- 1Department of Natural Sciences, University of Agder, Kristiansand, Norway
- 2Norwegian Institute for Water Research, Grimstad, Norway
- 3Centre for Coastal Research, University of Agder, Kristiansand, Norway
Organic matter (OM) in aquatic systems is either produced internally (autochthonous OM) or delivered from the terrestrial environment (ter-OM). For eutrophication (or the reverse – oligotrophication), the amount of autochthonous OM plays a key role for coastal ecosystem health. However, the influence of ter-OM on eutrophication or oligotrophication processes of coastal ecosystems is largely unclear. Therefore, ter-OM, or ter-OM proxies are currently not included in most policies or monitoring programs on eutrophication. Nevertheless, ter-OM is increasingly recognized as a strong driver of aquatic productivity: By influencing underwater light conditions and nutrient- and carbon availability, increased ter-OM input may shift systems from autotrophic toward heterotrophic production, but also alter the interactions between benthic, and pelagic habitats. Thus, by changing baseline conditions in coastal zones, ongoing, and predicted changes in inputs of ter-OM due to climate change (e.g., in precipitation) and anthropogenic activities (e.g., reduced sulfate deposition, damming, and coastal erosion) may strongly modify eutrophication symptoms within affected ecosystems, but also hinder recovery from eutrophication following a reduction in nutrient loadings (i.e., oligotrophication). In this review, we aim to shed light upon the role of ter-OM for coastal eutrophication and oligotrophication processes and ecosystem health. Specifically, we (1) discuss the theoretical interactions between ter-OM and eutrophication and oligotrophication processes in coastal waters, (2) present global case studies where altered ter-OM supply to coastal ecosystems has shifted baseline conditions, with implications for eutrophication and oligotrophication processes, and (3) provide an outlook and recommendations for the future management of coastal zones given changes in ter-OM input. We conclude that it is essential to include and target all OM sources (i.e., also ter-OM) in monitoring programs to better understand the consequences of both eutrophication and oligotrophication processes on coastal ecosystems. Our review strongly urges to include ter-OM, or ter-OM proxies in eutrophication monitoring, and policies to safeguard coastal ecosystem health also under changing climatic conditions and globally increasing anthropogenic perturbations of coastal ecosystems.
Introduction
Eutrophication has remained a major threat to coastal ecosystems globally throughout the past decades and is today acknowledged as a complex process caused by increased inputs of various nutrients (Nixon, 1995; Boesch, 2002; Seitzinger et al., 2010). Under natural conditions, nutrient runoff from land plays a crucial role in fueling the productivity of coastal, and estuarine systems and by providing key ecosystem services (Nixon, 1988; Barbier et al., 2011; Cloern and Jassby, 2012; Cloern et al., 2014). However, excess supply of nutrients may overstimulate internal production of organic matter (autochthonous OM), resulting in reduced water quality and ecosystem health by causing symptoms such as increased growth of macrophytes and harmful algae, as well as a depletion in bottom water oxygen, and periodic fish kills (Nixon, 1995; Schindler, 2006; Diaz and Rosenberg, 2008; Conley et al., 2009).
Historically, the main nutrients within the focus of eutrophication research and policy have been nitrogen (N) and phosphorus (P) (Schindler, 2006). The increased input of these elements, especially their inorganic forms, can be linked directly to anthropogenic activities within the catchment of a waterbody (e.g., agriculture, fertilization, and wastewater) or from deposition of long-range transported air pollutants (e.g., industrial or agricultural emissions) (Nixon, 1995; Diaz and Rosenberg, 2008; Conley et al., 2009). Therefore, reducing inorganic nutrient loads to coastal zones has been the main strategy in national and international policies to reduce eutrophication (Boesch, 2002; Carstensen et al., 2006; Duarte et al., 2009). The resulting reduction in nutrient loading to coastal systems has been termed “oligotrophication,” indicating a recovery from eutrophication (Nixon, 2009).
Besides inorganic nutrients, terrestrial organic matter (ter-OM) is an important component of riverine runoff that has been gaining increasing attention in recent years (Solomon et al., 2015; Ward et al., 2017; Bianchi et al., 2018). Historically, ter-OM was assumed to be stable and relatively resistant to transformations within coastal and estuarine systems (Carlson and Hansell, 2015). However, there was an expressed discrepancy between the high amounts of carbon being transported from land to coasts, compared to the relatively small amounts of terrestrial carbon detectable in oceanic waters and sediments (Hedges et al., 1997; Bianchi, 2011). Today, there is strong evidence that significant transformations of both particulate and dissolved ter-OM fractions occur in coastal and estuarine waters, as well as along their transport downstream (Coble, 2007; Osburn and Bianchi, 2016; Massicotte et al., 2017). These transformational processes are photodegradation, biodegradation and flocculation (causing increased sedimentation), and the relative importance of these processes will vary along the salinity gradient (Weyhenmeyer et al., 2012; Riedel et al., 2016; Massicotte et al., 2017; Bianchi et al., 2018).
Terrestrial organic matter export to coastal ecosystems has been increasing in both boreal (Figures 1A,B; Monteith et al., 2007; de Wit et al., 2016), and arctic regions (Figures 1C,D; Dickson et al., 2000; Peterson et al., 2002; Carmack et al., 2015). Climate drivers such as precipitation changes and increased temperatures are believed to be important for both regions, however, with decreasing sulfate depositions (i.e., acid rain) as an additional driver of ter-OM input in boreal regions (resulting in the often termed “browning” of boreal freshwaters). In addition, structural changes to hydrological cycles (dams, land use), coastal erosion, sea level rise and urbanization (Figures 1G,H), are causing altered (increasing or decreasing) inputs of ter-OM globally, with impacts on ecosystems already under significant stress, such as tropical coral reefs or seagrass meadows (Figures 1E,F; Nyström et al., 2000; Butler et al., 2015; Selvaraj et al., 2015; Regier and Jaffé, 2016).
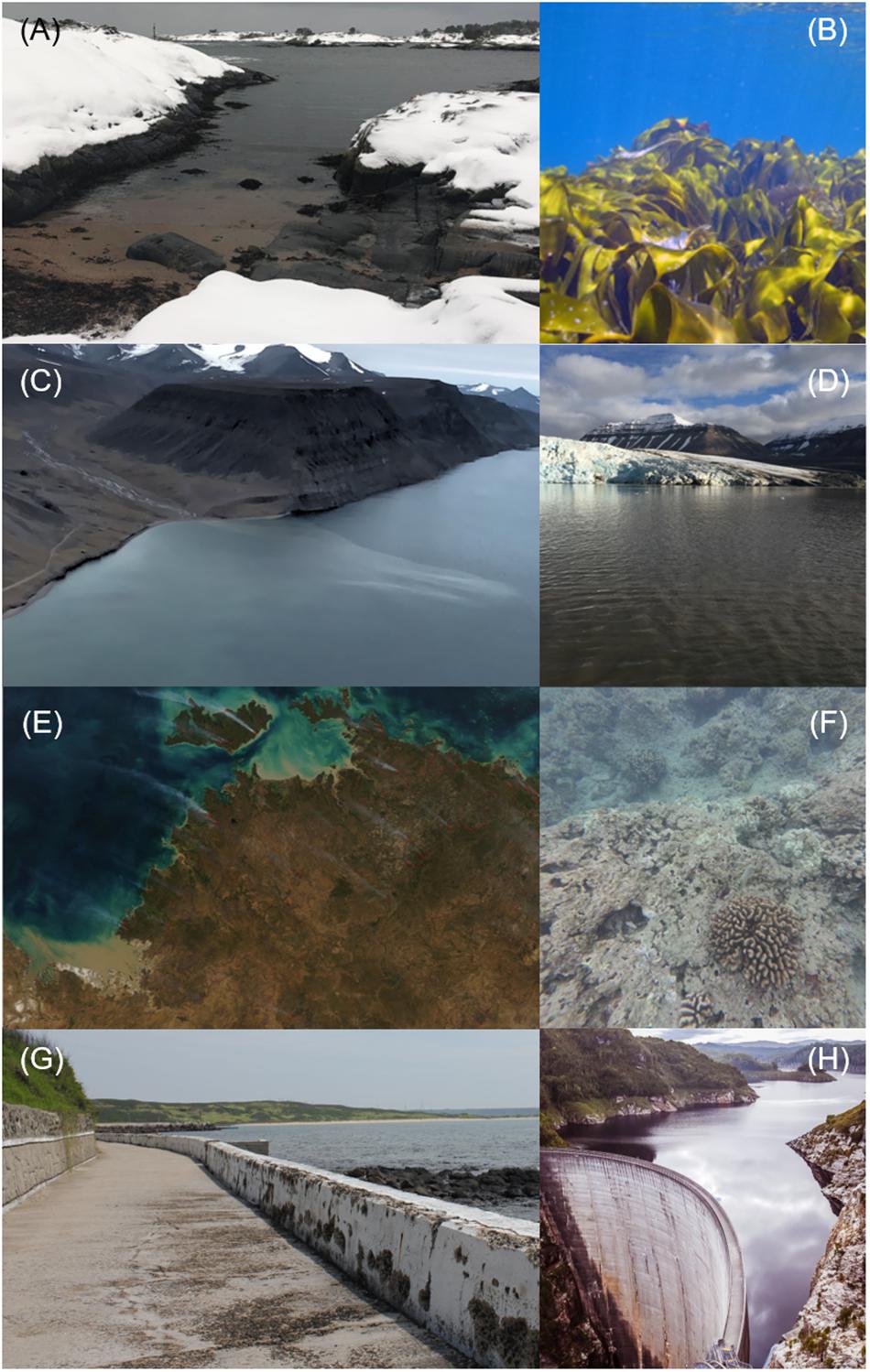
Figure 1. Examples of altered ter-OM loads to coastal ecosystems in (A,B) the boreal zone, due to increased precipitation with potential effects on kelp forests (Skagerrak, Norway), (C,D) the arctic zone, due to thawing permafrost and melting glaciers (Longyearbyen, Spitzbergen), (E,F) tropical zones, due to episodic extreme events with potential effects on e.g., coral reefs (Australia), and (G,H) global coastal zones due to direct human impacts on land-ocean interactions, such as structural modifications of shorelines (Pescadores Islands, Taiwan), or dam construction (Gordon Dam, Australia). Photo courtesy: (A,C,D,F,G) A. Deininger, (B) J. Gitmark, (E) J. Schmaltz (NASA), and (H) L. Lagrainge.
How and to what extent changed input of ter-OM might influence coastal zones simultaneously receiving excess supply of inorganic nutrients (i.e., eutrophication) is currently an unanswered question (Nixon, 2009; Ferreira et al., 2011; Newton et al., 2012), especially since ter-OM or ter-OM proxies are not considered in most monitoring programs or policies aimed to address eutrophication. However, there are indications that increased ter-OM may lead to symptoms typically associated with eutrophication, such as net heterotrophy, anoxic bottom water zones or a decline in macrophyte cover (Andersson et al., 2013). This overlap in symptoms might be problematic, since it may lead to a misdiagnosis of the causes based on the symptoms, especially since information regarding changes in ter-OM input in monitoring programs is often lacking. Duarte et al. (2009) describe how several coastal ecosystems have not returned to original conditions or reference status following nutrient reductions to alleviate eutrophication effects, which the authors attribute to changed baseline conditions of the ecosystems over time. Increased ter-OM inputs to coastal ecosystems will affect baseline conditions, together with other natural, and anthropogenic pressures. It is therefore possible that altered runoff of ter-OM might prevent improvement in ecosystem health of systems undergoing oligotrophication (i.e., decreased inorganic nutrient inputs).
With this review, we aim to reevaluate the role of ter-OM for coastal eutrophication, oligotrophication and ecosystem health. Firstly, we will discuss potential theoretical consequences of altered ter-OM input on coastal ecosystems undergoing eutrophication or oligotrophication, by combining existing knowledge collected within the fields of ter-OM and eutrophication research. Secondly, we will provide case studies where interactions of ter-OM with eutrophication or oligotrophication are already visible, and expected to increase with future human perturbations or climate impacts on these ecosystems. In sum, our paper seeks to improve the knowledge on the interactions between eutrophication and altered ter-OM runoff to coastal ecosystems, and to strengthen the collaboration between these two fields of research. We advice current eutrophication programs and policies to include all OM sources (i.e., also ter-OM) in order to improve our understanding of the interactive effects of human pertubations to coastal ecosystems and to secure coastal ecosystem health also in the face of accelerating human pressures and climate change.
Theoretical Interactions of ter-Om With Eutrophication and Oligotrophication
Terrestrial organic matter may interact with its environment in different ways, depending largely on its chemical and optical properties, but also abiotic (e.g., temperature, salinity), and biotic conditions (e.g., bacterial community composition) (Hedges et al., 1997; Bianchi et al., 2018). When moving from land to sea, ter-OM compounds may undergo substantial modifications along the whole gradient from the upstream catchment to the downstream coastal region (Massicotte et al., 2017). Understanding the different processes involved, as well as the various consequences for ecosystem functioning is currently a vivid field of research (Massicotte et al., 2017; Bianchi et al., 2018; Painter et al., 2018).
Generally, the different ter-OM compounds may be transformed via three main types of reactions: (1) biodegradation, (2) photochemical oxidation (i.e., photodegradation), and (3) flocculation (causing increased sedimentation) (Weyhenmeyer et al., 2012; Riedel et al., 2016; Massicotte et al., 2017; Bianchi et al., 2018). Below, we discuss the various physical and chemical characteristics of the carbon, nutrient and micronutrient fractions.
The chromophoric fraction (CDOM) of the dissolved component of ter-OM, or CDOM, is a major driver of the underwater light climate and photochemistry (Figure 2A; Coble, 2007; Kirk, 2010; Osburn et al., 2016). By absorbing light, CDOM may either cause protection of underwater organisms against harmful ultraviolet (UV) radiation, but also induce light-limitation for aquatic primary producers, causing a decrease in autotrophic production after a certain threshold (Figure 2B; Falkowski and Laroche, 1991; Wikner and Andersson, 2012; Thrane et al., 2014). Additionally, the coastal light environment can also be strongly impacted by larger sized particulate-OM, as well as inorganic particles entering with the terrestrial runoff. These particles can not only cause light absorption, but also shading and scattering, and could potentially have an even stronger impact on the coastal light environment than CDOM, depending on respective concentrations, size and chemical properties (Sholkovitz, 1976; Kirk, 2010; Bainbridge et al., 2018; Margvelashvili et al., 2018). Through its UV-absorption potential, ter-OM may also reduce the amount of light reaching benthic environments and may, depending on depth, result in a shift from benthic to pelagic primary production (Jones, 1992; Fabricius, 2005; Karlsson et al., 2009). Further, shading caused by ter-OM might inhibit growth-stimulating nutrient effects on autotrophs, and lead to an additional advantage for heterotrophs though reduced niche competition (Wikner and Andersson, 2012; Andersson et al., 2013). This resulting release from nutrient competition with autotrophs, may enhance nutrient availability for bacterial production and heterotrophic processes in both pelagic and benthic habitats (Figure 2C; Jones, 1992; Jansson et al., 2003). Decreased oxygen production by autotrophs and increased oxygen demand by heterotrophs (i.e., respiration) might shift coastal ecosystems toward net heterotrophy, with increased release of greenhouse gases (e.g., CO2, N2O) and a potential increase in coastal dead zones (Figure 2D; Wikner and Andersson, 2012; Andersson et al., 2013; Lapierre et al., 2013). Additionally, changes in CDOM might induce shifts in benthic and pelagic community composition toward mixo- and heterotrophic species due to altered baseline light conditions in these environments (Fabricius, 2005; Moy and Christie, 2012; Deininger et al., 2017). Another consequence of a shift from autotrophic to heterotrophic production might be a reduction in food quantity, quality, and food web efficiency to higher trophic levels: bacerial-based food chains are typically lower in essential polyunsaturated fatty acids (PUFAs) important for consumers, as well as longer and therefore less efficient in energy transfer than phytoplankton-based food chains (Nixon, 1988; Müller-Navarra et al., 2000; Berglund et al., 2007). Lastly, reduced light levels caused by higher ter-OM conditions may hinder re-establishment of macrophyte cover, coral survival, or original phytoplankton communities following oligotrophication of coastal ecosystems (Fabricius, 2005; Karlsson et al., 2009; Filbee-Dexter and Wernberg, 2018).
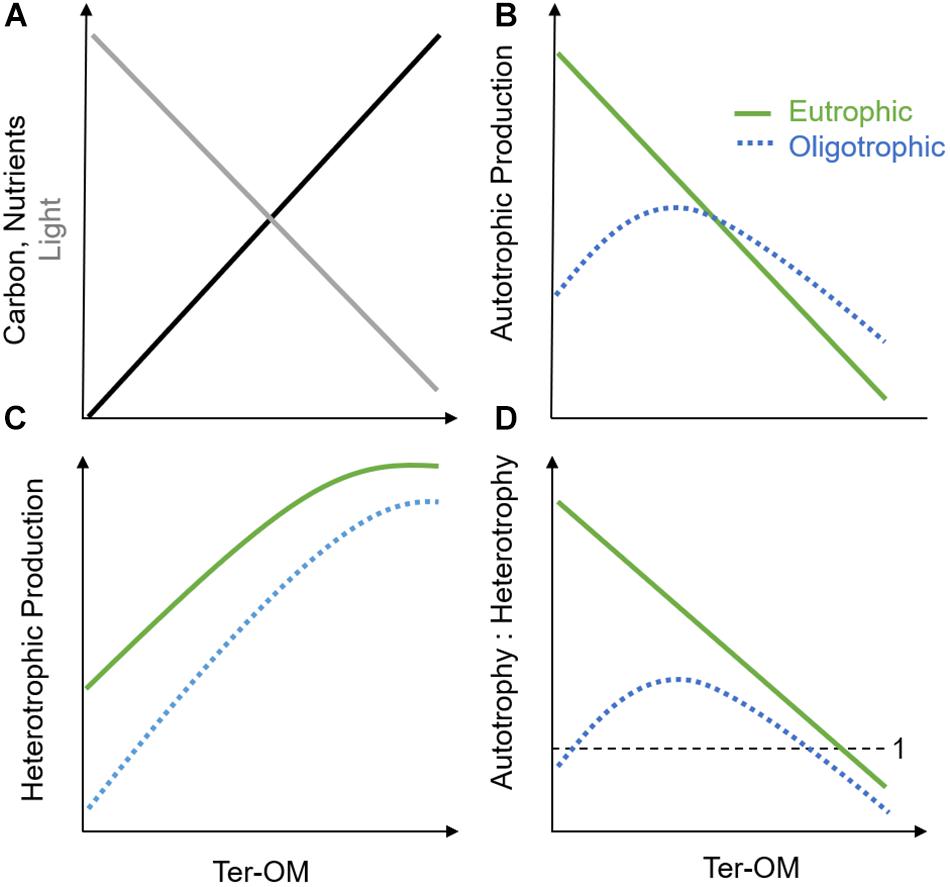
Figure 2. Simplified conceptual framework illustrating the influence of increasing ter-OM on (A) baseline light (gray), nutrient, and carbon availability (black) in aquatic systems and hypothesized effects on (B) autotrophic production, (C) heterotrophic production, and (D) autotrophic vs. heterotrophic production in oligotrophic (blue, dotted line) and eutrophic (green, solid line) systems.
The carbon compounds of ter-OM (i.e., dissolved organic carbon, DOC) may serve as a major energy source for hetero- and mixotrophic producers and stimulate heterotrophic production, until other factors will further limit growth (e.g., temperature, predation) (Figures 2A,C; Stepanauskas et al., 2002; Berglund et al., 2007). However, the bioavailability of DOC has been shown to strongly depend on molecule size and molecule structure of the carbon component, as well as the present bacterial community composition (Kamjunke et al., 2017; Creed et al., 2018). Nevertheless, it is likely that heterotrophic production in general will be stimulated by ter-OM inputs, supporting higher levels of production at high nutrient inputs (i.e., Figure 2C, eutrophic scenario), than at relatively lower nutrient inputs (Figure 2C, oligotrophic scenario). Further, this response will depend on the initial trophic state of the system and the duration of the nutrient pulses (hours, days, weeks), with different functional groups dominating depending on their adaptation strategies (Berglund et al., 2007; Deininger et al., 2016, 2017).
Besides the carbon compounds, ter-OM also contains complexed elements, such as macronutrients, micronutrients, and contaminants. The macronutrient compounds of ter-OM consist mainly of N and P (i.e., organic N, P; Figure 2A). Also for these ter-DOM compounds, bioavailability may be highly variable irrespective of total concentrations (i.e., TN, TP), making measurements of TN and TP potentially poor indicators for predicting productivity responses (Bergström, 2010; Berggren et al., 2015; Soares et al., 2017). However, in contrast to carbon compounds, macronutrients can support auto-, mixo- and heterotrophic processes, depending on bioavailability, competition between species, as well as other abiotic conditions such as light availability (Figures 2B–D; Berggren et al., 2015; Soares et al., 2017). Due to these multiple interactions, it is difficult to predict, which productivity processes organic N and P components might ultimately influence. Nutrient effects might strongly depend on initial baseline nutrient conditions: In oligotrophic and ter-OM poor systems, it has been hypothesized that increasing ter-OM concentrations might initially increase autotrophic production until systems eventually become increasingly light-limited (e.g., ∼5 mg L−1 in boreal and arctic lakes) (Figure 2B, oligotrophic scenario) (Seekell et al., 2015). A similar response might not be expected in eutrophic systems, where ter-OM-attributed nutrient effects might comparably be diluted in the overall high-nutrient baseline conditions. Additionally, self-shading effects might occur through increased autochthonous OM production (i.e., phytoplankton blooms), which can substantially decrease light availability (Figure 2B, eutrophic scenario), with increasing habitat suitability for heterotrophs (Figures 2C,D). Also micronutrient compounds, and especially iron (Fe), may strongly stimulate coastal basal production, especially heterotrophic- and N-fixing bacteria, which have been shown to be more efficient in accessing complexed Fe than autotrophs (Hyenstrand et al., 2000; Sorichetti et al., 2014, 2016). In coastal zones, the concentration of Fe is comparably higher than in open oceans due to the large shelf sources (Johnson et al., 1997, 1999). Fe-DOM complexes are photoreactive, however given its strong positive charge, Fe is typically strongly bound, and precipitates fast when dissociated from DOM.
Lastly, ter-OM may also affect contaminant dynamics in coastal zones, as many contaminants are also positively charged, such as mercury (Hg), either to its inorganic [Hg(II)] or its organic form (methyl-Hg) (Haitzer et al., 2003; Ravichandran, 2004). The latter is a highly potent neurotoxin, and biomagnifies in aquatic food webs (Morel et al., 1998; Ullrich et al., 2001; Schartup et al., 2017). Thus, increased inflow of ter-OM might ultimately result in increased inflow of attributed toxic contaminants, such as methyl-Hg, threatening both ecosystem health and water quality. Interaction effects with eutro- or oligotrophication are currently unclear but might also depend largely on the ter-OM-induced shifts from autotrophic toward heterotrophic production, which might increase the direct or indirect consumption of ter-OM by aquatic consumers, and therefore the attributed methyl-Hg uptake (Karlsson et al., 2012; Berggren et al., 2015; Schartup et al., 2017).
In summary, the effect of changing inorganic nutrient supply (i.e., eutro-, oligotrophication) on coastal ecosystems might systematically be altered by shifted baseline conditions caused by changes in ter-OM input. Ter-OM may alter light quantity and quality for autotrophic producers through its chromophoric compounds (Figures 2A,B), stimulate growth of different producers and consumers via its carbon, macronutrient (N, P) (Figures 2A–D) and micronutrient (mainly iron, Fe) fractions, and/or induce toxicity through its contaminant compounds (e.g., mercury) (Stepanauskas et al., 2002; Fabricius, 2005; Creed et al., 2018). Importantly, to understand the interaction of ter-OM with its surrounding environment (and eutro-, oligotrophication), both chemical and optical properties, but also the reactivity of the material has to be considered (Hedges et al., 1997; Osburn and Bianchi, 2016; Bianchi et al., 2018).
Case Studies of Altered ter-Om Loads to Coastal Ecosystems
To date, most mechanistic understanding related to ter-OM effects on aquatic ecosystems comes from systems where aquatic ter-OM concentrations are amongst the highest globally, namely boreal freshwaters and the Baltic Sea (Sobek et al., 2007; Kirk, 2010). Here, ter-OM has been recognized for decades as a fundamental driver of aquatic ecosystem productivity, ecosystem structure, functioning, and greenhouse gas emission through its light-absorbing, nutrient- and carbon-providing properties (i.e., Figure 2; Solomon et al., 2015; Andersson et al., 2018; Creed et al., 2018). However, there is increasing evidence that there are similar processes involved for ter-OM processing and cycling when entering systems with comparably lower concentrations than above, such as the Amazon or the Mississippi river to ocean continuum, the Great Barrier reef, and the Arctic Ocean (Figure 1) (Fabricius, 2005; Seidel et al., 2015; Duan et al., 2017; Tanski et al., 2017). Below, we will present case studies from ecosystems where increased ter-OM has already changed biotic and abiotic conditions (e.g., Baltic Sea), to ecosystems where ter-OM has recently started to increase (boreal, arctic, tropical, and global patterns), to cases where ter-OM input is abrupt, extreme, short-term and predicted to increase in frequency (globally, episodic events).
Pilot Case: Baltic Sea
The Baltic sea is the world’s largest semi-enclosed sea. This setting might be the reason why eutrophication has drastically affected its ecosystem health, making the Baltic a pilot study for both research and implementing policies on eutrophication (Larsson et al., 1985; Heisler et al., 2008; Andersen et al., 2011; Paasche et al., 2015). In addition, the nature of the catchment (i.e., high amounts of peatland), as well as long water residence times might be additional contributing factors to why runoff of ter-OM has already led to comparably high ter-OM concentrations and ecosystem effects compared to other coastal ecosystems with higher water mass exchange (Søndergaard and Thomas, 2004; Andersson et al., 2013). Recently, interactions between eutrophication and ter-OM in the Baltic have received increasing attention (Wikner and Andersson, 2012; Andersson et al., 2013). In a mesocosm experiment, Andersson et al. (2013) showed that additions of inorganic nutrients alone resulted in typical eutrophication symptoms, whereas additional supply of humic carbon resulted in increased bacterial production and increased net heterotrophy (Figures 2C,D). This agrees with an analysis of long-term monitoring data of the northern Baltic Sea, showing that increased riverine discharge over a 40-year period correlated with increased bacterial production and net heterotrophy (Wikner and Andersson, 2012). However, the trend in heterotrophy was not only driven by increased bacterial production, but largely also (71%) by induced light limitation, and a reduction in phytoplankton productivity (Figure 2B). These findings indicate that ter-OM plays an important role as a regulator of coastal productivity, but more studies are needed to confirm these observed patterns both at high- (e.g., northern Baltic) and lower ter-OM (southern Baltic) concentrations.
Other Boreal Cases
As a result of the increased ter-OM input and consequent browning of boreal freshwaters, most cases of increased downstream ter-OM runoff to coastal zones are reported for the boreal zone. Besides the Baltic, other European cases of potentially increased inputs of ter-OM have been reported for the Norwegian coast, e.g., the “coastal darkening” of the Skagerrak and the North Sea (Figure 1A; Aksnes et al., 2009; Dupont and Aksnes, 2013; Frigstad et al., 2013). Here, increases in ter-OM are hypothesized to be connected both to increased discharge of rivers flowing into the Skagerrak (i.e., increasing the loads of OM) (Skarbøvik et al., 2017), but also an increase in the concentrations of the various components of OM per se (de Wit et al., 2016). For example, Frigstad et al. (2013) found a decrease in the inorganic nutrient fraction for the coastal Skagerrak area (southern Norway), while there was an increase in the dissolved and particulate organic fractions of both carbon and nutrients, believed to be related to increased loading of ter-OM (Figure 2A). In the same area, a large-scale shift from sugar kelp (Saccharina latissima, Figure 1B) to ephemeral algae occurred around 2002 (Moy and Christie, 2012), connected with high summer temperatures and siltation from river runoff. Over the same time-period, the soft-bottom fauna in Skagerrak has shown improved status believed to be related to an improvement in eutrophication status (i.e., oligotrophication) (Trannum et al., 2018). However, to be able to disentangle the effects of eutrophication, oligotrophication and increased ter-OM in this region, it is important to have consistent ecosystem monitoring that also includes measurements relevant for detecting changes in ter-OM, such as DOC, CDOM, and light.
Arctic- and Subarctic Cases
Increases in ter-OM have been reported for many coastal shelf areas of the Arctic (Dickson et al., 2000; Peterson et al., 2002; ACIA, 2004; Kaiser et al., 2017). In this region, increased precipitation driven by climate change, and thawing of permafrost and glaciers have already resulted in increased riverine runoff of ter-OM to arctic and subarctic coasts across Scandinavia, Russia, and North America (Figures 1C,D; Dickson et al., 2000; Peterson et al., 2002; ACIA, 2004; Kaiser et al., 2017). These trends are likely to continue, given the predicted increases in both precipitation and temperature, further inducing permafrost thawing, and ter-OM runoff (Froese et al., 2008; Schuur et al., 2008; Carmack et al., 2016). Upstream arctic and subarctic catchments harbor up to 50% of the worlds soil carbon, wherefore increased runoff will potentially have large consequences for carbon stocks and cycling (Gorham, 1991; Waelbroeck et al., 1997; Kaiser et al., 2017). Thus, runoff from both permafrost and glaciers can be expected to have large consequences for downstream coastal ecosystems and ecosystem baseline conditions (Figures 2B–D; Dunton et al., 2012; Lydersen et al., 2014; Kaiser et al., 2017). Although eutrophication is not a major problem in the Arctic, including ter-OM measurements in current monitoring programs and research expeditions will greatly benefit basic research around the interactions effects of ter-OM and eutrophication, since arctic shelf seas may serve as unique reference systems and provide important insights into current and potential future drivers of primary production (Mann et al., 2016). Additionally, studying ter-OM in the Arctic will greatly improve current understanding behind the triggers of ter-OM runoff, but also interactions with e.g., coastal freshening and the importance of ter-OM quality and source (e.g., boreal soils vs. glacier runoff) (Blair and Aller, 2012).
Tropical Cases
Human activities have been causing large changes to land-ocean interactions in tropical regions by affecting upstream catchments properties (details see section “Global Cases Caused by Structural Modifications”), altering floodplain structures and inundation patterns (e.g., for rice paddies, shrimp farms), as well as by potentially affecting weather patterns leading to an increasing frequency of extreme precipitation events (i.e., hurricanes and cyclones causing episodic input of ter-OM) (Nyström et al., 2000; Butler et al., 2015; Selvaraj et al., 2015; Regier and Jaffé, 2016). Generally, tropical land-ocean transects are important hot-spots for biogeochemical ter-OM cycling due to several reasons: their comparably high temperatures, their seasonal inundations over large areas, and their diversity in catchment characteristics, ranging from tropical forests to dryland and from pristine areas to heavily human perturbed areas (dams, river withdraw, and channel alterations) (Burns et al., 2008; Hamilton, 2010; Seidel et al., 2015). Much is still unclear about the ecological equilibrium within these diverse systems, especially within benthic environments, such as coral reefs or macrophyte meadows which are currently decreasing globally (Nyström et al., 2000; Comte and Pendleton, 2018; Filbee-Dexter and Wernberg, 2018). Findings management strategies for these threatened ecosystems is under strong investigation (Pittman and Armitage, 2016; Regier and Jaffé, 2016; Comte and Pendleton, 2018). For example, recent studies from Australia have indicated that increased input of ter-OM following episodic rain events and attributed increases in nutrient concentrations, turbidity, and sedimentation may strongly change baseline conditions important for the growth, survival, but also reproduction and recruitment of corals, and organisms living within coral associated habitats (Figures 1E,F; Nyström et al., 2000; Fabricius, 2005; Regier and Jaffé, 2016). However, the current state of knowledge does not allow to identify the specific drivers influencing tropical coastal ecosystems, largely due to missing monitoring data of comparable parameters along the land-ocean continuum, but also the complexity of processes along the gradient (Burns et al., 2008; Hamilton, 2010; Seidel et al., 2015; Regier and Jaffé, 2016). Similarly, it is difficult to predict the effects of increased temperature due to global warming on these highly dynamic ecosystem processes (Hamilton, 2010). However, including ter-OM or ter-OM proxies, in addition to eutrophication proxies, in the monitoring of tropical coastal waters would aid in understanding these hotspots, but also hot moments (i.e., episodic events) of ter-OM input and cycling in these rapidly changing ecosystems (Hamilton, 2010; Seidel et al., 2015).
Global Cases Caused by Structural Modifications
Terrestrial organic matter input may also be systematically altered directly through human activities. For example, decreased river runoff may in some cases be directly attributed to anthropogenic activities within the river catchment. These activities may be the building of dams and reservoirs, but also land use changes (agriculture, forestry), urbanization, structural changes to shoreline or the extraction of water for irrigation purposes (Figures 1G,H) (Milliman et al., 2008; García-Ruiz et al., 2011; Su et al., 2018). Within mid-latitude rivers for example, river runoff has decreased by ∼60% during the last half of the 20th century (Milliman et al., 2008). As discussed above, changing upstream runoff has large consequences for downstream ecosystems, by changing the transport, transformation, and delivery of material (both ter-OM and inorganic nutrients) into coastal zones (Seitzinger et al., 2010). Given the ongoing and predicted demographic pressures in many water-scarce regions of the world (Africa, Asia, Australia, Mediterranean, southern Mexico, and northern Brazil), the decrease in river runoff is likely to accelerate and deserve careful monitoring of downstream ecosystems (Milliman et al., 2008; García-Ruiz et al., 2011; Donnelly et al., 2017).
Furthermore, coastal erosion is increasing globally, and is currently impacting at least 75% of the world’s shorelines (Rangel-Buitrago et al., 2018b). Generally, erosion from landmasses (both ter-OM and inorganic material) into the ocean is a natural phenomenon that can occur both as a slow pervasive process, but also rapidly during extreme events (Larson and Kraus, 1995; Rangel-Buitrago et al., 2018b). However, the ongoing and predicted human urbanization of coastal zones, as well as the various anthropogenic effects on the climate (temperature, precipitation, and storm events) increases the rates of coastal erosion, with large ecological, economic, and societal impacts (Pranzini, 2018; Rangel-Buitrago et al., 2018a; Williams et al., 2018). The ongoing increase in sea level is also a significant driver of coastal erosion (Toimil et al., 2017; Rangel-Buitrago et al., 2018a).
Currently, none of the above-mentioned human impacts on ter-OM transport to coastal zones have been discussed in relation to coastal eutrophication or oligotrophication, especially not along the aquatic continuum. However, filling these knowledge gaps is essential for understanding and predicting the future state and baseline conditions of global coastal zones (Newton et al., 2012; Hyndes et al., 2014; Ward et al., 2017).
Episodic Weather Events
Lastly, climate-driven changes in precipitation can strongly influence runoff patterns from land to sea (Milliman et al., 2008; Donnelly et al., 2017; Su et al., 2018). According to climate change scenarios, the frequency and intensity of extreme, episodic weather events is going to increase in various regions around the globe (Milly et al., 2002; Sánchez et al., 2004; Huntington, 2006; Kyselı et al., 2012). Increased storm runoff will potentially have similar effects on coastal ecosystems as the cases discussed above and will strongly depend on ter-OM quality and quantity. Thus, while the carbon fraction may serve as an additional energy source for bacteria, nutrients will, depending on light and trophic conditions, additionally support primary producers (Figures 2B–D) (Guadayol et al., 2009; Bec et al., 2011; Pecqueur et al., 2011; Liess et al., 2016). Since storm runoff events occur globally, including ter-OM in coastal eutrophication monitoring is not only crucial for the boreal, arctic or tropical zones, but in general.
Summary, Outlook, and Recommendations for Management
The theoretical interactions and case studies described above illustrate that there are considerable complexities in the interactions between altered ter-OM inputs and inorganic nutrient loadings, making it currently challenging to understand and predict overall ecological responses, and effects of these stressors on ecosystem health. In Figure 2, we suggest a simplified conceptual framework to summarize how increasing ter-OM may affect coastal systems under high inorganic nutrient, and low inorganic nutrient conditions (i.e., eutrophic and oligotrophic scenarios).
In general, ter-OM may change light quality and quantity through its CDOM and shading/scattering caused by particles and inorganic material (Figure 2A, gray line), while ter-OM will also increase the carbon- and nutrient availability in receiving waters, with the ecological response depending on the bioavailability of the various ter-OM components, but also ecosystem community compositions (Figure 2A, black line). We predict an overall negative effect on autotrophic production of increasing ter-OM (Figure 2B). However, in oligotrophic systems, the nutrient fractions within ter-OM would initially act to increase production up to a certain threshold value, after which light-limitation would lead to decreasing autotrophic production. This threshold value would be dependent on the composition of the ter-OM, and specifically the light attenuation of the CDOM fraction. With increasing eutrophication of the system, we hypothesize that this nutrient effect of ter-OM would decrease, and eventually vanish, with light limitation being the main driver for the reduction in autotrophic productivity. With the reduction in light quantity and quality along the gradient of increasing ter-OM, a decrease in benthic: pelagic productivity can be hypothesized. For heterotrophs (Figure 2C), we predict an overall positive effect of increasing ter-OM on productivity for both nutrient scenarios (i.e., oligo- and eutrophic scenarios), until a saturation threshold is reached. At nutrient saturation, any additional nutrients and carbon will not lead to higher productivity and instead other factors will be limiting growth of heterotrophs (e.g., temperature, predation etc.). In sum, the overall effect on the metabolic balance (i.e., autotrophic: heterotrophic production) of increasing ter-OM would be a shift toward net heterotrophy, by causing a decrease in autotrophic processes and a simultaneous increase in heterotrophic respiration. By its influence on the metabolic balance of coastal ecosystems (i.e., net heterotrophy), changes in ter-OM input could have implications for the global carbon budget of coastal ecosystems, causing increased release of greenhouse gases (e.g., CO2, CH4) and a potential increase in coastal dead zones (Wikner and Andersson, 2012; Andersson et al., 2013; Lapierre et al., 2013), which deserves increased attention.
This developed conceptual framework, describing interactions between ter-OM and eutrophication or oligotrophication processes, remains to be challenged in future studies by conducting e.g., full-factorial experiments, using long-term monitoring data and performing meta-analysis of global case studies in different global coastal ecosystems, but also along the land-ocean continuum and by changing abiotic conditions (e.g., salinity, temperature). Such studies will greatly improve our understanding of baseline shifts within coastal ecosystems and help to determine thresholds where shifts could be expected, for example for light-limitation of autotrophs or transitions from net autotrophy to heterotrophy in different coastal systems.
In addition, there is a need for methodological harmonization between the different research areas and along salinity-gradients (i.e., catchment, freshwater, coastal, and marine). Although the case studies described above all deal with changes in ter-OM and nutrient input, they have rarely been put into context with each other. This might be due to the different drivers between systems (e.g., precipitation, reduced sulfate deposition, and structural changes in hydrological cycles), but also the situation in different regions (arctic, boreal, and tropical), and the different methodologies used. Future harmonization and improvements in methodology will strongly improve and aid the realization of comparative studies. To date, ter-OM is operationally classified by its dissolved organic carbon (DOC) content, where DOC is defined by its size (i.e., liquid that passes through a 0.45 μm filter in most, but also 0.2 or 0.7 μm filter in some cases) (Creed et al., 2018). However, there is currently a rapid development in methods for improving the characterization and determination of ter-OM, and CDOM, which should aid comparisons between regions and studies in the future (Bravo et al., 2018; Wünsch et al., 2018).
Our review shows that despite the growing recognition of the importance of ter-OM for aquatic productivity (Fabricius, 2005; Solomon et al., 2015; Creed et al., 2018), there is still significant improvements to be made in incorporating this knowledge into both research and policy efforts on eutrophication. Even though alternate sources of OM have been discussed as contributing to eutrophication for decades (Nixon, 1995; Nixon, 2009), the focus of most policies and monitoring programs is still connected to the classical “nutrient-enrichment” understanding of the eutrophication problem (e.g., in the European water framework directive, WFD). We argue that the similarity in effects between eutrophication and ter-OM (reduced light availability, net heterotrophy, anoxic bottom water zones, and decrease in macrophyte cover) make it challenging for both researchers and policy makers to properly determine the correct cause-effects relationships. A potential misdiagnosis hinders our understanding of the complex responses observed in coastal ecosystems, and more worryingly could be interpreted as that current environmental policies to reduce inorganic nutrient loadings are inefficient in improving eutrophication status and ultimately ecosystem health.
Nixon (2009) argued for the need of “macroscopes” when understanding and managing eutrophication, including effects of climate change, and changes in the total supply of organic matter (i.e., both autochthonous and ter-OM) to the system. Andersen et al. (2006) argued that measurements of primary production should be mandatory when monitoring eutrophication. We echo these recommendations, and specifically also advice to include measurements of ter-OM or ter-OM proxies into eutrophication monitoring programs, such as DOC, CDOM, and light (including spectral composition). We believe this is timely, given both the number of cases showing a failure of reducing inorganic nutrient loadings for improving environmental conditions and ecosystem health in many coastal areas (Duarte et al., 2009), and the improving methodology for determining the quality and quantity of ter-OM (Mann et al., 2016; Osburn and Bianchi, 2016; Bianchi et al., 2018; Creed et al., 2018). Adding measurements of ter-OM in coastal monitoring programs would reduce uncertainty in predicting future states and baseline shifts of coastal ecosystems in response to both eutrophication and oligotrophication. This is important, since ignoring interactions between ter-OM and eutrophication and oligotrophication might slow down future efforts to secure coastal ecosystem health.
Author Contributions
Both authors wrote the manuscript, contributed to revision, and approved the submitted version.
Conflict of Interest Statement
The authors declare that the research was conducted in the absence of any commercial or financial relationships that could be construed as a potential conflict of interest.
Acknowledgments
We thank the strategic group on Land Ocean Interactions at the Norwegian Institute of Water Research (NIVA) for providing a fruitful context for us and specifically Ø. Kaste, A. Poste, and A. L. King, as well as the reviewer for their valuable comments on the earlier version of this manuscript. Further, we are grateful to the marine section at NIVA that supported us to attend the 4th International Symposium on Research and Management of Eutrophication in Coastal Ecosystems (EUTRO 2018) that has resulted in this work.
References
ACIA (ed.). (2004). “Impacts of a warming Arctic-Arctic climate impact assessment,” in Impacts of a Warming Arctic-Arctic Climate Impact Assessment, (Cambridge: Cambridge University Press), 144.
Aksnes, D. L., Dupont, N., Staby, A., Fiksen, O., Kaartvedt, S., and Aure, J. (2009). Coastal water darkening and implications for mesopelagic regime shifts in Norwegian fjords. Mar. Ecol. Prog. Ser. 387, 39–49. doi: 10.3354/meps08120
Andersen, J. H., Axe, P., Backer, H., Carstensen, J., Claussen, U., Fleming-Lehtinen, V., et al. (2011). Getting the measure of eutrophication in the Baltic Sea: towards improved assessment principles and methods. Biogeochemistry 106, 137–156. doi: 10.1007/s10533-010-9508-4
Andersen, J. H., Schlüter, L., and Ærtebjerg, G. (2006). Coastal eutrophication: recent developments in definitions and implications for monitoring strategies. J. Plankton Res. 28, 621–628. doi: 10.1093/plankt/fbl001
Andersson, A., Brugel, S., Paczkowska, J., Rowe, O. F., Figueroa, D., Kratzer, S., et al. (2018). Influence of allochthonous dissolved organic matter on pelagic basal production in a northerly estuary. Estuar. Coast. Shelf Sci. 2014, 225–235. doi: 10.1016/j.ecss.2018.02.032
Andersson, A., Jurgensone, I., Rowe, O. F., Simonelli, P., Bignert, A., Lundberg, E., et al. (2013). Can humic water discharge counteract eutrophication in coastal waters? PLoS One 8:e61293. doi: 10.1371/journal.pone.0061293
Bainbridge, Z., Lewis, S., Bartley, R., Fabricius, K., Collier, C., Waterhouse, J., et al. (2018). Fine sediment and particulate organic matter: a review and case study on ridge-to-reef transport, transformations, fates, and impacts on marine ecosystems. Mar. Pollut. Bull. 135, 1205–1220. doi: 10.1016/j.marpolbul.2018.08.002
Barbier, E. B., Hacker, S. D., Kennedy, C., Koch, E. W., Stier, A. C., and Silliman, B. R. (2011). The value of estuarine and coastal ecosystem services. Ecol. Monogr. 81, 169–193.
Bec, B., Collos, Y., Souchu, P., Vaquer, A., Lautier, J., Fiandrino, A., et al. (2011). Distribution of picophytoplankton and nanophytoplankton along an anthropogenic eutrophication gradient in French Mediterranean coastal lagoons. Aquat. Microb. Ecol. 63, 29–45. doi: 10.3354/ame01480
Berggren, M., Bergström, A.-K., and Karlsson, J. (2015). Intraspecific autochthonous and allochthonous resource use by zooplankton in a humic lake during the transitions between winter, summer and fall. PLoS One 10:e0120575. doi: 10.1371/journal.pone.0120575
Berglund, J., Muren, U., Båmstedt, U., and Andersson, A. (2007). Efficiency of a phytoplankton-based and a bacteria-based food web in a pelagic marine system. Limnol. Oceanogr. 52, 121–131. doi: 10.4319/lo.2007.52.1.0121
Bergström, A. K. (2010). The use of TN:TP and DIN:TP ratios as indicators for phytoplankton nutrient limitation in oligotrophic lakes affected by N deposition. Aquat. Sci. 72, 277–281. doi: 10.1007/s00027-010-0132-0
Bianchi, T. S. (2011). The role of terrestrially derived organic carbon in the coastal ocean: a changing paradigm and the priming effect. Proc. Natl. Acad. Sci. U.S.A. 108, 19473–19481. doi: 10.1073/pnas.1017982108
Bianchi, T. S., Cui, X., Blair, N. E., Burdige, D. J., Eglinton, T. I., and Galy, V. (2018). Centers of organic carbon burial and oxidation at the land-ocean interface. Org. Geochem. 115, 138–155. doi: 10.1016/j.orggeochem.2017.09.008
Blair, N. E., and Aller, R. C. (2012). The fate of terrestrial organic carbon in the marine environment. Annu. Rev. Mar. Sci. 4, 401–423. doi: 10.1146/annurev-marine-120709-142717
Boesch, D. F. (2002). Challenges and opportunities for science in reducing nutrient over-enrichment of coastal ecosystems. Estuaries 25, 886–900. doi: 10.1007/bf02804914
Bravo, A. G., Kothawala, D. N., Attermeyer, K., Tessier, E., Bodmer, P., Ledesma, J. L. J., et al. (2018). The interplay between total mercury, methylmercury and dissolved organic matter in fluvial systems: a latitudinal study across Europe. Water Res. 144, 172–182. doi: 10.1016/j.watres.2018.06.064
Burns, K. A., Brunskill, G., Brinkman, D., and Zagorskis, I. (2008). Organic carbon and nutrient fluxes to the coastal zone from the Sepik River outflow. Cont. Shelf Res. 28, 283–301. doi: 10.1016/j.csr.2007.08.004
Butler, I. R., Sommer, B., Zann, M., Zhao, J. X., and Pandolfi, J. M. (2015). The cumulative impacts of repeated heavy rainfall, flooding and altered Water quality on the high-latitude coral reefs of Hervey Bay, Queensland, Australia. Mar. Pollut. Bull. 96, 356–367. doi: 10.1016/j.marpolbul.2015.04.047
Carlson, C. A., and Hansell, D. A. (2015). “DOM sources, sinks, reactivity, and budgets,” in Biogeochemistry of Marine Dissolved Organic Matter, eds D. A. Hansell and C. A. Carlson (Amsterdam: Elsevier), 65–126. doi: 10.1016/b978-0-12-405940-5.00003-0
Carmack, E., Winsor, P., and Williams, W. (2015). The contiguous panarctic Riverine coastal domain: a unifying concept. Prog. Oceanogr. 139, 13–23. doi: 10.1016/j.pocean.2015.07.014
Carmack, E. C., Yamamoto-Kawai, M., Haine, T. W. N., Bacon, S., Bluhm, B. A., Lique, C., et al. (2016). Freshwater and its role in the Arctic Marine System: sources, disposition, storage, export, and physical and biogeochemical consequences in the Arctic and global oceans. J. Geophys. Res. Biogeosci. 121, 675–717. doi: 10.1002/2015jg003140
Carstensen, J., Conley, D. J., Andersen, J. H., and Ærtebjerg, G. (2006). Coastal eutrophication and trend reversal: a Danish case study. Limnol. Oceanogr. 51, 398–408. doi: 10.4319/lo.2006.51.1_part_2.0398
Cloern, J. E., Foster, S., and Kleckner, A. (2014). Phytoplankton primary production in the world’s estuarine-coastal ecosystems. Biogeosciences 11, 2477–2501. doi: 10.5194/bg-11-2477-2014
Cloern, J. E., and Jassby, A. D. (2012). Drivers of change in estuarine-coastal ecosystems: discoveries from four decades of study in San Francisco Bay. Rev. Geophys. 50:33.
Coble, P. G. (2007). Marine optical biogeochemistry: the chemistry of ocean color. Chem. Rev. 107, 402–418. doi: 10.1021/cr050350
Comte, A., and Pendleton, L. H. (2018). Management strategies for coral reefs and people under global environmental change: 25 years of scientific research. J. Environ. Manage. 209, 462–474. doi: 10.1016/j.jenvman.2017.12.051
Conley, D. J., Paerl, H. W., Howarth, R. W., Boesch, D. F., Seitzinger, S. P., Havens, K. E., et al. (2009). Controlling eutrophication: nitrogen and phosphorus. Science 323, 1014–1015.
Creed, I. F., Bergström, A.-K., Trick, C. G., Grimm, N. B., Hessen, D. O., Karlsson, J., et al. (2018). Global change-driven effects on dissolved organic matter composition: implications for food webs of northern lakes. Glob. Change Biol. 24, 3692–3714. doi: 10.1111/gcb.14129
de Wit, H. A., Valinia, S., Weyhenmeyer, G. A., Futter, M. N., Kortelainen, P., Austnes, K., et al. (2016). Current browning of surface waters will be further promoted by wetter climate. Environ. Sci. Technol. Lett. 3, 430–435. doi: 10.1021/acs.estlett.6b00396
Deininger, A., Faithfull, C. L., and Bergström, A. K. (2017). Phytoplankton response to whole lake inorganic N fertilization along a gradient in dissolved organic carbon. Ecology 98, 982–994. doi: 10.1002/ecy.1758
Deininger, A., Faithfull, C. L., Lange, K., Bayer, T., Vidussi, F., and Liess, A. (2016). Simulated terrestrial runoff triggered a phytoplankton succession and changed seston stoichiometry in coastal lagoon mesocosms. Mar. Environ. Res. 119, 40–50. doi: 10.1016/j.marenvres.2016.05.001
Diaz, R. J., and Rosenberg, R. (2008). Spreading dead zones and consequences for marine ecosystems. Science 321, 926–929. doi: 10.1126/science.1156401
Dickson, R. R., Osborn, T. J., Hurrell, J. W., Meincke, J., Blindheim, J., Adlandsvik, B., et al. (2000). The arctic ocean response to the North Atlantic oscillation. J. Clim. 13, 2671–2696.
Donnelly, C., Greuell, W., Andersson, J., Gerten, D., Pisacane, G., Roudier, P., et al. (2017). Impacts of climate change on European hydrology at 1.5, 2 and 3 degrees mean global warming above preindustrial level. Clim. Change 143, 13–26. doi: 10.1007/s10584-017-1971-7
Duan, S., He, Y., Kaushal, S. S., Bianchi, T. S., Ward, N. D., and Guo, L. (2017). Impact of wetland decline on decreasing dissolved organic carbon concentrations along the Mississippi river continuum. Front. Mar. Sci. 3:280. doi: 10.3389/fmars.2016.00280
Duarte, C. M., Conley, D. J., Carstensen, J., and Sánchez-Camacho, M. (2009). Return to neverland: shifting baselines affect eutrophication restoration targets. Estuaries Coast. 32, 29–36. doi: 10.1007/s12237-008-9111-2
Dunton, K. H., Schonberg, S. V., and Cooper, L. W. (2012). Food web structure of the Alaskan nearshore shelf and estuarine lagoons of the Beaufort Sea. Estuaries Coast. 35, 416–435. doi: 10.1007/s12237-012-9475-1
Dupont, N., and Aksnes, D. L. (2013). Centennial changes in water clarity of the Baltic Sea and the North Sea. Estuar. Coast. Shelf Sci. 131, 282–289. doi: 10.1016/j.ecss.2013.08.010
Fabricius, K. E. (2005). Effects of terrestrial runoff on the ecology of corals and coral reefs: review and synthesis. Mar. Pollut. Bull. 50, 125–146. doi: 10.1016/j.marpolbul.2004.11.028
Falkowski, P. G., and Laroche, J. (1991). Acclimation to spectral irradiance in algae. J. Phycol. 27, 8–14. doi: 10.1111/j.0022-3646.1991.00008.x
Ferreira, J. G., Andersen, J. H., Borja, A., Bricker, S. B., Camp, J., da Silva, M. C., et al. (2011). Overview of eutrophication indicators to assess environmental status within the European Marine Strategy Framework Directive. Estuar. Coast. Shelf Sci. 93, 117–131. doi: 10.1016/j.ecss.2011.03.014
Filbee-Dexter, K., and Wernberg, T. (2018). Rise of turfs: a new battlefront for globally declining kelp forests. Bioscience 68, 64–76. doi: 10.1093/biosci/bix147
Frigstad, H., Andersen, T., Hessen, D. O., Jeansson, E., Skogen, M., Naustvoll, L.-J., et al. (2013). Long-term trends in carbon, nutrients and stoichiometry in Norwegian coastal waters: evidence of a regime shift. Prog. Oceanogr. 111, 113–124. doi: 10.1016/j.pocean.2013.01.006
Froese, D. G., Westgate, J. A., Reyes, A. V., Enkin, R. J., and Preece, S. J. (2008). Ancient permafrost and a future, warmer Arctic. Science 321:1648. doi: 10.1126/science.1157525
García-Ruiz, J. M., López-Moreno, J. I., Vicente-Serrano, S. M., Lasanta-Martínez, T., and Beguería, S. (2011). Mediterranean water resources in a global change scenario. Earth Sci. Rev. 105, 121–139. doi: 10.1016/j.earscirev.2011.01.006
Gorham, E. (1991). Northern peatlands: role in the carbon cycle and probable responses to climatic warming. Ecol. Appl. 1, 182–195. doi: 10.2307/1941811
Guadayol, O., Peters, F., Marrase, C., Gasol, J. M., Roldan, C., Berdalet, E., et al. (2009). Episodic meteorological and nutrient-load events as drivers of coastal planktonic ecosystem dynamics: a time-series analysis. Mar. Ecol. Prog. Ser. 381, 139–155. doi: 10.3354/meps07939
Haitzer, M., Aiken, G. R., and Ryan, J. N. (2003). Binding of mercury(II) to aquatic humic substances:? influence of pH and source of humic substances. Environ. Sci. Technol. 37, 2436–2441. doi: 10.1021/es026291o
Hamilton, S. K. (2010). Biogeochemical implications of climate change for tropical rivers and floodplains. Hydrobiologia 657, 19–35. doi: 10.1007/s10750-009-0086-1
Hedges, J. I., Keil, R. G., and Benner, R. (1997). What happens to terrestrial organic matter in the ocean? Org. Geochem. 27, 195–212. doi: 10.1016/s0146-6380(97)00066-1
Heisler, J., Glibert, P. M., Burkholder, J. M., Anderson, D. M., Cochlan, W., Dennison, W. C., et al. (2008). Eutrophication and harmful algal blooms: a scientific consensus. Harmful Algae 8, 3–13. doi: 10.1016/j.hal.2008.08.006
Huntington, T. G. (2006). Evidence for intensification of the global water cycle: review and synthesis. J. Hydrol. 319, 83–95. doi: 10.1016/j.jhydrol.2005.07.003
Hyenstrand, P., Rydin, E., and Gunnerhed, M. (2000). Response of pelagic Cyanobacteria to iron additions—enclosure experiments from Lake Erken. J. Plankton Res. 22, 1113–1126. doi: 10.1093/plankt/22.6.1113
Hyndes, G. A., Nagelkerken, I., McLeod, R. J., Connolly, R. M., Lavery, P. S., and Vanderklift, M. A. (2014). Mechanisms and ecological role of carbon transfer within coastal seascapes. Biol. Rev. 89, 232–254. doi: 10.1111/brv.12055
Jansson, M., Karlsson, J., and Blomqvist, P. (2003). Allochthonous organic carbon decreases pelagic energy mobilization in lakes. Limnol. Oceanogr. 48,1711–1716. doi: 10.4319/lo.2003.48.4.1711
Johnson, K. S., Chavez, F. P., and Friederich, G. E. (1999). Continental-shelf sediment as a primary source of iron for coastal phytoplankton. Nature 398, 697–700. doi: 10.1038/19511
Johnson, K. S., Gordon, R. M., and Coale, K. H. (1997). What controls dissolved iron concentrations in the world ocean? Mar. Chem. 57, 137–161. doi: 10.1016/s0304-4203(97)00043-1
Jones, R. I. (1992). The influence of humic substances on lacustrine planktonic food-chains. Hydrobiologia 229, 73–91. doi: 10.1007/bf00006992
Kaiser, K., Benner, R., and Amon, R. M. W. (2017). The fate of terrigenous dissolved organic carbon on the Eurasian shelves and export to the North Atlantic. J. Geophys. Res. Oceans 122, 4–22. doi: 10.1002/2016jc012380
Kamjunke, N., von Tümpling, W., Hertkorn, N., Harir, M., Schmitt-Kopplin, P., Norf, H., et al. (2017). A new approach for evaluating transformations of dissolved organic matter (DOM) via high-resolution mass spectrometry and relating it to bacterial activity. Water Res. 123, 513–523. doi: 10.1016/j.watres.2017.07.008
Karlsson, J., Berggren, M., Ask, J., Bystrom, P., Jonsson, A., Laudon, H., et al. (2012). Terrestrial organic matter support of lake food webs: evidence from lake metabolism and stable hydrogen isotopes of consumers. Limnol. Oceanogr. 57, 1042–1048. doi: 10.4319/lo.2012.57.4.1042
Karlsson, J., Bystrom, P., Ask, J., Ask, P., Persson, L., and Jansson, M. (2009). Light limitation of nutrient-poor lake ecosystems. Nature 460, 506–580. doi: 10.1038/nature08179
Kirk, J. T. O. (2010). Light and Photosynthesis in Aquatic Ecosystems. Cambridge: Cambridge University Press.
Kyselı, J., Beguería, S., Beranová, R., Gaál, L., and López-Moreno, J. I. (2012). Different patterns of climate change scenarios for short-term and multi-day precipitation extremes in the Mediterranean. Glob. Planet. Change 98, 63–72. doi: 10.1016/j.gloplacha.2012.06.010
Lapierre, J. F., Guillemette, F., Berggren, M., and del Giorgio, P. A. (2013). Increases in terrestrially derived carbon stimulate organic carbon processing and CO2 emissions in boreal aquatic ecosystems. Nat. Commun. 4:2972.
Larson, M., and Kraus, N. C. (1995). Prediction of cross-shore sediment transport at different spatial and temporal scales. Mar. Geol. 126, 111–127. doi: 10.1016/0025-3227(95)00068-a
Larsson, U., Elmgren, R., and Wulff, F. (1985). Eutrophication and the Baltic Sea - Causes and consequences. Ambio 14, 9–14.
Liess, A., Rowe, O., Francoeur, S. N., Guo, J., Lange, K., Schroeder, A., et al. (2016). Terrestrial runoff boosts phytoplankton in a Mediterranean coastal lagoon, but these effects do not propagate to higher trophic levels. Hydrobiologia 766, 275–291. doi: 10.1007/s10750-015-2461-4
Lydersen, C., Assmy, P., Falk-Petersen, S., Kohler, J., Kovacs, K. M., Reigstad, M., et al. (2014). The importance of tidewater glaciers for marine mammals and seabirds in Svalbard, Norway. J. Mar. Syst. 129, 452–471. doi: 10.1016/j.jmarsys.2013.09.006
Mann, P. J., Spencer, R. G. M., Hernes, P. J., Six, J., Aiken, G. R., Tank, S. E., et al. (2016). Pan-arctic trends in terrestrial dissolved organic matter from optical measurements. Front. Earth Sci. 4:25. doi: 10.3389/feart.2016.00025
Margvelashvili, N., Andrewartha, J., Baird, M., Herzfeld, M., Jones, E., Mongin, M., et al. (2018). Simulated fate of catchment-derived sediment on the Great Barrier Reef shelf. Mar. Pollut. Bull. 135, 954–962. doi: 10.1016/j.marpolbul.2018.08.018
Massicotte, P., Asmala, E., Stedmon, C., and Markager, S. (2017). Global distribution of dissolved organic matter along the aquatic continuum: across rivers, lakes and oceans. Sci. Total Environ. 609, 180–191. doi: 10.1016/j.scitotenv.2017.07.076
Milliman, J. D., Farnsworth, K., Jones, P., Xu, K., and Smith, L. (2008). Climatic and anthropogenic factors affecting river discharge to the global ocean, 1951–2000. Glob. Planet. Change 62, 187–194. doi: 10.1016/j.gloplacha.2008.03.001
Milly, P. C. D., Wetherald, R. T., Dunne, K., and Delworth, T. L. (2002). Increasing risk of great floods in a changing climate. Nature 415, 514–517. doi: 10.1038/415514a
Monteith, D. T., Stoddard, J. L., Evans, C. D., de Wit, H. A., Forsius, M., Hogasen, T., et al. (2007). Dissolved organic carbon trends resulting from changes in atmospheric deposition chemistry. Nature 450, 537–539.
Morel, F. M. M., Kraepiel, A. M. L., and Amyot, M. (1998). The chemical cycle and bioaccumulation of mercury. Annu. Rev. Ecol. Syst. 29, 543–566. doi: 10.1146/annurev.ecolsys.29.1.543
Moy, F. E., and Christie, H. (2012). Large-scale shift from sugar kelp (Saccharina latissima) to ephemeral algae along the south and west coast of Norway. Mar. Biol. Res. 8, 309–321. doi: 10.1080/17451000.2011.637561
Müller-Navarra, D. C., Brett, M. T., Liston, A. M., and Goldman, C. R. (2000). A highly unsaturated fatty acid predicts carbon transfer between primary producers and consumers. Nature 403, 74–77. doi: 10.1038/47469
Newton, A., Carruthers, T. J. B., and Icely, J. (2012). The coastal syndromes and hotspots on the coast. Estuar. Coast. Shelf Sci. 96, 39–47. doi: 10.1016/j.ecss.2011.07.012
Nixon, S. W. (1988). Physical energy inputs and the comparative ecology of lake and marine ecosystems. Limnol. Oceanogr. 33, 1005–1025. doi: 10.4319/lo.1988.33.4_part_2.1005
Nixon, S. W. (1995). Coastal marine eutrophication: a definition, social causes, and future concerns. Ophelia 41, 199–219. doi: 10.1080/00785236.1995.10422044
Nixon, S. W. (2009). Eutrophication and the macroscope. Hydrobiologia 629, 5–19. doi: 10.1007/978-90-481-3385-7_2
Nyström, M., Folke, C., and Moberg, F. (2000). Coral reef disturbance and resilience in a human-dominated environment. Trends Ecol. Evol. 15, 413–417. doi: 10.1016/s0169-5347(00)01948-0
Osburn, C. L., and Bianchi, T. S. (2016). Editorial: linking optical and chemical properties of dissolved organic matter in natural waters. Front. Mar. Sci. 3:223. doi: 10.3389/fmars.2016.00223
Osburn, C. L., Boyd, T. J., Montgomery, M. T., Bianchi, T. S., Coffin, R. B., and Paerl, H. W. (2016). Optical proxies for terrestrial dissolved organic matter in estuaries and coastal waters. Front. Mar. Sci. 2:127. doi: 10.3389/fmars.2015.00127
Paasche, Ø., Österblom, H., Neuenfeldt, S., Bonsdorff, E., Brander, K., Conley, D. J., et al. (2015). Connecting the seas of norden. Nat. Clim. Change 5, 89–92. doi: 10.1038/nclimate2471
Painter, S. C., Lapworth, D. J., Woodward, E. M. S., Kroeger, S., Evans, C. D., Mayor, D. J., et al. (2018). Terrestrial dissolved organic matter distribution in the North Sea. Sci. Total Environ. 630, 630–647. doi: 10.1016/j.scitotenv.2018.02.237
Pecqueur, D., Vidussi, F., Fouilland, E., Le Floc’h, E., Mas, S., Roques, C., et al. (2011). Dynamics of microbial planktonic food web components during a river flash flood in a Mediterranean coastal lagoon. Hydrobiologia 673, 13–27. doi: 10.1007/s10750-011-0745-x
Peterson, B. J., Holmes, R. M., McClelland, J. W., Vorosmarty, C. J., Lammers, R. B., Shiklomanov, A. I., et al. (2002). Increasing river discharge to the Arctic Ocean. Science 298, 2171–2173. doi: 10.1126/science.1077445
Pittman, J., and Armitage, D. (2016). Governance across the land-sea interface: a systematic review. Environ. Sci. Policy 64, 9–17. doi: 10.1016/j.envsci.2016.05.022
Pranzini, E. (2018). Shore protection in Italy: from hard to soft engineering … and back. Ocean Coast. Manag. 156, 43–57. doi: 10.1016/j.ocecoaman.2017.04.018
Rangel-Buitrago, N., de Jonge, V. N., and Neal, W. (2018a). How to make integrated coastal erosion management a reality. Ocean Coast. Manag. 156, 290–299. doi: 10.1016/j.ocecoaman.2018.01.027
Rangel-Buitrago, N., Williams, A. T., Pranzini, E., and Anfuso, G. (2018b). Preface to the special issue: management strategies for coastal erosion processes. Ocean Coast. Manag. 156, 1–3. doi: 10.1016/j.ocecoaman.2017.11.020
Ravichandran, M. (2004). Interactions between mercury and dissolved organic matter—-a review. Chemosphere 55, 319–331. doi: 10.1016/j.chemosphere.2003.11.011
Regier, P., and Jaffé, R. (2016). Short-term dissolved organic carbon dynamics reflect tidal, water management, and precipitation patterns in a subtropical estuary. Front. Mar. Sci. 3:250. doi: 10.3389/fmars.2016.00250
Riedel, T., Zark, M., Vähätalo, A. V., Niggemann, J., Spencer, R. G. M., Hernes, P. J., et al. (2016). Molecular signatures of biogeochemical transformations in dissolved organic matter from ten world rivers. Front. Earth Sci. 4:85. doi: 10.3389/feart.2016.00085
Sánchez, E., Gallardo, C., Gaertner, M., Arribas, A., and Castro, M. (2004). Future climate extreme events in the Mediterranean simulated by a regional climate model: a first approach. Glob. Planet. Change 44, 163–180. doi: 10.1016/j.gloplacha.2004.06.010
Schartup, A. T., Qureshi, A., Dassuncao, C., Thackray, C. P., Harding, G., and Sunderland, E. M. (2017). A model for methylmercury uptake and trophic transfer by marine plankton. Environ. Sci. Technol. 52, 654–662. doi: 10.1021/acs.est.7b03821
Schindler, D. W. (2006). Recent advances in the understanding and management of eutrophication. Limnol. Oceanogr. 51, 356–363. doi: 10.1007/s13280-015-0755-4
Schuur, E. A., Bockheim, J., Canadell, J. G., Euskirchen, E., Field, C. B., Goryachkin, S. V., et al. (2008). Vulnerability of permafrost carbon to climate change: implications for the global carbon cycle. AIBS Bull. 58, 701–714.
Seekell, D. A., Lapierre, J.-F., Ask, J., Bergström, A.-K., Deininger, A., Rodriguez, P., et al. (2015). The influence of dissolved organic carbon on primary production in northern lakes. Limnol. Oceanogr. 60, 1276–1285. doi: 10.1002/lno.10096
Seidel, M., Yager, P. L., Ward, N. D., Carpenter, E. J., Gomes, H. R., Krusche, A. V., et al. (2015). Molecular-level changes of dissolved organic matter along the Amazon River-to-ocean continuum. Mar. Chem. 177, 218–231. doi: 10.1016/j.marchem.2015.06.019
Seitzinger, S., Mayorga, E., Bouwman, A., Kroeze, C., Beusen, A., Billen, G., et al. (2010). Global river nutrient export: a scenario analysis of past and future trends. Glob. Biogeochem. Cycles 24:GB0A08. doi: 10.1016/j.scitotenv.2009.12.015
Selvaraj, K., Lee, T. Y., Yang, J. Y. T., Canuel, E. A., Huang, J. C., Dai, M., et al. (2015). Stable isotopic and biomarker evidence of terrigenous organic matter export to the deep sea during tropical storms. Mar. Geol. 364, 32–42. doi: 10.1016/j.margeo.2015.03.005
Sholkovitz, E. R. (1976). Flocculation of dissolved organic and inorganic matter during the mixing of river water and seawater. Geochim. Cosmochim. Acta 40, 831–845. doi: 10.1016/0016-7037(76)90035-1
Skarbøvik, E., Allan, I., Sample, J. E., Greipsland, I., Selvik, J. R., Schanke, L. B., et al. (2017). Riverine inputs and direct discharges to Norwegian coastal waters-2016. Miljødirektoratet Rapportserie 862:206.
Soares, A. R., Bergström, A.-K., Sponseller, R. A., Moberg, J. M., Giesler, R., Kritzberg, E. S., et al. (2017). New insights on resource stoichiometry: assessing availability of carbon, nitrogen, and phosphorus to bacterioplankton. Biogeosciences 14, 1527–1539. doi: 10.5194/bg-14-1527-2017
Sobek, S., Tranvik, L. J., Prairie, Y. T., Kortelainen, P., and Cole, J. J. (2007). Patterns and regulation of dissolved organic carbon: an analysis of 7,500 widely distributed lakes. Limnol. Oceanogr. 52, 1208–1219. doi: 10.4319/lo.2007.52.3.1208
Solomon, C. T., Jones, S. E., Weidel, B. C., Buffam, I., Fork, M. L., Karlsson, J., et al. (2015). Ecosystem consequences of changing inputs of terrestrial dissolved organic matter to lakes: current knowledge and future challenges. Ecosystems 18, 376–389. doi: 10.1007/s10021-015-9848-y
Søndergaard, D. N. (2004). Dissolved organic matter (DOM) in aquatic ecosystems: a study of European catchments and coastal waters. Eur. Union Domain Proj. 76, 1–76.
Sorichetti, R. J., Creed, I. F., and Trick, C. G. (2014). The influence of iron, siderophores and refractory DOM on cyanobacterial biomass in oligotrophic lakes. Freshw. Biol. 59, 1423–1436. doi: 10.1111/fwb.12355
Sorichetti, R. J., Creed, I. F., and Trick, C. G. (2016). Iron and iron-binding ligands as cofactors that limit cyanobacterial biomass across a lake trophic gradient. Freshw. Biol. 61, 146–157. doi: 10.1111/fwb.12689
Stepanauskas, R., Jørgensen, N. O. G., Eigaard, O. R., Žvikas, A., Tranvik, L. J., and Leonardson, L. (2002). Summer inputs of riverine nutrients to the Baltic Sea: bioavailability and eutrophication relevance. Ecol. Monogr. 72, 579–597. doi: 10.1890/0012-9615(2002)072%5B0579:siornt%5D2.0.co;2x
Su, L., Miao, C., Kong, D., Duan, Q., Lei, X., Hou, Q., et al. (2018). Long-term trends in global river flow and the causal relationships between river flow and ocean signals. J. Hydrol. 563, 818–833. doi: 10.1016/j.jhydrol.2018.06.058
Tanski, G., Lantuit, H., Ruttor, S., Knoblauch, C., Radosavljevic, B., Strauss, J., et al. (2017). Transformation of terrestrial organic matter along Thermokarst-affected permafrost coasts in the Arctic. Sci. Total Environ. 581, 434–447. doi: 10.1016/j.scitotenv.2016.12.152
Thrane, J.-E., Hessen, D., and Andersen, T. (2014). The absorption of light in lakes: negative impact of dissolved organic carbon on primary productivity. Ecosystems 17, 1040–1052. doi: 10.1007/s10021-014-9776-2
Toimil, A., Losada, I. J., Camus, P., and Díaz-Simal, P. (2017). Managing coastal erosion under climate change at the regional scale. Coast. Eng. 128, 106–122. doi: 10.1016/j.coastaleng.2017.08.004
Trannum, H. C., Gundersen, H., Oug, E., Rygg, B., and Norderhaug, K. M. (2018). Soft bottom benthos and responses to climate variation and eutrophication in Skagerrak. J. Sea Res. 141, 83–98. doi: 10.1016/j.seares.2018.08.007
Ullrich, S. M., Tanton, T. W., and Abdrashitova, S. A. (2001). Mercury in the aquatic environment: a review of factors affecting methylation. Crit. Rev. Environ. Sci. Technol. 31, 241–293. doi: 10.1080/20016491089226
Waelbroeck, C., Monfray, P., Oechel, W. C., Hastings, S., and Vourlitis, G. (1997). The impact of permafrost thawing on the carbon dynamics of tundra. Geophys. Res. Lett. 24, 229–232. doi: 10.1029/97gl00071
Ward, N. D., Bianchi, T. S., Medeiros, P. M., Seidel, M., Richey, J. E., Keil, R. G., et al. (2017). Where carbon goes when water flows: carbon cycling across the aquatic continuum. Front. Mar. Sci. 4:7. doi: 10.3389/fmars.2017.00007
Weyhenmeyer, G. A., Fröberg, M., Karltun, E., Khalili, M., Kothawala, D., Temnerud, J., et al. (2012). Selective decay of terrestrial organic carbon during transport from land to sea. Glob. Change Biol. 18, 349–355. doi: 10.1111/j.1365-2486.2011.02544.x
Wikner, J., and Andersson, A. (2012). Increased freshwater discharge shifts the trophic balance in the coastal zone of the northern Baltic Sea. Glob. Change Biol. 18, 2509–2519. doi: 10.1111/j.1365-2486.2012.02718.x
Williams, A. T., Rangel-Buitrago, N., Pranzini, E., and Anfuso, G. (2018). The management of coastal erosion. Ocean Coast. Manag. 156, 4–20. doi: 10.1016/j.ocecoaman.2017.03.022
Keywords: browning, coastal darkening, dissolved organic carbon, eutrophication, nutrients, organic carbon, terrestrial organic matter
Citation: Deininger A and Frigstad H (2019) Reevaluating the Role of Organic Matter Sources for Coastal Eutrophication, Oligotrophication, and Ecosystem Health. Front. Mar. Sci. 6:210. doi: 10.3389/fmars.2019.00210
Received: 01 October 2018; Accepted: 03 April 2019;
Published: 25 April 2019.
Edited by:
Marianne Holmer, University of Southern Denmark, DenmarkReviewed by:
Rodrigo Riera, Catholic University of the Most Holy Conception, ChileCesar De Castro Martins, Federal University of Paraná, Brazil
Copyright © 2019 Deininger and Frigstad. This is an open-access article distributed under the terms of the Creative Commons Attribution License (CC BY). The use, distribution or reproduction in other forums is permitted, provided the original author(s) and the copyright owner(s) are credited and that the original publication in this journal is cited, in accordance with accepted academic practice. No use, distribution or reproduction is permitted which does not comply with these terms.
*Correspondence: Anne Deininger, YW5uZS5kZWluaW5nZXJAbml2YS5ubw==