- 1Red Sea Research Center (RSRC) and Computational Bioscience Research Center (CBRC), King Abdullah University of Science and Technology (KAUST), Thuwal, Saudi Arabia
- 2Instituto Andaluz de Ciencias de la Tierra, CSIC-UGR, Armilla, Spain
- 3Department of Biology, College of Science, Imam Abdulrahman Bin Faisal University (IAU), Dammam, Saudi Arabia
- 4Global Change Research Group, Institut Mediterrani d’Estudis Avançats (IMEDEA, CSIC-UIB), Esporles, Spain
- 5Department of Bioscience, Aarhus University, Aarhus, Denmark
Coastal vegetated ecosystems are intense global carbon (C) sinks; however, seagrasses and mangroves in the Central Red Sea are depleted in organic C (Corg). Here, we tested whether Corg depletion prevails along the Red Sea, or if sediment Corg and nitrogen (N) stocks reflect the latitudinal productivity gradient of the Red Sea. We assessed Corg and N concentrations, stocks, isotopic compositions (δ13C and δ15N), and the potential contribution of primary producers to the organic matter accumulation in seagrass and mangrove sediments along the Eastern coast of the Red Sea. Sediment Corg concentration was higher in mangroves than seagrasses, while N concentrations were similar, resulting in higher C/N ratios in mangrove than seagrass sediments. Mangrove Corg stocks (integrated over the top 10 cm) were twofold higher than those of seagrasses. N concentrations and stocks decreased from south to north in seagrass sediments matching the productivity gradient while Corg concentrations and stocks were uniform. The δ15N decreased from south to north in seagrass and mangrove sediments, reflecting a shift from nitrate and nitrite as N sources in the south, to N2 fixation toward the north. Stable isotope mixing models showed that seagrass leaves and macroalgae blades were the major contributors to the organic matter accumulation in seagrass sediments; while mangrove leaves were the major contributors in mangrove sediments. Overall, vegetated sediments in the Red Sea tend to be carbonate-rich and depleted in Corg and N, compared to coastal habitats elsewhere. Specifically, mean Corg stocks in Red Sea seagrass and mangrove sediments (7.2 ± 0.4 and 14.5 ± 1.4 Mg C ha-1, respectively) are lower than previously reported mean global values. This new information of Blue Carbon resources in the Red Sea provides a background for Blue Carbon programs in the region while also helping to balance global estimates.
Introduction
Coastal vegetated ecosystems, such as seagrasses, mangroves, and saltmarshes, also known as Blue Carbon ecosystems (Nellemann et al., 2009; Duarte et al., 2013), are intense carbon sink habitats. Due to their high rates of primary production and their capacity to trap allochthonous particles, these ecosystems can bury organic and inorganic carbon in their sediments for millennia (McLeod et al., 2011; Duarte, 2017). Therefore, Blue Carbon ecosystems play an important role in climate change mitigation by removing atmospheric CO2 (Duarte et al., 2013). Moreover, these ecosystems protect the coastline from sea level rise by their sediment accretion capacity. Globally, the organic C (Corg) burial capacity of Blue Carbon ecosystems ranges from 114 to 131 Tg C yr-1, of which seagrasses, mangroves, and saltmarshes are responsible for 27.4 to 44, 17 to 23.6, and 60.4 to 70 Tg C yr-1, respectively (Nellemann et al., 2009). In seagrass ecosystems, the habitat-forming seagrass is responsible for about half of the total buried Corg on average (Kennedy et al., 2010), based on the analysis of stable C isotopic data extracted from more than 200 seagrass sites. Specifically, Kennedy et al. (2010) estimated that global Corg burial in seagrass ecosystems could account for 48 to 112 Tg yr-1, combining global average net community production and global average for allochthonous Corg trapped in sediments.
Globally, sediment Corg stocks have been estimated at 2.6 Pg C for mangroves (Atwood et al., 2017) and between 4.2 and 8.9 Pg C for seagrasses (Fourqurean et al., 2012). However, these global estimates are based on available published data and are therefore skewed toward those areas heavily represented in literature (Lavery et al., 2013), with minor representation of coastal vegetated habitats in arid regions. Thus, additional studies covering poorly represented areas in global estimates are needed to better ascertain global sediment Corg stocks in Blue Carbon ecosystems.
Despite the scarcity of data on Blue Carbon ecosystems from arid regions, recent studies have reported that sediments of seagrass and mangrove ecosystems in the Central Red Sea and the Arabian Gulf are depleted in Corg (Almahasheer et al., 2017; Cusack et al., 2018; Serrano et al., 2018), but contain high carbonate loads (Saderne et al., 2018). These observations are consistent with the lack of riverine inputs and the oligotrophic nature of the Red Sea, that lead to stunted mangroves (Almahasheer et al., 2016b) and iron-depleted leaves of mangroves and seagrasses (Almahasheer et al., 2016a; Anton et al., 2018), and its warm and salty nature, that favors carbonate deposition. However, these previous assessments of sediment Corg stocks in Red Sea seagrasses and mangroves are limited to the Central Red Sea (Almahasheer et al., 2017; Serrano et al., 2018), and no information exists on the associated nitrogen (N) stocks. The Red Sea is characterized by a south to north gradient in oligotrophy, salinity, and temperature (Chaidez et al., 2017), which affects productivity regimes (Raitsos et al., 2013) and may also affect Corg and N stocks in sediments. The variability of Corg and N stocks in seagrass and mangrove habitats in Red Sea sediments has not yet been evaluated, so whether the low Corg stocks found in the Central Red Sea (Almahasheer et al., 2017; Serrano et al., 2018) prevail across the entire Red Sea is still unknown.
Here, we test the following hypotheses: (i) that Corg and N stocks of Red Sea seagrass and mangrove sediments are lower than those in other regions; (ii) that the south to north productivity gradient in the Red Sea is reflected in a south to north gradient in sediment Corg and N concentrations and stocks; and (iii) that the habitat-forming primary producers are the main sources of the accumulated organic matter in Red Sea seagrass and mangrove sediments. We do so by estimating the C (organic and inorganic) and N concentrations, stocks, and isotopic compositions in seagrass and mangrove sediments sampled over a broad latitudinal gradient along the Red Sea. We estimate the potential contribution of primary producers to the accumulated organic matter in seagrass and mangrove sediments by applying stable isotope mixing models.
Materials and Methods
Study Site
We sampled 43 stations along the Saudi coast of the Red Sea, including 27 seagrass meadows and 16 mangrove stands (Figure 1) on four coastal cruises onboard the R/V Thuwal on February 2016, January 2017, March 2017, and July 2017 (for further details on cruises and survey methods see Garcias-Bonet and Duarte, 2017; Anton et al., 2018; Duarte et al., 2018; Sea et al., 2018). In addition, stations located in the Central Red Sea were sampled using outboard motor boats on November 2014, February 2016, January 2017, March 2017, and July 2017. The sampled seagrass and mangrove stations encompass a latitudinal range of 10 and 8.9°, respectively. At each station, we collected triplicate sediment samples using cylindrical plastic cores (inner diameter = 9.5 cm, length = 30 cm) containing at least 10 cm of sediment. We standardized the sediment sampling to the top 10 cm because most of the sampled seagrass meadows held thin sediment layers. We collected sediment samples from each seagrass species present at each station. The samples were transported back to the laboratory and processed immediately after collection.
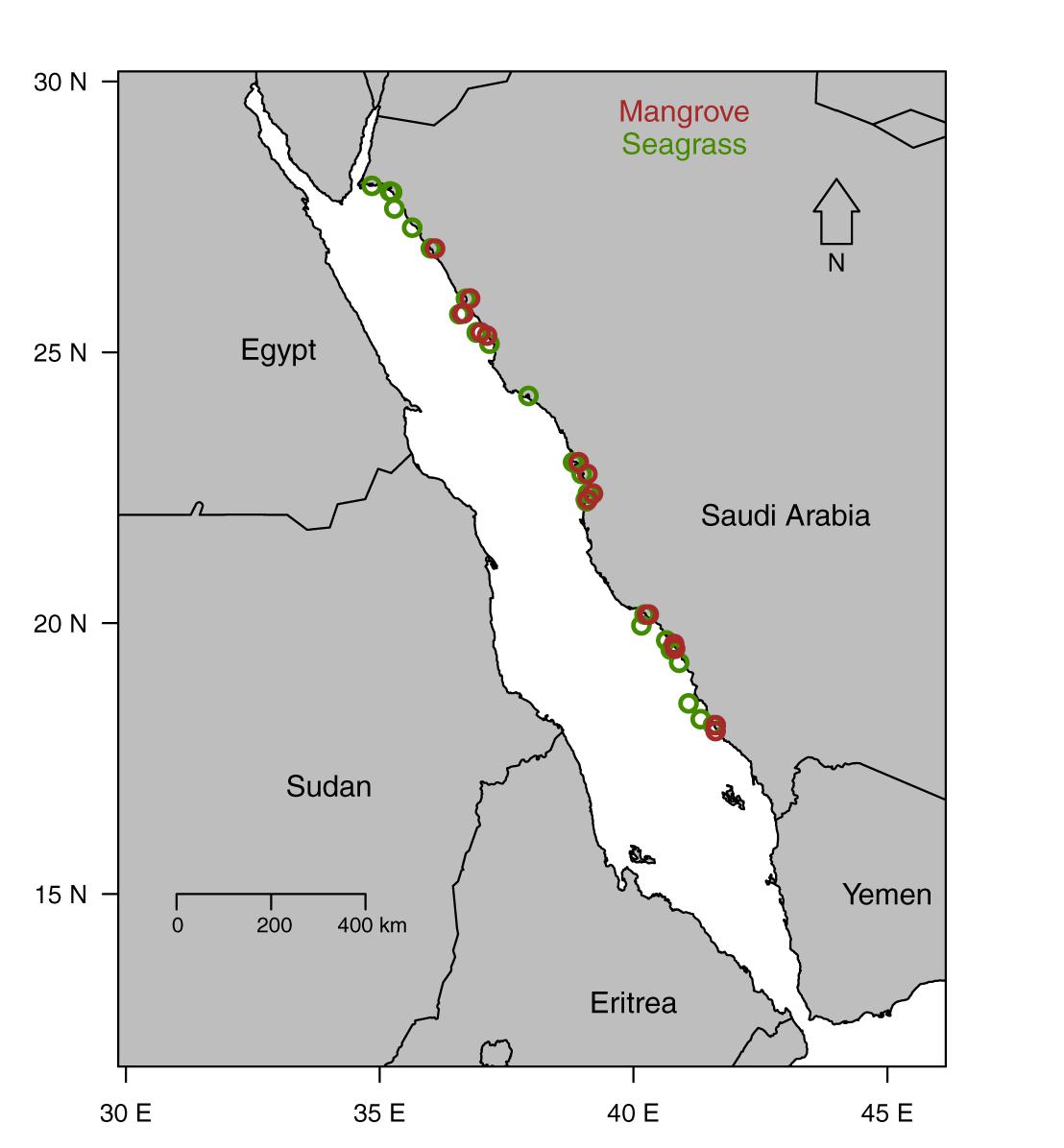
Figure 1. Map showing the location of seagrass (green circles) and mangrove (brown circles) study sites.
Sediment samples were carefully extruded from the cylindrical cores, and the sediment was cut in three layers: from the surface to 1, 1–5, and 5–10 cm below the sediment surface. Seagrass and mangrove tissues were removed from sediment samples after which sediment samples were dried at 60°C (Ryba and Burgess, 2002) until constant weight.
Seagrass and Mangrove Sediment Analysis
The sediment bulk density was calculated as the mass of dry solids in a given volume of sediment (expressed in g DW sediment cm-3). Dried sediments were ground using mortar and pestle, and a sediment subsample (1 g DW sediment) was used to estimate the sediment carbonate and inorganic carbon (Cinorg) concentrations using a calcimeter as described by Müller and Gastner (1971). Sediment bulk density, carbonate concentration, and Cinorg concentration were analyzed on triplicate for each sediment horizon at each station. The remaining sediment was acidified in order to remove carbonates (Hedges and Stern, 1984) before analyzing the organic carbon (Corg) and nitrogen (N) concentrations and their stable isotopic compositions (δ13C and δ15N). Specifically, we added chloride (1M HCl) to each sediment sample until no bubble formation was detectable, then the sediment samples were rinsed, centrifuged and re-dried overnight. The use of a weak HCl solution (up to 2M) has been shown to be free of biases in analysis of δ13C and δ15N in sediments (Kennedy et al., 2005).
Elemental Corg and N concentrations of sediment samples were analyzed with an Organic Elemental Analyzer Flash 200 (Thermo Fisher Scientific, Waltham, MA, United States). The accuracy was <0.2 and <0.1% for C and N, respectively. The δ13C and δ15N analyses of sediment samples were performed by elemental analysis isotope ratio mass spectrometry. Two replicates of Corg and N concentrations and their stable isotopic compositions were analyzed for each sediment horizon. Specifically, analyses of samples collected on November 2014 were performed on a Delta V mass spectrometer with Conflo III coupled to a Costech 4010 elemental analyzer (Thermo Scientific, Waltham, MA, United States) at the UH Hilo Analytical Laboratory (United States). Analyses of samples collected from February 2016 to July 2017 were performed on a Carlo Elba NC1500 (Milan, Italy) elemental analyzer along with a Delta Plus XP (Thermo-Finnigan, Bremen, Germany) mass spectrometer (EA-IRMS) at the Stable Isotope Laboratory of the Andalusian Institute of Earth Sciences (CSIC-UGR, Spain). Commercial CO2 and N2 were used as the internal standard for the C and N isotopic analyses. For δ13C analysis, we used internal standards of -30.63 and -11.65‰ (V-PDB), and for δ15N analysis, we used internal standards of -1.02 and +16.01‰ (AIR). The analytical precision was better than ± 0.1‰ for δ15N and δ13C, calculated from standards systematically interspersed in sample batches and after correcting for the daily drift of the mass spectrometer. The standard for reporting C measurements is V-PDB (Vienna-PDB) and for N measurements the standard is atmospheric nitrogen (AIR). The stable isotopic composition is reported as δ values:
where R = 13C/12C for δ13C, and R = 15N/14N for δ15N values.
Then, we calculated the weighted average per replica taking into account the depth of each horizon. Moreover, we calculated inorganic C (Cinorg), organic C (Corg), and N stocks integrated over the top 10 cm taking into account the sediment Cinorg, Corg, and N concentrations and the bulk density for each sediment horizon. We only integrated the stocks over the analyzed top 10 cm of sediment, despite the general recommendation for sediment stock analysis to sample the top 1 m (Macreadie et al., 2014), because our analyses were restricted by the available sediment thickness at seagrass stations. In many reef lagoons, particularly in the southern Red Sea, sampled seagrass meadows were composed of thin sediment layers, often just barely over 10 cm thick, overlaying carbonate rock. It is, therefore, more appropriate, for comparative purposes, to compare the top 10 cm stock than to extrapolate to 1 m, beyond the sediment thickness present in many of the meadows sampled here. The thickness we used, however, is the same as in a recent study on Corg, N, and phosphorous sediment stocks in Danish seagrass meadows (Kindeberg et al., 2018).
Contribution of Red Sea Primary Producers to Seagrass and Mangrove Sediment
We used recently reported stable isotopic compositions of primary producers in the Red Sea (Duarte et al., 2018) to assess their contribution to the organic matter accumulation in seagrass and mangrove sediments. Specifically, we compiled available δ13C and δ15N values of macroalgae blades, seagrass leaves, halophytes leaves, mangrove leaves, and seston sampled along the Red Sea (Duarte et al., 2018).
We complemented the compilation of stable isotopic composition values of primary producers in the Red Sea reported by Duarte et al. (2018) by sampling and analyzing the δ13C of microbial mats found in Central Red Sea mangrove stands, which was included in the larger data set. Microbial mats were sampled from the sediment near a mangrove stand at the ‘Ibn Sina field research station and nature conservation area’ in the Central Red Sea (22° 20′ 25.032″ N; 39° 5′ 17.411″ E) in April 2018 by carefully collecting the upper layer with a spatula. The δ13C was analyzed by cavity ring-down spectroscopy (CM-CRDS G2201-I, Picarro, Santa Clara, CA, United States) attached to a combustion module (Costech Analytical Technologies, Inc., Valencia, CA, United States) at the Tarek Ahmed Juffali Research Chair in Red Sea Ecology Laboratory (KAUST, Saudi Arabia). Microbial mat samples were dried, acidified and encapsulated in tin boats. We used two CO2 gas Stable Isotope Calibration Standards UN1956 (δ13C = 25 and -40‰, Air Liquide America Specialty Gases, Plumsteadville, PA, United States) and three certified solid C standards from the Reston Stable Isotope Laboratory [United States Geological Survey (USGS), Reston, VA, United States]: USGS62 (Caffeine, δ13C = -14.79‰), USGS40 (glutamic acid, δ13C = -26.39‰), and USGS41a (L- glutamic acid enriched in 13C, δ13C = +36.55‰). The analytical precision and accuracy of δ13C measurements were ± 0.13 and ± 0.69‰, respectively.
We calculated the difference in δ13C and δ15N between sediments and the overlying vegetation for each station, by subtracting the δ13C and δ15N of primary producers from the δ13C and δ15N of sediments, respectively.
Finally, the relative contribution of different primary producers as potential sources of organic matter in seagrass and mangrove sediments was estimated using Bayesian mixing models (Parnell et al., 2010; Phillips et al., 2014). We run the mixing models separately for each type of sediment and we only included as potential sources those primary producers for which both δ13C and δ15N values were available (i.e., macroalgae blades, seagrass leaves, halophytes leaves, and mangrove leaves).
Statistical Analysis
Differences in Cinorg concentration, Corg concentration, N concentration, C/N ratio, bulk density, δ13C, δ15N, Corg stock, N stock, and Cinorg stock between seagrass and mangrove sediments were tested by the parametric unpaired t-test, when data follow the normality assumption, and the non-parametric Mann–Whitney test, when data did not follow the normality assumption. Data normality was checked by the Shapiro–Wilk test. Relationships between latitude and sediment parameters were assessed with linear regression analysis. Differences in the δ13C of seagrass and mangrove sediments and the δ13C of the overlying plants were tested by Wilcoxon matched-pairs signed rank test. Statistical analyses were performed using JMP (SAS Institute, Inc., United States) and PRISM (GraphPad Software, Inc., United States) statistical software; while Bayesian mixing models, used to constrain the likely contribution of different primary producers to seagrass and mangrove sediments, were run using the stable isotope mixing model (simmr) package (Parnell, 2016) in RStudio 1.2.1335 (RStudio Team, 2018).
The data set is available in the data repository Pangaea: Garcias-Bonet et al. (2018). Data set on carbon and nitrogen concentrations, stocks and stable isotope compositions (δ13C, δ15N) in Red Sea seagrass and mangrove sediments. PANGAEA. https://doi.pangaea.de/10.1594/PANGAEA.895644.
Results
Sediment Cinorg, Corg, and N Concentrations
The Cinorg concentration of seagrass and mangrove sediments was 0.09 ± 0.005 and 0.08 ± 0.007 g Cinorg g DW sediment-1, respectively (Table 1); with no significant differences between sediment types (Mann–Whitney test, U = 183, p > 0.05).
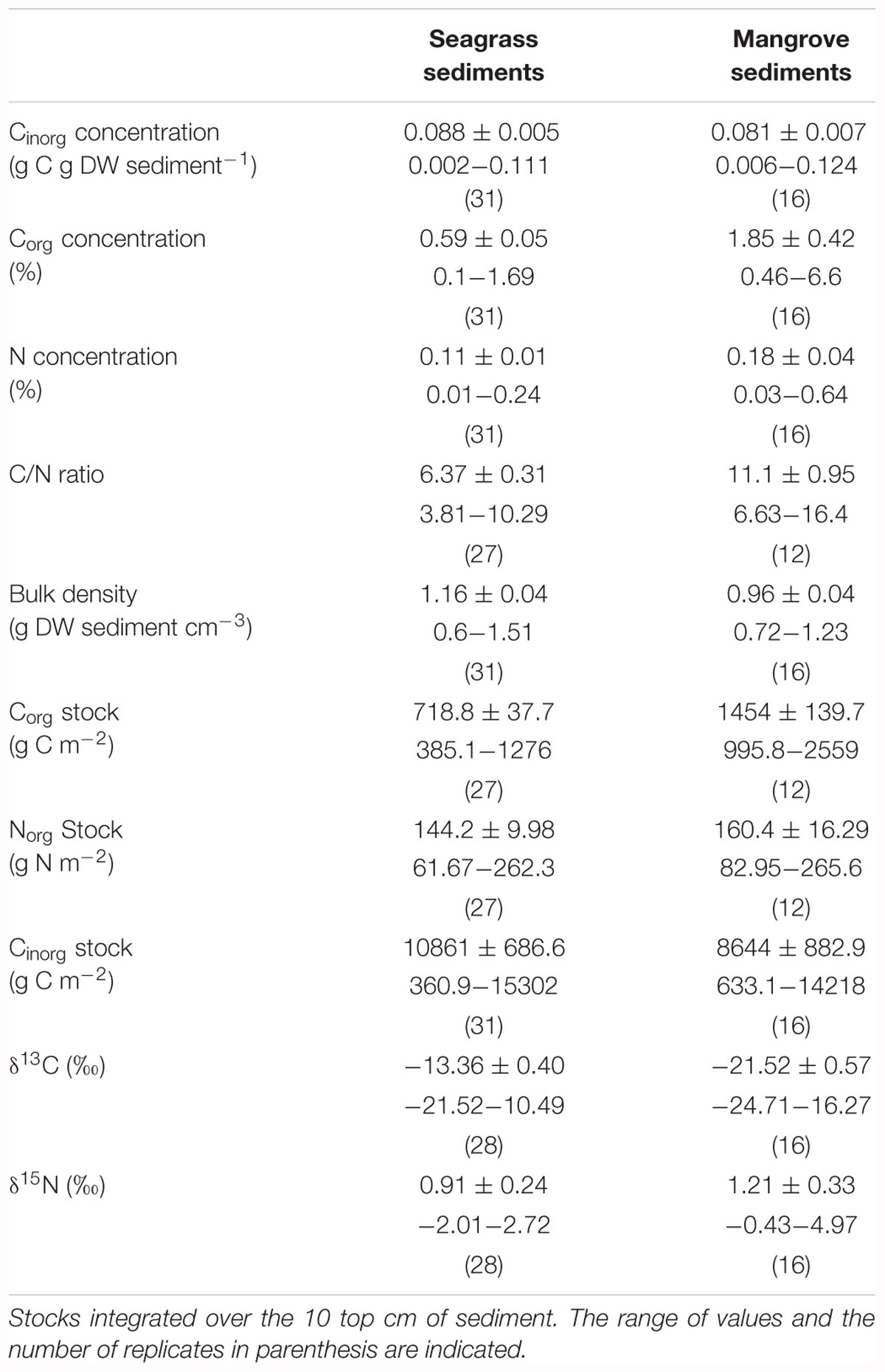
Table 1. Mean ( ± SE) inorganic carbon (Cinorg) concentration, organic carbon (Corg) concentration, nitrogen (N) concentration, C/N ratio, bulk density, Corg stock, organic N (Norg) stock, Cinorg stock, δ13C, and δ15N values of seagrass and mangrove sediments.
The Corg and N concentrations of seagrass and mangrove sediments ranged broadly across the Red Sea, particularly for mangrove sediments. In seagrass sediments, Corg and N concentrations ranged from 0.1 to 1.69% DW and from 0.01 to 0.24% DW, respectively. In mangrove sediments, Corg and N concentrations ranged from 0.46 to 6.6% DW and from 0.03 to 0.64% DW, respectively (Figure 2 and Table 1). The Corg concentration was higher in mangrove (1.85 ± 0.42%) than in seagrass (0.59 ± 0.05%) sediments (Mann–Whitney test, U = 71, p < 0.0001). However, seagrass and mangrove sediments did not have significantly different N concentrations (0.11 ± 0.01 and 0.18 ± 0.04%, respectively; Mann–Whitney test, U = 169, p > 0.05). The sediment N concentration increased linearly with that of Corg in seagrass (Y = 0.13X + 0.02, R2 = 0.51, p < 0.0001, Figure 3A), and mangrove (Y = 0.08X + 0.02, R2 = 0.92, p < 0.0001, Figure 3B) sediments, with significant difference in the slopes between seagrass and mangrove sediments (ANCOVA, F2,142 = 13.34, p < 0.0001). N concentration tended to be higher, for a given Corg concentration, in seagrass compared to mangrove sediments. This implies significantly higher C/N ratios for mangrove (11.10 ± 0.95) than for seagrass (6.37 ± 0.31) sediments (Figure 2 and Table 1, Mann–Whitney test, U = 22, p < 0.0001). Bulk density was higher in seagrass (1.16 ± 0.04 g DW sediment cm-3) compared to mangrove (0.96 ± 0.04 g DW sediment cm-3) sediments (Figure 2 and Table 1, Unpaired t-test, t45 = 3.182, p < 0.01).
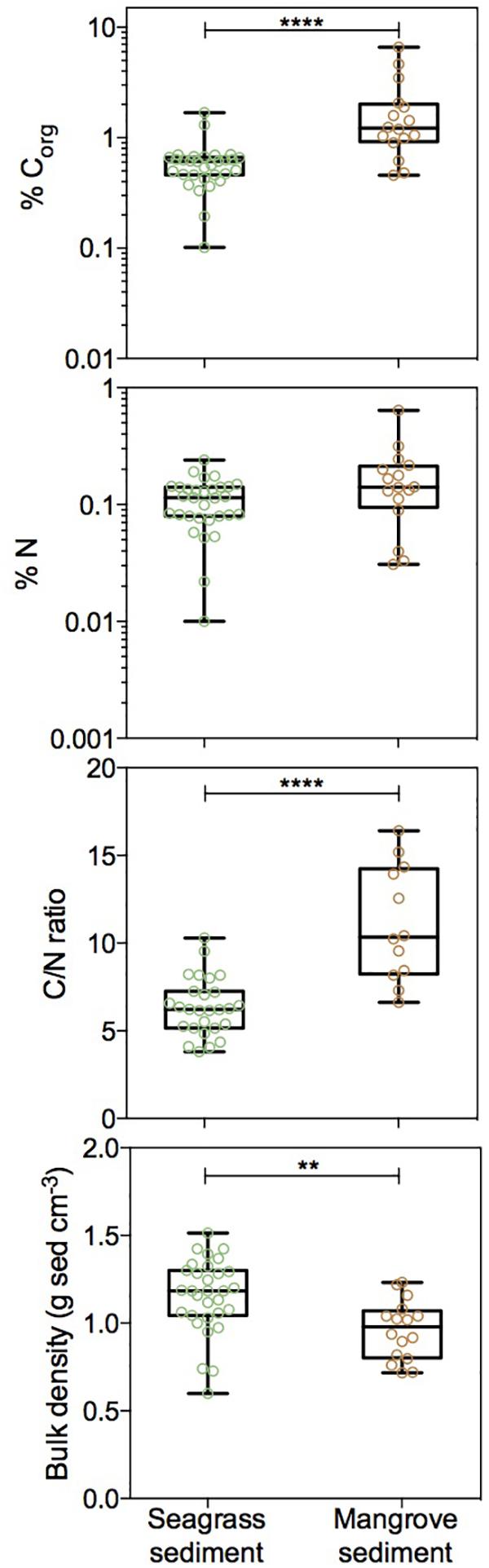
Figure 2. Box plots of Corg concentration, N concentration, C/N ratio, and bulk density for seagrass (green) and mangrove (brown) sediments showing all data points. Boxes extend from the 25th to 75th percentiles, lines inside boxes represent median values, and whiskers span from minimum to maximum values. Statistically significant differences are indicated by asterisks where ∗∗ indicates p < 0.01 and ∗∗∗∗ indicates p < 0.0001.
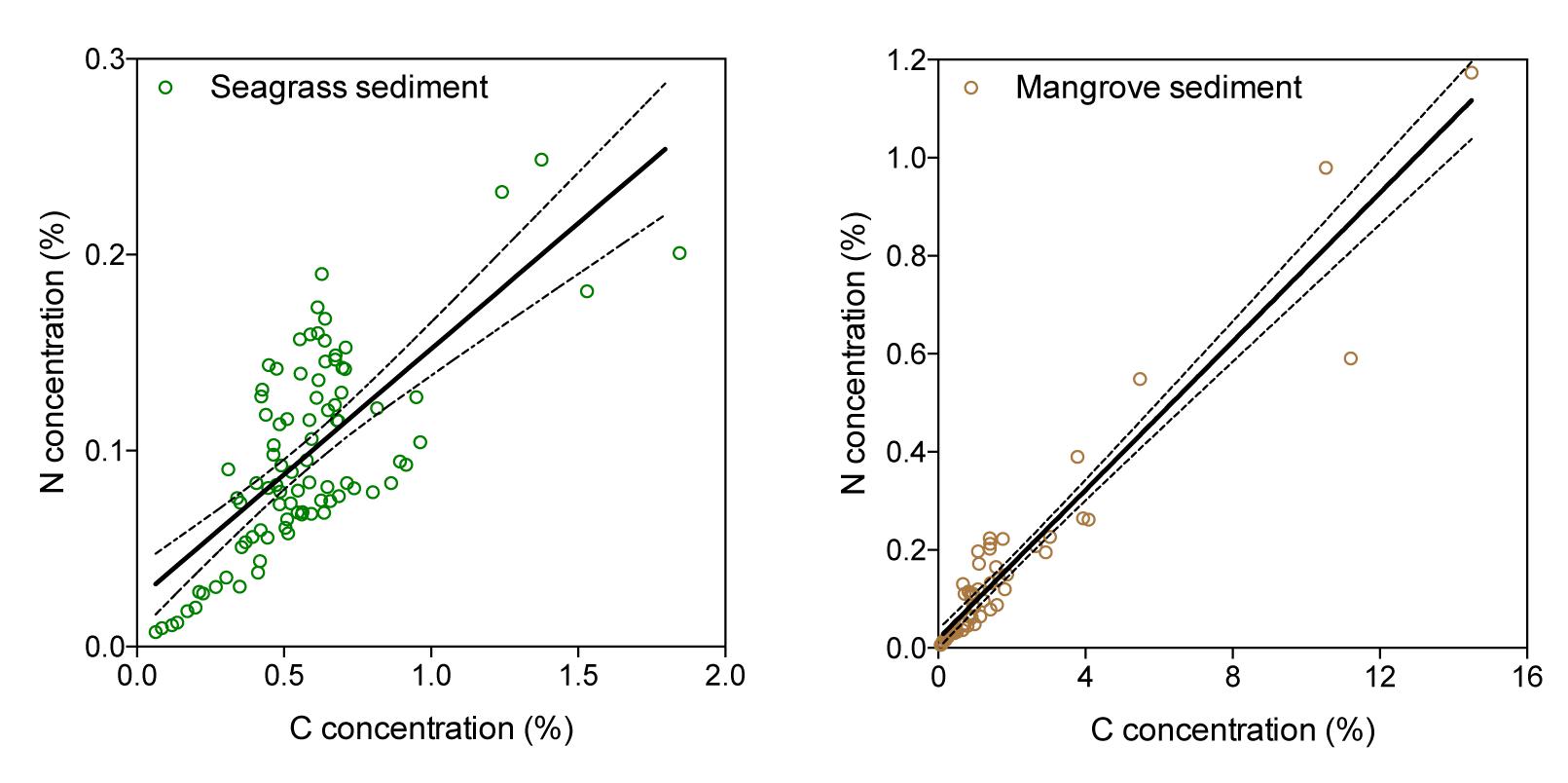
Figure 3. Relationships between N and Corg concentrations in seagrass (left) and mangrove (right) sediments, showing the fitted regression lines (solid lines) and the 95% c.i. (dashed lines).
There was, as hypothesized, a trend for N concentration to decrease from south to north (Figure 4) in seagrass (Y = -0.005X + 0.22, R2 = 0.08; p < 0.01), although this relationship was not significant for mangrove sediments (R2 = 0.05; p > 0.05), which showed high variability in sediment N concentrations across mangrove stands for any one latitude (Figure 4). However, Corg concentration did not show any latitudinal trend in seagrass (R2 = 0.01; p > 0.05) and mangrove (R2 = 0.01; p > 0.05) sediments. Yet, the C/N ratio increased from north to south for both seagrass (Y = 0.27X + 0.28, R2 = 0.23; p < 0.0001) and mangrove (Y = 0.75X - 5.52, R2 = 0.58; p < 0.0001) sediments (Figure 4).
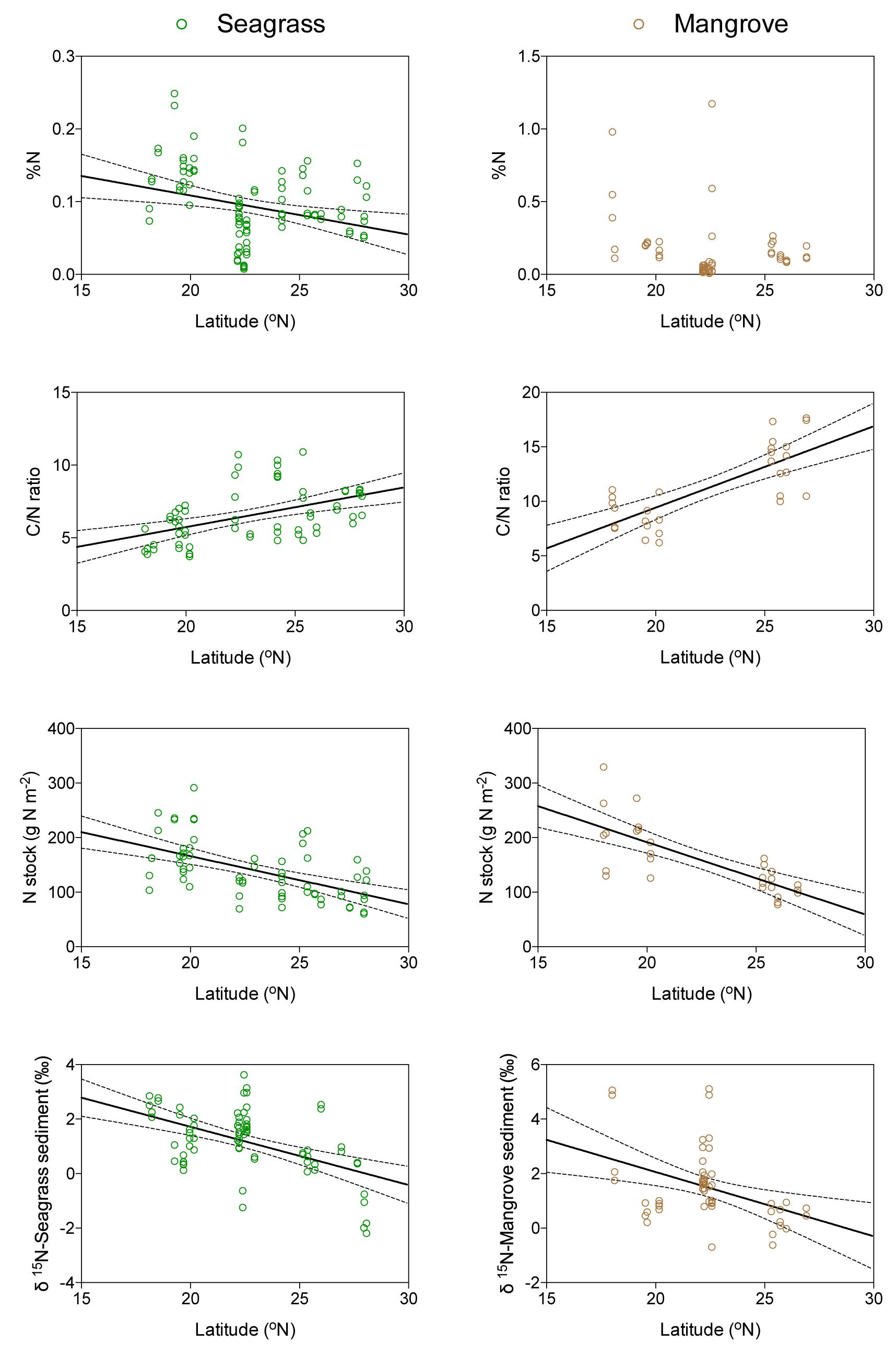
Figure 4. Relationship between latitude and the N concentration, the C/N ratio, the N stock over the top 10 cm of sediment, and the δ15N composition of seagrass (green) and mangrove (brown) sediments. The solid lines represent the linear regression ± 95% c.i. (dashed lines).
Sediment Cinorg, Corg, and N Stocks
Seagrass sediments contained higher Cinorg stocks integrated over the top 10 cm than mangrove sediments (Figure 5 and Table 1, Mann–Whitney test, U = 143, p < 0.05). Mangrove Corg stocks exceeded, on average, twofold those in seagrass meadows (Figure 5 and Table 1, Mann–Whitney test, U = 8, p < 0.0001). However, mangrove sediments contained similar N stocks than seagrass sediments (Figure 5 and Table 1, Mann–Whitney test, U = 136, p > 0.05). Sediment N stocks decreased from south to north in seagrass (Y = -8.81 + 342.4, R2 = 0.31; p < 0.0001) and in mangrove (Y = -13.25X + 456.6, R2 = 0.56; p < 0.0001) sediments (Figure 4). However, Corg stocks did not show any latitudinal trend in seagrass (R2 = 0.06; p > 0.05) and mangrove (R2 = 0.05; p > 0.05) sediments.
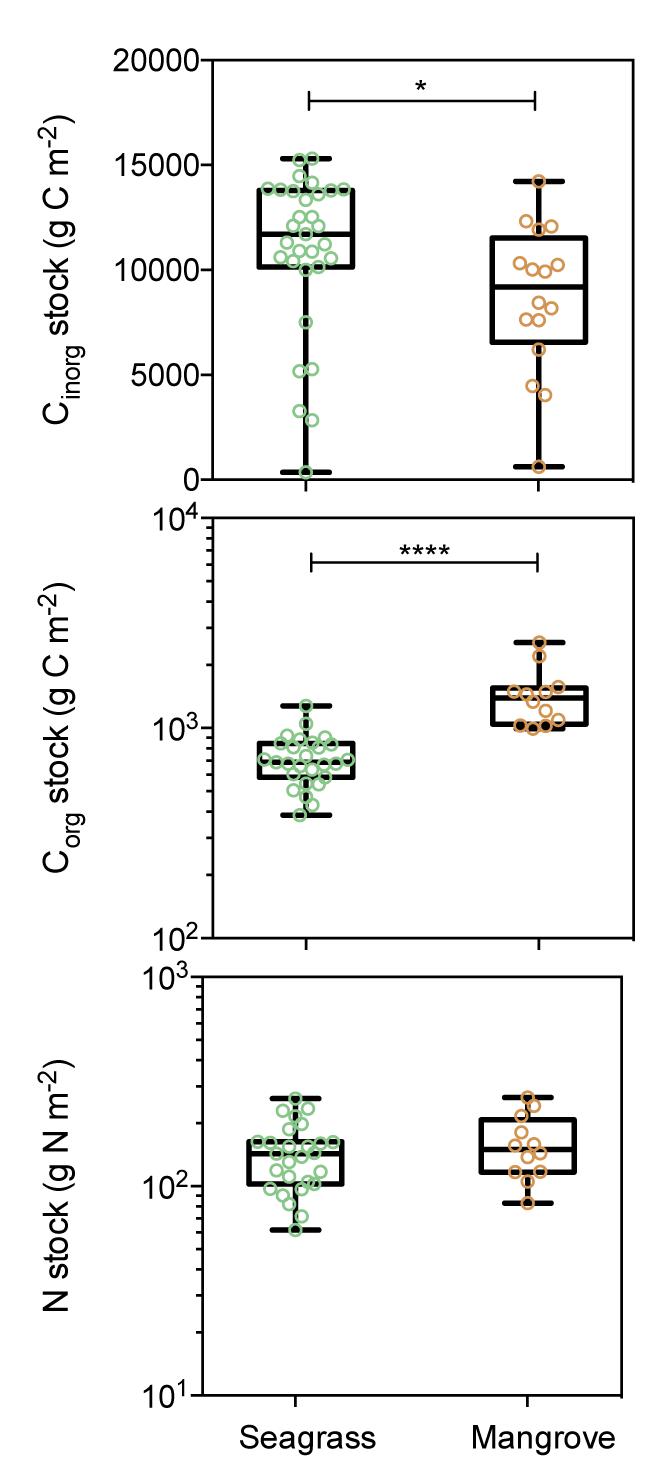
Figure 5. Box plots of Cinorg, Corg, and N stocks for seagrass (green) and mangrove (brown) sediments showing all data points. Stocks integrated over the top 10 cm of sediment. Boxes extend from the 25th to 75th percentiles, lines inside boxes represent median values, and whiskers span from minimum to maximum values. Statistically significant differences are indicated by asterisks where ∗ indicates p < 0.05, and ∗∗∗∗ indicates p < 0.0001.
Sediment C and N Stable Isotope Compositions
The isotopic signature of Corg in seagrass sediments was enriched in 13C relative to that of mangrove sediments (δ13C = -13.36 ± 0.40 and -21.52 ± 0.57‰, respectively; Mann–Whitney test, U = 7, p < 0.0001), while sediment N isotopic signatures were comparable between the two habitats (δ15N = 0.91 ± 0.24 and 1.21 ± 0.33‰ in seagrass and mangrove, respectively; Figure 6 and Table 1). Corg isotope signatures in seagrass sediments were independent of latitude in seagrass and mangrove sediments, while N isotopic composition became lighter from south to north in both seagrass (Y = -0.21X + 5.99, R2 = 0.26; p < 0.0001) and mangrove (Y = -0.24X + 6.78, R2 = 0.16; p < 0.01) sediments (Figure 4).
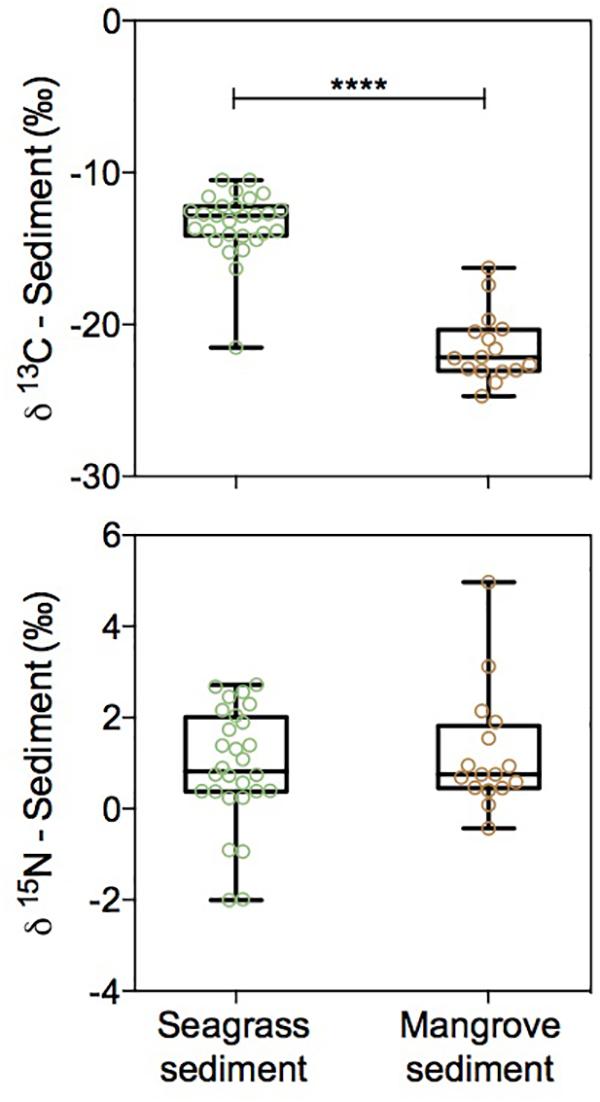
Figure 6. Box plots of δ13C and δ15N for seagrass (green) and mangrove (brown) sediments showing all data points. Boxes extend from the 25th to 75th percentiles, lines inside boxes represent median values, and whiskers span from minimum to maximum values. Statistically significant differences are indicated by asterisks where ∗∗∗∗ indicates p < 0.0001.
Comparison of C and N Stable Isotope Compositions of Seagrass and Mangrove Sediments and Red Sea Primary Producers
The δ13C ( ± SD) of Red Sea primary producers compiled here ranged broadly from -26.59 ± 1.06‰ in mangrove leaves to -7.73 ± 1.48‰ in seagrass leaves, with intermediate values for seston, halophytes leaves, microbial mats, and macroalgae blades (Table 2). Similarly, the δ15N ( ± SD) of Red Sea primary producers ranged from 0.20 ± 2.94‰ in seagrass leaves to 4.19 ± 2.91‰ in halophytes leaves, while mangrove leaves and macroalgae blades had similar mean values (1.72 ± 2.30 and 1.74 ± 1.59‰, respectively; Table 2). No δ15N data available for seston and microbial mats.
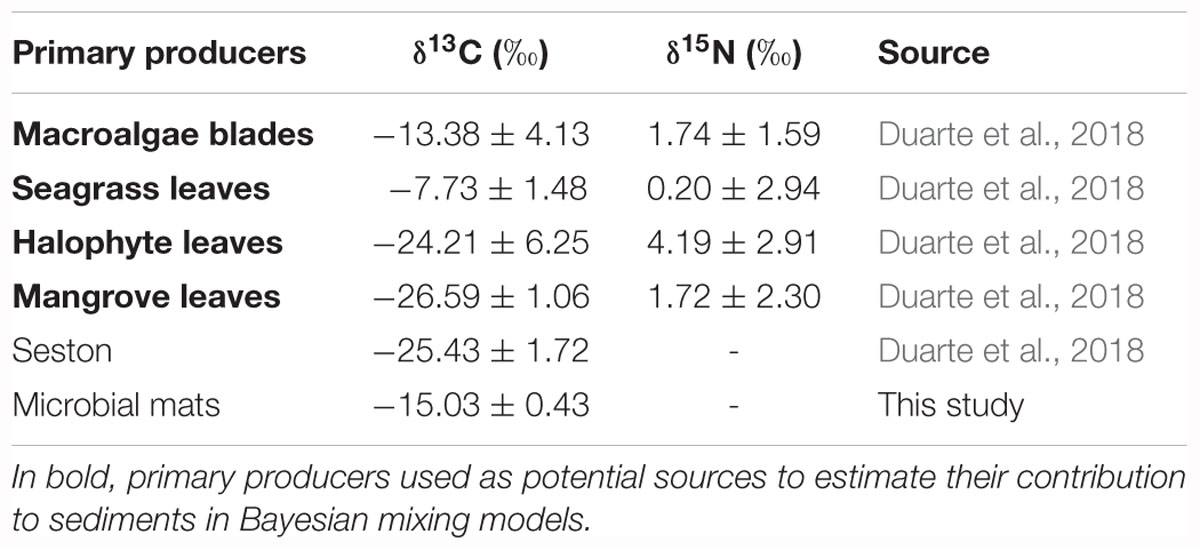
Table 2. Mean ( ± SD) δ13C and δ15N of potential sources for Red Sea seagrass and mangrove sediments.
When comparing the stable isotope compositions of sediments and the overlying vegetation found at each station, seagrass sediments showed lighter Corg isotopic composition than the leaves of overlying seagrass plants (mean δ13C = -13.57 ± 0.40 and -7.69 ± 0.16‰, respectively; Wilcoxon matched-pairs signed rank test, p < 0.0001), resulting in an average shift of -6.17 ± 0.4‰ between the two compartments. Mangrove sediments tended to have isotopically heavier δ13C than the overlying mangrove leaves at each station (mean δ13C = -21.52 ± 0.57 and -26.64 ± 0.20‰, respectively; Wilcoxon matched-pairs signed rank test, p < 0.0001), resulting in an average shift of +5.12 ± 0.45‰ between the two compartments (Supplementary Figures S1, S2). However, the δ13C of seagrass sediments did not differ significantly from that of overlying macroalgae at each station with mean δ13C = -13.44 ± 0.45‰ (Wilcoxon matched-pairs signed rank test, p > 0.05; difference -0.93 ± 0.96‰).
Seagrass and mangrove sediments and overlying plant canopies at each station had similar mean N isotopic compositions (δ15N = 0.91 ± 0.24 and 1.21 ± 0.33‰ in seagrass and mangrove sediments, respectively; δ15N = -0.25 ± 0.56 and 1.37 ± 0.52‰ in seagrass and mangrove leaves, respectively; Supplementary Figure S1).
Potential Contribution of Red Sea Primary Producers to Seagrass and Mangrove Sediments
Mean δ13C and δ15N values of seagrass and mangrove sediments laid within the convex hull defined by δ13C and δ15N mean values of primary producers (Figure 7). The Bayesian mixing models identified different primary producers as main sources of organic matter in seagrass and mangrove sediments (Figure 8 and Table 3). Seagrass leaves and macroalgae blades were the major potential contributors in seagrass sediments (mean ± SD proportion = 0.43 ± 0.08 and 0.37 ± 0.12, respectively); while mangrove and halophytes leaves had a minor contribution. Mangrove leaves were the main potential contributors in mangrove sediments (mean ± SD proportion = 0.56 ± 0.08), with minor contributions of macroalgae blades, seagrass leaves and halophytes leaves (Figure 8 and Table 3).
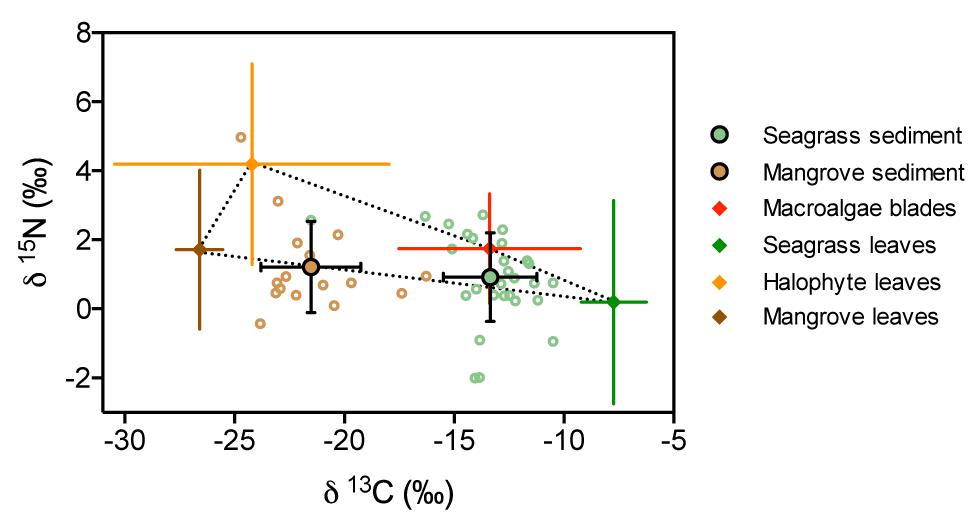
Figure 7. Iso-space plot showing mean ( ± SD) δ13C and δ15N values of seagrass and mangrove sediments (green and brown circles, respectively) and potential sources contributing to their accumulated organic matter (color coded diamonds). For seagrass and mangrove sediments all data points are shown (green and brown open circles, respectively). Bars indicate standard deviation used in the input of Bayesian mixing models. Dotted line shows the convex hull defined by potential sources.
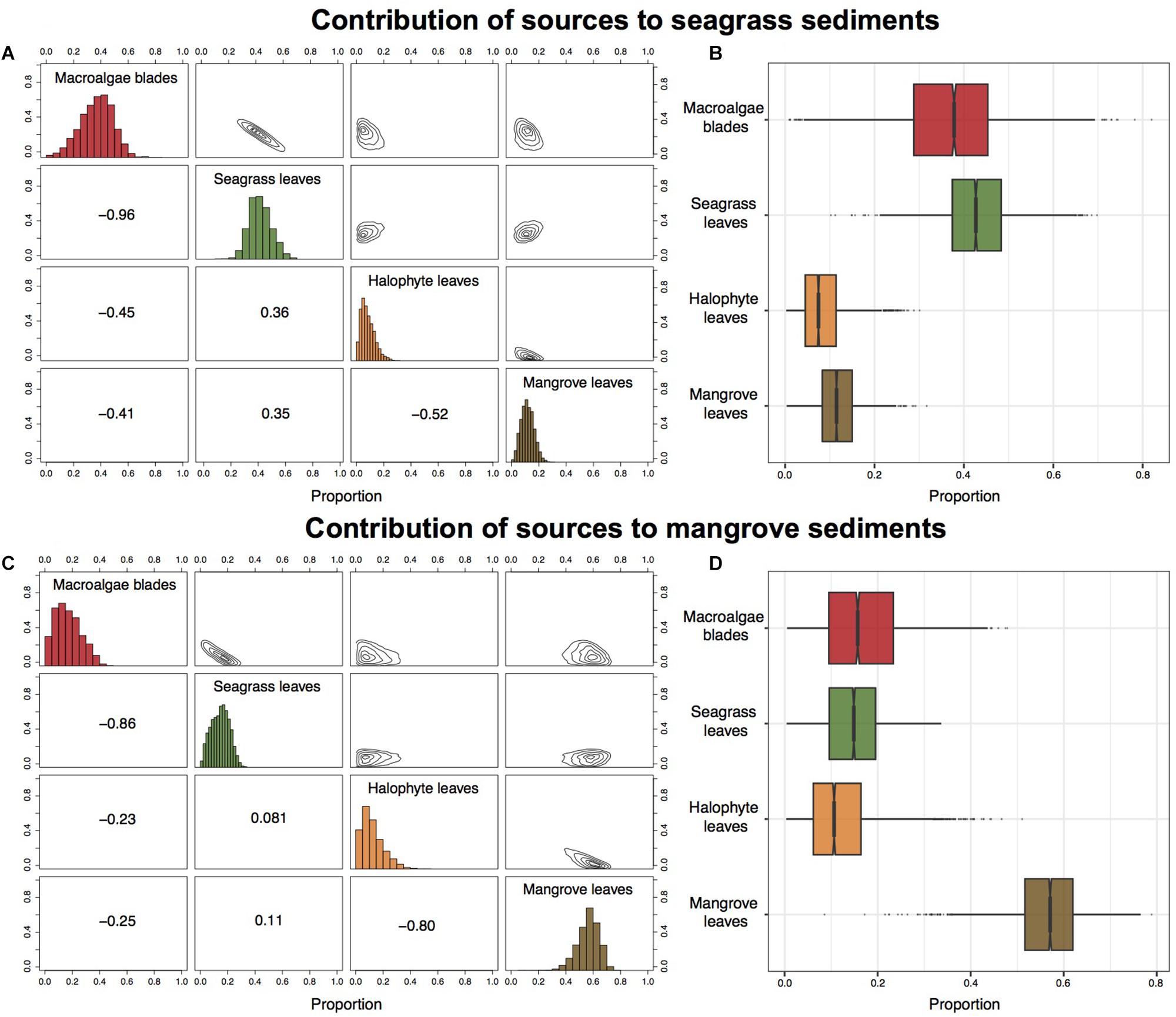
Figure 8. Contribution of primary producers to the accumulated organic matter in seagrass (A,B) and mangrove (C,D) sediments in the Red Sea calculated using Bayesian mixing models. Matrix plots for seagrass (A) and mangrove (C) sediments, showing probability distributions of each source (in diagonal panels), the joint probability between pairs of sources (in top panels), and the correlations between pairs of sources (in bottom panels). Box plots of the proportional contribution by each source to seagrass (B) and mangrove (D) sediments. Boxes extend from the 25th to 75th percentiles and lines inside boxes represent mean values.
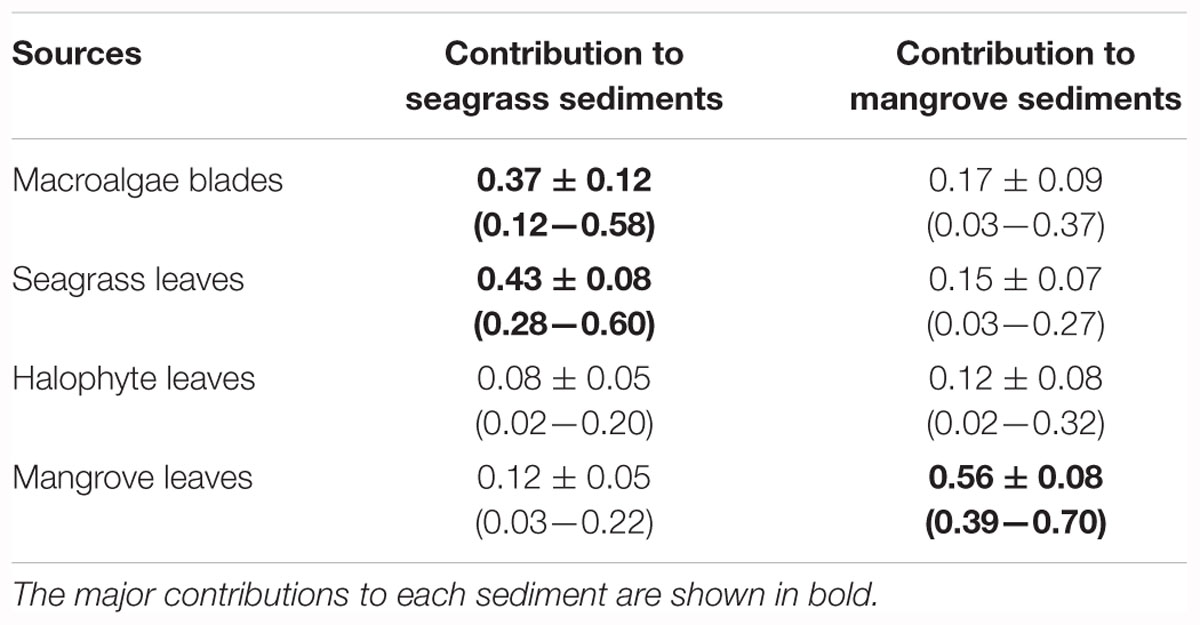
Table 3. Contribution of each source to the accumulated organic matter in seagrass and mangrove sediments estimated using stable isotope mixing models (simmr), showing mean ± SD and 95% credible intervals in parenthesis.
Discussion
Red Sea Sediment Stocks
Seagrass and mangrove sediments in the Red Sea are carbonate-rich compared to previous reports (Mazarrasa et al., 2015; Saderne et al., 2018). Red Sea seagrass Cinorg stocks in the top 10 cm of sediment (10861 ± 686.6 g C m-2) are higher than previous global seagrass estimates (6540 ± 240 g C m-2 in 10 cm of sediment, Mazarrasa et al., 2015) and previous estimates of central Red Sea seagrass sediments (5700 ± 300 g C m-2 in 10 cm of sediment, Saderne et al., 2018). Mangrove Cinorg stocks in the top 10 cm of sediment (8644 ± 882.9 g C m-2) are equally large, and comparable to previous data from the central Red Sea (8900 ± 400 g C m-2 in 10 cm of sediment, Saderne et al., 2018). However, seagrass and mangrove sediments were depleted in Corg and N relative to sediments in these coastal habitats elsewhere (Donato et al., 2011; Fourqurean et al., 2012; Atwood et al., 2017), consistent with previous reports pointing at low concentrations of Corg in Central Red Sea mangrove (Almahasheer et al., 2017) and seagrass (Serrano et al., 2018) sediments. The low concentration of Corg in Red Sea Blue Carbon habitats is partially attributable to the absence of rivers in the Red Sea, thereby limiting the supply of allochthonous C to these sediments (Almahasheer et al., 2017; Serrano et al., 2018). Indeed, arid marine ecosystems are poorly represented in global syntheses of the Corg stocks in Blue Carbon habitats (Almahasheer et al., 2017). Red Sea mangrove Corg stocks integrated over the top 10 cm of sediment (14.5 ± 1.4 Mg C ha-1) are lower than mean values for mangrove sediments at the latitudinal range encompassed by the Red Sea (20 to 31 Mg C ha-1 in 10 cm of sediment, Atwood et al., 2017). Similarly, Red Sea seagrass Corg stocks integrated over the top 10 cm of sediment (7.2 ± 0.4 Mg C ha-1) are lower than mean global values (ranging from 11.5 to 82.9 Mg C ha-1 in 10 cm of sediment, Fourqurean et al., 2012). However, these global values are heavily skewed toward carbon-dense Mediterranean Posidonia oceanica meadows, and therefore likely overestimate global mean Corg stocks in seagrass sediments (e.g., Lavery et al., 2013). Yet, our estimates on seagrass Corg stocks are twofold higher than recently reported stocks for seagrasses in the Central Red Sea (3.4 ± 0.3 Mg C ha-1 in 10 cm of sediment, Serrano et al., 2018). These results confirm that the general tendency for mangrove sediments to be more organic carbon-rich than seagrass sediments (McLeod et al., 2011; Duarte et al., 2013) also applies to the arid sediments of the Red Sea, lacking riverine inputs. Since available data are reported as stocks over the top 1 m of sediment, we recalculated the fraction corresponding to 10 cm of sediment to allow comparison. This may generate uncertainties, as this assumes that the Corg content is relatively uniform, but is also consistent with the fact that literature estimates for seagrass sediments were mostly based on extrapolating from sediment corers well below 1 m (Fourqurean et al., 2012; Mazarrasa et al., 2015).
Corg and N sediment stocks were closely coupled, reflecting the stoichiometric compositions in overlying vegetation and suggesting that N supply constrains sediment Corg stocks. However, mangrove sediments were depleted in N, relative to Corg, compared to seagrass sediments. This is consistent with the generally higher C/N ratio of mangrove leaves compared to seagrass leaves (Cebrián and Duarte, 1995). As the C/N ratio of organic matter is a robust predictor of decomposition rates (Enríquez et al., 1993), high C/N ratios in mangrove leaves and sediments, compared to those in seagrass meadows, imply lower decomposition rates and, hence, higher accumulation rates in mangrove sediments relative to seagrass sediments.
A Latitudinal Gradient in Productivity Affects Sediment N in the Red Sea
The results confirmed that the south to north gradient of decreasing nutrient inputs characteristic of the Red Sea (Raitsos et al., 2015) is reflected in a decrease in sediment N stocks and an increase in sediment C/N ratios from south to north. The gradient is also reflected in a tendency for the δ15N isotopic ratio in sediments to decrease from south to north. This gradient suggests a shift from nitrate and nitrite delivered from the incoming Indian Ocean as N sources in the south, to nitrogen fixation, characterized by lower δ15N (Peterson and Fry, 1987; Papadimitriou et al., 2005; Garcias-Bonet et al., 2016), in the absence of allochthonous sources of reactive N, toward the north. However, there was no such latitudinal trend in Corg stocks in seagrass and mangrove sediments, indicating that the higher nutrient supply in the southern Red Sea does not result in higher sediment Corg stocks. This could be explained by lower sediment C/N ratios in the Southern Red Sea resulting in higher decomposition rates than in the comparatively N-depleted sediments in the Northern Red Sea.
Primary Producers as Sources of Sediment Organic Matter
Mean δ13C of Red Sea primary producers were reported to range from highly negative for mangrove leaves to the heaviest values for seagrass leaves, with intermediate values for halophyte leaves, macroalgae blades, and seston (Duarte et al., 2018). In addition, sampled microbial mats showed intermediate mean δ13C (-15.03 ± 0.25‰), similar to previously reported values for these communities (between -14.4 and -13‰, Oakes and Eyre, 2014).
The difference in δ13C between seagrass and mangrove sediments and their leaves suggests that other sources of carbon than habitat-forming primary producers contribute to the sediment Corg stocks. Stable isotope mixing models showed that in Red Sea seagrass sediments both seagrass leaves and macroalgae blades were the major contributors to the accumulated organic matter, accounting for 80% together; while in Red Sea mangrove sediments, mangrove leaves were the major contributors, with a 56%. The important contribution of sources other than the habitat-forming primary producers to sediment organic matter is consistent with previous results. For instance, Kennedy et al. (2010) reported that half of the Corg in seagrass sediment stocks derived from other primary producers than seagrass. Other potential sources not included in the mixing models could also contribute to the accumulated organic matter in seagrass and mangrove sediments. For instance, previous results for mangrove sediments elsewhere, pointed at an important role of microbial mats as a source of Corg to underlying sediments and mangrove food webs (Newell et al., 1995; Bouillon et al., 2008; Kristensen et al., 2008). Moreover, the high correlation observed between pairs of sources in both types of sediment indicated that the mixing model could not successfully distinguish between them. This is the case of macroalgae blades and seagrass leaves in seagrass sediments, which were strongly negatively correlated (-0.96); indicating that if macroalgae blades contributed to seagrass sediments at the top of their outcome probability range, most likely seagrass leaves contributed at the bottom of their probability range. Similarly, in mangrove sediments, we observed two pairs of sources strongly negatively correlated: macroalgae blades – seagrass leaves (-0.86) and halophytes leaves – mangrove leaves (-0.80).
Nitrogen sources to sediments include nitrate advected with the inflow of Indian Ocean waters (δ15N = + 6‰, Brandes et al., 1998), and N fixation (δ15N near 0, Peterson and Fry, 1987). The δ15N of sediment N stocks in the south is consistent with a dominance of nitrate as the main source of N, while the δ15N of sediment N stocks in the north points at a prevalence of N fixation. However, due to diagenetic processes, the isotopic signature of sediment organic matter might change during decomposition (Macko et al., 1994). Therefore, the identification of potential sources and contributions to seagrass and mangrove sediments based on stable isotopes needs to be interpreted with care.
Conclusion
Corg and N stocks in seagrass and mangrove sediments were low, particularly for seagrass sediments, in the Eastern Red Sea. Whereas sediment N concentration and stocks decreased from south to north in the Red Sea, Corg stocks did not change consistently with latitude. Seagrass and mangrove leaves largely contributed to the accumulated organic matter in their sediments, with additional contribution from macroalgae in seagrass sediments. These results represent a major advancement for sediment Corg, Cinorg, and N stock assessments of seagrass and mangrove in the Red Sea, providing a background for Blue Carbon programs in the region. By filling a regional gap of knowledge, these data also help to balance global Blue Carbon assessments.
Author Contributions
NG-B and CD analyzed the data and wrote the first draft of the manuscript. All authors designed the research, conducted sampling and analyses, contributed to writing the manuscript and approved the submission.
Funding
This research was funded by the King Abdullah University of Science and Technology through base-line funding to CD and CCF funding to the Red Sea Research Center.
Conflict of Interest Statement
The authors declare that the research was conducted in the absence of any commercial or financial relationships that could be construed as a potential conflict of interest.
Acknowledgments
We thank Vijayalaxmi Dasari, Isidora Mendia Saez de Zuazola, Mongi Ennasri, Nadia Haj Salah, and Arsenio Granados for support in the laboratory analysis and CMOR, Amr Gusti, Mohammed Aljehdali, and the R. V. Thuwal crew for logistical support.
Supplementary Material
The Supplementary Material for this article can be found online at: https://www.frontiersin.org/articles/10.3389/fmars.2019.00267/full#supplementary-material
References
Almahasheer, H., Duarte, C. M., and Irigoien, X. (2016a). Nutrient limitation in central red sea mangroves. Front. Mar. Sci. 3:271. doi: 10.1038/s41598-017-10424-9
Almahasheer, H., Duarte, C. M., and Irigoien, X. (2016b). Phenology and growth dynamics of avicennia marina in the central red sea. Sci. Rep. 6:37785. doi: 10.1038/srep37785
Almahasheer, H., Serrano, O., Duarte, C. M., Arias-Ortiz, A., Masque, P., and Irigoien, X. (2017). Low carbon sink capacity of Red Sea mangroves. Sci. Rep. 7:9700. doi: 10.1038/s41598-017-10424-9
Anton, A., Hendriks, I. E., Marba, N., Krause-Jensen, D., Garcias-Bonet, N., and Duarte, C. M. (2018). Iron deficiency in seagrasses and macroalgae in the red sea is unrelated to latitude and physiological performance. Front. Mar. Sci. 5:74. doi: 10.3389/fmars.2018.00074
Atwood, T. B., Connolly, R. M., Almahasheer, H., Carnell, P. E., Duarte, C. M., Lewis, C. J. E., et al. (2017). Global patterns in mangrove soil carbon stocks and losses. Nat. Clim. Change 7:523.
Bouillon, S., Connolly, R., and Lee, S. (2008). Organic matter exchange and cycling in mangrove ecosystems: recent insights from stable isotope studies. J. Sea Res. 59, 44–58. doi: 10.1016/j.seares.2007.05.001
Brandes, J. A., Devol, A. H., Yoshinari, T., Jayakumar, D., and Naqvi, S. (1998). Isotopic composition of nitrate in the central Arabian Sea and eastern tropical North Pacific: a tracer for mixing and nitrogen cycles. Limnol. Oceanogr. 43, 1680–1689. doi: 10.4319/lo.1998.43.7.1680
Cebrián, J., and Duarte, C. M. (1995). Plant growth-rate dependence of detrital carbon storage in ecosystems. Science 268, 1606–1608. doi: 10.1126/science.268.5217.1606
Chaidez, V., Dreano, D., Agusti, S., Duarte, C. M., and Hoteit, I. (2017). Decadal trends in Red Sea maximum surface temperature. Sci. Rep. 7:8144. doi: 10.1038/s41598-017-08146-z
Cusack, M., Saderne, V., Arias-Ortiz, A., Masqué, P., Krishnakumar, P., Rabaoui, L., et al. (2018). Organic carbon sequestration and storage in vegetated coastal habitats along the western coast of the Arabian Gulf. Environ. Res. Lett. 13, 074007. doi: 10.1088/1748-9326/aac899
Donato, D. C., Kauffman, J. B., Murdiyarso, D., Kurnianto, S., Stidham, M., and Kanninen, M. (2011). Mangroves among the most carbon-rich forests in the tropics. Nat. Geosci. 4:293.
Duarte, C. M. (2017). Reviews and syntheses: hidden forests, the role of vegetated coastal habitats in the ocean carbon budget. Biogeosciences 14:301. doi: 10.5194/bg-14-301-2017
Duarte, C. M., Delgado-Huertas, A., Anton, A., Carrillo-de-Albornoz, P., Lopez Sandoval, D. C., Agusti, S., et al. (2018). Stable isotope (δ13C, δ15N, δ18O, δD) composition and nutrient concentration of red sea primary producers. Front. Mar. Sci. 5:298. doi: 10.3389/fmars.2018.00298
Duarte, C. M., Losada, I. J., Hendriks, I. E., Mazarrasa, I., and Marba, N. (2013). The role of coastal plant communities for climate change mitigation and adaptation. Nat. Clim. Change 3, 961–968. doi: 10.1038/nclimate1970
Enríquez, S., Duarte, C. M., and Sand-Jensen, K. (1993). Patterns in decomposition rates among photosynthetic organisms: the importance of detritus C: N: P content. Oecologia 94, 457–471. doi: 10.1007/BF00566960
Fourqurean, J. W., Duarte, C. M., Kennedy, H., Marbà, N., Holmer, M., Mateo, M. A., et al. (2012). Seagrass ecosystems as a globally significant carbon stock. Nat. Geosci. 5, 505–509. doi: 10.1002/eap.1489
Garcias-Bonet, N., Arrieta, J. M., Duarte, C. M., and Marba, N. (2016). Nitrogen-fixing bacteria in Mediterranean seagrass (Posidonia oceanica) roots. Aquat. Bot. 131, 57–60. doi: 10.1016/j.aquabot.2016.03.002
Garcias-Bonet, N., and Duarte, C. M. (2017). Methane production by seagrass ecosystems in the Red Sea. Front. Mar. Sci. 4:340. doi: 10.3389/fmars.2017.00340
Garcias-Bonet, N., Delgado Huertas, A., Carrillo-de-Albornoz, P., Anton, A., Almhasheer, H., Marbá, N., et al. (2018). Data set on carbon and nitrogen concentrations, stocks, and isotopic composition in Red Sea seagrass and mangrove sediments. PANGAEA. doi: 10.1594/PANGAEA.895644
Hedges, J. I., and Stern, J. H. (1984). Carbon and nitrogen determinations of carbonate-containing solids1. Limnol. Oceanogr. 29, 657–663. doi: 10.4319/lo.1984.29.3.0657
Kennedy, H., Beggins, J., Duarte, C. M., Fourqurean, J. W., Holmer, M., Marbà, N., et al. (2010). Seagrass sediments as a global carbon sink: isotopic constraints. Global Biogeochem. Cycles 24, GB4026.
Kennedy, P., Kennedy, H., and Papadimitriou, S. (2005). The effect of acidification on the determination of organic carbon, total nitrogen and their stable isotopic composition in algae and marine sediment. Rapid Commun. in Mass Spectrom. 19, 1063–1068. doi: 10.1002/rcm.1889
Kindeberg, T., Ørberg, S. B., Röhr, M. E., Holmer, M., and Krause-Jensen, D. (2018). Sediment stocks of carbon, nitrogen, and phosphorus in danish eelgrass meadows. Front. Mar. Sci. 5:474. doi: 10.3389/fmars.2018.00474
Kristensen, E., Bouillon, S., Dittmar T., and Marchand, C. (2008). Organic carbon dynamics in mangrove ecosystems: a review. Aquatic botany 89, 201–219. doi: 10.1016/j.aquabot.2007.12.005
Lavery, P. S., Mateo, M. -Á, Serrano, O., and Rozaimi, M. (2013). Variability in the carbon storage of seagrass habitats and its implications for global estimates of blue carbon ecosystem service. PloS One 8:e73748. doi: 10.1371/journal.pone.0073748
Macko, S. A., Engel, M. H., and Qian, Y. (1994). Early diagenesis and organic matter preservation – a molecular stable carbon isotope perspective. Chem. Geol. 114, 365–379. doi: 10.1016/0009-2541(94)90064-7
Macreadie, P. I., Baird, M. E., Trevathan-Tackett, S. M., Larkum, A. W. D., and Ralph, P. J. (2014). Quantifying and modelling the carbon sequestration capacity of seagrass meadows–a critical assessment. Mar. Pollut. Bull. 83, 430–439. doi: 10.1016/j.marpolbul.2013.07.038
Mazarrasa, I., Marbá, N., Lovelock C. E., Serrano, O., Lavery, P., Fourqurean, J., et al. (2015). Seagrass meadows as a globally significant carbonate reservoir. Biogeosci. 12, 4993–5003. doi: 10.5194/bg-12-4993-2015
McLeod, E., Chmura, G. L., Bouillon, S., Salm, R., Björk, M., Duarte, C. M., et al. (2011). A blueprint for blue carbon: toward an improved understanding of the role of vegetated coastal habitats in sequestering CO2. Front. Ecol. Environ. 9:552–560. doi: 10.1890/110004
Müller, G., and Gastner, M. (1971). The’Karbonat-Bombe’, a simple device for the determination of carbonate content in sediment, soils, and other materials. Neues Jahrbuch für Mineralogie-Monatshefte Bremerhaven, Monatshefte,466–469.
Nellemann, C., Corcoran, E., Duarte, C. M., Valdés, L., De Young, C., Fonseca, L., (Eds) et al. (2009). Blue Carbon. A Rapid Response Assessment. Nairobi, United Nations Environment Programme, GRID-Arendal
Newell, R., Marshall, N., Sasekumar, A., and Chong, V. (1995). Relative importance of benthic microalgae, phytoplankton, and mangroves as sources of nutrition for penaeid prawns and other coastal invertebrates from Malaysia. Mar. Biol. 123, 595–606. doi: 10.1007/bf00349238
Oakes, J. M., and Eyre, B. D. (2014). Transformation and fate of microphytobenthos carbon in subtropical, intertidal sediments: potential for long-term carbon retention revealed by 13 C-labeling. Biogeosciences 11, 1927–1940. doi: 10.5194/bg-11-1927-2014
Papadimitriou, S., Kennedy, H., Kennedy, D. P., Duarte, C. M., and Marba, N. (2005). Sources of organic matter in seagrass-colonized sediments: a stable isotope study of the silt and clay fraction from Posidonia oceanica meadows in the western Mediterranean. Org. Geochem. 36, 949–961. doi: 10.1016/j.orggeochem.2004.12.002
Parnell, A. (2016). Simmr: a stable isotope mixing model. R package version 0.3. https://CRAN.R-project.org/package=simmr (accessed Janauary 19, 2016).
Parnell, A. C., Inger, R., Bearhop, S., and Jackson, A. L. (2010). Source partitioning using stable isotopes: coping with too much variation. PLoS One 5:e9672. doi: 10.1371/journal.pone.0009672
Peterson, B. J., and Fry, B. (1987). Stable isotopes in ecosystem studies. Ann. Rev. Ecol. System. 18, 293–320. doi: 10.1146/annurev.ecolsys.18.1.293
Phillips, D. L., Inger, R., Bearhop, S., Jackson, A. L., Moore, J. W., Parnell, A. C., et al. (2014). Best practices for use of stable isotope mixing models in food-web studies. Can. J. Zool. 92, 823–835. doi: 10.1139/cjz-2014-0127
Raitsos, D. E., Pradhan, Y., Brewin, R. J. W., Stenchikov, G., and Hoteit, I. (2013). Remote Sensing the Phytoplankton Seasonal Succession of the Red Sea. PLoS One 8:e64909. doi: 10.1371/journal.pone.0064909
Raitsos, D. E., Yi, X., Platt, T., Racault, M. F., Brewin, R. J., Pradhan, Y., et al. (2015). Monsoon oscillations regulate fertility of the Red Sea. Geophy. Res. Lett. 42, 855–862. doi: 10.1002/2014gl062882
Ryba, S. A., and Burgess, R. M. (2002). Effects of sample preparation on the measurement of organic carbon, hydrogen, nitrogen, sulfur, and oxygen concentrations in marine sediments. Chemosphere 48, 139–147. doi: 10.1016/s0045-6535(02)00027-9
Saderne, V., Cusack, M., Almahasheer, H., Serrano, O., Masqué, P., Arias-Ortiz, A., et al. (2018). Accumulation of carbonates contributes to coastal vegetated ecosystems keeping pace with sea level rise in an arid region (Arabian Peninsula). J. Geophy. Res.: Biogeosci. 123, 1498–1510. doi: 10.1029/2017jg004288
Sea, M. A., Garcias-Bonet, N., Saderne, V., and Duarte, C. M. (2018). Carbon dioxide and methane fluxes at the air–sea interface of Red Sea mangroves. Biogeosciences 15, 5365–5375. doi: 10.5194/bg-15-5365-2018
Keywords: nitrogen, carbon, sediment stocks, stable isotopes, stable isotope mixing model, seagrasses, mangroves
Citation: Garcias-Bonet N, Delgado-Huertas A, Carrillo-de-Albornoz P, Anton A, Almahasheer H, Marbà N, Hendriks IE, Krause-Jensen D and Duarte CM (2019) Carbon and Nitrogen Concentrations, Stocks, and Isotopic Compositions in Red Sea Seagrass and Mangrove Sediments. Front. Mar. Sci. 6:267. doi: 10.3389/fmars.2019.00267
Received: 10 October 2018; Accepted: 03 May 2019;
Published: 29 May 2019.
Edited by:
Selvaraj Kandasamy, Xiamen University, ChinaReviewed by:
Limin Hu, State Oceanic Administration, ChinaAntonio Bode, Instituto Español de Oceanografía (IEO), Spain
Arvind Singh, Physical Research Laboratory, India
Copyright © 2019 Garcias-Bonet, Delgado-Huertas, Carrillo-de-Albornoz, Anton, Almahasheer, Marbà, Hendriks, Krause-Jensen and Duarte. This is an open-access article distributed under the terms of the Creative Commons Attribution License (CC BY). The use, distribution or reproduction in other forums is permitted, provided the original author(s) and the copyright owner(s) are credited and that the original publication in this journal is cited, in accordance with accepted academic practice. No use, distribution or reproduction is permitted which does not comply with these terms.
*Correspondence: Neus Garcias-Bonet, bmV1cy5nYXJjaWFzYm9uZXRAa2F1c3QuZWR1LnNh