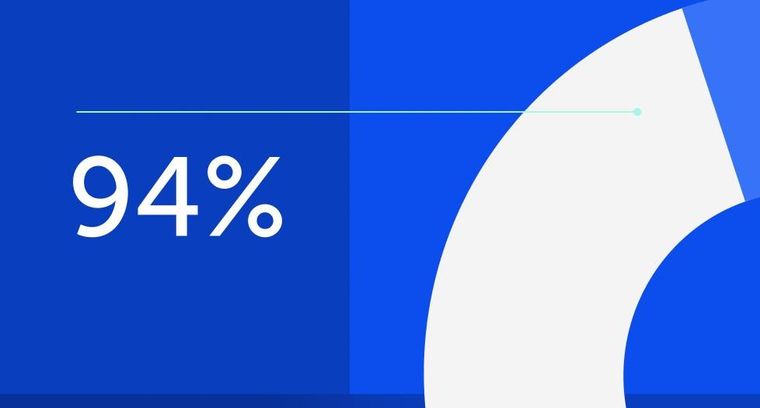
94% of researchers rate our articles as excellent or good
Learn more about the work of our research integrity team to safeguard the quality of each article we publish.
Find out more
REVIEW article
Front. Mar. Sci., 02 April 2019
Sec. Marine Megafauna
Volume 6 - 2019 | https://doi.org/10.3389/fmars.2019.00087
This article is part of the Research TopicFuture Directions in Research on Marine MegafaunaView all 20 articles
Long-lived species share life history traits such as slow growth, late maturity, and low fecundity, which lead to slow recovery rates and increase a population’s vulnerability to disturbance. The Greenland shark (Somniosus microcephalus) has recently been recognized as the world’s longest-lived vertebrate, but many questions regarding its biology, physiology, and ecology remain unanswered. Here we review how current and future research will fill knowledge gaps about the Greenland shark and provide an overall framework to guide research and management priorities for this species. Key advances include the potential for specialized aging techniques and demographic studies to shed light on the distribution and age-class structure of Greenland shark populations. Advances in population genetics and genomics will reveal key factors contributing to the Greenland shark’s extreme longevity, range and population size, and susceptibility to environmental change. New tagging technologies and improvements in experimental and analytical design will allow detailed monitoring of movement behaviors and interactions among Greenland sharks and other marine species, while shedding light on habitat use and susceptibility to fisheries interactions. Interdisciplinary approaches, such as the combined use of stable isotope analysis and high-tech data-logging devices (i.e., accelerometers and acoustic hydrophones) have the potential to improve knowledge of feeding strategies, predatory capabilities, and the trophic role of Greenland sharks. Measures of physiology, including estimation of metabolic rate, as well as heart rate and function, will advance our understanding of the causes and consequences of long lifespans. Determining the extent and effects of current threats (as well as potential mitigation measures) will assist the development of policies, recommendations, and actions relevant for the management of this potentially vulnerable species. Through an interdisciplinary lens, we propose innovative approaches to direct the future study of Greenland sharks and promote the consideration of longevity as an important factor in research on aquatic and terrestrial predators.
The classification of life history traits along a continuum between r- and K-selection is regarded as a foundational tool for predicting the ability of animal populations to effectively respond to environmental and/or anthropogenic disturbance (Pianka, 1970). In higher order vertebrates, extreme longevity is commonly associated with the possession of K-selected life history traits that influence maximum intrinsic population growth rates (rmax) – such as slow growth, late maturity, and relatively low recruitment rates (McCann and Shuter, 1997). Such traits are thought to limit the ability of animal populations to sustain high levels of mortality (Adams, 1980; Musick, 1999a; Simpfendorfer and Kyne, 2009; Norse et al., 2012). In chondrichthyans, the frequency of these K-selected traits becomes more pronounced with increasing depth (Rigby and Simpfendorfer, 2015), resulting in significantly lower rates of population increase in deep-water species, and a heightened vulnerability to exploitation and incidental mortality relative to their shallow-water counterparts (García et al., 2008; Simpfendorfer and Kyne, 2009). Many deep-water species are currently targeted in commercial fisheries (Barker and Schluessel, 2005) and the number of deep-water habitats affected by fishing is expected to increase (Halpern et al., 2008). In addition, impacts from other human activities (e.g., seismic surveys) on deep-sea biota are virtually unknown, as are the chronic and cumulative impacts from multiple stressors, including climate change and pollution.
The importance of longevity in influencing the vulnerability of animal populations to overexploitation has long been overlooked in conservation and management planning. It is only relatively recently, following well-known examples of overfishing (e.g., New Zealand orange roughly, Hoplostethus atlanticus, and North Atlantic cod, Gadus morhua; Walters and Maguire, 1996; Clark, 2001) and declines in many long-lived marine species (Myers and Worm, 2003; Heppell et al., 2005), that there has beenx increased consideration of K-selected traits in marine management policy (Musick, 1999b; Musick et al., 2000; Baum et al., 2003). This is especially true for the protection of species targeted by commercial or recreational fisheries, including some deep-water chondrichthyan and teleost fishes (Stevens et al., 2000; Simpfendorfer and Kyne, 2009; Norse et al., 2012; Rigby and Simpfendorfer, 2015). While current literature asserts the importance of longevity for predicting the effects of anthropogenic disturbance on wild populations, the mechanisms driving extreme longevity and its influences on animal behavior and population dynamics are not well understood.
The Greenland shark (Somniosus microcephalus) is a newly recognized example of an extremely long-lived deep-water chondrichthyan (estimated lifespan of at least 272 years; Nielsen et al., 2016), that is both data deficient and vulnerable to human threats such as fishery-related mortality (Davis et al., 2013). Greenland sharks are primarily known to inhabit deep-water and coastal regions of the Arctic and North Atlantic (Yano et al., 2007, see Figure 1 for capture locations from the reviewed studies), but have the potential to range globally where deep-water temperatures are <5°C (MacNeil et al., 2012) and have been observed both at the surface and at confirmed depths up to 1,816 m (Campana et al., 2015a, Somniosid spp. have also been observed at 2,200 and 2,992 m; Herdendorf and Berra, 1995; Porteiro et al., 2017, respectively). While historically fished in the waters off Iceland, Greenland, and Norway to support a liver oil industry, decreased demand in the mid 20th century prompted the closure of targeted fisheries; currently, Greenland sharks are primarily caught as bycatch in commercial longline, gillnet, and bottom trawl fisheries (Davis et al., 2013). Despite a recent spike in public interest due to its reported longevity, logistical challenges continue to impede the detailed study of this species. Consequently, relatively little is known about several important aspects of Greenland shark physiology (e.g., metabolism, reproduction), ecology (e.g., age class structure, distribution, ecological role, genetic variability), and behavior (e.g., predatory capabilities, horizontal movement patterns). The primary objective of this paper is to highlight important knowledge gaps that limit the development of a management framework for the Greenland shark. In the following text, we discuss a variety of forward-thinking experimental approaches that will enable researchers to answer key questions about Greenland shark biology and ecology (Table 1). Additionally, we present these current and future advances in Greenland shark research within the context of broader issues regarding the study of long-lived species (Table 2).
Figure 1. Geographic coverage of Greenland shark studies by sampling location and research topic: (A) locations of Greenland shark capture/sampling (points represent sampling regions reported by each study, multiple points used for studies with several disparate sampling sites), (B) study count by country of Greenland shark capture/sampling and research focus (studies counted more than once where multiple sampled countries are reported).
Table 1. An overview of current knowledge and data deficiencies in eight fields of Greenland shark (Somniosus microcephalus) research, strategies to address data deficiencies, and potential actions to support management organization recommendations and Greenland shark conservation.
Experts in the study of Greenland sharks from several research and management institutions were consulted. Guided by the previous scientific contributions of this expert team, eight topics were deemed to be of high priority to assist future Greenland shark research and management. The chosen topics are outlined in the text as follows: (1) Demographics and life history, (2) Population genetics and genomics, (3) Movement ecology, (4) Behavior, (5) Physiological adaptations, (6) Diet and trophic ecology, (7) Threats to Greenland shark populations, and (8) Management (see Figure 1 for study count by research topic). Each topic was then organized into four key sections: (i) current knowledge in the chosen field, (ii) identified knowledge gaps and limitations, (iii) proposed strategies to address identified data deficiencies, and finally, (iv) potential recommendations for Greenland shark management. While some topic overlap among sections was unavoidable due to the interconnected nature of much of the examined and proposed research, attempts were made to minimize this.
Recent radiocarbon dating of Greenland shark eye lenses supports the long-standing prediction of exceptional longevity in this species (Nielsen et al., 2016; Figure 2A). These data also provide the first age-at-length data for Greenland sharks.
Figure 2. Examples of Greenland shark demographics research. (A) Greenland shark eye lens for use in radiocarbon dating (photo Julius Nielsen, used with permission). (B) Measuring the length of a Greenland shark captured through ice (photo Gregory Skomal, used with permission). (C) Multiple Greenland sharks captured on BRUV footage (photo Brynn Devine, used with permission). (D) BRUV image showing scar patterns used for identification of individual Greenland sharks (photo Brynn Devine, used with permission). (E) Greenland sharks caught as bycatch in a fisheries trawl (photo Julius Nielsen, used with permission).
Current knowledge of Greenland shark distribution and population abundance are based on exploratory fisheries surveys, stock assessments of commercial species (e.g., Greenland halibut, Reinhardtius hippoglossoides, and Northern shrimp, Pandalus borealis), and bycatch reports from commercial and historical fisheries. Contemporary catch is restricted to small-scale targeted fisheries in Greenland and Iceland, as well as incidental bycatch in a variety of North Atlantic and Arctic fisheries (ICES, 2017). In the Barents Sea, annual Greenland shark bycatch is estimated to be 140–150 tons (Rusyaev and Orlov, 2013) and in northern Canada, 105 tons between 1996 and 2015 (Department of Fisheries, and Oceans [DFO], 2016). However, annual bycatch is likely to be much higher, with an estimated bycatch of 1000 tons/yr in the Uummannaq district in northwestern Greenland alone (Gunnarsdottir and Jørgensen, 2008; ICES, 2017).
Scientific catch data suggest regional differences in the relative abundance of Greenland sharks by life stage/size and sex. For example, no adult females [>4 m total length (LT), Yano et al., 2007] have been reported throughout the Canadian Arctic and in Svalbard (Norway) despite intense sampling (N > 300 individuals, Skomal and Benz, 2004; Fisk et al., 2002, 2012; Leclerc et al., 2012; Hussey et al., 2015a, 2018; Devine et al., 2018). In contrast, adult females appear to be more frequent off southwestern Greenland, Iceland, and Newfoundland (Canada) (Yano et al., 2007; McMeans et al., 2010; Nielsen et al., 2014, 2016; Campana et al., 2015a), and several females > 5 m have been measured off Atlantic Canada (Campana personal communication). Juvenile sharks, defined as individuals ≤200 cm LT, have been observed in both inshore and offshore waters (Hussey et al., 2015a), including animals within the birth size range (40–100 cm, MacNeil et al., 2012). Specifically, juveniles were observed in longline, trawl, and camera surveys within Scott Inlet, Baffin Island, and in offshore waters >1000 m depth (Fisk et al., 2002; Yano et al., 2007; Nielsen et al., 2014; Hussey et al., 2015a; Devine et al., 2018).
Baited remote underwater video (BRUV) surveys have provided the first estimates of the relative abundance of Greenland sharks in the Canadian Arctic (independent of bycatch data; Devine et al., 2018, Figure 2C). By identifying individuals using scar patterns and coloration, the number of sharks observed in each camera deployment was quantified, allowing estimations of observation rate across sampled regions (Devine et al., 2018, Figure 2D). BRUV surveys and exploratory fisheries in the Canadian Arctic Archipelago have also expanded the species’ known northern and western ranges in inshore Canadian waters, highlighting the extent to which their range was historically defined by the spatial extent of commercial fisheries.
While radiocarbon dating of eye lenses has provided baseline age estimates for this species, these estimates still need to be verified with an independent, accurate, and precise age determination technique. However, the absence of hard tissues containing growth bands (such as fin spines, calcified vertebra, etc.) complicates such a validation. Other chemical dating techniques, such as aspartic acid racemization (which has been successfully applied on several cetaceans; George et al., 1999; Garde et al., 2007) have proven to be unsuccessful in providing independent age estimates for this species (Nielsen, 2013), highlighting the need for innovative aging methodologies.
In addition, many questions concerning population demographics – particularly population size and productivity, as well as reproductive biology, fertility, and natural mortality remain unknown or poorly understood. For example, knowledge of the reproductive biology of Greenland sharks is extremely limited. Only one pregnant female with ten near-term pups of 37 cm LT has been reported (Koefoed, 1957). Observations of a high ovarian fecundity (>400 goose-egg sized unfertilized ova; Bjerkan, 1957; Yano et al., 2007; Nielsen et al., 2014; Campana personal communication) suggest that embryos may be aplacental viviparous, similar to those of spiny dogfish (Squalus acanthias) and Portuguese sharks (Centroscymnus coelolepis) (Campana et al., 2009; Castro, 2011). The gestation period, the locations of mating and pupping grounds, and the mating period all remain unknown. Greenland sharks may exhibit seasonal migrations for reproductive purposes (mating and pupping), but the existence or possible extent of connectivity between regions has yet to be documented. Given its extended longevity and the low productivity of sharks relative to teleosts (Musick, 1999a), the Greenland shark is expected to have particularly low productivity.
There is also a general lack of knowledge on the size and sex of Greenland sharks caught as bycatch in commercial fisheries (past and present) (Section “Threats to Greenland Shark Populations,” Figure 2E). Consequently, data available to evaluate the spatial distribution of shark life stages are sparse. Fishery-derived data are also problematic for estimating population abundance due to the spatially targeted nature of fisheries which limits the ability to extrapolate catch rates to larger areas. Estimation of the spatial distribution of Greenland sharks using commercial bycatch records is further confounded by misidentification with other large (but unrelated) shark species, for instance the basking shark (Cetorhinus maximus; Campana et al., 2008b).
Improved or novel age determination methods need to be developed for the routine aging of Greenland sharks (and sleeper sharks in general). With uncertainty surrounding age estimates for Greenland sharks, an improved understanding of the residence time and pathway of past bomb radiocarbon pulses into deep Arctic waters and in Greenland shark prey is required to improve the precision and accuracy of the radiocarbon dating method. Data are available for the otoliths of some Arctic fishes, beluga whale teeth, and vertebrae from several lamniform sharks (Stewart et al., 2006; Campana et al., 2008a; Hamady et al., 2014), but the organic pathway for the eye lens is not well-documented. Equally, mark-recapture methods could provide accurate estimates of size-dependent growth, which can be used to model growth rate (Francis, 1988), and thus age, with few assumptions. However, acquiring precise lengths for such large sharks at both tagging and recapture requires careful measurement in the field under logistically challenging conditions (as described by Hansen, 1963; see also Figure 2B) and a reliance on fishers to provide accurate length estimates for bycaught sharks.
The broad-scale use of BRUVs and modification of established population assessment methodologies should address many remaining questions about Greenland shark distribution and abundance (Table 1). Systematic surveys of local traditional knowledge and accounts from fishermen could help fill gaps in distribution, while the expansion of BRUV surveys could explore differences in relative abundances among sites, and seasonal BRUV surveys could help determine cyclic patterns in distribution. Although scar patterns and the coloration of individuals may change over time (Robbins and Fox, 2012), the use of colored Floy tags or other external markers could provide reliable long-term identification for both BRUVs and fishery-mediated surveys (Table 2). By modifying conventional mark-recapture models to integrate auxiliary data derived from acoustic or satellite telemetry, the effects of low recapture rates can be offset, resulting in more precise estimates of demographic parameters such as apparent survival and abundance (Dudgeon et al., 2015).
More data are needed to evaluate variation in life stage distribution patterns among regions. Such data can be collected from sharks caught in commercial fisheries as bycatch (e.g., improving data collection on capture location, LT, and sex; see Table 1), as well as by expanding large-scale, long-term tagging studies to include all life stages (see section “Movement Ecology”). Detailed reporting of Greenland shark bycatch will also improve growth rate estimates, as well as our understanding of exploitation rates and migration pathways, and could highlight sensitive areas for specific life stages. Tagging and release of mature females with pop-up archival satellite tags (during the open water season) could help identify critical habitats or areas that are important for mating and/or pupping, while at-sea sampling of bycatch mortalities will provide opportunities for the biological examination of mature or maturing females and additional data on ovarian and uterine fecundity.
Biological reference points and conservation targets typically require either population productivity values (based on growth and reproduction) or stock-recruitment curves (based on spawning stock biomass and fecundity). In the absence of these data for Greenland sharks, precautionary measures will be needed to protect sensitive habitats (such as potential mating grounds, pupping grounds, and nursery areas) and limit total catch. Identification of these ecologically important habitats is therefore critical for mitigating bycatch (see section “Movement Ecology”). Another key difficulty in setting conservation limits is uncertainty over whether historical catch rates of around 50,000 individuals/yr in the northern European liver oil fishery (MacNeil et al., 2012) were sustained by local populations or emigration from a wider meta-population. Given the importance of these population-level data for implementing precautionary measures, assessments of current Greenland shark abundance and distribution should be made a high priority (Table 1).
Molecular genetic data, primarily based on mitochondrial DNA (mtDNA), has yielded important insight into the phylogeographic evolutionary history of the Greenland shark and other members of the genus Somniosus. Early work focused on the Pacific sleeper shark (S. pacificus), but also included a number of Greenland sharks and revealed relatively shallow genetic divergence between these two species (∼1.8% sequence divergence; Murray et al., 2008). This study also documented the first evidence of haplotype sharing between two recognized Somniosus species (S. pacificus and the southern sleeper shark, S. antarcticus; Murray et al., 2008), contrasting the earlier resurrection of S. antarcticus as a distinct species (Yano et al., 2004). Further evidence of interspecies haplotype sharing has been reported for several juvenile Greenland sharks from the high Arctic that were found to be carrying S. pacificus mtDNA haplotypes (Hussey et al., 2015a). More recently, a full mtDNA genome sequenced with phylogenetic reconstruction placed the Greenland shark and Pacific sleeper shark as sister species with respect to other major shark orders (Santaquiteria et al., 2017). By calibrating the mitogenomic phylogenetic reconstruction with fossil records and geological events, the speciation time of these two species was confirmed to be 3.5 million years ago (mya) (Santaquiteria, 2016; in agreement with Murray et al., 2008). A further phylogeographic study also confirmed mtDNA haplotype sharing between Greenland and Pacific sleeper sharks at additional locations as well as nuclear admixture, implicating interspecific hybridization as a phenomenon among Atlantic Somniosids (Walter et al., 2017). These data yielded a more recent speciation time for Greenland sharks than findings obtained using whole mitogenomes (2.1 mya versus 3.5 mya). Reconciling the rates of intra- and inter-specific admixture among the Somniosids with (incipient) speciation therefore holds much promise for clarifying the phylogeographic evolutionary history of this genus.
Reliance on mtDNA data to date paints an incomplete picture of the evolutionary history of the Greenland shark. Shared genetic signatures among extant species have led researchers to question the validity of current species descriptions, suggesting that a more comprehensive systematic revision of the genus is needed. In addition to signals of interspecific gene flow and incipient speciation, the demographic history of the Greenland shark across its range indicates that the Atlantic population has persisted at a low, but stable size over the last 5 million years. This was likely followed by a recent population increase around 500,000 years ago, possibly linked to fluctuations in Arctic sea ice conditions during the Pleistocene. As yet, no clear or conspicuous regional geographic structure has emerged from the mtDNA data, aside from indications of genetic admixture (Murray et al., 2008; Walter et al., 2017).
The potential for extreme longevity exhibited by Greenland sharks (Nielsen et al., 2016) presents unique challenges for estimating relevant conservation parameters such as effective population size (Ne) (Table 2). Firstly, the value of Ne is highly sensitive to generation time. While estimates of Ne (both long-term and short-term estimates) are possible from genetic data, the translation of these values to biologically relevant terms is not straightforward. For example, coalescent-based estimates of long-term Ne were obtained from a 702 bp fragment of Cytochrome b using 20 and 150 years generation times (Walter et al., 2017), the latter based on an estimated age at first reproduction of 156 ± 22 years (Nielsen et al., 2016). These long generation times yielded Ne estimates 7.5× smaller than if a 20 years generation time was used. It is important to note that long-term Ne estimates are largely only applicable for comparative, rather than conservation purposes. Nevertheless, these scalar problems will remain a challenge, even with genomic advances, until firm generation times for Greenland sharks are determined.
Estimates of mutation rates in elasmobranchs are considered to be among the slowest in the animal kingdom (Martin et al., 1992). To date, mutation rates for Somniosus spp. are unknown. Consequently, much of the dating for speciation times are hypothetical, however, the accumulation of genome-wide variation among multiple Greenland shark individuals holds promise for estimating Somniosus-specific mutation rates. Obtaining approximate timing of speciation events, population expansions and reductions, and other genus-specific events using genetic methods will continue to be problematic until accurate mutation rates can be estimated.
Advances in population genomics, including complete mitogenomes, nuclear genomes, and genotype-by-sequencing approaches, appear promising for detecting population structure and evolutionary relationships for the Greenland shark and Somniosids in general. The most desirable nuclear molecular markers are those that permit detection and spatial delineation of populations (Ahonen et al., 2009). Such resolution is critical for determining evolutionarily significant units (ESUs; Table 2) or management units for conservation and management planning (Moritz, 1994). Genomic and genetic resources, such as numerous single nucleotide polymorphism (SNP) loci and highly polymorphic nuclear microsatellites, may provide the tools for determining the number of extant populations and the extent of connectivity among them (Milano et al., 2014). Novel sampling methodologies, such as the collection of external parasites to obtain host mitochondrial DNA sequences (Meekan et al., 2017), have proven effective for obtaining genetic samples for such analyses. These invertebrate DNA (iDNA) sequences have helped resolve the genetic structure and connectivity of global whale shark populations (Rhincodon typus; Meekan et al., 2017) and could be similarly applied using copepods sampled from parasitized Greenland sharks. While the spatial scale of elasmobranch populations varies widely from species to species, the geographical range of Greenland shark populations is likely far-reaching. The coupling of population genomic structure for S. microcephalus in the Atlantic and beyond, with movement data for analyzed individuals, will yield the most accurate distribution data for this species.
Despite growing conservation concerns for many shark species, the assignment of conservation status and the effective management of at-risk populations is commonly hindered by a lack of knowledge of stock structure and estimates of absolute population size. Detailed examination of the genomic structure of Greenland shark populations (using non-lethal biopsies or iDNA; Meekan et al., 2017) might allow researchers to define genetically distinct stocks, thereby supporting the delineation of appropriate management boundaries and multinational agreements. Furthermore, the examination of genetic relatedness among conspecifics is becoming an increasingly popular tool to estimate total population abundance (Table 1). For example, a recent study used genetic analyses to identify half-sibling pairs (HSPs) and unrelated pairs (UPs) to estimate abundance and survival rates of adult white sharks in Eastern Australia and New Zealand (Carcharodon carcharias). These data were further supplemented by acoustic tag data to estimate juvenile survival rates. Ultimately this method provided direct estimates of total abundance across both spatial and temporal life-history gradients (Hillary et al., 2018) and could similarly be used to assess Greenland shark populations.
Data on Greenland shark movements have been primarily recorded using a variety of telemetry technologies. Early work involved active short-term acoustic tracking of sharks under ice (Skomal and Benz, 2004; Figure 3F) and in estuarine waters (Stokesbury et al., 2005; Gallant et al., 2016). These tracks recorded vertical movements and light-based depth preferences, indicating that the Greenland shark is not strictly a demersal species. More recently, acoustic tracking in the St. Lawrence Estuary revealed that vertical movements into shallow and warmer water coincided with the pre-dawn high tide (Gallant et al., 2016). Archived depth records from pop-off archival satellite tags have also captured Greenland sharks swimming in the water column in deep-water offshore regions of the Arctic and North Atlantic (Campana et al., 2015a). These records demonstrate a preference among Greenland sharks for greater depths at lower latitudes (mean time-weighted depth over 6 h was 367 ± 4 m for Arctic sharks, compared to 949 ± 10 m for N. Atlantic sharks) and revealed long-distance horizontal movements, including one shark that traveled 1015 km over 125 days (Campana et al., 2015a).
Figure 3. Examples of Greenland shark movement studies: (A) A biologging package containing an accelerometer (Little Leonardo) mounted to the head (photo © Yuuki Watanabe, used with permission). (B) A biologging package containing a spot 6 tag (Wildlife Computers), a VHF tag, an accelerometer (Maritime Biologgers), and a CTD (Star Oddi) (photo © HusseyLab, used with permission). (C) An archival satellite tag and multiple mrPAT tags (Wildlife Comupters) mounted to the dorsal fin (photo © HusseyLab, used with permission). (D) A fin-mounted archival satellite tag (Wildlife Computers) and external marker tag (photo © HusseyLab, used with permission). (E) Internal implantation of an acoustic tag (VEMCO) for static acoustic tracking (photo © Kelsey Johnson, used with permission). (F) A dorsal fin-mounted acoustic tag (VEMCO) for mobile acoustic tracking (photo © Gregory Skomal, used with permission).
Past studies using biotelemetry to record Greenland shark movements have largely relied on two methods, mobile acoustic tracking (Figure 3F) and archival satellite telemetry (Figures 3C,D); two approaches that require researchers to sacrifice either monitoring duration or data resolution. For example, active acoustic tracking can provide fine-scale horizontal movement data, but over limited timespans (hours or days; Skomal and Benz, 2004; Stokesbury et al., 2005; Gallant et al., 2016). In contrast, satellite tags can remain on animals for periods of up to several months (Stokesbury et al., 2005; Campana et al., 2015a), but produce only straight-line trajectories of tagged animals (via extrapolation between the location of animal capture and the ARGOS location transmitted by satellite tags following their pre-programmed release). Knowledge of the locations and timing of movement pathways, aggregation sites, and areas of ecological importance for Greenland sharks (e.g., feeding grounds, and nurseries) therefore remains limited. Understanding the biological significance of movement behaviors is consequently difficult to assess and will require further study using available technologies and experimental design innovations.
The application of extensive passive acoustic telemetry infrastructure (fixed receivers that detect tagged individuals within their detection range; Heupel et al., 2005; Hussey et al., 2015b) and long lifespan transmitters (∼10 years; Figure 3E) provides one solution to examine the long-term movements of Greenland sharks over a range of spatial scales. By combining multi-year records of animal detections with environmental data (ice cover, temperature, salinity, and dissolved oxygen), it will be possible to determine the spatiotemporal scales of predictable horizontal movements. Specifically, these data will delineate migration routes and activity hotspots as well as the environmental factors driving observed behaviors. The continued growth of a large-scale network telemetry approach in the Arctic (Hussey et al., 2015b) will promote collaborative data storage and handling and will rapidly increase data collection. For example, a telemetry network established in Baffin Bay was designed by the Ocean Tracking Network (OTN) in collaboration with the Canadian fishing industry and the Department of Fisheries and Oceans Canada (DFO) and is maintained by the joint efforts of all three groups (Cooke et al., 2011).
Innovative experimental designs, including the novel application or combination of existing telemetry technologies, can also allow researchers to overcome some of the limitations of tag design. For example, the attachment of multiple timed-release mark-report satellite tags (mrPATs, Wildlife Computers; Figure 3C) to individual sharks, has allowed the detection of a potential migration pathway and revealed synchronicity in Greenland shark movements (Hussey et al., 2018). The intermediate location estimates provided by this approach (typically not captured by satellite tag studies on non-surfacing animals) increased the resolution of recorded movement trajectories and provided data which are key for identifying suitable locations for the deployment of future acoustic telemetry infrastructure (Hussey et al., 2018). Furthermore, by combining a variety of telemetry technologies on individuals (e.g., satellite tags, acoustic transmitters and receivers, and accelerometers; Figures 3A,B), researchers can compare movement behaviors at different data resolutions and timescales, informing habitat use, environmental conditions, and intra- or interspecies interactions, while maximizing the data collected for each tagged individual (Holland et al., 2009; Meyer et al., 2010; Hussey et al., 2018).
Despite rapid advances in telemetry technology and applications, capturing the ranges and movements of extremely long-lived species will require continued improvements in equipment design to increase tag longevity and the maximum depth ratings of telemetry equipment. Technological developments such as piezoelectric energy harvesting, which powers battery-free acoustic tags using the flexing motions of swimming fish (Li et al., 2016), will alleviate the limitations imposed by tag battery life. Additionally, mobile monitoring using animal-borne transceivers or autonomous vehicles (gliders; Lennox et al., 2017) will increase the coverage of telemetry studies and maximize potential data retrieval. Acoustic transceivers (VEMCO Mobile Transceiver; VMT) are transforming animals into mobile receivers capable of detecting other tagged individuals (Lidgard et al., 2012; Baker et al., 2014; Figure 3B) and have, thus far, been used to detect conspecific interactions in remote habitats (Holland et al., 2009) and seasonal patterns in group dynamics (fission–fusion behavior; Haulsee et al., 2016). However, these methods currently require the animal to be recaptured to recover the tag and associated data. The development of Bluetooth technology linking VMTs and pop-off archival satellite tags (following the approach of Lidgard et al., 2014) will allow the remote transfer of animal detection data from VMTs to ARGOS satellites, eliminating the need for tag retrieval. This will thereby reveal interactions between Greenland sharks and other tagged animals, providing insight into their feeding and predatory behaviors (see section “Behavior”). Further advancement of archival satellite tags, including the recording of high quality oceanographic data and faster data transmission to ARGOS satellites (increasing the volume of data transmitted and received), will also improve our understanding of environmental factors influencing the spatial habitat use of Greenland sharks.
Understanding the seasonal horizontal movements and spatial habitat use of Greenland sharks is critical for marine spatial planning (e.g., ATLAS case study for Davis Strait, Eastern Arctic). Large-scale movements recorded by acoustic and/or satellite telemetry (tied with genomic data to identify population units, see section “Population Genetics and Genomics”) will help to refine our understanding of broad-scale Greenland shark distributions, allowing for the establishment of bilateral or multilateral agreements between nations or via Regional Management Organizations (RFMOs, see section “Management”) to manage at the population level (Table 1). At a finer scale, telemetry approaches will allow high-use or hotspot areas to be identified, and predictable movement patterns to be defined. These data, along with the distributions of potential threats (such as fisheries activity), can be integrated into predator-prey models (McClellan et al., 2009) which will indicate the spatial and temporal extent of regions that should be avoided or where fishing should be time-restricted during periods of increased Greenland shark presence. If necessary, this information would allow the implementation of precautionary measures such as Dynamic Area Management (DAM; NOAA, 2002) to reduce the likelihood of excessive bycatch (Table 1). Developing an understanding of the environmental drivers affecting Greenland shark movements will also improve our ability to predict how these patterns will be modified under various climate change scenarios, further directing the implementation of precautionary measures. Systematic conservation planning using spatial prioritization software tools, such as Marxan (Ball et al., 2009), will provide an approach to integrate these data to produce direct management advice (Metcalfe et al., 2015; Table 1).
To date, direct observations of Greenland shark behavior are extremely limited. Our understanding of their foraging mechanisms and predatory capabilities are largely based on combined evidence from movement records (see section “Movement Ecology”) and dietary analyses (see section “Diet and Trophic Ecology”). A single study on the three-dimensional movements of Greenland sharks recorded by high-resolution data loggers reported the slowest observed mean swim speed (0.34 ms-1) and tailbeat frequency (0.15 Hz) relative to size for any fish species, leading researchers to question how they might catch much faster-moving prey (Watanabe et al., 2012). While scavenging behavior has been well documented (e.g., inertial suction feeding from a demersal pot, Grant et al., 2018; photo evidence of Greenland sharks feeding on carrion at the surface, MacNeil et al., 2012; see section “Diet and Trophic Ecology”), direct observations of active predation have yet to be recorded. Despite this fact, several lines of evidence, including freshly ingested seal remains in Greenland shark stomachs and bite marks observed on live seals and overwintering beluga whales (Delphinapterus leucas), suggest that active predation on fast-moving prey like marine mammals does occur (Fisk et al., 2002; Leclerc et al., 2012; MacNeil et al., 2012; Nielsen et al., 2014). Members of the Inuit community of Pangnirtung (Nunavut, Canada) have also documented Greenland sharks scavenging seals and beluga from nets and have reported their belief in the shark’s ability to capture and consume newborn seal pups (based on whole specimens found in shark stomachs) and to attack adult seals visiting breathing holes in the ice (from observations of bite wounds; Idrobo and Berkes, 2012). Given their observed slow swimming speed, it is hypothesized that sharks may adopt a stealth approach to target seals when they rest underwater or at the surface (Skomal and Benz, 2004; Watanabe et al., 2012).
With the vision of Greenland sharks in the Arctic considered to be impaired by copepod parasites (Ommatokoita elongata; Berland, 1961; Borucinska et al., 1998; Skomal and Benz, 2004; Figure 4B) and their slow swim speeds (Watanabe et al., 2012), the mechanism of active predation remains unknown. Consequently, the primary role of this species as a predator or scavenger of marine mammals has not been established (Bigelow and Schroeder, 1948; Ridoux et al., 1998; Lucas and McAlpine, 2002; Horning and Mellish, 2014), despite the importance of this information to our understanding of their true ecological role.
Figure 4. Examples of Greenland shark physiological studies: (A) Eye with exposed cornea (photo © Julius Nielsen, used with permission). (B) Eye with parasitic copepod (O. elongata; photo © Gregory Skomal, used with permission). (C) Attachment of electrodes to heart (photo © Holly Shiels, used with permission). (D) Attachment of electrodes to the musculature (photo © Julius Nielsen, used with permission). (E) Shark and respirometry tank setup (photo © John Fleng Steffensen, used with permission). (F) Shark in respirometry swimming pool setup (photo © Eric Ste Marie, used with permission).
Another key question concerns the feeding frequency of Greenland sharks, particularly in relation to their metabolic rate and energy budgets. Given their large body mass, ectothermic physiology, and cold water habitat, these sharks are likely to have extremely low mass-specific metabolic rates as a result of slow growth, long life spans (Nielsen et al., 2016), and slow swimming speeds (Watanabe et al., 2012). Seals provide a highly energy-rich food due to their large body size and high fat content (Stirling and McEwan, 1975; Addison and Stobo, 1993; Lucas and Natanson, 2010). It is possible, therefore, that Greenland sharks could survive for several months without feeding following the consumption of such energy-dense prey. The consumption rate of teleost prey (e.g., Atlantic cod, Gadus morhua, or Greenland halibut; Nielsen et al., 2014) by Greenland sharks may also be much lower than that of other large sharks (time scale of days rather than hours).
The frequent vertical movements exhibited by Greenland sharks raise additional questions over the physiological and metabolic costs of inhabiting and transitioning between shallow and deep-water environments and the factors influencing such movements. Deep-sea sharks rely on a high liver mass to provide energy storage for ovary production during maturation, however, at shallow depths, this large proportion of fatty tissue (relative to body mass) results in positive buoyancy, potentially leading to a higher energetic requirement during descent (Nakamura et al., 2015). Further insight into the energetic costs of these movements may help to resolve questions regarding the frequency of feeding and quantity of prey intake required to maintain them.
To address questions surrounding feeding mechanisms and frequency, direct observation of interactions and hunting behavior using animal-borne video cameras (e.g., Shark Tag camera; Kukulya et al., 2015) or other technologies (e.g., active sonar) are needed. In recent years, telemetry advances have increasingly allowed indirect observations of animals in remote environments (Hussey et al., 2015b). For instance, active predation of pinnipeds by Pacific sleeper sharks was inferred from post-mortem temperature records collected by dual life history tags (LHX tags) implanted in Stellar sea lions (Horning and Mellish, 2014). Camera-bearing AUVs, such as Remote Environmental Monitoring UnitS (REMUS), have provided unique observations of the feeding behavior of white sharks in situ (Skomal et al., 2015) and could be easily adapted to monitor Greenland sharks. Several other technologies could provide indirect methods of recording Greenland shark interactions with conspecifics and potential prey. For example, through the deployment of hydrophones that record ambient noise on sharks (D-tag or Acousonde; Oleson et al., 2010), it may be possible to assess shark proximity to vocalizing marine mammals (sperm whale, Physeter macrocephalus, narwhal, Monodon monoceros, and ringed seal, Pusa hispida). In addition, recoverable VMT packages or satellite-linked units deployed on Greenland sharks (see section “Movement Ecology”) could provide data on co-occurrence and the potential rate of interactions among tagged sharks, and between sharks and potential prey species equipped with acoustic tags (e.g., Greenland halibut, Arctic skate, Amblyraja hyperborea, and narwhal; Broell et al. personal communication).
The combined use of accelerometers and animal-borne cameras on individual sharks provides one possible approach to examine the cost of vertical movement and determine the feeding frequency of Greenland sharks. For example, following the use of this technology on two deep-water species (bluntnose sixgill shark, Hexanchus griseus, and prickly shark, Echinorhinus cookei), researchers suggested that the positive buoyancy exhibited by sharks in shallow water may facilitate upward migrations or hunting near the surface, but may also lead to variable costs related to the habitat depth occupied. While this approach can help to infer the energetic costs associated with vertical movement (including potential foraging behaviors), to fully understand the energetic requirements of this species it will also be necessary to record fine-scale foraging behavior (actual feeding events) for sufficient periods of time (days to weeks). For example, acceleration-triggered video cameras allowed the identification of the individual feeding attempts of deep-diving elephant seals (Naito et al., 2017), providing a promising approach to capture low-frequency events given technological constraints (e.g., logging duration of video tags and attachment of large tag packages for long durations). Modified pop-up archival tags that are placed in the stomach of the animal (through ingestion with bait) and record stomach pH (Papastamatiou et al., 2007) or bulk electrical impedance and stomach temperature (Meyer and Holland, 2012) also provide unique methods to address this question.
Direct observations of feeding behavior on free ranging prey and interactions with various forms of fishing gear will provide further insight into the development of deterrents, gear adaptations, and bait restrictions to reduce Greenland shark bycatch. Importantly, fine-scale movement data recorded by accelerometers attached to fishing gear or deployed on animals can also be used to assess behavioral responses to fisheries capture (Gallagher et al., 2016; Bouyoucos et al., 2017) and can provide evidence of post-release recovery and survival following capture and release (Brownscombe et al., 2013; Table 1). This method (using time-series vertical dive data recorded by animal-borne accelerometers) is currently being used to determine survival rates and the time required for Greenland sharks to return to normal behaviors following release from capture by bottom longlines (Watanabe et al., personal communication).
Due to the Greenland shark’s large size and remote habitat, which have precluded sophisticated in vivo experimentation and captive studies, many studies are conducted under field conditions that favor experiments on isolated tissues rather than whole animals. As a result, the basic physiology of the Greenland shark remains largely a mystery (MacNeil et al., 2012; Herbert et al., 2017).
While the cardiovascular system underlies every facet of an animal’s life, relatively few studies have examined this in the Greenland shark. A recent study on the whole blood of Greenland sharks noted that their blood properties (high oxygen affinity and low Bohr effect) were typical of sluggish elasmobranchs (Herbert et al., 2017). However, using isolated haemoglobins, the blood was found to have a relatively low affinity and consist of three hemoglobin isoforms with no functional differences (Russo et al., 2017). This analysis suggested that cellular changes in allosteric effectors may be important for controlling the O2 transport properties of the blood. Additionally, in an investigation of the stress response of Greenland sharks caught on bottom longlines, capture-induced changes in blood glucose and lactate values were found to be variable and were weakly related to capture depth (lactate) and body length (glucose; Barkley et al., 2017).
Characteristics of the Greenland shark’s heart and blood vessels are also beginning to emerge. Greenland sharks were found to have a low intrinsic heart rate paired with a high ventricular volume (measured ex vivo; Shiels et al., 2018) (Figure 4C). Histological analysis of heart samples showed significant fibrosis that increased with age; a preliminary analysis revealed no evidence of atherosclerosis. Efforts to quantify details of heart performance using isolated cardiac heart strips (Larsen et al., 2017) have proved to be largely unsuccessful as the tissue does not remain viable for long periods. In heart strips that appeared to be contracting normally, a single contraction/relaxation cycle (i.e., a twitch) required 3–5 s to complete at 5°C, suggesting that maximal heart rate ranges from 12 to 20 beats/min (in agreement with Shiels et al., 2018). While blood pressure in Greenland sharks has never been directly measured, it has been estimated by analyzing the relative amounts of elastin and collagen in the wall of the ventral aorta and measuring its compliance characteristics over a range of pressures (Shadwick et al., 2018). These data suggest that the Greenland shark’s average blood pressure is approximately 2.3–2.8 kPa, much lower than other slow swimming sharks, such as the epaulet shark, Hemiscyllium ocellatum (3.9 kPa), or catshark, Scyliorhinus canicula (5.3 kPa), where it has been directly measured (Taylor et al., 1977; Speers-Roesch et al., 2012).
Further physiological studies have largely focused on systems which are likely to play an important role in foraging behaviors. Olfactory cues are typically important for locating prey, especially where visual cues are limited. An anatomical study of the olfactory rosette in the Greenland shark indicated that while the arrangement of the olfactory lamellae and epithelium are similar to those found in benthic∖slow swimming animals, the relatively large olfactory epithelium surface area is more reflective of a bentho-pelagic animal (Ferrando et al., 2016).
Preliminary biochemical assays and work loops (force vs. length curves used to determine the mechanical work of muscle fibers) indicate that both red and white skeletal muscle fibers have very low metabolic capacities and contraction (twitch) rates (Bernal personal communication). This suggests that they are unable to sustain high levels of either anaerobic or aerobic muscle contraction for extended periods. These data consequently raise questions over how Greenland sharks actively forage in the water column for teleosts and marine mammals (Fisk et al., 2012; MacNeil et al., 2012; Nielsen et al., 2014, see section “Behavior”).
While sharks, in general, have good vision, little is known about sharks’ visual adaptations to their environment and how this is impacted by their ecology and habitat (McComb et al., 2010). Greenland sharks are thought to have poor vision, potentially as a result of corneal damage produced by the attachment of the parasitic copepod, O. elongata (Berland, 1961; Borucinska et al., 1998; Figure 4B) as well as the dark environment they inhabit (300–500 m; Nielsen et al., 2014). In the absence of active parasitism, sharks may still possess scar tissue on the cornea from previous infections, however, no evidence of a correlation between shark size/age and degree of corneal damage has yet been recorded (Nielsen personal communication). Additionally, while a high incidence of copepod parasitism has been reported at high latitudes in the Eastern Canadian Arctic and off eastern and western Greenland (∼90% of sharks showing parasitism, Steffensen personal communication), a much lower incidence of active parasitism has been recorded in the St. Lawrence Estuary and in waters off southern Atlantic Canada (<10% of observed sharks, Gallant personal communication, and no parasitized sharks observed, Campana personal communication, respectively). Despite this observed spatial disparity in copepod presence/absence, the degree of corneal damage present in sharks from these discrete locations has yet to be examined thoroughly. Systematic investigations of this disparity, and of the cumulative effects of multiple parasitic infections on corneal structure and vision are thereby required. Furthermore, novel records of vision-associated behaviors (defensive posturing) performed by non-parasitized sharks in shallow waters (St. Lawrence Estuary; Harvey-Clark et al., 2005), and evidence to suggest that Greenland sharks are potential active predators (Watanabe et al., 2012; Nielsen et al., 2014), further warrant a thorough understanding of their visual system.
Despite the limitations precluding in vivo experimentation, future anatomical studies and in vitro experiments will lead to important insights with regards to whole animal function. For example, studies directed at defining skeletal muscle characteristics (fiber type, distribution, contraction rates and pH buffering properties; Figure 4D), and cardiorespiratory characteristics (e.g., heart rate, stroke volume, blood pressure, ventilation rate, ventilation volume, extraction efficiency, and blood buffering capacity; Figure 4C) will be important in building a complete picture of the Greenland shark as a top predator in the Arctic ecosystem. Understanding these physiological attributes is also key for improving knowledge of the mechanisms driving longevity.
Established in vitro techniques will also facilitate future examination of the visual system in Greenland sharks (Figure 4A). Approaches such as electroretinography could be used to determine spectral/luminous sensitivities and temporal resolution (Kalinoski et al., 2014), while microspectrophotometry can elucidate the type and distribution of visual pigments (Hart et al., 2005). Of particular interest, given the long-lived nature of this species, is the study of ontogenetic changes in brain organization that might occur over 200 years, potentially altering sensory acuity and therefore the relative importance of senses such as vision and olfaction over individual lifespans (Lisney et al., 2017).
Further study of the importance of olfaction and electroreception for prey location will provide insight into the foraging behavior of Greenland sharks, including factors affecting incidental capture by fishing gear. Specifically, age-related changes in the olfactory epithelium could be examined given documented changes in other species (Ferrando et al., 2016). Greenland sharks also appear to have a well-developed network of electroreceptors (Ampullae of Lorenzini) that allow them to detect the bio-electrical activity of animals at close range (Kalmijn, 1971), but their arrangement has yet to be described. Given the lack of response exhibited by Greenland sharks presented with electropositive metals (Grant et al., 2018), further study of the sensitivity of their electroreceptors may be beneficial for the development of effective hook deterrents to mitigate bycatch.
Understanding the role of the Greenland shark as a potential apex predator also requires accurate measurement of its metabolic rate and scope (aerobic and anaerobic) during resting, swimming, and digestion cycles (Figures 4E,F). In addition to establishing rates of energy expenditure for these activities, metabolic rate has also been shown as a determinant of life span based on temperature and body size (Gillooly et al., 2001). Preliminary studies on ∼250 kg Greenland sharks found that they did not have an unusual metabolic rate (Steffensen et al., personal communication; Figure 4E) compared to what could be expected given its large size and body temperature. More metabolic studies are warranted, particularly comparing the resting metabolic rate of starved vs. fed sharks to evaluate the time course and energy expenditure associated with digestion (Specific Dynamic Action, SDA; Sims and Davies, 1994; Ferry-Graham and Gibb, 2001).
A greater understanding of these physiological traits and energetic requirements will ultimately lead to improved species management for both Greenland sharks, and their commercially targeted prey species. Specifically, there is a fundamental need for baseline (unstressed) values for virtually all blood parameters from Greenland sharks and for the accurate quantification of capture stress. Furthermore, understanding the bioenergetic requirements of individual Greenland sharks (via field measurements of metabolic rate and direct observations of consumption rate, see section “Behavior”) will help to estimate the energetic needs of sharks at the population level. This could be crucial for informing harvest levels (e.g., Total Allowable Catches, TAC) of targeted Greenland shark prey species to ensure that the remaining biomass is resilient to sustained fishing effort (Table 1).
The diet of Greenland sharks includes an impressive diversity of prey (Yano et al., 2007; McMeans et al., 2010; Leclerc et al., 2012; Nielsen et al., 2014; Nielsen, 2017). For example, stomachs of 39 Greenland sharks from western Greenland waters contained 25 different fish species, at least 3 pinniped species, and several groups of invertebrates (molluscs, echinoderms, decapods; Yano et al., 2007). Live prey capture is possible (see section “Behavior”), however, scavenging of marine mammal carrion (Williamson, 1963; Beck and Mansfield, 1969; Leclerc et al., 2011) and cannibalism of conspecifics captured in fishing gear (Jensen, 1948; Nielsen et al., 2014) are known to occur. Several reports indicate that Greenland sharks consume both benthic and pelagic prey (MacNeil et al., 2012), and the simultaneous occurrence of both prey types in the diet of Greenland sharks is consistent with diurnal behavior observed using biotelemetry (Campana et al., 2015a). Spatial variations in the relative importance of pelagic prey (i.e., a higher proportion of pelagic fishes in shark stomachs from Iceland vs. Canadian Arctic) have also been reported, however, this may reflect differences in sampling method (gillnet and trawl vs. bottom longline) or LT (mean ± S.E. 416 ± 25; 284 ± 44 cm, respectively; Fisk et al., 2002), rather than true dietary variations (MacNeil et al., 2012). Stable isotopes, fatty acids, and biomagnifying contaminants confirm a high trophic position and consumption of diverse prey types (Fisk et al., 2002; McMeans et al., 2010, 2015; Hussey et al., 2014). Geographical variation in diet further suggests that Greenland sharks exhibit a flexible response to variation in prey availability (McMeans et al., 2013a; Nielsen et al., 2014). However, some prey selectivity may occur because some abundant fish species (e.g., redfish; Sebastes spp.) are rarely present in shark stomachs from Greenland waters (Nielsen et al., personal communication). Stomach contents and biochemical markers also provide evidence that larger sharks consume more teleost and marine mammal prey compared to smaller sharks (less than 200 cm LT; Yano et al., 2007; McMeans et al., 2013a; Nielsen, 2017), which have been found to feed predominantly on lower trophic level prey such as squid (Nielsen, 2017).
Greenland sharks are mobile, opportunistic top predators that obtain and couple energy from multiple habitats within an ecosystem (McMeans et al., 2013b) and across geographically distant ecosystems (see section “Movement Ecology”). Although their effect on food web dynamics has yet to be quantified, existing food web theory suggests that mobile, opportunistic top predators are important for food web stability (McCann et al., 2005; Rooney et al., 2006). Empirical examples for this theory include Atlantic cod (G. morhua), which are thought to stabilize the ecosystem by preventing oscillatory, overshoot dynamics in their prey (Frank et al., 2011). Given their broad distribution and potential capacity for active predation (see section “Behavior”), Greenland shark populations could play a similar role in marine food webs, despite expected slow metabolism and low consumption rates (see section “Behavior”). While it has been suggested that Greenland sharks may be a significant source of mortality for some seal populations (Leclerc et al., 2012), the effects of consumption by Greenland sharks on prey populations must be examined further. This is of particular importance, as facultative scavenging may amplify the top-down trophic effects exerted by Greenland sharks on their food web because high-quality carrion can inflate predator biomass and increase a predator’s capacity for prey control (e.g., in wolves; Wilson and Wolkovich, 2011). As both potential predators and scavengers of many prey taxa, Greenland sharks could therefore have widespread effects on food webs throughout the Arctic and North Atlantic. A lack of evidence to define potential sub-population dietary specialization, as well as further uncertainty surrounding the role of extreme longevity in nutrient and energy transfer in marine food webs also indicate the potential value of more detailed study of the trophic role of Greenland sharks in Arctic marine environments.
Future work will need to quantify the type (active predation vs. scavenging) and frequency of trophic interactions between Greenland sharks and their prey (Table 1). Video observations and tracking technology (see section “Behavior”), combined with non-lethal sampling of tissues (blood and muscle) for dietary analysis (stable isotopes, amino acid compound specific isotope analysis, and fatty acids) will allow a more comprehensive understanding of Greenland shark diet and their functional role within food webs without the need to sacrifice individuals. Through a combination of accurate estimates of diet, field measurements of consumption and metabolic rates (see sections “Behavior” and “Physiological Adaptations,” respectively), detailed movement behaviors (see sections “Movement Ecology” and “Behavior”) and abundance estimates (see section “Demographics and Life History”), it will be possible to quantify energy flow and trophic transfer efficiencies, shedding light on Arctic food web dynamics (e.g., biomass structure – pyramid/inverted pyramid and interaction strengths) that may prove important for understanding past and future food web stability (McCann et al., 2005). Geographical comparisons of biomass structure (the distribution of biomass across trophic levels) could be undertaken to study how variation in Greenland shark abundance regulates these food webs. A particularly exciting possibility is to synthesize food web data (i.e., predator prey relationships and abundance data through time), which can then be used to estimate indices of stability (e.g., the coefficient of variation of prey population dynamics; Korpimäki, 1984). Such an effort would provide quantitative data on how Greenland shark density and foraging behavior (e.g., the extent of cross habitat foraging) influences food web dynamics.
Ecosystem models (EcoPath and EcoSim) can help predict the effects of various fisheries management decisions (and associated changes in fishing pressure) on marine food webs (Travers et al., 2010). Through these models, diet and abundance metrics can be used to predict the potential effects of changes in Greenland shark abundance on prey species (including those affected by commercially harvest, e.g., Greenland halibut). Bycatch limits for Greenland sharks could then be set at levels to ensure that the remaining shark biomass is sufficient to maintain ecosystem function while also maintaining adequate prey biomass (Table 1).
Despite occupying great depths and remote, polar regions, Greenland sharks experience many of the same anthropogenic and climatic stressors as other long-lived marine species (e.g., fisheries, vessel traffic, seismic surveys, pollution, and climate change; Clarke and Harris, 2003; Halpern et al., 2008; Davis et al., 2013). This may be particularly true for sharks inhabiting coastal regions of the North and Norwegian seas and the North American eastern seaboard; areas that are predicted to be cumulatively impacted by numerous anthropogenic drivers of ecological change (Halpern et al., 2008). Importantly, given their K-selected nature, impacted Greenland shark populations will likely be slow to recover.
Of these threats, fisheries have the greatest impact on Greenland shark populations. Directed fisheries for Greenland sharks existed in Norway, Iceland and Greenland from the 1600s to the mid-1990s (Davis et al., 2013). Catch rates grew to 15,000 Greenland sharks per year by the end of the 18th century with a peak catch of 32,000 individuals in Greenland in 1910 (Davis et al., 2013). Greenland shark catches continue at much lower levels today (see section “Demographics and Life History”). This includes Greenland shark bycatch in fisheries targeting other species, particularly Greenland halibut or shrimp, which occurred during the period of directed fishing and have continued since (Davis et al., 2013). Bycatch is typically returned to the ocean, but the proportion of animals that survive varies with fishing gear type, fishing duration, and handling method. A recent examination of Greenland shark bycatch in Canada found that 36% of sharks caught in bottom trawls (Greenland halibut and northern shrimp fisheries) and 16% of sharks caught on benthic longlines (Greenland halibut fisheries) were already dead when released (North Atlantic Fisheries Organization [NAFO], 2018b,c) and the percentage of dead sharks increased with trawl duration (North Atlantic Fisheries Organization [NAFO], 2018c).
The threats posed by incidental capture are not limited to post-release mortality. Like many elasmobranchs, Greenland sharks are often viewed as competitors for commercially valuable species by harvesters. For example, Greenland sharks that feed from and are incidentally captured by bottom longlines can become entangled in the fishing gear and rolling behavior can result in the mainline becoming tightly wrapped around the caudal peduncle, complicating release efforts (Edwards personal observation; Figures 5A,B). For this reason, Greenland sharks are often considered a nuisance species in commercial fisheries and entangled individuals may be removed by severing the caudal fin (Davis et al., 2013). Historically, Greenland sharks in the Saguenay Fjord were hauled out as trophies, but now they must be released while still in the water. This law has been strictly enforced by the Department of Fisheries and Oceans Canada since 2006 (Gallant personal communication).
Figure 5. Incidental capture of Greenland sharks via scientific bottom longline. (A) Shark entangled in mainline around caudle peduncle. (B) Shark hooked by mouth (photos Jena Edwards).
Even in the absence of directed fisheries, the rate of interactions among Greenland sharks and commercial fishing gear can be expected to increase in coming years. Ongoing reductions in sea ice extent and duration in the Arctic and changes in the composition and productivity of Arctic marine communities (Clarke and Harris, 2003) are allowing existing fisheries (bottom longline, trawl, and gillnet) to expand northward into previously unfinished habitats and extend their fishing season, while also creating the potential for new fisheries to develop (Christiansen et al., 2014).
In addition to fisheries pressures, Greenland sharks can also be affected by chronic and acute changes in their environment from pollution, increased noise, and climate change; factors which are likely to affect most fish and marine mammals in the Arctic (Clarke and Harris, 2003; Huntington, 2009). Pollution from global atmospheric deposition, coastal communities, and vessels can affect Greenland sharks directly by impacting their physiological balances (e.g., heavy metals, hormones, toxins; Strid et al., 2007, 2010; Corsolini et al., 2014) or indirectly by altering ecosystem productivity which can lead to eutrophication and reduced oxygen availability (e.g., St. Lawrence hypoxic dead zone; Belley et al., 2010; Howarth et al., 2011). Alongside natural sounds from animals, tides and currents, anthropogenic noise from vessels and industrial development are increasing in the Arctic with unknown effects (Ivanova et al., 2018).
Mirroring this extensive list of potential threats to Greenland shark populations is a series of questions that must be addressed. In the absence of population size or demographic data, it is currently not possible to estimate the impacts of historical fisheries on Greenland shark populations (size, age, sex, or maturity trends), or to examine changes in population metrics (e.g., recovery rate) following the cessation of directed fishing. In addition, when examining the available commercial bycatch data, it is important to consider potential inaccuracies. Large animals such as Greenland sharks are typically not weighed. Instead, bycatch records are generally limited to counts and may include supplementary data such as individual lengths, sex, and status (alive or dead). When weights are recorded, they are often a best guess based on the experience of the observer at estimating the weight of fish in a net.
There is also insufficient data to accurately estimate post-release mortality rates for bycaught Greenland sharks and to determine the effects of key factors such as gear type. While post-release mortality rates of up to 50% have been recorded in other sharks (as measured with PSATs; Campana et al., 2015b), this is known to vary widely across species. Additional data will therefore be required to accurately estimate the effects of incidental capture on Greenland shark populations and determine handling practices that will ensure optimum survival rates (see section “Behavior”).
While the precise impacts of indirect threats such as noise pollution and seismic surveys on Greenland shark behavior and physiology have not been quantified, sharks may need to adapt to changes in prey availability in areas where seismic surveys are conducted. Vessel noise can alter both short and long-term habitat use decisions by animals, affecting overall species distributions (Sarà et al., 2007; Slabbekoorn et al., 2010; McCarthy et al., 2011; Ivanova et al., 2018). For example, research in the Barents Sea has shown that seismic shooting can significantly affect fish distribution, local abundance, and catch rates (Engås et al., 1996). High intensity sounds from seismic surveys or pile driving could also have more direct impacts on individuals, causing sensory damage (and potentially death), leading to avoidance behaviors and changes in habitat use (Popper and Hastings, 2009).
Scientific efforts to increase our understanding of the many threats faced by Greenland shark populations and their surrounding ecosystems are underway. In an effort to improve the quality of data obtained from Greenland shark bycatch, DFO requested, on a trial basis, that at-sea observers use a statistical length-weight key to estimate Greenland shark weight based on a measured length, along with criteria to assess condition.
Changes to fishing gear (Grant et al., 2018) and practices such as limiting set duration (Cosandey-Godin et al., 2014) have been examined as methods to reduce Greenland shark bycatch mortality. However, testing of additional bycatch mitigation strategies (e.g., gear modifications such as modified mono-filament gangions) as well as the data required to monitor the status of Greenland shark populations (abundance and/or biomass indices, size and sex ratios; see section “Demographics and Life History”) will also be necessary for maintaining sustainable mortality levels. Bycatch records and video observations of Greenland sharks interacting with fishing gear (Grant et al., 2018) are invaluable for adapting gear to minimize the probability of shark capture or entanglement and to allow monitoring of the frequency of interactions. Similarly, data from telemetry and archival tags are revealing the horizontal and vertical movement patterns of Greenland sharks (see section “Movement Ecology”), which can be used to adapt fishing practices (e.g., harvester avoidance of high use areas, DAM plans; NOAA, 2002).
Continuing to articulate the link between threats and Greenland shark population(s) status in the scientific literature is useful for fishery management and marine spatial planning. Furthermore, regular communication of Greenland shark research results to managers, stakeholders, listing organizations, and the general public is crucial for raising awareness of the role of Greenland sharks in Arctic ecosystems, and for drawing attention to the need for their effective management. This can be achieved through various forms of outreach, including publications/reports, presentations, media (social, radio, print, and television), community posters, student engagement, and music videos.
Fisheries managed by Arctic countries, NAFO, and ICES apply the precautionary approach to fishery management (Food and Agricultural Organization [FAO], 1996; North Atlantic Fisheries Organization [NAFO], 2004; Department of Fisheries, and Oceans [DFO], 2006, 2009; ICES, 2012). While full stock assessments are typically limited to species that are commercially harvested, a similar level of analysis and management for key bycatch species is being considered in some jurisdictions (Department of Fisheries, and Oceans [DFO], 2013) and should be considered for the Greenland shark. Ecosystem-based fishery management (EBFM) involves managing fisheries with consideration of supporting ecosystem components and impacts on marine habitats (e.g., physical disruption and soundscape) and communities (e.g., direct mortality, competition with predators), in addition to commercial harvests and stock status (Pikitch et al., 2004; Trochta et al., 2018). Incorporating Greenland sharks into EBFM frameworks would require an accurate understanding of the shark’s role in the ecosystem and a model of community trophic linkages, data which are not currently available. EBFM also includes environmental drivers of ecosystem and animal productivity (i.e., to rebuild and sustain populations, species, biological communities, and marine ecosystems at high levels of productivity and biological diversity while providing food, revenue, and recreation for humans; U.S. National Research Council, 1998). The full application of the EBFM approach to fisheries with Greenland shark bycatch will therefore require new information regarding the nature and magnitude of each driver’s effects, both in isolation and in combination. Marine spatial planning that accounts for potential impacts on Greenland sharks will require the same data and analyses to be effective. In the meantime, multi-species stock assessment models provide one option to incorporate Greenland shark management actions into current directed fisheries management plans (Moffitt et al., 2016). Alternatively, trophic pyramid modeling provides a method in which catch limits are applied to trophic levels rather than individual species in an effort to reduce a fishery’s impacts on overall community structure (Garcia et al., 2012, 2014; Froese et al., 2015; Burgess et al., 2016). Results from such modeling exercises can inform management decisions and be incorporated in fishery-level documents such as the Integrated Fishery Management Plans that are developed for Canadian fisheries (see section “Management”). Fishery-level documents can then be referenced by and linked to marine spatial planning initiatives at national and international levels to achieve integration among management sectors and activities.
Laws and policies relevant to Greenland shark management occur at global, regional and national levels.
A fragmented array of global agreements and guidance documents have emerged to promote sustainable fisheries and marine biodiversity conservation in general (Russell and VanderZwaag, 2010; Techera and Klein, 2017), but shark-specific law and policy developments are limited to four main avenues (Davis et al., 2013). First, various shark species have been listed as endangered or threatened under the Convention on International Trade in Endangered Species of Wild Fauna and Flora (CITES) with resultant international trade restrictions imposed through export and import permit requirements (Hutton and Dickson, 2000; CITES, n.d.). The Greenland shark has not been considered for CITES listing due to its conservation status, being categorized as near threatened under the IUCN Red List of Threatened Species (IUCN, 2017), and lack of trade concerns.
Second, under the Convention on the Conservation of Migratory Species of Wild Animals (CMS), a Memorandum of Understanding on the Conservation of Migratory Sharks (Sharks MOU) has been adopted that encourages signatories to apply precautionary and ecosystem approaches to shark conservation (CMS, 2016). A Conservation Plan, included in Annex 3 to the Sharks MOU, sets out numerous implementation actions that should be prioritized by signatory states including: improving migratory shark research and monitoring; strengthening controls over directed and non-directed shark fisheries; ensuring the protection of critical habitats and migratory corridors; and increasing public awareness of the threats to sharks (CMS, 2016). However, the MOU has limited application, and is only applicable to 29 listed shark and shark-related species, which does not include the Greenland shark (CMS, 2018a). Additionally, the MOU only has 48 signatories (CMS, 2018b), which do not include key Arctic states such as Canada, Iceland, Norway, and the Russian Federation (CMS, 2018b).
Third, the Food and Agriculture Organization (FAO) has adopted an International Plan of Action for the Conservation and Management of Sharks (Food and Agricultural Organization [FAO], 1999), which encourages states to adopt national plans of action for the conservation and management of sharks in directed or non-directed fisheries. Such plans often seek to minimize the unutilized incidental catch of sharks, contribute to the protection of biodiversity and ecosystem structure and function, and facilitate improved species-specific catch and landings data. States are further encouraged to assess implementation of national plans at least every 4 years and to consider, where appropriate, the development of sub-regional or regional shark plans.
A fourth global avenue of specific shark-related law and policy development is through the UN General Assembly and its adoption of annual resolutions on sustainable fisheries, which include paragraphs urging states and RFMOs (see section “Movement Ecology”) to better protect sharks. For example, the sustainable fisheries resolution (Res. 72/72), adopted in December 2017, urges states to take multiple science-based measures to manage shark stocks including: setting limits on catch or fishing efforts, requiring the reporting of species-specific data on discards and landings, undertaking comprehensive stock assessments of sharks, and reducing shark bycatch and bycatch mortality. Regional Fisheries Management Organizations (RFMOs) with competence to regulate highly migratory species are urged to strengthen or establish precautionary, science-based, conservation and management measures for sharks.
A major regional development relevant to Greenland shark conservation was the conclusion in November 2017 of a draft Agreement to Prevent Unregulated High Seas Fisheries in the Central Arctic Ocean (U.S. Department of State, 2017). The Agreement, expected to be adopted by the five Arctic Ocean coastal states along with China, Iceland, Japan, South Korea, and the EU, aims to apply a precautionary approach to possible future commercial fisheries in the high seas of the central Arctic Ocean (CAO). The Agreement pledges parties to not authorize their flagged vessels to conduct commercial fishing in the CAO until conservation and management measures for fish stocks are adopted by one or more regional or sub-regional fisheries management organizations. Within 2 years of entry into force, parties agree to establish a Joint Program of Scientific Research and Monitoring with the objectives of improving understandings of ecosystems in the CAO and of determining whether fish stocks might exist that could be harvested on a sustainable basis. Party meetings are to be held every 2 years to review implementation of the Agreement, to review all available scientific information and to consider whether to commence negotiations for one or more additional regional or sub-regional fisheries management organizations or arrangements. The Agreement holds promise to substantially expand knowledge of the CAO and adjacent ecosystems and to provide refuge for Greenland sharks from potential unregulated fishing in the high Arctic.
Three North Atlantic regional fisheries management organizations have also addressed shark conservation in limited ways (Davis et al., 2013). The Northwest Atlantic Fisheries Organization (NAFO) has adopted general shark conservation and enforcement measures (North Atlantic Fisheries Organization [NAFO], 2018a). Shark finning is prohibited by requiring shark fins to remain attached to carcasses, and for non-directed shark catches, parties are required to encourage the live release of sharks (especially juveniles) that are not intended for use as food or subsistence. Parties are urged to undertake research in relation to sharks including: ways to make fishing gear more selective, key biological and ecological parameters, life history, behavioral traits and migration patterns, and the identification of potential mating, pupping, and nursery grounds of key shark species. Since 2012, NAFO has required, to the extent possible, species-specific reporting of shark catches in the NAFO Regulatory Area with the amount of Greenland sharks caught in 2013 reported as 22.2 tons (North Atlantic Fisheries Organization [NAFO], 2014) and rising to 203.2 tons in 2016 (North Atlantic Fisheries Organization [NAFO], 2017). Not all shark catches have been reported to the species level and it is not known how many shark species were simply lumped into the category of dogfishes (North Atlantic Fisheries Organization [NAFO], 2017).
NAFO’s Scientific Council, upon a request from the Commission, has been undertaking a review of the available information on the life history, population status, and current fishing mortality of Greenland sharks. Notably, at their most recent meeting held in June 2018, the Scientific Council (SC) made several suggestions, which included the prohibition of directed fishing and bycatch retention and the required release of live Greenland sharks captured within the NAFO Convention Area (North Atlantic Fisheries Organization [NAFO], 2018b). In addition, the SC recommended the promotion of safe handling practices by fishers, and that improvements be made to the reporting of all shark species by fisheries observers, including the collection of shark numbers, measurements (when feasible), and the recording of sex data and discard disposition (i.e., dead or alive; North Atlantic Fisheries Organization [NAFO], 2018b). To reduce bycatch, the application of gear restrictions and modifications, and/or spatial and temporal closures (where appropriate) was also recommended (North Atlantic Fisheries Organization [NAFO], 2018b). Approval of these suggestions by the Commission is pending, and further discussion was scheduled to take place at the annual NAFO meeting in September 2018 (North Atlantic Fisheries Organization [NAFO], 2018b).
The North-East Atlantic Fisheries Commission (NEAFC) has adopted shark conservation measures similar to those of NAFO. NEAFC also prohibits shark finning, encourages the live release of sharks caught in non-directed fisheries, and requires annual reporting of shark catches (NEAFC, 2015). NEAFC continues to prohibit directed fishing for deep sea sharks, including the Greenland shark, in NEAFC’s Regulatory Area and encourages parties to adopt prohibitions within national waters as well (NEAFC, 2017).
The International Commission for the Conservation of Atlantic Tunas (ICCAT) has adopted various recommendations relating to shark conservation over the years (Davis et al., 2013). Some of the most recent include recommendations on biological sampling for shark species where retention is prohibited (ICCAT, 2014) and on shortfin mako (Isurus oxyrinchus) porbeagle (Lamna nasus), and blue sharks (Prionace glauca) caught in association with ICCAT fisheries (ICCAT, 2013, 2015, 2016). No recommendations have been adopted specific to Greenland sharks, however, these are needed.
Besides a broad array of general legislative, regulatory, and policy provisions in support of sustainable fisheries and marine biodiversity protection (VanderZwaag et al., 2012; Sybersma, 2015), Canada adopted a National Plan of Action for the Conservation and Management of Sharks in 2007, which describes the Canadian legislative and regulatory framework for managing shark populations and fisheries, noting the importance of following precautionary and ecosystem approaches in future implementation (Fisheries, and Ocean Canada, 2007). Two limited references are made to the Greenland shark, noting the problem of Greenland shark bycatch and discards at sea in the Arctic region. The Plan commits Canada to move ahead with measures that increase knowledge about the life history and abundance of elasmobranchs in the Arctic, including the Greenland shark. Given the numerous knowledge gaps discussed in this paper, and the suspected implications of extreme longevity to limit the recovery of Greenland shark populations to disturbance, it is important that checks and balances are put in place to ensure that these research goals are met.
A Canadian progress report on implementation of the National Plan of Action for Sharks (NPOA-sharks), issued in 2012, provided little further information regarding Greenland shark conservation issues and efforts (Fisheries, and Oceans Canada, 2012). The progress report once again noted the bycatch and discarding reality in the Arctic and recognized the need for further research on the life history and abundance estimates. The report mentioned the completion of satellite tagging of Arctic shark species with results forthcoming. The report also noted that Canada was considering developing future projects to evaluate how changing Arctic conditions, such as climate change and increased shipping, may affect shark species.
An integrated fisheries management plan (IFMP) for one of Canada’s main commercial fisheries in the Arctic has addressed Greenland shark bycatch in limited ways. The IFMP for Greenland halibut, effective in 2014, noted the ongoing problem of inaccurate bycatch reporting and the need to improve bycatch management (Fisheries, and Oceans Canada, 2014). The IFMP listed Greenland shark as a species of concern, noted that the Committee on the Status of Endangered Wildlife in Canada (COSEWIC) had not yet assessed the conservation status of Greenland shark, and indicated the main bycatch management measure was to require release of incidental fish catches other than groundfish, and where alive, in a manner causing the least harm.
Organisms characterized by low productivity are particularly susceptible to overfishing and stock collapse and therefore are incapable of supporting more than nominal fishing mortality rates (Stevens et al., 2000). Despite historically high catch rates in some regions, Greenland sharks may be very sensitive to overfishing, even compared to other shark species. With recent evidence to support extreme longevity and conservative life history traits, coupled with their vulnerability to incidental capture by commercial fisheries, the sustainability of Greenland shark populations is of growing concern among fisheries management organizations. In spite of continued developments in the field of Greenland shark research, many questions still remain unanswered. To develop appropriate management actions aimed at preventing population declines, there is a current need to address these knowledge gaps through focused study and continued innovations in technology and experimental design. In this paper we identify key biological questions from several fields of Greenland shark research and, by drawing from recent tools and techniques from the broader literature, we propose a number of strategies which could be used to address these key questions. In particular, knowledge of the generation times, migration patterns, stock status, the locations of mating grounds and detailed information on reproduction of Greenland sharks will improve our ability to effectively manage this long-lived species. Importantly, vulnerability to population decline and the biological traits that influence it are common among long-lived species. Consequently, the critical parameters outlined in this paper and proposed methodologies to quantify them are likely applicable to most long-lived aquatic species. There is a need for researchers to use both traditional and creative approaches to engage stakeholders, build trust, and enhance collaboration, actions that will ultimately lead to improved management of this species, and long-lived species in general.
JE was responsible for contacting and coordinating the collaborating authors, and wrote the Abstract, Introduction, Methods, and Movement Ecology section (with NH). The Demographics section was written by BD, JN, and SC. Population genetics and genomics was written by RW and KP. Behavior and interactions was written by YW, FB, and GS. JS, PB, and JC wrote the Physiological adaptations section. Diet and trophic ecology was written by BM, JN, JC, and JS. The Threats section was written by KH, the outline was created by EH, and comments were provided by EH, YW, and SC. The Laws and Policies section was written by DV. Table 1, which formed the basis for the structure of this paper was created by EH and NH, and revised by JE. Final edits were completed by MM, JE, and NH. All authors contributed to the writing and editing of the final manuscript.
The authors declare that the research was conducted in the absence of any commercial or financial relationships that could be construed as a potential conflict of interest.
We would like to sincerely thank S. M. Grant, C. J. Harvey-Clark, and J. Bhak for their enthusiasm and feedback in the early stages of this work, and M. Treble for your insightful review which helped to refine this manuscript. We would also like to personally thank E. Davidson and S. Popov for their invaluable advice, assistance, and encouragement, as well as our reviewers whose constructive guidance fostered further improvement of this research. Additional support for NH was provided by an NSERC Discovery Grant.
Adams, P. B. (1980). Life history patterns in marine fishes and their consequences for fisheries management. Fish. Bull. 78, 1–12.
Addison, R. F., and Stobo, W. T. (1993). Organochlorine residue concentrations and burdens in grey seal (Halichoerus grypus) during the first year of life. J. Zool. Lond. 230, 443–450. doi: 10.1111/j.1469-7998.1993.tb02696.x
Ahonen, H., Harcourt, R. G., and Stow, A. J. (2009). Nuclear and mitochondrial DNA reveals isolation of imperilled grey nurse shark populations (Carcharias taurus). Mol. Ecol. 18, 4409–4421. doi: 10.1111/j.1365-294X.2009.04377.x
Baker, L. L., Jonsen, I. D., Mills, Flemming JE, Lidgard, D. C., Bowen, W. D., and Iverson, S. J. (2014). Probability of detecting marine predator-prey and species interactions using novel hybrid acoustic transmitter-receiver tags. PLoS One 9:e98117. doi: 10.1371/journal.pone.0098117
Ball, I. R., Possingham, H. P., and Watts, M. (2009). “Marxan and relatives: software for spatial conservation prioritisation,” in Spatial Conservation Prioritisation: Quantitative Methods And Computational Tools, eds A. Moilanen, K. A. Wilson, and H. P. Possingham (Oxford: Oxford University Press), 185–195.
Barker, M. J., and Schluessel, V. (2005). Managing global shark fisheries: suggestions for prioritizing management strategies. Aquat. Conserv. Mar. Freshw. Ecosyst. 15, 325–347. doi: 10.1002/aqc.660
Barkley, A. N., Cooke, S. J., Fisk, A. T., Hedges, K., and Hussey, N. E. (2017). Capture-induced stress in deep-water Arctic fish species. Polar Biol. 40, 213–220. doi: 10.1007/s00300-016-1928-8
Baum, J. K., Myers, R. A., Kehler, D. G., Worm, B., Harley, S. J., and Doherty, P. A. (2003). Collapse and conservation of shark populations in the Northwest Atlantic. Science 299, 389–392. doi: 10.1126/science.1079777
Beck, B., and Mansfield, A. W. (1969). Observations on the Greenland Shark, Somniosus microcephalus, in Northern Baffin Island. J. Fishe. Res. Board Can. 26, 143–145.
Belley, R., Archambault, P., Sundby, B., Gilbert, F., and Gagnon, J. M. (2010). Effects of hypoxia on benthic macrofauna and bioturbation in the Estuary and Gulf of St. Lawrence, Canada. Continent. Shelf Res. 30, 1302–1313. doi: 10.1016/j.csr.2010.04.010
Berland, B. (1961). Copepod Ommatokoita elongata (Grant) in the eyes of the Greenland shark—a possible cause of mutual dependence. Nature 191, 829–830. doi: 10.1038/191829a0
Bigelow, H. B., and Schroeder, W. C. (1948). Fishes of the Western North Atlantic, Part 1, Lancelets, Cyclostomes, Sharks. Mem. Sears Found. Mar. Res. 1, 29–58.
Binder, T. R., Holbrook, C. M., Miehls, S. M., Thompson, H. T., and Krueger, C. C. (2014). Use of oviduct-inserted acoustic transmitters and positional telemetry to estimate timing and location of spawning: a feasibility study in lake trout, Salvelinus namaycush. Anim. Biotelemetry 2:14. doi: 10.1186/2050-3385-2-14
Bjerkan, P. A. U. L. (1957). Notes on the Greenland Shark Acanthorhinus carcharias (Gunn). 1. The reproduction problem of the Greenland shark. Rep. Norwegian Fish. Mar. Investigat. 11, 1–7.
Borucinska, J. D., Benz, G. W., and Whiteley, H. E. (1998). Ocular lesions associated with attachment of the parasitic copepod Ommatokoita elongata (Grant) to corneas of Greenland sharks, Somniosus microcephalus (Bloch & Schneider). J. Fish Dis. 21, 415–422. doi: 10.1046/j.1365-2761.1998.00122.x
Bouyoucos, I. A., Suski, C. D., Mandelman, J. W., and Brooks, E. J. (2017). The energetic, physiological, and behavioral response of lemon sharks (Negaprion brevirostris) to simulated longline capture. Comp. Biochem. Physiol. A. 207, 65–72. doi: 10.1016/j.cbpa.2017.02.023
Brownscombe, J. W., Thiem, J. D., Hatry, C., Cull, F., Haak, C. R., Danylchuk, A. J., et al. (2013). Recovery bags reduce post-release impairments in locomotory activity and behavior of bonefish (Albula spp.) following exposure to angling-related stressors. J. Exp. Mar. Biol. Ecol. 440, 207–215. doi: 10.1016/j.jembe.2012.12.004
Burgess, M. G., Diekert, F. K., Jacobsen, N. S., Andersen, K. H., and Gaines, S. D. (2016). Remaining questions in the case for balanced harvesting. Fish Fish. 17, 1216–1226. doi: 10.1111/faf.12123
Campana, S. E., Casselman, J. M., and Jones, C. M. (2008a). Bomb radiocarbon chronologies in the Arctic, with implications for the age validation of lake trout (Salvelinus namaycush) and other Arctic species. Can. J. Fish. Aquat. Sci. 65, 733–743. doi: 10.1139/f08-012
Campana, S. E., Fisk, A. T., and Klimley, A. P. (2015a). Movements of Arctic and northwest Atlantic Greenland sharks (Somniosus microcephalus) monitored with archival satellite pop-up tags suggest long-range migrations. Deep Sea Res. II Top. Stud. Oceanogr. 115, 109–115. doi: 10.1016/j.dsr2.2013.11.001
Campana, S. E., Gibson, J., Brazner, J., Marks, L., Joyce, W., Gosselin, J.-F., et al. (2008b). Status of Basking Sharks in Atlantic Canada. Canadian Stock Assessment. Sec. Res. Doc. 2008/004. Dartmouth, NS: Fisheries and Oceans. doi: 10.1371/journal.pone.0082074
Campana, S. E., Joyce, W., Fowler, M., and Showell, M. (2015b). Discards, hooking, and post-release mortality of porbeagle (Lamna nasus), shortfin mako (Isurus oxyrinchus), and blue shark (Prionace glauca) in the Canadian pelagic longline fishery. ICES J. Mar. Sci. 73, 520–528. doi: 10.1093/icesjms/fsv234
Campana, S. E., Joyce, W., and Kulka, D. W. (2009). “Growth and reproduction of spiny dogfish off the eastern coast of Canada, including inferences on stock structure,” in Biology and Management of Dogfish Sharks, eds V. F. Gallucci, G. A. McFarlane, and G. G. Bargmann (Bethesda: American Fisheries Society), 195–208.
Christiansen, J. S., Mecklenburg, C. W., and Karamushko, O. V. (2014). Arctic marine fishes and their fisheries in light of global change. Glob. Change Biol. 20, 352–359. doi: 10.1111/gcb.12395
CITES. (n.d.). How CITES Works. Available at: https://www.cites.org/eng/disc/how.php
Clark, M. (2001). Are Deepwater Fisheries Sustainable? - the Example of Orange Roughy (Hoplostethus atlanticus) in New Zealand. Fish. Res. 51, 123–135. doi: 10.1016/S0165-7836(01)00240-5
Clarke, A., and Harris, C. M. (2003). Polar marine ecosystems: major threats and future change. Environ. Conserv. 30, 1–25. doi: 10.1098/rsbl.2016.0796
CMS (2016). Memorandum of Understanding on the Conservation of Migratory Sharks (as Amended by the Signatories at their Second Meeting, Costa Rica, February 2016). Available at: https://www.cms.int/sharks/en/page/sharks-mou-text
CMS (2018a). Memorandum of Understanding on the Conservation of Migratory Sharks: Signatories and Range Status. Available at: https://www.cms.int/sharks/en/meetings/meetings-of-signatories
CMS (2018b). Memorandum of Understanding on the Conservation of Migratory Sharks: Species. Available at: https://www.cms.int/sharks/en/species [accessed June 22, 2018].
Cooke, S. J., Iverson, S. J., Stokesbury, M. J., Hinch, S. G., Fisk, A. T., VanderZwaag, D. L., et al. (2011). Ocean Tracking Network Canada: a network approach to addressing critical issues in fisheries and resource management with implications for ocean governance. Fisheries 36, 583–592. doi: 10.1080/03632415.2011.633464
Corsolini, S., Ancora, S., Bianchi, N., Mariotti, G., Leonzio, C., and Christiansen, J. S. (2014). Organotropism of persistent organic pollutants and heavy metals in the Greenland shark Somniosus microcephalus in NE Greenland. Mar. Pollut. Bull. 87, 381–387. doi: 10.1016/j.marpolbul.2014.07.021
Cosandey-Godin, A., Krainski, E. T., Worm, B., and Flemming, J. M. (2014). Applying Bayesian spatiotemporal models to fisheries bycatch in the Canadian Arctic. Can. J. Fish. Aquat. Sci. 72, 186–197. doi: 10.1139/cjfas-2014-0159
Davis, B., VanderZwaag, D. L., Cosandey-Godin, A., Hussey, N. E., Kessel, S. T., and Worm, B. (2013). The conservation of the Greenland shark (Somniosus microcephalus): setting scientific, law, and policy coordinates for avoiding a species at risk. J. Int. Wildlife Law Policy 16, 300–330. doi: 10.1080/13880292.2013.805073
Department of Fisheries, and Oceans [DFO] (2006). A Harvest Strategy Compliant with the Precautionary. (Approach): Canadian Science Advisory Secretariat Science Advisory Report. Ottawa, ON: Fisheries and Oceans Canada. 23,2006,
Department of Fisheries, and Oceans [DFO] (2009). A Fishery Decision-Making Framework Incorporating the Precautionary Approach. Sustainable Fisheries Framework [website], (23 March 2009). Available at: http://www.dfo-mpo.gc.ca/reports-rapports/regs/sff-cpd/precaution-back-fiche-eng.htm
Department of Fisheries, and Oceans [DFO] (2013). Policy on Managing Bycatch. Sustainable Fisheries Framework [website], (8 January 2013). Available at: http://www.dfo-mpo.gc.ca/reports-rapports/regs/sff-cpd/bycatch-policy-prise-access-eng.htm
Department of Fisheries, and Oceans [DFO] (2016). Greenland Shark Bycatch Estimates in NAFO areas 0A and 0B. Dartmouth, NS: Bedford Institute of Oceanography.
Devine, B. M., Wheeland, L. J., and Fisher, J. A. (2018). First estimates of Greenland shark (Somniosus microcephalus) local abundances in Arctic waters. Sci. Rep. 8:974. doi: 10.1038/s41598-017-19115-x
Dudgeon, C. L., Pollock, K. H., Braccini, J. M., Semmens, J. M., and Barnett, A. (2015). Integrating acoustic telemetry into mark–recapture models to improve the precision of apparent survival and abundance estimates. Oecologia 178, 761–772. doi: 10.1007/s00442-015-3280-z
Engås, A., Løkkeborg, S., Ona, E., and Soldal, A. V. (1996). Effects of seismic shooting on local abundance and catch rates of cod ((Gadus morhua) and haddock) (Melanogrammus aeglefinus). Can. J. Fish. Aquat. Sci. 53, 2238–2249. doi: 10.1139/f96-177
Ferrando, S., Gallus, L., Ghigliotti, L., Vacchi, M., Nielsen, J., Christiansen, J. S., et al. (2016). Gross morphology and histology of the olfactory organ of the Greenland shark Somniosus microcephalus. Polar Biol. 39, 1399–1409. doi: 10.1007/s00300-015-1862-1
Ferry-Graham, L. A., and Gibb, A. C. (2001). Comparison of fasting and postfeeding metabolic rates in a sedentary shark, Cephaloscyllium ventriosum. Copeia 2001, 1108–1113. doi: 10.1643/0045-8511(2001)001[1108:COFAPM]2.0.CO;2
Fisheries, and Ocean Canada (2007). National Plan of Action for the Conservation and Management of Sharks. Ottawa, ON: Fish And Fisheries.
Fisheries, and Oceans Canada (2012). Canada’s Progress Report on the Implementation of Key Actions Taken Pursuant to the National Plan of Action on the Conservation and Management of Sharks (March 2007). Ottawa, ON: Fish And Fisheries.
Fisheries, and Oceans Canada (2014). Integrated Fishery Management Plan Greenland Halibut (Reinhardtius hippoglossoides) Northwest Atlantic Fisheries Organization Subarea O. Winnipeg, MB: Fish And Fisheries.
Fisk, A. T., Lydersen, C., and Kovacs, K. M. (2012). Archival pop-off tag tracking of Greenland sharks Somniosus microcephalus in the High Arctic waters of Svalbard, Norway. Mar. Ecol. Prog. Ser. 468, 255–265. doi: 10.3354/meps09962
Fisk, A. T., Tittlemier, S. A., Pranschke, J. L., and Norstrom, R. J. (2002). Using anthropogenic contaminants and stable isotopes to assess the feeding ecology of Greenland sharks. Ecology 83, 2162–2172. doi: 10.1890/0012-9658(2002)083[2162:UACASI]2.0.CO;2
Food and Agricultural Organization [FAO] (1996). Precautionary Approach to Fisheries. 1. Guidelines on the precautionary Approach to Capture Fisheries and Species Introductions. Rome: Food and Agricultural Organization.
Food and Agricultural Organization [FAO] (1999). International Plan of Action for the Conservation and Management of Sharks. Rome: Food and Agricultural Organization.
Francis, R. I. C. C. (1988). Maximum likelihood estimation of growth and growth variability from tagging data. N. Z. J. Mar. Freshwater Res. 22, 43–51. doi: 10.1080/00288330.1988.9516276
Frank, K. T., Petrie, B., Fisher, J. A., and Leggett, W. C. (2011). Transient dynamics of an altered large marine ecosystem. Nature 477:86. doi: 10.1038/nature10285
Fraser, D. J., and Bernatchez, L. (2001). Adaptive evolutionary conservation: towards a unified concept for defining conservation units. Mol. Ecol. 10, 2741–2752. doi: 10.1046/j.1365-294X.2001.t01-1-01411.x
Froese, R., Walters, C., Pauly, D., Winker, H., Weyl, O. L., Demirel, N., et al. (2015). A critique of the balanced harvesting approach to fishing. ICES J. Mar. Sci. 73, 1640–1650. doi: 10.1093/icesjms/fsv122
Gallagher, A. J., Staaterman, E. R., Cooke, S. J., and Hammerschlag, N. (2016). Behavioural responses to fisheries capture among sharks caught using experimental fishery gear. Can. J. Fish. Aquat. Sci. 74, 1–7. doi: 10.1139/cjfas-2016-0165
Gallant, J. J., Rodriguez, M. A., Stokesbury, M. J., and Harvey-Clark, C. (2016). Influence of environmental variables on the diel movements of the greenland shark (Somniosus microcephalus) in the St. Lawrence Estuary. Can. Field Nat. 130, 1–14. doi: 10.22621/cfn.v130i1.1784
Garcia, S. M., Bianchi, G., Charles, A., Kolding, J., Rice, J., Rochet, M.-J., et al. (2014). Balanced Harvest in the Real World. Scientific, Policy and Operational Issues in an Ecosystem Approach to Fisheries. Report of an international scientific workshop of the IUCN Fisheries Expert Group (IUCN/CEM/FEG) organized in close cooperation with the Food and Agriculture Organization of the United Nations (FAO), Rome, 29/09-02/10/2014. Available at: https://library.wur.nl/WebQuery/wurpubs/492588
Garcia, S. M., Kolding, J., Rice, J., Rochet, M. J., Zhou, S., Arimoto, T., et al. (2012). Reconsidering the consequences of selective fisheries. Science 335, 1045–1047. doi: 10.1126/science.1214594
García, V. B., Lucifora, L. O., and Myers, R. A. (2008). The importance of habitat and life history to extinction risk in sharks, skates, rays and chimaeras. Proc. R. Soc. Lond. B Biol. Sci. 275, 83–89. doi: 10.1098/rspb.2007.1295
Garde, E., Heide-Jørgensen, M. P., Hansen, S. H., Nachman, G., and Forchhammer, M. C. (2007). Age-specific growth and remarkable longevity in narwhals (Monodon monoceros) from West Greenland as estimated by aspartic acid racemization. J. Mammal. 88, 49–58. doi: 10.1644/06-MAMM-A-056R.1
George, J. C., Bada, J., Zeh, J., Scott, L., Brown, S. E., O’hara, T., et al. (1999). Age and growth estimates of bowhead whales (Balaena mysticetus) via aspartic acid racemization. Can. J. Zool. 77, 571–580. doi: 10.1139/z99-015
Gillooly, J. F., Brown, J. H., West, G. B., Savage, V. M., and Charnov, E. L. (2001). Effects of size and temperature on metabolic rate. Science 293, 2248–2251. doi: 10.1126/science.1061967
Grant, S. M., Sullivan, R., and Hedges, K. J. (2018). Greenland shark (Somniosus microcephalus) feeding behavior on static fishing gear, effect of SMART (Selective Magnetic and Repellent-Treated) hook deterrent technology, and factors influencing entanglement in bottom longlines. PeerJ 6:e4751. doi: 10.7717/peerj.4751
Gunnarsdottir, R., and Jørgensen, M. W. (2008). “Utilization possibilites of waste products from fishing and hunting to biogas and bio-oil production in Uummannaq County,” in Proceedings of the Sustainable Energy Supply in the Arctic-sun, wind, water etc.: ARTEK Event, (Copenhagen: Arctic Technology Centre), 52–58.
Halpern, B. S., Walbridge, S., Selkoe, K. A., Kappel, C. V., Micheli, F., D’agrosa, C., et al. (2008). A global map of human impact on marine ecosystems. Science 319, 948–952. doi: 10.1126/science.1149345
Hamady, L. L., Natanson, L. J., Skomal, G. B., and Thorrold, S. R. (2014). Vertebral bomb radiocarbon suggests extreme longevity in white sharks. PLoS One 9:e84006. doi: 10.1371/journal.pone.0084006
Hansen, P. M. (1963). Tagging experiments with the Greenland shark (Somniosus microcephalus (Bloch and Schneider)) in subarea 1. Int. Comm. Northwest Atl. Fish. Spec. Publ. 4, 172–175.
Hart, N. S., Lisney, T. J., Marshall, N. J., and Collin, S. P. (2005). Multiple cone visual pigments and the potential for trichromatic colour vision in two species of elasmobranch. J. Exp. Biol. 207, 4587–4594. doi: 10.1242/jeb.01314
Harvey-Clark, C. J., Gallant, J. J., and Batt, J. H. (2005). Vision and its relationship to novel behaviour in St. Lawrence River Greenland Sharks, Somniosus microcephalus. Can. Field Nat. 119, 355–358. doi: 10.22621/cfn.v119i3.145
Haulsee, D. E., Fox, D. A., Breece, M. W., Brown, L. M., Kneebone, J., Skomal, G. B., et al. (2016). Social network analysis reveals potential fission-fusion behavior in a shark. Sci. Rep. 6:34087. doi: 10.1038/srep34087
Heppell, S. S., Heppell, S. A., Read, A. J., and Crowder, L. B. (2005). Effects of Fishing on Long-Lived Marine Organisms. Marine Conservation Biology: the Science of Maintaining the sea’s Biodiversity. Washington, DC: Island Press,211–231.
Herbert, N. A., Skov, P. V., Tirsgaard, B., Bushnell, P. G., Brill, R. W., Clark, C. H., et al. (2017). Blood O 2 affinity of a large polar elasmobranch, the Greenland shark Somniosus microcephalus. Polar Biol. 40, 2297–2305. doi: 10.1007/s00300-017-2142-z
Herdendorf, C. E., and Berra, T. M. (1995). A Greenland shark from the wreck of the SS Central America at 2,200 meters. Trans. Am. Fish. Soc. 124, 950–953. doi: 10.1577/1548-8659(1995)124<0950:AGSFTW>2.3.CO;2
Heupel, M. R., Simpfendorfer, C. A., and Lowe, C. (2005). Passive Acoustic Telemetry Technology: Current Applications and Future Directions. Technical Report, No. 1066. Sarasota, FL: Mote Marine Laboratory,.
Hillary, R. M., Bravington, M. V., Patterson, T. A., Grewe, P., Bradford, R., Feutry, P., et al. (2018). Genetic relatedness reveals total population size of white sharks in eastern Australia and New Zealand. Sci. Rep. 8:2661. doi: 10.1038/s41598-018-20593-w
Holland, K. N., Meyer, C. G., and Dagorn, L. C. (2009). Inter-animal telemetry: results from first deployment of acoustic ‘business card’tags. Endanger. Species Res. 10, 287–293. doi: 10.3354/esr00226
Horning, M., and Mellish, J. A. E. (2014). In cold blood: evidence of Pacific sleeper shark (Somniosus pacificus) predation on Steller sea lions (Eumetopias jubatus) in the Gulf of Alaska. Fish. Bull. 112, 297–311. doi: 10.7755/FB.112.4.6
Howarth, R., Chan, F., Conley, D. J., Garnier, J., Doney, S. C., Marino, R., et al. (2011). Coupled biogeochemical cycles: eutrophication and hypoxia in temperate estuaries and coastal marine ecosystems. Front. Ecol. Environ. 9, 18–26. doi: 10.2307/41149673
Huntington, H. P. (2009). A preliminary assessment of threats to arctic marine mammals and their conservation in the coming decades. Mar. Policy 33, 77–82. doi: 10.1016/j.marpol.2008.04.003
Hussey, N. E., Cosandey-Godin, A., Walter, R. P., Hedges, K. J., VanGerwen-Toyne, M., Barkley, A. N., et al. (2015a). Juvenile Greenland sharks Somniosus microcephalus (Bloch & Schneider, 1801) in the canadian arctic. Polar Biol. 38, 493–504. doi: 10.1007/s00300-014-1610-y
Hussey, N. E., Kessel, S. T., Aarestrup, K., Cooke, S. J., Cowley, P. D., Fisk, A. T., et al. (2015b). Aquatic animal telemetry: a panoramic window into the underwater world. Science 348:1255642. doi: 10.1126/science.1255642
Hussey, N. E., MacNeil, M. A., McMeans, B. C., Olin, J. A., Dudley, S. F., Cliff, G., et al. (2014). Rescaling the trophic structure of marine food webs. Ecol. Lett. 17, 239–250. doi: 10.1111/ele.12226
Hussey, N. E., Orr, J., Fisk, A. T., Hedges, K. J., Ferguson, S. H., and Barkley, A. N. (2018). Mark report satellite tags (mrPATs) to detail large-scale horizontal movements of deep water species: First results for the Greenland shark (Somniosus microcephalus). Deep Sea Res. I Oceanogr. Res. Pap. 134, 32–40. doi: 10.1016/j.dsr.2018.03.002
Hutton, J. M., and Dickson, B. [eds]. (2000). Endangered species, threatened convention: the past, present and future of CITES, the Convention on International Trade in Endangered Species of Wild Fauna and Flora. London: Earthscan.
ICCAT (2013). Recommendation 13-10 on Biological Sampling of Prohibited Shark Species by Scientific Observers. Available at: https://www.iccat.int/Documents/Recs/compendiopdf-e/2013-10-e.pdf
ICCAT (2014). Recommendation 14-06 by ICCAT on Shortfin Mako Caught in Association with ICCAT Fisheries. Available at: https://www.iccat.int/Documents/Recs/compendiopdf-e/2014-06-e.pdf
ICCAT (2015). Recommendation 15-06 by ICCAT on Porbeagle Caught in Association with ICCAT Fisheries. Available at: https://www.iccat.int/Documents/Recs/compendiopdf-e/2015-06-e.pdf
ICCAT (2016). Recommendation 16-12 by ICCAT on Management Measures for the Conservation of Atlantic Blue Shark Caught in Association with ICCAT Fisheries. Available at: https://www.ccsbt.org/sites/default/files/userfiles/file/other_rfmo_measures/iccat/ICCAT_2016-12-e.pdf
ICES (2012). ICES Implementation of Advice for Data-Limited Stocks in 2012 in its 2012 Advice. ICES DLS Guidance Report 2012, ICES Advisory Committee. ICES CM 2012/ACOM 68. Available at: https://www.ices.dk/sites/pub/Publication%20Reports/Expert%20Group%20Report/acom/2012/ADHOC/DLS%20Guidance%20Report%202012.pdf
ICES (2017). Report of the North Western Working Group (NWWG). 27 April-4 May 2017. Copenhagen: ICES.
Idrobo, C. J., and Berkes, F. (2012). Pangnirtung inuit and the Greenland shark: co-producing knowledge of a little discussed species. Hum. Ecol. 40, 405–414. doi: 10.1007/s10745-012-9490-7
IUCN (2017). The IUCN Red List of Threatened Species. Available at: http://www.iucnredlist.org/details/60213/0
Ivanova, S. V., Kessel, S. T., Landry, J., O’Neill, C., McLean, M. F., Espinoza, M., et al. (2018). Impact of vessel traffic on the home ranges and movement of Shorthorn Sculpin (Myoxocephalus scorpius) in the nearshore environment of the high Arctic. Can. J. Fish. Aquat. Sci. 75, 2390–2400. doi: 10.1139/cjfas-2017-0418
Jensen, A. S. (1948). Contribution to the ichthyofauna of Greenland 8–24. Spolia. Zool. Mus.Haun. 9, 1–84.
Kalinoski, M., Hirons, A., Horodysky, A., and Brill, R. (2014). Spectral sensitivity, luminous sensitivity, and temporal resolution of the visual systems in three sympatric temperate coastal shark species. J. Comp. Physiol. A 200, 997–1013. doi: 10.1007/s00359-014-0950-y
Koefoed, E. (1957). Notes on the Greenland shark Acanthorhinus carcharias (Gunn). 2. A uterine foetus and the uterus from a Greenland shark. Rep. Norwegian Fish. Mar. Invest. 11, 8–12.
Korpimäki, E. (1984). Population dynamics of birds of prey in relation to fluctuations in small mammal populations in western Finland. Ann. Zool. Fennici 21, 287–293.
Kukulya, A. L., Stokey, R., Littlefield, R., Jaffre, F., Padilla, E. M. H., and Skomal, G. (2015). 3D Real-Time Tracking, Following and Imaging of White Sharks with an Autonomous Underwater Vehicle. OCEANS 2015-Genova. Available at: http://ieeexplore.ieee.org/document/7271546/
Larsen, J., Bushnell, P., Steffensen, J., Pedersen, M., Qvortrup, K., and Brill, R. (2017). Characterization of the functional and anatomical differences in the atrial and ventricular myocardium from three species of elasmobranch fishes: smooth dogfish (Mustelus canis), sandbar shark (Carcharhinus plumbeus), and clearnose skate (Raja eglanteria). J. Comp. Physiol. B 187, 291–313. doi: 10.1007/s00360-016-1034-9
Leclerc, L. M., Lydersen, C., Haug, T. A., Glover, K. T., Fisk, A., and Kovacs, K. (2011). Greenland sharks (Somniosus microcephalus) scavenge offal from minke (Balaenoptera acutorostrata) whaling operations in Svalbard (Norway). Polar Res. 30:7342. doi: 10.3402/polar.v30i0.7342
Leclerc, L. M. E., Lydersen, C., Haug, T., Bachmann, L., Fisk, A. T., and Kovacs, K. M. (2012). A missing piece in the Arctic food web puzzle? Stomach contents of Greenland sharks sampled in Svalbard, Norway. Polar Biol. 35, 1197–1208. doi: 10.1007/s00300-012-1166-7
Lennox, R. J., Aarestrup, K., Cooke, S. J., Cowley, P. D., Deng, Z. D., Fisk, A. T., et al. (2017). Envisioning the future of aquatic animal tracking: technology, science, and application. Bioscience 67, 884–896. doi: 10.1093/biosci/bix098
Li, H., Tian, C., Lu, J., Myjak, M. J., Martinez, J. J., Brown, R. S., et al. (2016). An energy harvesting underwater acoustic transmitter for aquatic animals. Sci. Rep. 6:33804. doi: 10.1038/srep33804
Lidgard, D. C., Bowen, W. D., Jonsen, I. D., and Iverson, S. J. (2012). Animal-borne acoustic transceivers reveal patterns of at-sea associations in an upper-trophic level predator. PLoS One 7:e48962. doi: 10.1371/journal.pone.0048962
Lidgard, D. C., Bowen, W. D., Jonsen, I. D., McConnell, B. J., Lovell, P., Webber, D. M., et al. (2014). Transmitting species-interaction data from animal-borne transceivers through Service Argos using Bluetooth communication. Methods Ecol. Evol. 5, 864–871. doi: 10.1111/2041-210X.12235
Lisney, T. J., Yopak, K. E., Camilieri-Asch, V., and Collin, S. P. (2017). Ontogenetic shifts in brain organization in the bluespotted stingray Neotrygon kuhlii (Chondrichthyes: Dasyatidae). Brain Behav. Evol. 89, 68–83. doi: 10.1159/000455223
Lucas, Z. N., and McAlpine, D. F. (2002). Extralimital occurrences of ringed seals, Phoca hispida, on Sable Island, Nova Scotia. Can. Field Nat. 116, 607–610.
Lucas, Z. N., and Natanson, L. J. (2010). “Two shark species involved in predation on seals at Sable Island, Nova Scotia, Canada,” in Proceedings of the Nova Scotian Institute of Science (NSIS) Nova Scotia.
MacNeil, M. A., McMeans, B. C., Hussey, N. E., Vecsei, P., Svavarsson, J., Kovacs, K. M., et al. (2012). Biology of the Greenland shark Somniosus microcephalus. J. Fish Biol. 80, 991–1018. doi: 10.1111/j.1095-8649.2012.03257.x
Martin, A. P., Naylor, G. J., and Palumbi, S. R. (1992). Rates of mitochondrial DNA evolution in sharks are slow compared with mammals. Nature 357:153. doi: 10.1038/357153a0
McCann, K., and Shuter, B. (1997). Bioenergetics of life history strategies and the comparative allometry of reproduction. Can. J. Fishe. Aquat. Sci. 54, 1289–1298. doi: 10.1139/f97-026
McCann, K. S., Rasmussen, J. B., and Umbanhowar, J. (2005). The dynamics of spatially coupled food webs. Ecol. Lett. 8, 513–523. doi: 10.1111/j.1461-0248.2005.00742.x
McCarthy, E., Moretti, D., Thomas, L., DiMarzio, N., Morrissey, R., Jarvis, S., et al. (2011). Changes in spatial and temporal distribution and vocal behavior of Blainville’s beaked whales (Mesoplodon densirostris) during multiship exercises with mid–frequency sonar. Mar. Mammal Sci. 27, 206–226. doi: 10.1111/j.1748-7692.2010.00457.x
McClellan, C. M., Read, A. J., Price, B. A., Cluse, W. M., and Godfrey, M. H. (2009). Using telemetry to mitigate the bycatch of long-lived marine vertebrates. Ecol. Appl. 19, 1660–1671. doi: 10.1890/08-1091.1
McComb, M., Frank, T. M., Hueter, R. E., and Kajiura, S. M. (2010). Physiological temporal resolution and spectral sensitivity of the visual system of three coastal shark species from different light environments. Physiol. Biochem. Zool. 83, 299–307. doi: 10.1086/648394
McMeans, B. C., Arts, M. T., and Fisk, A. T. (2015). Impacts of food web structure and feeding behavior on mercury exposure in Greenland Sharks (Somniosus microcephalus). Sci. Total Environ. 509, 216–225. doi: 10.1016/j.scitotenv.2014.01.128.
McMeans, B. C., Arts, M. T., Lydersen, C., Kovacs, K. M., Hop, H., Falk-Petersen, S., et al. (2013a). The role of Greenland sharks (Somniosus microcephalus) in an Arctic ecosystem: assessed via stable isotopes and fatty acids. Mar. Biol. 160, 1223–1238. doi: 10.1007/s00227-013-2174-z
McMeans, B. C., Rooney, N., Arts, M. T., and Fisk, A. T. (2013b). Food web structure of a coastal Arctic marine ecosystem and implications for stability. Mar. Ecol. Prog. Ser. 482, 17–28. doi: 10.3354/meps10278
McMeans, B. C., Svavarsson, J., Dennard, S., and Fisk, A. T. (2010). Diet and resource use among Greenland sharks (Somniosus microcephalus) and teleosts sampled in Icelandic waters, using δ13C, δ15N, and mercury. Can. J. Fish. Aquat. Sci. 67, 1428–1438. doi: 10.1139/F10-072
Meekan, M., Austin, C. M., Tan, M. H., Wei, N.-W. V., Miller, A., Pierce, S. J., et al. (2017). iDNA at sea: recovery of whale shark (Rhincodon typus) mitochondrial DNA sequences from the whale shark copepod (Pandarus rhincodonicus) confirms global population structure. Front. Marine Sci. 4:420. doi: 10.3389/fmars.2017.00420
Metcalfe, K., Vaz, S., Engelhard, G. H., Villanueva, M. C., Smith, R. J., and Mackinson, S. (2015). Evaluating conservation and fisheries management strategies by linking spatial prioritization software and ecosystem and fisheries modelling tools. J. Appl. Ecol. 52, 665–674. doi: 10.1111/1365-2664.12404
Meyer, C. G., and Holland, K. N. (2012). Autonomous measurement of ingestion and digestion processes in free-swimming sharks. J. Exp. Biol. 215, 3681–3684. doi: 10.1242/jeb.075432
Meyer, C. G., Papastamatiou, Y. P., and Holland, K. N. (2010). A multiple instrument approach to quantifying the movement patterns and habitat use of tiger (Galeocerdo cuvier) and Galapagos sharks (Carcharhinus galapagensis) at French Frigate Shoals. Hawaii. Mar. Biol. 157, 1857–1868. doi: 10.1007/s00227-010-1457-x
Milano, I., Babbucci, M., Cariani, A., Atanassova, M., Bekkevold, D., Carvalho, G. R., et al. (2014). Outlier SNP markers reveal fine-scale genetic structuring across E uropean hake populations (Merluccius merluccius). Mol. Ecol. 23, 118–135. doi: 10.1111/mec.12568
Moffitt, E. A., Punt, A. E., Holsman, K., Aydin, K. Y., Ianelli, J. N., and Ortiz, I. (2016). Moving towards ecosystem-based fisheries management: options for parameterizing multi-species biological reference points. Deep Sea Res. II 134, 350–359. doi: 10.1016/j.dsr2.2015.08.002
Moritz, C. (1994). Defining ‘evolutionarily significant units’ for conservation. Trends Ecol. Evol. 9, 373–375. doi: 10.1016/0169-5347(94)90057-4
Murray, B. W., Wang, J. Y., Yang, S. C., Stevens, J. D., Fisk, A., and Svavarsson, J. (2008). Mitochondrial cytochrome b variation in sleeper sharks (Squaliformes: Somniosidae). Mar. Biol. 153, 1015–1022. doi: 10.1007/s00227-007-0871-1
Musick, J. A. (1999a). Criteria to define extinction risk in marine fishes: the American Fisheries Society initiative. Fisheries 24, 6–14. doi: 10.1577/1548-8446(1999)024<0006:CTDERI>2.0.CO;2
Musick, J. A. (1999b). Ecology and conservation of long-lived marine animals. Am. Fish. Soc. Symp. 23, 1–10.
Musick, J. A., Berkeley, S. A., Cailliet, G. M., Camhi, M., Huntsman, G., Nammack, M., et al. (2000). Protection of marine fish stocks at risk of extinction. AFS policy statement. Fisheries 25, 6–8. doi: 10.1577/1548-8446(2000)025<0006:POMFSA>2.0.CO;2
Myers, R. A., and Worm, B. (2003). Rapid worldwide depletion of predatory fish communities. Nature 423, 280–283. doi: 10.1038/nature01610
Naito, Y., Costa, D. P., Adachi, T., Robinson, P. W., Peterson, S. H., Mitani, Y., et al. (2017). Oxygen minimum zone: an important oceanographic habitat for deep-diving northern elephant seals, Mirounga angustirostris. Ecol. Evol. 7, 6259–6270. doi: 10.1002/ece3.3202
Nakamura, I., Meyer, C. G., and Sato, K. (2015). Unexpected positive buoyancy in deep sea sharks, Hexanchus griseus, and a Echinorhinus cookei. PLoS One 10:e0127667. doi: 10.1371/journal.pone.0127667
NEAFC (2015). Recommendation 10:2015 on Conservation of Sharks Caught in Association with Fisheries Managed by the North-East Atlantic Fisheries Commission. Available at: http://www.iotc.org/cmm/resolution-1705-.-conservation-sharks-caught-association-fisheries-managed-iotc
NEAFC (2017). Recommendation 10:2017 on Conservation and Management Measures for Deep Sea Sharks in the NEAFC Regulatory Area for 2017 to 2019. London: NEAFC.
Nielsen, J. (2013). Age Estimation and Feeding Ecology of Greenland Sharks Somniosus microcephalus in Greenland waters. MSc. thesis, Aquatic Science and Technology, Danish Technical University, Denmark.
Nielsen, J. (2017). The Greenland shark (Somniosus microcephalus): Diet, Tracking and Radiocarbon Age Estimates Reveal the World’s Oldest Vertebrate. Doctoral dissertation, Department of Biology, Faculty of Science, University of Copenhagen, Denmark.
Nielsen, J., Hedeholm, R. B., Heinemeier, J., Bushnell, P. G., Christiansen, J. S., Olsen, J., et al. (2016). Eye lens radiocarbon reveals centuries of longevity in the Greenland shark (Somniosus microcephalus). Science 353, 702–704. doi: 10.1126/science.aaf1703
Nielsen, J., Hedeholm, R. B., Simon, M., and Steffensen, J. F. (2014). Distribution and feeding ecology of the Greenland shark (Somniosus microcephalus) in Greenland waters. Polar Biol. 37, 37–46. doi: 10.1007/s00300-013-1408-3
NOAA (2002). Taking of marine mammals incidental to commercial fishing operations; atlantic large whale take reduction plan regulations. US Department of Commerce. Fed. Regist. 67, 1133–1141.
Norse, E. A., Brooke, S., Cheung, W. W., Clark, M. R., Ekeland, I., Froese, R., et al. (2012). Sustainability of deep-sea fisheries. Mar. Policy 36, 307–320. doi: 10.1016/j.marpol.2011.06.008
North Atlantic Fisheries Organization [NAFO] (2004). NAFO. (Precautionary) Approach Framework, 2004 NAFO Fisheries Council Document, 04/18. Available at: https://www.nafo.int/Science/NAFO-Frameworks/NAFO-Precautionary-Approach
North Atlantic Fisheries Organization [NAFO] (2014). Annual Compliance Review 2014 (Compliance Report for Fishing Year 2013. Available at: https://www.nafo.int/Fisheries/Compliance
North Atlantic Fisheries Organization [NAFO] (2017). Annual Compliance Review 2017 (Compliance Report for Fishing Year 2016). Available at: https://www.nafo.int/Fisheries/Compliance
North Atlantic Fisheries Organization [NAFO] (2018a). Conservation and Enforcement Measures 2018. Available at: https://www.nafo.int/Fisheries/Conservation
North Atlantic Fisheries Organization [NAFO] (2018b). Report of the Scientific Council Meeting (1-14 June 2018; Halifax, Nova Scotia). Dartmouth, NS: NAFO. doi: 10.1051/alr:2008003
North Atlantic Fisheries Organization [NAFO] (2018c). Bryk, J.L., K.J. Hedges and M.A. Treble. Summary of Greenland Shark (Somniosus microcephalus) catch in Greenland Halibut (Reinhardtius hippoglossoides) fisheries and scientific surveys conducted in NAFO Subarea 0. Dartmouth: Northwest Atlantic Fisheries Organization. doi: 10.1086/282697
Oleson, E. M., Calambokidis, J., McKenna, M., and Hidebrand, J. (2010). “Blue whale behavioral response study & field testing of the new bioacoustic probe,” in Proceedings of the National Oceanic and Atmospheric Administration (Honolulu HI: International Tsunami Information Center). doi: 10.1126/science.1098222
Papastamatiou, Y. P., Meyer, C. G., and Holland, K. N. (2007). A new acoustic pH transmitter for studying the feeding habits of free-ranging sharks. Aquat. Living Resour. 20, 287–290. doi: 10.1111/j.1095-8649.2009.02319.x
Pikitch, E., Santora, C., Babcock, E. A., Bakun, A., Bonfil, R., Conover, D. O., et al. (2004). Ecosystem-based fishery management. Science 305, 346–347. doi: 10.1371/journal.pone.0022588
Popper, A. N., and Hastings, M. C. (2009). The effects of anthropogenic sources of sound on fishes. J. Fish Biol. 75, 455–489. doi: 10.1111/j.1095-8649.2009.02319.x
Porteiro, F. M., Sutton, T. T., Byrkjedal, I., Orlov, A., Heino, M. P., Menezes, G. M. M., et al. (2017). Fishes of the Northern Mid-Atlantic Ridge Collected During the MAR-ECO Cruise in June-July 2004. An Annotated Checklist. Available at: https://nsuworks.nova.edu/occ_facreports/102
Ridoux, V., Hall, A. J., Steingrimsson, G., and Olafsson, G. (1998). An inadvertent homing experiment with a young ringed seal, Phoca hispida. Mar. Mammal Sci. 14, 883–888. doi: 10.1038/nature04887
Rigby, C., and Simpfendorfer, C. A. (2015). Patterns in life history traits of deep-water chondrichthyans. Deep Sea Res. II 115, 30–40. doi: 10.1163/ej.9789004174405.i-545.9
Robbins, R., and Fox, A. (2012). Further evidence of pigmentation change in white sharks, Carcharodon carcharias. Mar. Freshwater Res. 63, 1215–1217. doi: 10.1371/journal.pone.0186181
Rooney, N., McCann, K., Gellner, G., and Moore, J. C. (2006). Structural asymmetry and the stability of diverse food webs. Nature 442:265. doi: 10.1134/S0032945213010128
Russell, D. A., and VanderZwaag, D. L. (2010). “The International Law and Policy Seascape Governing Transboundary Fisheries,” in Recasting Transboundary Fisheries Management Arrangements in Light of Sustainability Principles: Canadian and International Perspectives, eds A. Dawn, Russell, L. David, and VanderZwaag (Leiden: Martinus Nijhoff). doi: 10.1007/s12686-016-0676-y
Russo, R., Giordano, D., Paredi, G., Marchesani, F., Milazzo, L., Altomonte, G., et al. (2017). The Greenland shark Somniosus microcephalus—Hemoglobins and ligand-binding properties. PLoS One 12:e018618. doi: 10.1371/journal.pone.0186181
Rusyaev, S. M., and Orlov, A. M. (2013). Bycatches of the greenland shark Somniosus microcephalus (Squaliformes, Chondrichthyes) in the barents sea and the adjacent waters under bottom trawling data. J. Ichthyol. 53, 111–115. doi: 10.3354/meps331243
Santaquiteria, A., Nielsen, J., Klemetsen, T., Willassen, N. P., and Præbel, K. (2017). The complete mitochondrial genome of the long-lived Greenland shark (Somniosus microcephalus): characterization and phylogenetic position. Conserv. Genet. Resour. 9, 351–355. doi: 10.1242/jeb.186957
Santaquiteria, A. G. (2016). Mitogenomic Characterization and Phylogenetic Position of the Oldest Living Vertebrate Species - the Greenland shark (Somniosus microcephalus). MSc. thesis, UiT The Arctic University of Norway, Tromsø.
Sarà, G., Dean, J. M., d’Amato, D., Buscaino, G., Oliveri, A., Genovese, S., et al. (2007). Effect of boat noise on the behaviour of bluefin tuna Thunnus thynnus in the Mediterranean Sea. Mar. Ecol. Prog. Ser. 331, 243–253. doi: 10.1017/S0376892909990191
Shadwick, R. E., Bernal, D., Bushnell, P. G., and Steffensen, J. F. (2018). Blood pressure in the Greenland shark as estimated from ventral aortic elasticity. J. Exp. Biol. 221:jeb186957. doi: 10.1242/jeb.186957
Shiels, H. A., Kusu-Orkar, T.-E., Ahmad, S., Reid, B., Gurney, A., Bernal, D., et al. (2018). Cardiac structure of the world’s oldest vertebrate, the Greenland Shark. Paper Presented at Society Experimental Biology meeting, Florence. doi: 10.1007/s00227-004-1332-8
Simpfendorfer, C. A., and Kyne, P. M. (2009). Limited potential to recover from overfishing raises concerns for deep-sea sharks, rays and chimaeras. Environ. Conserv. 36, 97–103. doi: 10.1111/jfb.12828
Sims, D. W., and Davies, S. J. (1994). Does specific dynamic action (SDA) regulate return of appetite in the lesser spotted dogfish, Scyliorhinus caniculcla? J. Fish Biol. 45, 341–348. doi: 10.1016/j.tree.2010.04.005
Skomal, G. B., and Benz, G. W. (2004). Ultrasonic Tracking of Greenland Sharks, Somniosus Microcephalus, under Arctic Ice. Mar. Biol. 145, 489–498. doi: 10.1242/jeb.059667
Skomal, G. B., Hoyos-Padilla, E. M., Kukulya, A., and Stokey, R. (2015). Subsurface observations of white shark Carcharodon carcharias predatory behaviour using an autonomous underwater vehicle. J. Fish Biol. 87, 1293–1312. doi: 10.1111/jfb.12828
Slabbekoorn, H., Bouton, N., van Opzeeland, I., Coers, A., ten Cate, C., and Popper, A. N. (2010). A noisy spring: the impact of globally rising underwater sound levels on fish. Trends Ecol. Evol. 25, 419–427. doi: 10.1016/j.tree.2010.04.005
Speers-Roesch, B., Brauner, C. J., Farrell, A. P., Hickey, A. J., Renshaw, G. M., Wang, Y. S., et al. (2012). Hypoxia tolerance in elasmobranchs. II. Cardiovascular function and tissue metabolic responses during progressive and relative hypoxia exposures. J. Exp. Biol. 215, 103–114. doi: 10.1242/jeb.059667
Stevens, J. D., Bonfil, R., Dulvy, N. K., and Walker, P. A. (2000). The effects of fishing on sharks, rays, and chimaeras (chondrichthyans), and the implications for marine ecosystems. ICES J. Mar. Sci. 57, 476–494. doi: 10.1007/s00227-005-0061-y
Stewart, R. E. A., Campana, S. E., Jones, C. M., and Stewart, B. E. (2006). Bomb radiocarbon dating calibrates beluga (Delphinapterus leucas) age estimates. Can. J. Zool. 84, 1840–1852. doi: 10.1002/etc.330
Stirling, I., and McEwan, E. H. (1975). The caloric value of whole ringed seals (Phoca hispida) in relation to polar bear (Ursus maritimus) ecology and hunting behavior. Can. J. Zool. 8, 1021–1027. doi: 10.1016/j.marpolbul.2007.04.018
Stokesbury, M. J., Harvey-Clark, C., Gallant, J., Block, B. A., and Myers, R. A. (2005). Movement and environmental preferences of Greenland sharks (Somniosus microcephalus) electronically tagged in the St. Lawrence Estuary, Canada. Mar. Biol. 148, 159–165. doi: 10.1016/j.marpol.2015.07.008
Strid, A., Athanassiadis, I., Athanasiadou, M., Svavarsson, J., Päpke, O., and Bergman, Å (2010). Neutral and phenolic brominated organic compounds of natural and anthropogenic origin in northeast Atlantic Greenland shark (Somniosus microcephalus). Environ. Toxicol. Chem. 29, 2653–2659. doi: 10.1002/etc.330
Strid, A., Jörundsdóttir, H., Päpke, O., Svavarsson, J., and Bergman, Å (2007). Dioxins and PCBs in Greenland shark (Somniosus microcephalus) from the north-east Atlantic. Mar. Pollut. Bull. 54, 1514–1522. doi: 10.1163/9789004345515
Sybersma, S. (2015). Review of shark legislation in Canada as a conservation tool. Mar. Policy 61, 121–126. doi: 10.1016/j.jmarsys.2009.07.005
Taylor, E. W., Short, S. T., and Butler, P. J. (1977). The role of the cardiac vagus in the response of the dogfish Scyliorhinus canicula to hypoxia. J. Exp. Biol. 70, 57–75. doi: 10.1371/journal.pone.0190467
Techera, E. J., and Klein, N. (2017). International Law of Sharks: Obstacles, Options and Opportunities. Netherlands: Brill.
Travers, M., Watermeyer, K., Shannon, L. J., and Shin, Y. J. (2010). Changes in food web structure under scenarios of overfishing in the southern Benguela: comparison of the Ecosim and OSMOSE modelling approaches. J. Mar. Syst. 79, 101–111.
Trochta, J. T., Pons, M., Rudd, M. B., Krigbaum, M., Tanz, A., and Hilborn, R. (2018). Ecosystem-based fisheries management: perception on definitions, implementations, and aspirations. PLoS One 13:e0190467. doi: 10.1371/journal.pone.0190467
U.S. National Research Council (1998). A Report of the Committee on Ecosystem Management for Sustainable Fisheries. Washington, DC: National Academy Press. doi: 10.1002/ece3.3325
U.S. Department of State (2017). Meeting on High Seas Fisheries in the Central Arctic Ocean, 28-30 November 2017: Chairman’s Statement. Available at: https://www.state.gov/e/oes/ocns/opa/rls/276136.htm doi: 10.1007/BF00182340
VanderZwaag, D. L., Hutchings, J. A., Jennings, S., and Peterman, R. M. (2012). Canada’s international and national commitments to sustain marine biodiversity. Environ. Rev. 20, 312–352. doi: 10.1016/j.jembe.2012.04.021
Walter, R. P., Roy, D., Hussey, N. E., Stelbrink, B., Kovacs, K. M., Lydersen, C., et al. (2017). Origins of the Greenland shark (Somniosus microcephalus): Impacts of ice-olation and introgression. Ecol. Evol. 7, 8113–8125. doi: 10.1002/ece3.3325
Walters, C., and Maguire, J. J. (1996). Lessons for stock assessment from the northern cod collapse. Rev. Fish Biol. Fish. 6, 125–137. doi: 10.1016/j.tree.2010.12.011
Watanabe, Y. Y., Lydersen, C., Fisk, A. T., and Kovacs, K. M. (2012). The slowest fish: swim speed and tail-beat frequency of Greenland sharks. J. Exp. Mar. Biol. Ecol. 426, 5–11. doi: 10.1007/s10228-004-0244-4
Williamson, G. R. (1963). Common porpoise from the stomach of a Greenland shark. J. Fish. Res. Board Can. 20, 1085–1086. doi: 10.1111/j.1095-8649.2007.01308.x
Wilson, E. E., and Wolkovich, E. M. (2011). Scavenging: how carnivores and carrion structure communities. Trends Ecol. Evol. 26, 129–135. doi: 10.1016/j.tree.2010.12.011
Yano, K., Stevens, J. D., and Compagno, L. J. (2004). A review of the systematics of the sleeper shark genus Somniosus with redescriptions of Somniosus (Somniosus) antarcticus and Somniosus (Rhinoscymnus) longus (Squaliformes: Somniosidae). Ichthyol. Res. 51, 360–373.
Keywords: future directions, longevity, management, Somniosus microcephalus, Arctic ecosystem
Citation: Edwards JE, Hiltz E, Broell F, Bushnell PG, Campana SE, Christiansen JS, Devine BM, Gallant JJ, Hedges KJ, MacNeil MA, McMeans BC, Nielsen J, Præbel K, Skomal GB, Steffensen JF, Walter RP, Watanabe YY, VanderZwaag DL and Hussey NE (2019) Advancing Research for the Management of Long-Lived Species: A Case Study on the Greenland Shark. Front. Mar. Sci. 6:87. doi: 10.3389/fmars.2019.00087
Received: 14 August 2018; Accepted: 14 February 2019;
Published: 02 April 2019.
Edited by:
Rob Harcourt, Macquarie University, AustraliaReviewed by:
Heidi Dewar, Southwest Fisheries Science Center (NOAA), United StatesCopyright © 2019 Edwards, Hiltz, Broell, Bushnell, Campana, Christiansen, Devine, Gallant, Hedges, MacNeil, McMeans, Nielsen, Præbel, Skomal, Steffensen, Walter, Watanabe, VanderZwaag and Hussey. This is an open-access article distributed under the terms of the Creative Commons Attribution License (CC BY). The use, distribution or reproduction in other forums is permitted, provided the original author(s) and the copyright owner(s) are credited and that the original publication in this journal is cited, in accordance with accepted academic practice. No use, distribution or reproduction is permitted which does not comply with these terms.
*Correspondence: Jena E. Edwards, ZWR3YXIxMW5AdXdpbmRzb3IuY2E=
Disclaimer: All claims expressed in this article are solely those of the authors and do not necessarily represent those of their affiliated organizations, or those of the publisher, the editors and the reviewers. Any product that may be evaluated in this article or claim that may be made by its manufacturer is not guaranteed or endorsed by the publisher.
Research integrity at Frontiers
Learn more about the work of our research integrity team to safeguard the quality of each article we publish.