- 1Department of Biology, Marine Biological Research Centre, University of Southern Denmark, Kerteminde, Denmark
- 2Department of Chemistry and Bioscience, Aalborg University, Aalborg, Denmark
This study relates the lipid content of two marine filter-feeding demosponges, Halichondria panicea and H. bowerbanki to the seasonal availability of their suspended food, mainly free-living bacteria and phytoplankton at two study sites in Danish waters. The aim was to investigate if the lipid content of sponges is linked to food availability and season, and to what extend free-living bacteria are available in starvation periods where the phytoplankton biomass is low. The highest concentrations of bacteria were observed during summer when also chlorophyll a concentrations were high, and therefore bacteria and phytoplankton were available in similar ratio at all seasons. Bacterial cell carbon was estimated to contribute 2.9 and 4.6% compared to phytoplankton cell carbon in the food at the two sites, respectively, and free-living bacteria were available only as a minor food source at all seasons. Highest lipid contents (29.5% of sponge dry weight) were seen in H. panicea at the site with lowest food availability, while the lipid content of H. bowerbanki was 11.5% of dry weight. No seasonal variations in lipid content as fraction of sponge dry weight were observed, and the lipid content was not affected by food availability or starvation. It remains unclear why the lipid-content levels at the two study sites were conspicuously different.
Introduction
Halichondria is a common demosponge in for example coastal Dutch (Hummel et al., 1988), German (Barthel, 1986, 1989), Polish (Chojnacki, 2000), and Danish waters (Riisgård et al., 1993; Olesen and Weeks, 1994). Sponges are sessile filter-feeders, evolutionary adapted to nourish on a dilute suspension of microscopic food particles, mainly phytoplankton and free-living bacteria (Riisgård et al., 2016). Food uptake is low in autumn and winter (Koopmans et al., 2015) when low phytoplankton biomass may result in starvation and temporarily decreasing condition index, i.e., the ratio of organic to inorganic matter (Barthel, 1986; Lüskow et al., 2019). The seasonal role of possible energy-storage compounds in sponges is largely unknown. Analysis of the biomass composition of H. panicea from Kiel Bight revealed glycogen and lipid contents of 10–30 mg per g ash-free dry weight and 80–140 mg per g ash-free dry weight, respectively (Barthel, 1986). Neither the lipid, nor the glycogen content seemed, however, to be affected by season (Barthel, 1986). Glycogen is present only in low quantities (<1–3.7% of the dry weight), while lipids may constitute up to 17.3% of the dry weight in demosponges (Stone, 1970; Elvin, 1979). Potentially, lipids may therefore pose the largest energy-storage potential in demosponges, but this has hardly been studied since sponges have no organs or real tissue that may store fat (Rod’kina, 2005).
Sponges contain the greatest diversity of fatty acids among aquatic animals (Rod’kina, 2005), including a variety of long chain (>C22) fatty acids, which are synthesized by elongation of fatty acids from the diet (Carballeira et al., 1986; Rezanka and Sigler, 2009) and used as structural components in cellular membranes (Rod’kina, 2005). Short chained C16 and C18 fatty acids may also be abundant in H. panicea and other sponges (Lawson et al., 1984; Rod’kina et al., 2003, Rod’kina, 2005). Palmitic acid (C16:0) and stearic acid (C18:0) account for 1.5–33.0% of the fatty acids in most sponges (Rod’kina, 2005). These fatty acids can be acquired directly from the diet, but their metabolic roles in sponges have not attracted much attention. Sponges also contain filter-feeding trapped phytoplankton and free-living bacteria (Thomassen and Riisgård, 1995) as well as various symbiotic microorganisms (Lee et al., 2001; Hentschel et al., 2006), which also contribute to the lipids that can be extracted from sponges (Rod’kina, 2005).
Sponges efficiently retain free-living bacteria and other particles down to about 0.1 μm in diameter (Fjerdingstad, 1961; Reiswig, 1971, 1975; Langenbruch, 1983; Leys et al., 2011) and are thus more efficient in retaining such small particles than other co-occurring marine filter-feeding animals, such as, e.g., blue mussels (Lüskow and Riisgård, 2018). The importance of bacteria versus phytoplankton has therefore been debated (see, e.g., Koopmans et al., 2015; Riisgård et al., 2016) and bacteria contribute approximately 20% of the diet of sponges in Danish waters (Lüskow et al., 2019). Since odd numbered long chain fatty acids, stemming from elongation of shorter chain odd numbered fatty acids of bacterial origin can be found in sponges, including H. panicea from coastal Dutch waters, bacteria certainly play a nutritional role (Carballeira et al., 1986; Koopmans et al., 2015).
Coastal seawater contains 106 to 5 × 106 free-living bacteria ml–1 (Azam et al., 1983; Fenchel, 2008). Higher concentrations may also occur (Andersen and Sørensen, 1986) and vary with season (Bernbom et al., 2011; Lüskow et al., 2019; Richert et al., 2019). It is generally observed that the concentration of free-living heterotrophic bacteria living on dissolved organic matter lost from the phytoplankton is positively correlated with the concentration of chlorophyll a in marine environments (Azam et al., 1983; Bird and Kalff, 1984; Guererro et al., 1996; Lüskow et al., 2019; Richert et al., 2019), apparently with the tendency that the bacterial biomass make up a larger proportion of the total suspended biomass when phytoplankton biomass is low (Bird and Kalff, 1984; Pedrós-Alió et al., 1999).
This study relates the lipid content of sponges to the seasonal availability of free-living bacteria and phytoplankton at two study sites in temperate Danish waters; of which one is characterized by relatively low phytoplankton biomass and the presence of the common breadcrumb sponge H. panicea, and the second by relatively high phytoplankton biomass and the presence of a closely related species, H. bowerbanki. The aim was to link the lipid content of sponges to seasonal food availability with particular emphasis on the importance of free-living bacteria in order to achieve a better understanding of their nutritional role for sponges.
Materials and Methods
Collection and Storage of Sponges
Halichondria panicea (Fleming, 1828) was regularly collected in the inlet to Kerteminde Fjord, Denmark (55°26'59.1"N 10°39'41.0"E), while H. bowerbanki (Burton, 1930) was collected in Frederik den VII’s Kanal, an excavated harbor channel connected to Limfjorden, Denmark (56°57'51.1"N 9°14'27.0"E) between October 2016 and September 2017. Five specimens were cleaned of epifauna, weighed and stored separately in plastic bags at −20°C until analysis. Specimens were identified based on spicule morphology as H. panicea in Kerteminde Fjord and as H. bowerbanki in Frederik den VII’s Kanal.
Dry Weight and Lipid Content
The wet weight of sponge specimens was measured after water at their surface had been removed by a napkin. Dry weight was measured after drying for 24 h at 100°C. Ash free dry weight was measured after incubation for 6 h at 500°C. Concentrations of carbon present in sponges were estimated using a conversion factor of 0.142 mg C per μg sponge dry weight (Thomassen and Riisgård, 1995). Lipid contents were quantified in lyophilized subsamples of sponge tissue (approximately 1 g) after 24 h of extraction in chloroform/methanol and subsequent evaporation of the solvent as described by Pleissner and Eriksen (2012). Fatty acids were subsequently transmethylated in 3 ml CHOH:HCl:CHCl (10:1:1 v/v/v) at 90°C for 1 h, and the fatty acid methyl esters were extracted in hexane and analyzed using GC-FID, as also described by Pleissner and Eriksen (2012).
Chlorophyll a and Bacterial Concentrations
In the inlet to Kerteminde Fjord chlorophyll a concentrations were measured using an YSI 650 hand held fluorimeter (Yellowstone Scientific Instruments) at 1 m depth and 1 m away from the harbor wall. In Frederik den VII’s Kanal, phytoplankton was trapped on GF/F glass microfiber filters (Whatman) by vacuum filtration of 1 l of water sampled 20 cm below the surface at calm weather conditions. Chlorophyll a was extracted from the filters for 24 h at 4°C in 80% ethanol and its concentration was measured spectrophotometrically as described by Arvola (1981). Concentrations of carbon present in the phytoplankton were estimated using a conversion factor of 0.04 mg C per μg of chlorophyll a (Li et al., 2010).
The concentration of free-living bacteria was quantified in water sampled at the same time and position as chlorophyll a was measured. Bacterial cells were stained with either DAPI or acridine orange and counted under the microscope using either UV or blue light for excitation. Concentrations of carbon present in the suspended bacterial cells were estimated using a conversion factor of 7.8 × 10–15 g C per bacterial cell (Ferguson and Rublee, 1976).
Weight-Specific Chlorophyll a Content in Sponge Tissue
Sponges sampled at the inlet to Kerteminde Fjord were separated into two groups. One group of sponges was used for immediate quantification of chlorophyll a in the freshly collected sponge tissue. The second group was placed in bio-filtered (by blue mussels) and aerated seawater (the water was changed every 24 h, ambient salinity) and starved for up to 77 h before chlorophyll a was extracted and quantified. The sponges were cut into pieces of 0.33–1.80 g (n = 10) and placed in 8 ml 96% ethanol at 4°C. After 24 h the samples were whirl-mixed for 1 min and the sponge remains withdrawn. The extract was centrifuged for 10 min at 3,000 rpm. Chlorophyll a was calculated as the difference between absorbance measurements at 665 and 750 nm. The wet weight-specific chlorophyll a content in the sponge tissue was calculated by relating the chlorophyll a contents in the extracts to the sponge wet weights.
Results
Seasonality of Food Availability
Concentrations of chlorophyll a were generally lower in the inlet to Kerteminde Fjord (1–6 μg l–1) than in Frederik den VII’s Kanal (2 to 15 μg l–1). Bacterial concentrations were comparable, ranging from a minimum of 2 × 105 cells ml–1 at both sites to 1.3 × 106 cells ml–1 in the inlet to Kerteminde Fjord and 1.5 × 106 cells ml–1 in Frederik den VII’s Kanal (Figures 1A,C). Bacterial concentrations were generally highest when also the chlorophyll a concentration was high (Figure 1B), and bacteria and phytoplankton were primarily available from spring to autumn. On average, the estimated concentrations of cell carbon in phytoplankton and free-living bacteria were 123 ± 44 μg C l–1 in the inlet to Kerteminde Fjord and 322 ± 193 μg C l–1 and in Frederik den VII’s Kanal, with average contributions from bacteria of 4.6 ± 1.8 and 7.7 ± 2.8 μg C l–1, respectively, at the two sites. The ratio between estimated concentrations of carbon present in bacteria and phytoplankton is shown in Figure 1D. The phytoplankton always contributed the majority of the suspended cell carbon. In the inlet to Kerteminde Fjord bacteria contributed on average 4.6% of the cell carbon of phytoplankton. The relationship between bacteria and chlorophyll a concentration was generally higher during the summer period, from spring equinox (Day 80) until autumn equinox (Day 264) than during the winter period (Figure 1D). Bacteria in Frederik den VII’s Kanal accounted for 2.9% of the cell carbon, with the highest relative contribution in February and March (5–7%), when phytoplankton biomass was at a minimum.
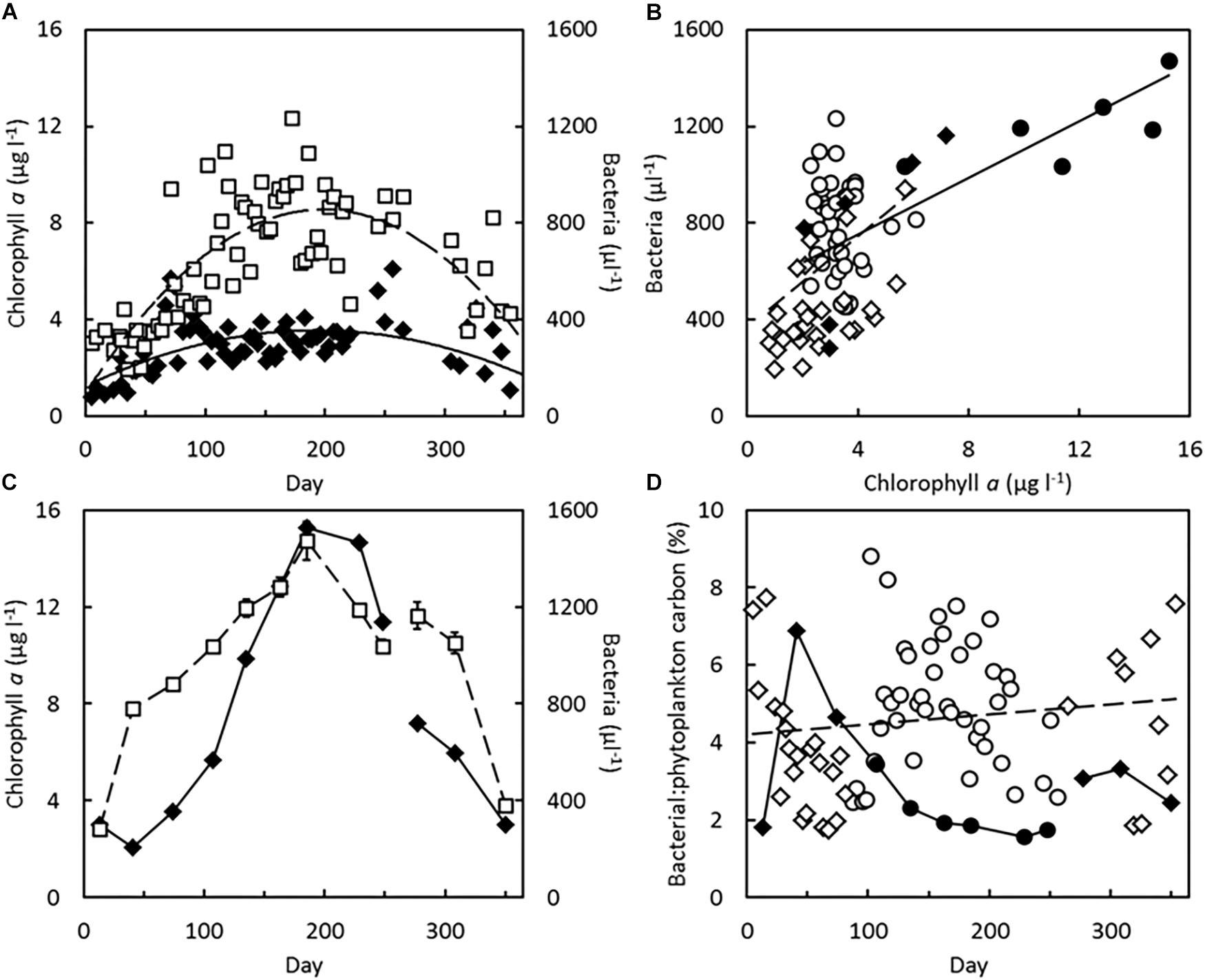
Figure 1. (A,C) Chlorophyll a (◆, —) and bacterial (□, —) concentrations in the water column measured over 1-year periods. (A) Inlet to Kerteminde Fjord, January 28, 2016 to January 30, 2017. Data redrawn from Lüskow et al. (2019), curves drawn by eye. (C) Frederik den VII’s Kanal, October 4, 2016 to September 5, 2017. Error bars indicate SEM of three samples. (B) Relationship between concentrations of bacteria and chlorophyll a in the inlet to Kerteminde Fjord [○ (summer), ◇ (winter), —], r2 = 0.16 and in Frederik den VII’s Kanal [ (summer) ◆, (winter), —], r2 = 0.63. (D) Ratio of concentrations of cellular carbon in phytoplankton and bacteria for 1-year periods in the inlet to Kerteminde Fjord [○(summer), ◇ (winter), —], r2 = 0.02 and in Frederik den VII’s Kanal [
(summer), ◆ (winter), —]. Summer period from spring equinox (Day 80) until autumn equinox (Day 264), winter period the remaining part of the year.
Lipid Content in Sponges
Sponges in the inlet to Kerteminde Fjord had an average dry weight content of 8.7% of the wet weight, unaffected by season while sponges in Frederik den VII’s Kanal had dry weight content of approximately 10% of the wet weight in October to February. From March to September, the average dry weight content of these sponges increased and peaked at around 20% of the wet weight. In October to November 2016, sponges in Frederik den VII’s Kanal had a condition index of 1.16 ± 0.17 (n = 6), which in January to February 2017 decreased to 0.53 ± 0.07 (n = 6). In April to May 2017, the condition index was 0.58 ± 0.09 (n = 6). The dry-weight specific lipid content in sponges from both sampling sites remained constant over the year and was considerably higher in sponges in the inlet to Kerteminde Fjord than in sponges in Frederik den VII’s Kanal (Figure 2).
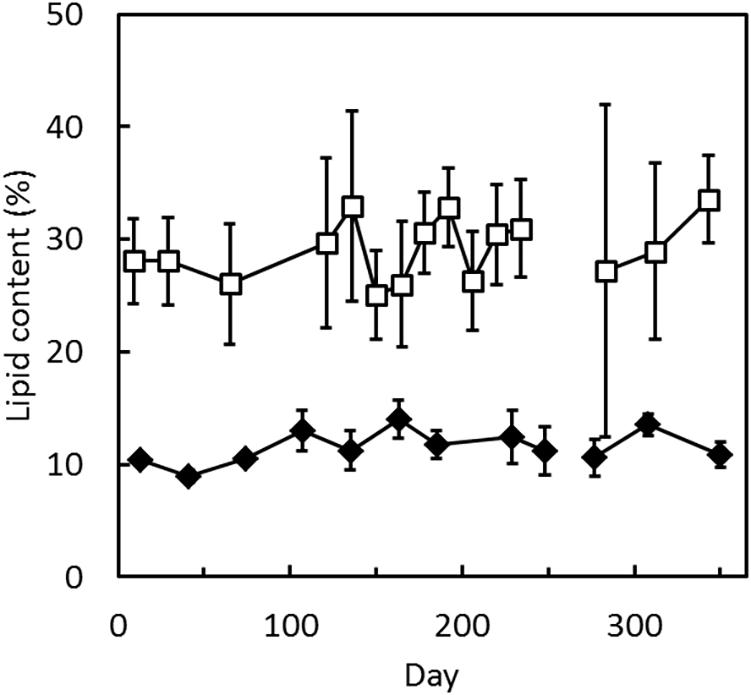
Figure 2. Halichondria panicea and H. bowerbanki. Lipid content as fraction of dry weight (□) in H. panicea from the inlet to Kerteminde Fjord, October 19, 2016 to September 9, 2017 and in H. bowerbanki from Frederik den VII’s Kanal, October 4, 2016 to September 5, 2017 (◆). Error bars indicate SEM from 3 to 5 specimens.
Composition of Short-Chain Fatty Acids in Sponge Lipids
Palmitic and stearic acid were the most abundant short-chain fatty acids in sponges from both sampling sites (Figure 3), as judged from gas chromatograms. The contents of these two fatty acids were variable, but in most specimens, each fatty acid made up less than 10% of the total lipid content in the sponges. They did, however, always co-occur in a C16:C18 ratio of 1.2–1.3 (Figure 3A). Other fatty acids were also present in the sponges along with a number of unidentified lipophilic compounds, each contributing less than 1% of the total lipid content.
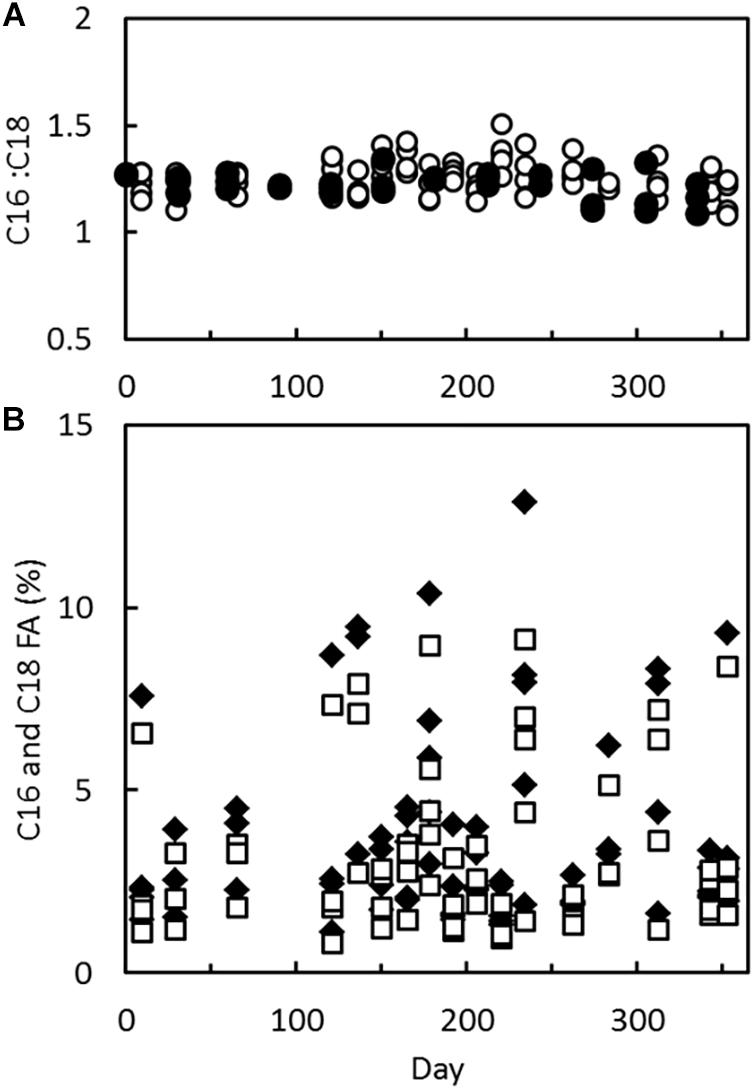
Figure 3. Halichondria panicea and H. bowerbanki. (A) Ratio of palmitic acid and stearic acid, C16:C18 in H. panicea from the inlet to Kerteminde Fjord, October 10, 2016 to September 9, 2017 () and in H. bowerbanki from Frederik den VII’s Kanal, October 4, 2016 to September 5, 2017 (○). (B) Fraction of palmitic acid, C16:0 (◆) and stearic acid, C18 (□) in lipids of H. panicea from the inlet to Kerteminde Fjord, October 4, 2016 to September 9, 2017.
Specific Chlorophyll a Content in Sponges
The decrease of chlorophyll a in sponges sampled in the inlet to Kerteminde Fjord was followed after they were transferred to seawater cleared from phytoplankton (Figure 4). Chlorophyll a was cleared from the sponge tissue at a specific rate of 0.017 h–1, corresponding to a half-life of approximately 40 h. Initially, the sponges contained 17 μg chlorophyll a per g wet weight, corresponding to a phytoplankton carbon contribution of approximately 0.5% of the organic carbon.
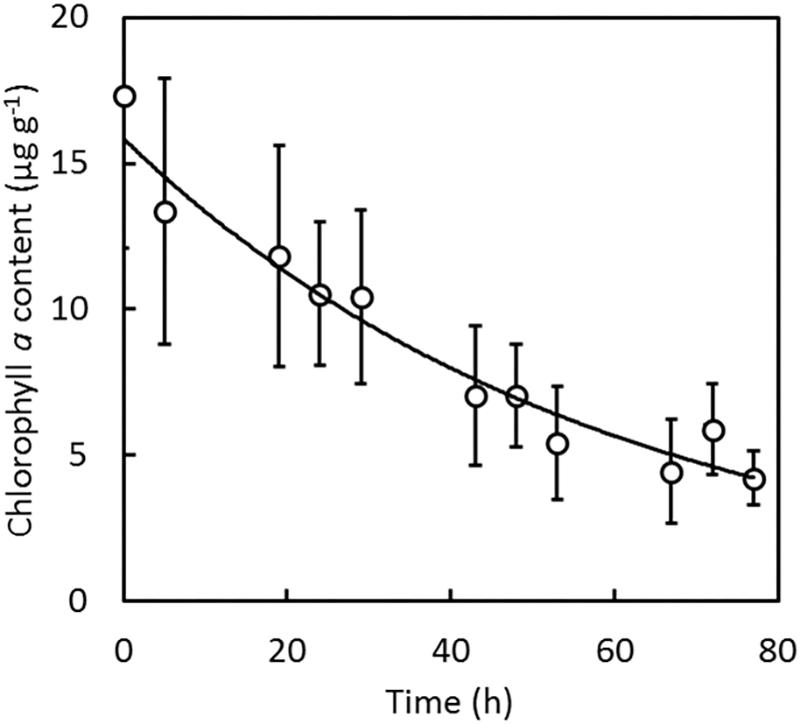
Figure 4. Halichondria panicea. Wet-weight specific chlorophyll a content in sponge tissue after transfer from the field to bio-filtered seawater without phytoplankton. Data points show average values ± SD of 10 individual specimens. Regression curve is a first order decay with a rate constant of 0.017 h–1, r2 = 0.94.
Discussion
The concentrations of chlorophyll a and free-living bacteria varied over the season at both study sites. Generally, chlorophyll a concentrations were lower in the inlet to Kerteminde Fjord (3 μg l–1 on average) than in Frederik den VII’s Kanal (8 μg l–1), while concentrations of bacteria stayed within similar concentration ranges at both sites (Figures 1A,C). Although food availability was highest in Frederik den VII’s Kanal, the specific lipid content was highest in Halichondria panicea from the inlet to Kerteminde Fjord. The specific lipid content did not show signs of seasonal variation. Bacteria made up a minor fraction of the available biomass all year round and have thus played a minor role as food for the sponges, in agreement with Lüskow et al. (2019).
In Frederik den VII’s Kanal, there was a correlation between concentration of bacteria and biomass of phytoplankton (measured as concentration of chlorophyll a) over the 1-year period (r2 = 0.63, Figure 1B). In February and March (Days 41 and 74), bacteria contributed more to the suspended biomass than in the rest of the year. In the inlet to Kerteminde Fjord, chlorophyll a concentrations remained within a narrower concentration range and the correlation between concentrations of bacteria and chlorophyll a was weak (r2 = 0.16, Figure 1B). At this site, the ratio between bacteria and chlorophyll a was generally higher in summer, when also the primary production and the loss of dissolved organic matter are high (Azam et al., 1983), than in winter (Figure 1D). In 1983, bacterial concentrations were followed from March to November at an open-coast location in the eutrophicated Limfjorden (Andersen and Sørensen, 1986). In early spring, bacterial concentrations were below 106 cells ml–1, while maximal summer concentrations were up to 15 × 106 cells ml–1. In comparison, the availability of free-living bacteria was therefore relatively low at both present study sites. The estimated bacterial carbon contributions (Figure 1D) were based on a conversion factor of 7.8 × 10–15 g C per bacterial cell (Ferguson and Rublee, 1976). Other studies have indicated cell-specific carbon contents of marine bacteria in Danish waters between 7 and 43 × 10–15 g C cell–1 (Fukuda et al., 1998). Thus, bacteria may have contributed between 4.1 and 25.2% of the carbon present in phytoplankton in the inlet to Kerteminde Fjord and 2.6 to 13.8% in Frederik den VII’s Kanal.
The lipid content was high in H. panicea from the inlet to Kerteminde Fjord (29.5%) compared to earlier reports, while the lipid content in H. bowerbanki from Frederik den VII’s Kanal (11.5%) was comparable to previously published values (Stone, 1970; Elvin, 1979; Barthel, 1986). No seasonality was observed in the lipid content (Figure 2). It seems to be a general trend that the lipid content of Halichondria is independent of season (Barthel, 1986), although negative growth and decreasing condition index indicate that the sponges are starving during autumn and winter (Barthel, 1986; Lüskow et al., 2019). Seasonality was also not observed in the contents of palmitic acid or stearic acid (Figure 3), indicating that the role of these fatty acids is not directly associated with energy metabolism. The high condition index of H. bowerbanki from Frederik den VII’s Kanal measured in October to November 2016 (1.16 ± 0.17) and the low condition index measured in January to February 2017 (0.53 ± 0.07) were similar to measurements of condition indices of H. panicea in the inlet to Kerteminde Fjord (Lüskow et al., 2019) and lipids have therefore contributed more of the organic fraction of the sponges in winter when the condition index was low. It is not clear why changes in condition index were not reflected in the lipid content as fraction of dry weight. Also in the blue mussel Mytilus edulis, which is common in the same habitats, there seems to be no clear relationship between lipid content and nutritional status, but in contrast to sponges, blue mussels contain a large pool of glycogen that can be mobilized during starvation periods (Pleissner et al., 2012).
Lipids from bacteria may to a minor extent have contributed to the lipids extracted from the sponges. Halichondria belongs to the so-called low microbial abundance (LMA) sponges (Knobloch et al., 2019), which according to Hentschel et al. (2006) contain between 105 and 106 bacterial cells per g wet weight. With an average dry weight of marine bacteria of 2.3 × 10–14 g cell–1 (Ferguson and Rublee, 1976) and average dry weight contents of 8.7–13.5% in sponges from the inlet to Kerteminde Fjord and Frederik den VII’s Kanal, respectively, the bacterial biomass made up a maximum of only 3 × 10–5 to 4 × 10–5% of the sponge dry weight. Furthermore, Gillan et al. (1988) found that fatty acids from bacteria made up only 56–437 μg g–1 (0.005–0.0437%) of the total fatty acids in five tropical sponge species. The contribution of lipids from trapped phytoplankton was also modest, although the sponges did contain measurable quantities of chlorophyll a (Figure 4). Chlorophyll a decayed in the sponges at a rate of 0.017 h–1 after transfer to phytoplankton-free seawater. This has also been observed in H. panicea by Thomassen and Riisgård (1995), from whose data it can be estimated that the rate of decay was 0.035 h–1 for sponges transferred directly from the field, and 0.031 h–1 for sponges fed algal cells in the laboratory prior to transfer to clean seawater. These observations indicate that chlorophyll a in Halichondria primarily stem from captured, but not yet digested phytoplankton. Using a conversion factor of 0.04 mg C per μg of chlorophyll a (Li et al., 2010), it can be estimated that phytoplankton contributed less than 0.5% to the organic biomass of H. panicea. It is also noticeably that palmitic acid and stearic acid were always present in a constant ratio of 1.26 ± 0.08 in H. panicea from the inlet to Kerteminde Fjord and 1.22 ± 0.06 in H. bowerbanki from Frederik den VII’s Kanal (Figure 3). This indicates that the composition of these lipids is under stricter control than would be the case if lipids were derived from a variety of trapped or symbiotic microorganisms.
Conclusion
Halichondria panicea and H. bowerbanki efficiently retain phytoplankton and bacteria, and since the abundance of bacteria follows the phytoplankton biomass and always make up the smallest fraction of the two food sources, consumption of free-living bacteria cannot explain why the lipid content of sponges remains stable, even when decreasing condition index and low chlorophyll a concentrations indicate starvation. Halichondria does not rely on accumulated energy reserves during periods of starvation but recycle their own cells during winter, apparently leaving the specific lipid content as a fraction of dry weight constant at all seasons. It remains unclear why the pronounced variation in condition index was not reflected in the lipid content, and why the lipid-content levels at the two study sites were conspicuously different.
Data Availability
The datasets generated for this study are available on request to the corresponding author.
Ethics Statement
The study was conducted on marine sponges (invertebrates) and therefore exempted from this requirement.
Author Contributions
FL carried out sampling and experimental work in the inlet to Kerteminde Fjord and wrote the first draft of the manuscript in collaboration with NE. KK-M quantified the lipids and carried out fatty acid analysis in collaboration with LP. JT carried out sampling and experimental work in Frederik den VII’s Kanal. LP carried out fatty acid analysis in collaboration with KK-M. HR conceived and designed the experiments in collaboration with FL and NE. NE conceived and designed the experiments in collaboration with FL and HR and wrote the first draft of the manuscript in collaboration with FL.
Funding
This work was supported by a research grant (9278) from VILLUM FONDEN (HR and NE).
Conflict of Interest Statement
The authors declare that the research was conducted in the absence of any commercial or financial relationships that could be construed as a potential conflict of interest.
Acknowledgments
We thank Ole S. Tendal, Zoological Museum, University of Copenhagen, for identification of sponges to species level. We also thank the reviewers for their constructive comments.
References
Andersen, P., and Sørensen, H. M. (1986). Population dynamics and trophic coupling in pelagic microorganisms in eutrophic coastal waters. Mar. Ecol. Prog. Ser. 33, 99–109. doi: 10.3354/meps033099
Arvola, L. (1981). Spectrophotometric determination of chlorophyll a and phaeopigments in ethanol extractions. Ann. Bot. Fenn. 18, 221–227.
Azam, F., Fenche1, T., Field, J. J. G., Gray, J. S., Meyer-Reil, L. A., and Thingstad, F. (1983). The ecological role of water-column microbes in the sea. Mar. Ecol. Prog. Ser. 10, 257–263. doi: 10.3354/meps010257
Barthel, D. (1986). On the ecophysiology of the sponge Halichondria panicea in kiel bight. I. substrate specificity, growth and reproduction. Mar. Ecol. Prog. Ser. 32, 291–298. doi: 10.3354/meps032291
Barthel, D. (1989). “Growth of the sponge Halichondria panicea in the North Sea habitat,” in Proceedings of the 21st EMBS, Gdañsk. doi: 10.3354/meps032291
Bernbom, N., Ng, Y. Y., Kjelleberg, S., Harder, T., and Gram, L. (2011). Marine bacteria from danish coastal waters show antifouling activity against the marine fouling bacterium Pseudoalteromonas sp. strain S91 and zoospores of the green alga Ulva australis independent of bacteriocidal activity. Appl. Environ. Microbiol. 77, 8557–8567. doi: 10.1128/AEM.06038-11
Bird, D. F., and Kalff, J. (1984). Empirical relationships between bacterial abundance and chlorophyll concentration in fresh and marine waters. Can. J. Fish. Aquat. Sci. 41, 1015–1023. doi: 10.1139/f84-118
Carballeira, N., Thompson, J. E., Ayanoglu, W., and Djerassi, C. (1986). Biosynthetic studies of marine lipids. 5. the biosynthesis of long-chain branched fatty acids in marine sponges. J. Org. Chem. 51, 2751–2756. doi: 10.1021/jo00364a024
Chojnacki, J. C. (2000). “Environmental effects of artificial reefs in the Southern Baltic (Pomeranian Bay),” in Artificial Reefs in European Seas, eds A. C. Jensen, K. J. Collins, and A. P. M. Lockwood (Dordrecht: Springer), 307–317. doi: 10.1007/978-94-011-4215-1-18
Elvin, D. W. (1979). The relationship of seasonal changes in the biochemical components to the reproductive behavior of the intertidal sponge Haliclona permollis. Biol. Bull. 156, 47–61. doi: 10.2307/1541002
Fenchel, T. (2008). The microbial loop - 25 years after. J. Exp. Mar. Biol. Ecol. 366, 99–103. doi: 10.1016/j.jembe.2008.07.013
Ferguson, R. L., and Rublee, P. (1976). Contribution of bacteria to standing crop of coastal plankton. Limnol. Oceanogr. 21, 141–145. doi: 10.4319/lo.1976.21.1.0141
Fjerdingstad, E. J. (1961). The ultrastructure of choanocyte collars in Spongilla lacustris (L.). Z. Zellforsch. Mikrosk. Anat. 53, 645–657. doi: 10.1007/BF00339512
Fukuda, R., Ogawa, H., Nagata, T., and Koike, K. (1998). Direct determination of carbon and nitrogen contents of natural bacterial assemblages in marine environments. Appl. Environ. Microbiol. 64, 3352–3358.
Gillan, F. T., Stoilov, I. L., Thompson, J. E., Hogg, R. W., Wilkinson, C. R., and Djerassi, C. (1988). Fatty acids as biological markers for bacterial symbionts in sponges. Lipids 23, 1139–1145. doi: 10.1007/BF02535280
Guererro, F., Jímenez-Gómez, F., Blanco, J. M., and Rodréguez, V. (1996). Summer relationships between bacterioplankton and chlorophyll-a concentration in a eutrophic coastal system of Alboran Sea (SE of Spain). Cah. Biol. Mar. 37, 221–225.
Hentschel, U., Usher, K. M., and Taylor, M. W. (2006). Marine sponges as microbial fermenters. FEMS Microbiol. Ecol. 55, 167–177. doi: 10.1111/j.1574-6941.2005.00046.x
Hummel, H., Sepers, A. B. J., de Wolf, L., and Melissen, F. W. (1988). Bacterial growth on the marine sponge Halichondria panicea induced by recued waterflow rate. Mar. Ecol. Prog. Ser. 42, 195–198. doi: 10.3354/meps042195
Knobloch, S., Jóhannsson, R., and Marteinsson, V. (2019). Bacterial diversity in the marine sponge Halichondria panicea from Icelandic waters and host-specificity of its dominant symbiont “Candidatus Halichondribacter symbioticus”. FEMS Microbiol. Ecol. 95:fiy220. doi: 10.1093/femsec/fiy220
Koopmans, M., van Rijswijk, P., Boschker, H. T. S., Marco, H., Martens, D., and Wijffels, R. H. (2015). Seasonal variation of fatty acids and stable carbon isotopes in sponges as indicators for nutrition: biomarkers in sponges identified. Mar. Biotechnol. 17, 43–54. doi: 10.1007/s10126-014-9594-8
Langenbruch, P. F. (1983). Untersuchungen zum Körperbau von Meeresschwämmen. II. Das Wasserleitungssystem von Halichondria panicea. Helgol. Meeresunters. 36, 337–346. doi: 10.1007/bf01983636
Lawson, M. P., Bergquist, P. R., and Cambie, R. C. (1984). Fatty acid composition and classification of the porifera. Biochem. Syst. Ecol. 12, 63–84. doi: 10.1016/0305-1978(84)90012-90017
Lee, Y. K., Lee, J.-H., and Lee, H. K. (2001). Microbial symbiosis in marine sponges. J. Microbiol. 39, 254–264.
Leys, S. P., Yahel, G., Reidenbach, M. A., Tunnicliffe, V., Shavit, U., and Reiswig, H. M. (2011). The sponge pump: the role of current induced flow in the design of the sponge body plan. PLoS One 6:e27787. doi: 10.1371/journal.pone.0027787
Li, Q. P., Franks, P. J. S., Landry, M. R., and Goericke, R. (2010). Modelling phytoplankton growth rates and chlorophyll to carbon ratios in california coastal and pelagic ecosystems. J. Geophys. Res. 115:G04003. doi: 10.1029/2009JG001111
Lüskow, F., and Riisgård, H. U. (2018). In situ filtration rates of blue mussels (Mytilus edulis) measured by an open-top chamber method. Open J. Mar. Sci. 8, 395–406. doi: 10.4236/ojms.2018.84022
Lüskow, F., Riisgård, H. U., Solovyeva, V., and Brewer, J. R. (2019). Seasonal changes in bacteria and phytoplankton biomass control the condition index of the demosponge Halichondria panicea in temperate Danish waters. Mar. Ecol. Prog. Ser. 608, 119–132. doi: 10.3354/meps12785
Olesen, T. M. E., and Weeks, J. M. (1994). Accumulation of Cd by the marine sponge Halichondria panicea pallas: effects upon filtration rate and its relevance for biomonitoring. Bull. Environ. Contam. Toxicol. 52, 722–728. doi: 10.1007/BF00195494
Pedrós-Alió, C., Calderón-Paz, J.-I., Guixa-Boixereu, N., Estrada, M., and Gasol, J. M. (1999). Bacterioplankton and phytoplankton biomass and production during summer stratification in the northwestern mediterranean Sea. Deep Sea Res. 46, 985–1019. doi: 10.1016/S0967-0637(98)00106-X
Pleissner, D., and Eriksen, N. T. (2012). Effects of phosphorous, nitrogen, and carbon limitation on biomass composition in batch and continuous flow cultures of the heterotrophic dinoflagellate Crypthecodinium cohnii. Biotechnol. Bioeng. 109, 2005–2016. doi: 10.1002/bit.24470
Pleissner, D., Eriksen, N. T., Lundgreen, K., and Riisgard, H. U. (2012). Biomass composition of blue mussels, Mytilus edulis, is affected by living site and species of ingested microalgae. ISRN Zool. 2012:12. doi: 10.5402/2012/902152
Reiswig, H. M. (1971). Particle feeding in natural populations of three marine demosponges. Biol. Bull. 141, 568–591. doi: 10.2307/1540270
Reiswig, H. M. (1975). Bacteria as food for temperate-water marine sponges. Can. J. Zool. 53, 582–589. doi: 10.1139/z75-072
Rezanka, T., and Sigler, K. (2009). Odd-numbered very-long-chain fatty acids from the microbial, animal and plant kingdoms. Prog. Lipid Res. 48, 206–238. doi: 10.1016/j.plipres.2009.03.003
Richert, I., Yager, P. L., Dinasquet, J., Logares, R., Riemann, L., Wendeberg, A., et al. (2019). Summer comes to the Southern Ocean: how phytoplankton shape bacterioplankton communities far into the deep dark sea. Ecosphere 10:e02641. doi: 10.1002/ecs2.2641
Riisgård, H. U., Kumala, L., and Charitonidou, K. (2016). Using the F/R-ratio for an evaluation of the ability of the demosponge Halichondria panicea to nourish solely on phytoplankton versus free-living bacteria in the sea. Mar. Biol. Res. 12, 907–916. doi: 10.1080/17451000.2016.1206941
Riisgård, H. U., Thomassen, S., Jakobsen, H., Weeks, J. M., and Larsen, P. S. (1993). Suspension feeding in marine sponges Halichondria panicea and Haliclona urceolus: effects of temperature on filtration rate and energy cost of pumping. Mar. Ecol. Prog. Ser. 96, 177–188. doi: 10.3354/meps096177
Rod’kina, S. A. (2005). Fatty acids and other lipids of marine sponges. Russ. J. Mar. Biol. 31, S49–S60. doi: 10.1007/s11179-006-0015-3
Rod’kina, S. A., Latyshev, N. A., and Imbs, A. B. (2003). Fatty acids from the Sea of Japan sponge Halichondria panicea. Russ. J. Bioorganic Chem. 29, 382–386. doi: 10.1023/A:1024957403078
Stone, A. R. (1970). Seasonal variation in the gross biochemical composition of Hymeniacidon perleve (Montagu). J. Exp. Mar. Biol. Ecol. 5, 265–271. doi: 10.1016/0022-0981(70)90005-5
Keywords: free-living bacteria, phytoplankton, fatty acids, filter-feeding, seasonality, lipids, Porifera
Citation: Lüskow F, Kløve-Mogensen K, Tophøj J, Pedersen LH, Riisgård HU and Eriksen NT (2019) Seasonality in Lipid Content of the Demosponges Halichondria panicea and H. bowerbanki at Two Study Sites in Temperate Danish Waters. Front. Mar. Sci. 6:328. doi: 10.3389/fmars.2019.00328
Received: 26 March 2019; Accepted: 28 May 2019;
Published: 12 June 2019.
Edited by:
Oscar Monroig, Spanish National Research Council (CSIC), SpainReviewed by:
Gilles Barnathan, L’Université Nantes Angers Le Mans (L’UNAM), FranceLorenzo Gallus, University of Genoa, Italy
Copyright © 2019 Lüskow, Kløve-Mogensen, Tophøj, Pedersen, Riisgård and Eriksen. This is an open-access article distributed under the terms of the Creative Commons Attribution License (CC BY). The use, distribution or reproduction in other forums is permitted, provided the original author(s) and the copyright owner(s) are credited and that the original publication in this journal is cited, in accordance with accepted academic practice. No use, distribution or reproduction is permitted which does not comply with these terms.
*Correspondence: Niels Thomas Eriksen, nte@bio.aau.dk
†Present address: Florian Lüskow, Department of Earth, Ocean and Atmospheric Sciences, University of British Columbia, Vancouver, BC, Canada