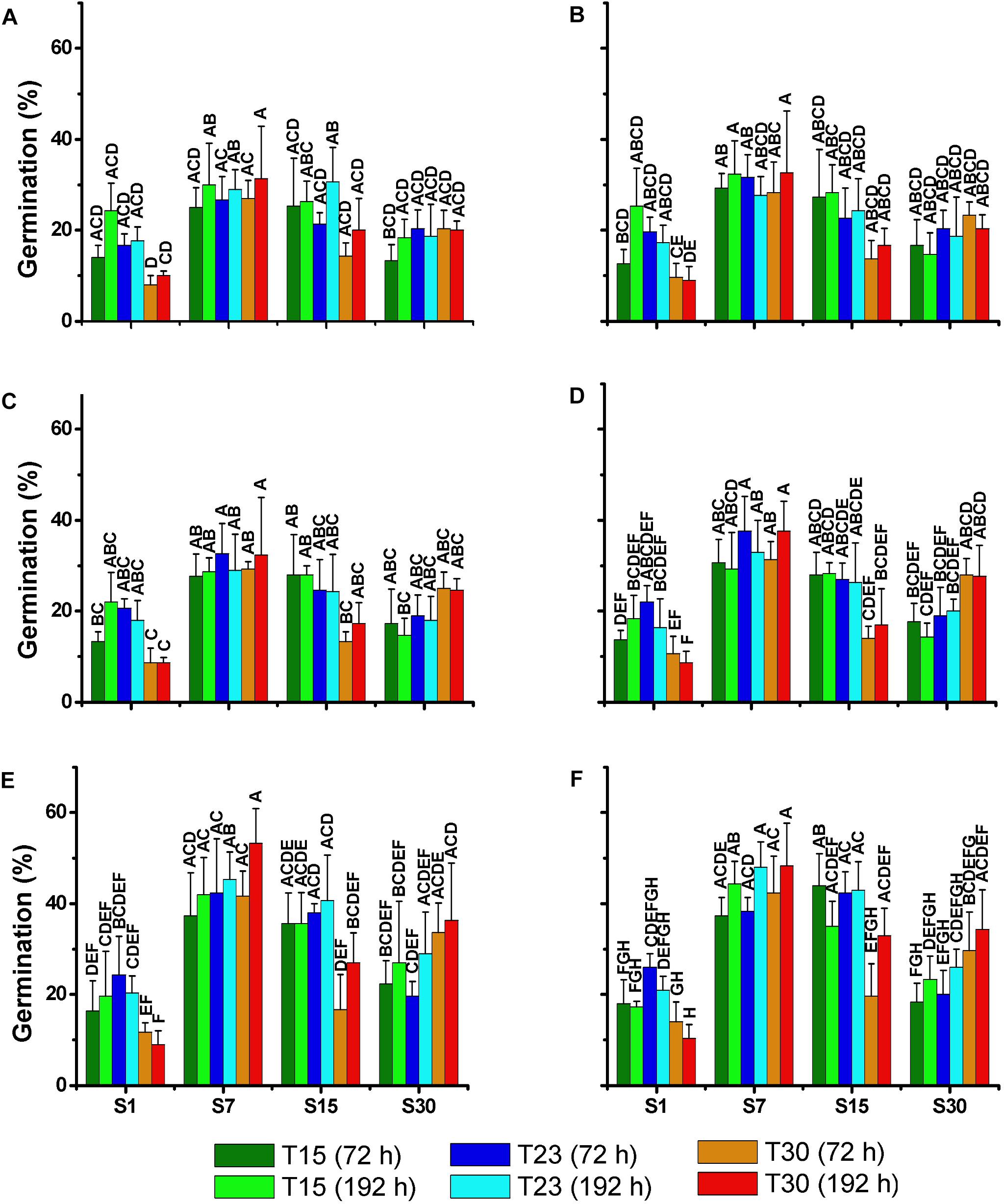
Figure 9. Mean percentage (±SD; n = 3) of akinetes germination of N. spumigena (NODSPU1) after 72 and 192 h of exposure to different treatments. Significant differences are indicated by letters. Previous to the germination experiment, dark incubation lasted for 30 (A), 60 (B), 90 (C), 120 (D), 180 (E), and 360 (F) days. Significant differences for each germination test are indicated by letters (ANOVA, Tukey’s p < 0.05).
Discussion
Cell Density, Growth, and Biomass
In our study, the highest cell densities of N. spumigena were observed in the treatments with salinity 7 and 30 ppm that demonstrated a relatively high (μ = 0.3–0.4 d-1) growth rate. In the former treatment (S7), the growth persisted for a longer period in the three test temperatures. Thus, it can be considered that this condition was more favorable for the development of N. spumigena. The cell growth in salinity 1 ppm was negligible and was different than the results obtained with two strains from the Baltic Sea that demonstrated a similar growth rate over a wide range of salinity (0–20, Moisander et al., 2002). Alternatively, studies conducted with other N. spumigena strains isolated from the Baltic Sea did not tolerate salinity values lower than 2 ppm, and the highest growth rate (μ = 0.15–0.20 d-1) was observed in salinity 8–10 ppm under conditions similar to those in our experiment (Mohlin and Wulff, 2009; Rakko and Seppälä, 2014). A strain (001E) from Australia showed a maximum rate of photosynthesis in salinity 6.6 ppm (Hobson and Fallowfield, 2001). Mazur-Marzec et al. (2005) observed a substantial difference when comparing strains from the Baltic Sea and Australia: while the Australian strain (NSPI-05) showed similar growth in treatments exposed to a wide range of salinity (3–35 ppm) with a slight inhibition in salinity 0 ppm, the Baltic Sea strain (NSGG-1), showed higher growth (μ = 0.11 d-1) in salinity 7 ppm, and negligible growth (μ = 0.05 d-1) in zero salinity. Thus, the physiology of the different Nodularia strains is complex, since strains isolated in different regions in the same country present different physiological responses. In our study, the 15 ppm salinity treatments, independently of temperature (S15T15; S15T23; S15T30), demonstrated very low growth unlike the salinity 7 and 30 ppm treatments (see Figure 2). We repeated the test with the S15 treatment (data not shown), and the same pattern was obtained. To understand this question it would be important to study the biochemical mechanisms that affect growth under the influence of differing salinities. Salinity affects the osmotic equilibrium and concentration of inorganic ions responsible for optimal cell function and growth (Teikari et al., 2018). Photosynthetic activity and cell division can be stopped with salinity changes (Moisander et al., 2002), but it is clear that the strains obtained in different environments, and even from the same one, do not always show a similar response to variations in salinity.
Our experiment on the effect of temperature showed that the Brazilian N. spumigena strain isolated grows equally well in the range tested (15–30°C) with differences related to its interactions with salinity. Studies conducted in the Baltic Sea (Pliński et al., 2007) and Lake Alexandrina, Australia (Hobson and Fallowfield, 2003) showed that temperature was more important and that values above 20°C favored bloom development, which did not happen in our study. Our experiments on growth indicated that the number of cells per trichome of N. spumigena generally decreased, particularly in the treatments that reached higher cell density. The shorter trichomes may have resulted from reproduction by hormogonia, or even by stress, as reported for Raphidiopsis brookii (Yunes et al., 2009). On exception was the high salinity and temperature treatment (S30T30) with the highest cell density of low density of longer trichomes.
Under adverse conditions in the environment, akinetes are responsible for the viability of the populations in the long term. In our experiment, the differentiation of the akinetes markedly increased in the treatments with a lower temperature (15°C), particularly at S15T15 in which 65% of the cells were akinetes, followed by S30T15 and S7T15. In S1T30, the culture did not grow well, and in the other low salinity (S1) treatments, only a few akinetes were observed (<3%). In fact, Li et al. (1997) reported that the differentiation of the akinetes in Anabaena spp. is also triggered at low temperatures. It is interesting to note that the volume of the akinetes tended to be smaller at a higher temperature (30°C) (see Figure 7B), indicating that low temperatures favor the formation not only of a large number of akinetes but also of a larger size. Salinity apparently did not influence the akinete differentiation or size, as was also found by Myers et al. (2011). However, Jones et al. (1994) reported more akinetes with increased salinity, and Mazur-Marzec et al. (2005) observed a large number of akinetes in high salinity and a reduction at zero salinity. It is likely that the interaction between temperature and salinity, and possibly other factors, are important in the control of akinete differentiation.
The distinct biomass measurements presented significant differences. For example, the S30T30 treatment with the highest cell density, presented low DW and AFDW, possibly due to the observed relatively low number of small akinetes (<5%). The high density of vegetative cells in this treatment was also not associated with Chla, which was highest in the salinity 7 ppm treatments. In the latter, highest Chla in the S7T23 did not correspond with density, which was highest in S7T30 and lowest in S7T23. These differences may be associated with the amount of Chla per cell, indicating a strong reduction compatible with cell growth over time, except for two treatments with minimal growth in high temperature (S1T30; S15T30). Given the constraints described above, and the best correlation obtained between optical density and cell density (Figure 1), we suggest using the optical density measurements for estimating the biomass of N. spumigena, instead of DW or AFDW. Chla measurements should be analyzed in conjunction with the cell density and cell size, as the latter presents a general pattern of reduction over time (Mohlin and Wulff, 2009).
Nodularin Production
Three N. spumigena strains isolated from the same shrimp ponds in southern Brazil were shown to produce nodularin (Silveira et al., 2017). It was also shown that one of the strains contains the genes to produce other bioactive compounds, such as spumigin and anabaenopeptin (Popin et al., 2016). The concentration of nodularin recorded in the stationary phase of our experiment (1.3–6.0 μg L-1) was relatively low, considering that values of 0.8–220 μg L-1 (Heresztyn and Nicholson, 1997; McGregor et al., 2012) and greater than 15,000 μg L-1 (Mazur and Pliński, 2003) had previously been found in the environment. It must be noted that the ELISA method used in our study does not detect the nodularin variants, such as NOD-R and [D-Asp’] NOD, which can be produced by our strain (Popin, 2017). Thus, the nodularin concentration found in this study is possibly underestimated. However, even low concentrations of nodularin potentially cause environmental problems, such as animal mortality, contamination of seafood, degradation of water quality (Van Buynder et al., 2001), a reduction in the production rate of copepod eggs (Karjalainen, 2005) and lower fish growth (Pääkkönen et al., 2008). In Southern Brazil, shrimp survival and growth were negatively affected in ponds with N. spumigena blooms (Costa et al., 2013).
In the present study, toxin production was not related to temperature or salinity but to cell density (see Figure 8), and the highest (underestimated) values (2.0–3.4 × 10-7 pg cell-1) were observed in the salinity treatments 15 and 1 ppm, in which cell growth was reduced. In contrast, low values of nodularin (3.5–7.3 × 10-9 pg cell-1) were recorded in salinity treatments 30 and 7 ppm with higher cell density. This type of relationship could be associated with cellular metabolism, which during the exponential phase is directed to cell growth, while there would be a deviation to toxin production during low growth conditions (Sivonen, 1996; Orr and Jones, 1998). However, Repka et al. (2001) suggested that toxin production would be positively related to cell division, and Hobson and Fallowfield (2003) observed higher production of nodularin at low temperature, indicating that the results cannot be easily generalized to other strains or conditions.
Akinete Germination
The recruitment of resistant cells from the sediment could be a key factor for the success of a species in the plankton community (Hansson, 1996). Knowledge of the conditions that favor akinete germination helps to predict cyanobacterial blooming events and represents an important step in management processes. In our study, the akinetes of N. spumigena germinated in all the treatments tested. However, the highest percentage of germination was observed in the treatments with salinity 7 and 15 ppm, in contrast to the lowest in low salinity, especially at a higher temperature (S1T30). A higher germination at salinity values between 5 and 15 ppm was also found in a strain isolated from Australia (Myers et al., 2010). In general, the effect of temperature influenced the akinete germination less than salinity in spite of the slight decrease in germination at high temperature (30°C). Huber (1985) observed that the influence of temperature on akinete germination varies depending on the species with N. spumigena demonstrating a high germination rate between 15 and 25°C. The population with the greatest potential recruitment success that was observed from the akinetes in salinity 7 matches our results of cellular growth. However, in salinity 15, high akinete germination coinciding with low cell density indicates that even without significant growth, the akinetes could potentially initiate the development of a population.
An examination of the storage time of the akinetes indicated that germination tended to increase with a longer storage period (see Figure 9), as has been observed for both N. spumigena (Myers et al., 2010) and Anabaena circinalis (Baker and Bellifemine, 2000). The longer storage period resulting in higher germination may be related to the maturation period of the akinetes (Karlsson-Elfgren et al., 2004). Yamamoto (1975) showed that the akinetes of a strain of Anabaena cylindrica survived for 5 years in a dark and dry environment.
Conclusion
The cyanobacterium N. spumigena isolated from shrimp ponds in southern Brazil grows under a wide range of salinity and temperature values, but responded more strongly to salinity. The highest cell densities at the temperatures tested were observed at salinity 7 ppm and the lowest at salinity 1 ppm. The toxin nodularin was produced in all the treatments, demonstrating an inverse relationship between the content of nodularin per cell and cell density. The akinetes were also differentiated in all the treatments, with the exception of S1T30. The largest proportion (65% of cells) occurred in the treatment S15T15 with low cell growth, possibly indicating an effort of the population to survive in the long term. The akinetes also germinated in all the treatments, particularly those cultivated at salinity 7 and 15 ppm with a higher germination percentage (except S15T30). In contrast, the lowest germination was observed in the treatments with salinity 1 ppm. Thus, the low salinity (1 ppm) at all temperatures tested has the potential to inhibit N. spumigena blooms, since it hampers cell growth and the differentiation and germination of the akinetes. These results could be useful to manage aquaculture nurseries and production ponds, in which the presence and persistence of N. spumigena has been a serious problem.
Author Contributions
SS performed all the activities, including the sampling, laboratory experiments, and the final drafting of the results. CO provided support to carry out the work from the planning and execution of the experiments to writing the final draft.
Funding
Financial support for this investigation was provided by the Brazilian Long-Term Ecological Research Program (PELD) of the Ministry of Sciences/National Research Council (CNPq, Proc. 403805/2012-0) and FAPERGS (Proc. 003122-2551/12-7) to CO and the Ministry of Education (CAPES) grant to SS.
Conflict of Interest Statement
The authors declare that the research was conducted in the absence of any commercial or financial relationships that could be construed as a potential conflict of interest.
Acknowledgments
We would like to thank Dr. Wilson Wasielesky for providing the experimental shrimp ponds of the Marine Station of Aquaculture (EMA), Federal University of Rio Grande-FURG.
Supplementary Material
The Supplementary Material for this article can be found online at: https://www.frontiersin.org/articles/10.3389/fmars.2019.00339/full#supplementary-material
References
Baker, P. D., and Bellifemine, D. (2000). Environmental influences on akinete germination of Anabaena circinalis and implications for management of cyanobacterial blooms. Hydrobiologia 427, 65–73.
Blackburn, S., McCausland, M. A., Bolch, C. J. S., Newman, S. J., and Jonas, G. J. (1996). Effect of salinity on growth and toxin production in cultures of the bloom-forming cyanobacterium Nodularia spumigena from Australian waters. Phycologia 35, 511–522. doi: 10.2216/i0031-8884-35-6-511-1
Carmichael, W. W. (1992). Cyanobacteria secondary metabolites – the cyanotoxins. J. Appl. Bacteriol. 72, 445–459. doi: 10.1111/j.1365-2672.1992.tb01858.x
Costa, C. M., Fóes, G. K., Odebrecht, C., Poersh, L., and Wasielesky, W. (2013). “Impacto da cianobactéria Nodularia spumigena em cultivo de camarões marinhos em sistema de bioflocos,” in Proceedings of the Reunião Latino Americana Sobre Algas Nocivas. Florianópolis
Guillard, R. R. L. (1975). “Culture of phytoplankton for feeding marine invertebrates,” in Culture of Marine Invertebrate Animals, eds W. L. Smith and M. H. Chanley (New York, NY: Plenum Press).
Hansson, L. A. (1993). Factors initiating algal life-form shift from sediment to water. Oecologia. 94, 286–294. doi: 10.1007/BF00341329
Hansson, L. A. (1996). Algal recruitment from lake sediments in relation to grazing sinking, and dominance patterns in the phytoplankton community. Limnol. Oceanogr. 41, 1312–1323. doi: 10.4319/lo.1996.41.6.1312
Heresztyn, T., and Nicholson, B. C. (1997). Nodularin concentrations in lakes Alexandrina and Albert, South Australia, during a bloom of the cyanobacterium (Blue-Green Alga) Nodularia spumigena and degradation of the toxin. Environ. Toxicol. 12, 273–282. doi: 10.1002/(sici)1098-2256(1997)12:4<273::aid-tox1>3.3.co;2-j
Hobson, P., and Fallowfield, H. (2001). Effect of salinity on photosynthetic activity of Nodularia spumigena. J. Appl. Phycol. 13, 493–499.
Hobson, P., and Fallowfield, H. J. (2003). Effect of irradiance, temperature and salinity on growth and toxin production by Nodularia spumigena. Hydrobiologia 493, 7–15.
Honkanen, R. E., Dukelow, M., Zwiller, J., Moore, R. R., Khatra, B. S., and Boynton, A. L. (1991). Cyanobacterial nodularin is a potent inhibitor of type 1 and type 2A protein phosphatases. Mol. Pharmacol. 40, 577–583.
Huber, A. L. (1985). Factors affecting the germination of akinetes of Nodularia spumigena (Cyanobacteriaceae). Appl. Environ. Microb. 49, 73–78.
Iranshahi, S., Nejadsattari, T., Soltani, N., Shokravi, S., and Dezfulian, M. (2014). The effect of salinity on morphological and molecular characters and physiological responses of Nostoc sp. ISC 101. Iran. J. Fish. Sci. 13, 907–917.
Jones, G. J., Blackburn, S. I., and Parker, N. S. (1994). A toxic bloom of Nodularia spumigena Mertens in Orielton Lagoon, Tasmania. Aust. J. Mar. Fresh. Res. 45, 787–800.
Karjalainen, M. (2005). Fate and Effects of Nodularia Spumigena and its Toxin, Nodularin, in Baltic Sea planktonic food webs. Dissertação de Mestrado. Finland: University of Helsinki.
Karlsson-Elfgren, I., Rengefors, K., and Gustafsson, S. (2004). Factors regulating recruitment from the sediment to the water column in the bloom-forming cyanobacterium Gloeotrichia echinulata. Fresh. Biol. 49, 265–273. doi: 10.1111/j.1365-2427.2004.01182.x
Kim, B. H., Lee, W. S., Kim, Y.-O., Lee, H.-O., and Han, M.-S. (2005). Relationship between akinete germination and vegetative population of Anabaena flos-aquae (Nostocales, Cyanobacteria) in Seokchon reservoir (Seoul, Korea). Arch. Hydrobiol. 163, 49–64. doi: 10.1127/0003-9136/2005/0163-0049
Komárek, J. (2013). “Cyanoprokaryota -3. Teil/Part 3: Heterocytous genera,” in Süßwasserflora von Mitteleuropa Freshwater Flora of Central Europe, eds B. Büdel, G. Gärtner, L. Krienitz, and M. Schagerl (Heidelberg: Springer).
Kononen, K. (1992). Dynamics of the toxic cyanobacterial blooms in the Baltic Sea. Finn. Mar. Res. 261, 3–36.
Ladas, N. P., and Papageorgiou, G. C. (2000). Cell turgor: a critical fator for the proliferation of cyanobacteria at unfavourable salinity. Photosysth. Res. 65, 155–164.
Lehtimäki, J., Sivonen, K., Luukkainen, R., and Niemelä, S. I. (1994). The effect of incubation time, temperature, light, salinity and phosphorus on growth and hepatotoxin production by Nodularia spumigena strains. Arch. Hydrobiol. 130, 269–282.
Li, R., Watanabe, M., and Watanabe, M. M. (1997). Akinete formation in planktonic Anabaena spp. (cyanobacteria) by treatment with low temperature. J. Phycol. 33, 576–584. doi: 10.1111/j.0022-3646.1997.00576.x
Ma, J., Lu, N., Qin, W., Xu, R., Wang, Y., and Chen, X. (2006). Differential responses of eight cyanobacterial and green algal species, to carbamate insecticides. Ecotox. Environ. Safe. 63, 268–274. doi: 10.1016/j.ecoenv.2004.12.002
Mackey, K. R. M., Payton, A., Caldeira, K., Grossman, A. R., Moran Mallvin, D., et al. (2013). Effect of temperature on photosynthesis and growth in marine Synechococcus spp. Plant Physiol. 163, 815–829. doi: 10.1104/pp.113.221937
Mazur, H., and Pliński, M. (2003). Nodularia spumigena blooms and the occurrence of hepatotoxin in the Gulf of Gdánsk. Oceanologia 45, 305–316.
Mazur-Marzec, H., Bertos-Fortis, M., Toruñska-Sitorg, A., Fidor, A., and Legrand, C. (2016). Chemical and genetic diversity of Nodularia spumigena from the Baltic Sea. Mar. Drugs 14, 209. doi: 10.3390/md14110209
Mazur-Marzec, H., Żegliñska, L., and Pliński, M. (2005). The effect of salinity on the growth, toxin production, and morphology of Nodularia spumigena isolated from the Gulf of Gdañsk, southern Baltic Sea. J. Appl. Phycol. 17, 171–179. doi: 10.1007/s10811-005-5767-1
McGregor, G. B., Stewart, I., Sendall, B. C., Sadler, R., Reardon, K., Carter, S., et al. (2012). First report of a toxic Nodularia spumigena (Nostocales/Cyanobacteria) bloom in sub-tropical Australia. I. phycological and public health investigations. Int. J. Environ. Res. Public Health 9, 2396–2411. doi: 10.3390/ijerph9072396
Mohlin, M., and Wulff, A. (2009). Interaction effects of ambient UV radiation and nutrient limitation on the toxic cyanobacterium. Microbial Ecol. 57, 675–686. doi: 10.1007/s00248-008-9427-2
Moisander, P. H., McClinton, E., III., and Paerl, H. W. (2002). Salinity effects on growth, photosynthetic parameters, and nitrogenase activity in estuarine planktonic cyanobacteria. Microb. Ecol. 43, 432–442. doi: 10.1007/soo248-001-1044-2
Myers, J. H., Beardall, J., Allinson, G., Salzman, S., and Gunthorpe, L. (2010). Environmental influences on akinete germination and development in Nodularia spumigena (Cyanobacteriaceae), isolated from the Gippsland Lakes, Victoria, Australia. Hydrobiologia 649, 239–247. doi: 10.1007/s10750-010-0252-5
Myers, J. H., Beardall, J., Allinson, G., Salzman, S., Robertson, S., and Gunthorpe, L. (2011). Potential triggers of akinete differentiation in Nodularia spumigena (Cyanobacteriaceae) isolated from Australia. Hydrobiologia 671, 165–180. doi: 10.1007/s10750-011-0714-4
Ohta, T., Sueoka, E., Iida, N., Komori, A., Suganuma, M., Nishiwaki, R., et al. (1994). Nodularin, a Potent inhibitor of protein phosphstases 1 and 2A, is a new environmental carcinogen in male F344 rat liver. Cancer Res. 54, 6402–6406.
Orr, P. T., and Jones, G. J. (1998). Relationship between microcystin production and cell division rates in nitrogen-limited Microcystis aeruginosa cultures. Limnol. Oceanogr. 43, 1604–1614. doi: 10.4319/lo.1998.43.7.1604
Pääkkönen, J.-P., Rönkkönen, S., Karjalainen, M., and Viitasalo, M. (2008). Physiological effects in juvenile three-spined sticklebacks feeding on toxic cyanobacterium Nodularia spumigena-exposed zooplankton. J. Fish. Biol. 72, 485–499. doi: 10.1111/j.1095-8649.2007.01707.x
Pliński, M., Mazur-Marzec, H., Jóźwiak, T., and Kobos, J. (2007). The potential causes of cyanobacterial bloom in Baltic Sea estuaries. Int. J. Oceanogr. Hydrobiol. 36, 125–137. doi: 10.2478/v10009-007-0001-x
Popin, R. V. (2017). Análise genômica e funcional da Nodularia spumigena CENA596 formadora de florações em tanques de produção de camarões. (Dissertação de Mestrado). Piracicaba: Universidade de São Paulo.
Popin, R. V., Rigonato, J., Abreu, V. A. C., Andreote, A. P. D., Silveira, S. B., Odebrecht, C., et al. (2016). Draft genome assembly of the bloom-forming cyanobacterium Nodularia spumigena strain CENA596 in shrimp production ponds. Genome Announc. 4, e466–e416. doi: 10.1128/genomeA.00466-416
Rakko, A., and Seppälä, J. (2014). Effect of salinity on the growth rate and nutrient stoichiometry of two Baltic Sea filamentous cyanobacterial species. Estonian J. Ecol. 63, 55–70. doi: 10.3176/eco.2014.2.01
Repka, S., Mehtonen, J., Vaitomaa, J., Saari, L., and Sivonen, K. (2001). Effects of nutrients on growth and nodularin production of Nodularia strain GR8b. Microb. Ecol. 42, 606–613. doi: 10.1007/s00248-001-0026-8
Reynolds, C. S. (1984). The Ecology of Freshwater Phytoplankton. Cambridge: Cambridge University Press.
Rinehart, K. L., Harada, K., Namikoshi, M., Chen, C., Harvis, C. A., Munro, M. H. G., et al. (1988). Nodularin, microcystin, and the configuration of adda. J. Am. Chem. Soc. 110, 8557–8558. doi: 10.1021/ja00233a049
Silveira, S. B., Wasielesky, W., Andreote, A. P. D., Fiore, M. F., and Odebrecht, C. (2017). Morphology phylogeny, growth rate and nodularin production of Nodularia spumigena from Brazil. Mar. Biol. Res. 13, 1095–1107. doi: 10.1080/17451000.2017.1336587
Singh, S. C., Sinha, R. P., and Häder, D. P. (2002). Role of lipids and fatty acids in stress tolerance in cyanobacteria. Acta Protozool. 41, 296–308.
Sivonen, K. (1996). Cyanobacteria toxins and toxin production. Phycologia 35, 12–24. doi: 10.2216/i0031-8884-35-6S-12.1
Sukenik, A., Beardall, J., and Hadas, O. (2007). Photosynthetic characterization of developing and mature akinetes of Aphanizomenon ovalisporum (Cyanoprokaryota). J. Phycol. 43, 780–788. doi: 10.1111/j.1529-8817.2007.00374.x
Sun, J., and Liu, D. (2003). Geometric models for calculating cell biovolume and surface area for phytoplankton. J. Plankton Res. 25, 1331–1346. doi: 10.1093/plankt/fbg096
Teikari, J. E., Hou, S., Wahlsten, M., Hess, W. R., and Sivonen, K. (2018). Comparative genomics of the Baltic Sea toxic cyanobacteria Nodularia spumigena UHCC0039 and its response to varying salinity. Front. Microbiol. 9:356. doi: 10.3389/fmicb.2018.00356
Van Buynder, P. G., Oughtred, T., Kirkby, B., Phillips, S., Eaglesham, G., Thomas, K., et al. (2001). Nodularin uptake by seafood during a cyanobacterial bloom. Environ. Toxicol. 16, 468–471. doi: 10.1002/tox.10004
Wood, A. M., Everroad, R. C., and Wingard, L. M. (2005). “Measuring growth rates in microalgal cultures,” in Algal Culturing Techniques, ed. R. Andersen (Cambridge: Academic Press).
Yamamoto, Y. (1975). Effect of desiccation on the germination of akinetes of Anabaena cylindrical. Plant Cell. Physiol. 16, 749–752. doi: 10.1093/oxfordjournals.pcp.a075195
Yunes, J. S., De La Rocha, S., Giroldo, D., Silveira, S. B., Comin, R., Bicho, M. S., et al. (2009). Release of carbohydrates and proteins by a subtropical strain of Raphidiopsis brookii (Cyanobacteria) able to produce saxitoxin at three nitrate concentrations. J. Phycol. 45, 585–591. doi: 10.1111/j.1529-8817.2009.00673.x
Keywords: cyanobacteria, nodularin, harmful, bloom, environmental factors
Citation: Silveira SB and Odebrecht C (2019) Effects of Salinity and Temperature on the Growth, Toxin Production, and Akinete Germination of the Cyanobacterium Nodularia spumigena. Front. Mar. Sci. 6:339. doi: 10.3389/fmars.2019.00339
Received: 21 May 2018; Accepted: 03 June 2019;
Published: 19 June 2019.
Edited by:
Jorge I. Mardones, Instituto de Fomento Pesquero (IFOP), ChileReviewed by:
Jonna Emilia Teikari, Universität Potsdam, GermanyViviana Patricia Almanza, Universidad de Concepción, Chile
Copyright © 2019 Silveira and Odebrecht. This is an open-access article distributed under the terms of the Creative Commons Attribution License (CC BY). The use, distribution or reproduction in other forums is permitted, provided the original author(s) and the copyright owner(s) are credited and that the original publication in this journal is cited, in accordance with accepted academic practice. No use, distribution or reproduction is permitted which does not comply with these terms.
*Correspondence: Savênia B. Silveira, c2Jvbm90b0B5YWhvby5jb20uYnI=