- 1School of GeoSciences, Grant Institute, University of Edinburgh, Edinburgh, United Kingdom
- 2School of Engineering, University of Edinburgh, Edinburgh, United Kingdom
- 3School of Materials, University of Manchester, Manchester, United Kingdom
The extent of marine litter and microplastic occurrence across ocean biomes and species remains poorly characterised, particularly in remote deep-water ecosystems. The present study in the East Mingulay Special Area of Conservation (a Marine Protected Area in the Sea of the Hebrides, western Scotland) used historic surveys and benthic samples to obtain baseline levels of anthropogenic debris and microparticle ingestion. Most debris identified in the MPA was fisheries-related. A total of 11% of benthic macrofauna from Mingulay Reef Area 1 and Banana Reef had ingested microplastics, with no statistically significant effect of feeding guild, station, or reef, on ingestion rates. However, the ingestion rate was highest at a station located in a topographic hollow along a gentle sloping area with strong variable ocean currents where fine-scale interactions between bathymetry and hydrography may have helped trap and focus microparticles. Raman spectroscopy of microparticles revealed several types of polymers being ingested, tentatively identified as polypropylene (PP), polyurethane (PU), polystyrene (PS), and polyethylene terephthalate (PET). Besides establishing a baseline assessment of marine litter and microparticles in a deep-water setting, the approach demonstrates the utility of using historic data and specimens collected for other purposes to expand the geographic and ecosystem coverage for larger more regional-scale and even basin-wide assessments such as those needed to inform Good Environmental Status in European waters, as called for by the Marine Strategy Framework Directive.
Introduction
Marine litter is entering the world's oceans at an unprecedented rate at around 4.8 to 12.7 million metric tons each year (Jambeck et al., 2015). The European Commission Decision (2017/848/EU) defined marine litter as, “…any persistent, manufactured or processed solid material discarded, disposed of or abandoned in the marine and coastal environment” (Galgani et al., 2010). These items include artificial polymer materials such as plastics, rubber, cloths and textiles, paper, processed or worked wood, metal, glass and ceramics. Litter can pose serious threats to human health and safety, impact aesthetics and economies, cause wildlife entanglement, and ingestion, habitat destruction, act as vectors for alien species introduction and bacterial infection, bind chemical pollutants, and can cause vessel damage (Mato et al., 2001; Sheavly and Register, 2007; Rochman et al., 2015). While marine litter has been identified in almost every marine habitat, from coastal areas and continental shelves to the deep sea (Pham et al., 2014), the variability of scale makes management difficult. However, holistic and adaptive management strategies that use robust science to underpin changes in policies and management regimes can be successful at reducing litter input rates (Rochman et al., 2016), e.g., fulmar plastic ingestion has significantly decreased in the North Atlantic gyre over the last 25 years (Van Franeker and Law, 2015).
Today, plastics make up the largest percentage of accumulated marine litter because of the cheap production cost, high robustness, high disposability, low recovery, and persistence in the environment (Rocha-Santos and Duarte, 2015). In the northeast Atlantic alone, plastics make up more than 75% of the reported litter items (Galgani et al., 2013; OSPAR, 2018). Due to the pervasive nature of plastics, the costs of cleanup, and other economic damages, marine litter is now one of the fastest growing threats to world ocean health (European Commission, 2018).
A major concern is the physical mechanical degradation of marine litter, with litter items and plastic polymers being degraded into smaller fragments through exposure to ultraviolet radiation and then biodegrading more when these items become biologically available (Moore, 2008; Cole et al., 2011; Rocha-Santos and Duarte, 2015). As fragment size decreases, litter and plastics become more bioavailable to a wider range of marine organisms (Cózar et al., 2014). At the large end of the degradation size spectrum, macro-debris can comprise still recognizable fishing nets, large pieces of Styrofoam, or items that have been lost or discarded from cargo ships. At the smallest end of the spectrum are nanoparticles, which are either manufactured as such, or represent the end state of microplastic degradation, completely invisible to the naked eye. Microparticles comprise the middle of the size spectrum and include size fractions roughly 2–5 mm in diameter (Galgani et al., 2010; Vince and Stoett, 2018), the most commonly encountered types being microplastics and microfibres.
Primary microplastics are initially < 5 mm diameter, while secondary microplastics are a result of photodegradation of larger items, generally 2–5 mm in diameter (Rochman et al., 2016). Primary microplastics include “nurdles” or pre-production pellets, microbeads from cosmetics and personal care, and fibres from clothes washed in washing machines (Rocha-Santos and Duarte, 2015), as well as styrene-butadiene rubbers from wearing of car tires. Pre-production nurdles are usually introduced into the environment as a result of accidents during ship transport (Ellison, 2007). Point sources for primary microplastics are also relatively easy to identify (Vince and Stoett, 2018) as these derive from personal products washed down shower and sink drains that empty into water courses. Related to these are microfibres, which arrive in the marine environment from sewage wastewater of washing facilities. The origins of secondary microplastics and fibres cannot be as easily deduced, as they are degraded by products of any source microparticles introduced into the oceans (Andrady, 2011), but these usually originate on the sea surface or close to shore where the presence of sunlight readily deteriorates them (Cole et al., 2011).
While the issue of marine litter and plastics has come into public view with greater intensity over the last decade (Jefferson et al., 2014), conclusive evidence as to the extent of the issue is still lacking. For example, until recently, the perception of the deep-sea biome was that it is free from harm (Roberts, 2003; Pahl et al., 2017). But with litter, including plastics, now being reported in some of Earth's most remote and deep ocean environments in the Southern Ocean, Arctic Ocean, the Atlantic, Pacific, and Mediterranean Sea (Van Cauwenberghe et al., 2013; Bergmann et al., 2017; Courtene-Jones et al., 2017a; Jamieson et al., 2017), it is likely that few, if any, deep or open-ocean areas are unimpacted by anthropogenic debris to some extent (Chiba et al., 2018). The topographic and hydrographic complexity of some deep marine ecosystems could act in synergy to essentially funnel and accumulate marine litter and microplastics (Jamieson et al., 2017). Not all litter and plastics remain buoyant in surface waters, with a report of microplastics being four times higher in the deep sea than in overlying waters (Woodall et al., 2014). Degradation, predation, and biofouling can remove these items from the surface and draw them down deeper into the water column and seafloor (Cózar et al., 2014). For example, microplastic abundance in Arctic deep-sea fauna was positively correlated with chlorophyll-a content of surface waters, which suggested particles were vertically transported through incorporation with ice-algal aggregates that caused plastics to sink (Bergmann et al., 2017). The ecological niches of some deep-water fauna, such as sessile invertebrates that filter-feed particles drawn from the water column, may therefore make these taxa inherently even more susceptible to litter and plastic ingestion. However, we lack even baseline assessments from which to gauge the effectiveness of changes in policies or management regimes that aim to reduce litter input rates and minimise risks to deep-water ecosystems and fauna.
Ecological impacts of microparticle ingestion on deep-water fauna have not been studied, but these could pose significant problems to individuals that may then scale up to whole ecosystem effects. Studies from shallow-water ecosystems show that ingestion may block an animal's digestive tract and cause internal injuries; it may result in diminished feeding stimuli, reduced growth rates, and lower rates of development and reproduction (Galgani et al., 2010; Wright et al., 2013). While microparticles specifically have been identified in every trophic level across many ecosystems (Rochman et al., 2015), an organism's feeding strategy can make some taxa more susceptible to ingesting microparticles than others (Cole et al., 2013). For example, microparticle ingestions could be higher in taxa that draw particles non-selectively from the water column before possibly size-selecting and finally assimilating them into their guts. Microparticle ingestion has been shown to vary across deep-sea feeding guilds, and these can vary within species (Taylor et al., 2016; Courtene-Jones et al., 2017a). If particular taxa are disproportionately impacted, microparticle ingestion could stand to significantly alter ecosystem functioning and integrity. Some deep-water ecosystems, such as sponge grounds, seamounts, cold-water coral reefs, and gardens, are biodiversity hotspots of filter and suspension feeders, including the ecosystem engineering sponges and corals themselves (Cathalot et al., 2015). The hydraulic mechanisms by which high quality food is transported to these seafloor ecosystems could also import marine litter (Davies et al., 2009).
Unlike food supply to the deep seafloor, litter enters the marine environment from terrestrial sources and often, inputs by vessels. These reach deep-water masses and the seafloor through ocean currents and offshore winds, but also through biofilm accumulation and biofouling organisms that make litter negatively buoyant, which then sinks to the seafloor (Lobelle and Cunliffe, 2011; Cózar et al., 2014; Chapron et al., 2018). At the seafloor, regional and local bathymetry contribute significantly to the spread and topographic focusing of litter in the deep ocean (Barnes et al., 2009; Tubau et al., 2015; Jamieson et al., 2017), with high accumulation rates occurring in steeply-sided canyons and trenches. Without extreme topography, regional and local hydrodynamics can also affect rates of litter transfer and accumulation, exporting litter from onshore to offshore environments, including the deep sea (Tubau et al., 2015).
Continental shelf zones are often thought to have lower incidences of litter (Galgani et al., 2010; Pham et al., 2014) because most large items originating on the coasts are transferred, assumed to be exported by surface winds and currents to offshore environments beyond the shelf zone (Galgani et al., 2010). However, litter on Earth's continental shelves is still under-reported relative to the coasts (Woodall et al., 2014; Chapron et al., 2018). Furthermore, the density of three-dimensionally complex deep-water ecosystems, such as cold-water coral reefs, are particularly high on continental shelves around the globe (Roberts et al., 2006). These ecosystems help draw surface waters down in phenomena that may be related to local tidal downwelling (Davies et al., 2009), and fine-scale interactions with permeable living objects such as coral heads and mounds baffle sediments and locally reduce current speeds (Huvenne et al., 2009) enough to maximize particle capture rates by the underlying feeding corals and associated fauna (Roberts et al., 2009). Therefore, a straightforward prediction is that cold-water coral reefs on the continental shelf that harbour high diversity and abundances of filter and suspension feeders, and which can topographically focus matter from the surface to the seafloor, are highly likely to have marine litter and show evidence for microparticle ingestion.
The European Union (EU) developed the Marine Strategy Framework Directive (MSFD) in 2008 (Marine Strategy Framework Directive (MSFD), 2008/56/EC) as part of an adaptive management approach to protect and preserve marine resources throughout the EU. The MSFD aims to encourage Member States to ensure and promote Good Environmental Status (GES) of the marine environment by 2020. Under Article 3 of the MSFD, GES is “the environmental status of marine waters where these provide ecologically diverse and dynamic oceans and seas which are clean, healthy and productive”. Under the MSFD, Member States are to apply criteria and indicators to assess GES across 11 Descriptors, including Descriptor 10: Marine Litter, ensuring an interdisciplinary approach to conserving and protecting the marine realm (Borja et al., 2010). The MSFD also calls for consistent and standardised monitoring methods and assessment of European seas, which may be scaled down to regional seas and sub-regional seas, i.e., the Baltic Sea, North East Atlantic Ocean, Mediterranean Sea, and Black Sea (Commission Decision, 2017/848/EU).
While the Mediterranean Sea and the North East Atlantic GES assessment regions support many cold-water coral reefs and gardens on their continental shelves, no deep-water indicators have been established for Descriptor 10. Thus, baseline assessments for these ecosystems, which cover a large number of the assessment areas and a disproportionate amount of deep-sea biodiversity (up to three times higher than non-coral areas, sensu Henry and Roberts, 2007), are missing from regional GES assessments. Therefore, GES assessments risk over-extrapolating the scale of monitoring to the assessment objective: Descriptor 10 (as with all other Descriptors) are only measured in certain spatial assessment units, primarily shallow water areas. These assessments are then used to allude to the status of the entire water body, including deep-water areas, and thus, the measurement truly only reflected that particular site, ecosystem, or region (Borja et al., 2010).
In the United Kingdom, the definition of GES was developed in consultation with UK government and advisors, including the Centre for Environment, Fisheries and Aquaculture Science and the Joint Nature Conservation Committee (HM Government, 2012); participation in Scotland was driven by government and non-governmental organizations, including Marine Scotland, the Scottish Coastal Forum, Crown Estate Scotland, the Marine Conservation Society, Fidra, Zero Waste Scotland, and others (Scottish Government, 2018). Currently, the only Descriptor 10 assessment for the UK is comprised of systematic beach surveys conducted by Marine Conservation Society (HM Government, 2012). Surveillance and monitoring targets of litter on the seafloor and in the water column are not yet established because baseline levels cannot be deduced (HM Government, 2015).
The purpose of the present study was to establish baseline levels of marine litter and microparticles for a deep-water setting on an area of Scotland's continental shelf that is characterised by topographical and hydrographic complexity and cold-water coral reef ecosystems densely populated by diverse feeding guilds. The study hypothesised that suspension feeders have a higher number of ingested microparticles than other feeding guilds and that specimens from hollow topographic areas have a higher number of particles compared to elevated areas. The study also provided the opportunity to demonstrate the value of using historic data and samples collected for other purposes (the “collect once, use many times principle”), from which one can obtain baseline values for marine litter and D10 in areas traditionally under-represented in GES assessments, such as deep-sea habitats (Miyake et al., 2011; Pham et al., 2014).
Methods
Study Area: the East Mingulay Marine Protected Area
The East Mingulay Special Area of Conservation is a Marine Protected Area (MPA) located 13 km to the east of the now uninhabited island of Mingulay in the Sea of Hebrides, off western Scotland. This MPA represents the only known inshore occurrence of reefs formed by the protected species of scleractinian cold-water coral, Lophelia pertusa (Roberts et al., 2005). The Mingulay Reef Complex (MRC) was first mapped in 2003 (Roberts et al., 2005), and is made up of five bedrock ridges consisting of extensive Lophelia coverage forming reef mounds up to 5 m high, surrounded by aprons of coral rubble and shelly hash (Roberts et al., 2009). Reefs support a high biodiversity of fauna, including a biologically rich filter- and suspension feeding communities (Henry et al., 2010, 2013; Kazanidis and Witte, 2016; Kazanidis et al., 2016). Geochronology of vibrocore samples across some of these mounds demonstrate a Holocene coral occurrence, with Lophelia occurring periodically since at least 7,000 years ago (Douarin et al., 2013, 2014).
Potential sources of marine litter for the reef complex include litter introduced by fisheries and items originating from coastal communities that are transferred offshore. A zoned fisheries management regime is in place to protect the reefs from anthropogenic damage (Scottish Government, 2014), with all types of mobile fishing gear and some types of static gear prohibited year-round. Yet the footprint of historic fisheries operations could still be potential sources of marine litter, as would be today's Nephrops fisheries that continue to operate in adjacent areas. Although nearest to the uninhabited island of Mingulay, marine litter disposal from nearby inhabited islands of the Inner and Outer Hebrides could be exported from the coast to the offshore environment. Besides local fisheries and coastal communities, a third possible route for litter in the Sea of the Hebrides would originate in the open Atlantic (Lusher et al., 2014). The reef complex is supplied by ocean currents that develop as a result of the subpolar and subtropical North Atlantic gyres (Davies et al., 2009), which can collect marine litter and distribute these items globally (Law et al., 2010; Reisser et al., 2015).
Because much of the coral ecosystem's food supply is derived from diurnal tidal exchanges from the surface to the seafloor, (Davies et al., 2009), litter and microplastics from local fisheries, coastal communities, and the open Atlantic could, in theory, be rapidly downwelled from the sea surface to the reefs in under 20 min. The fine-scale and permeable morphology of the coral heads themselves can act to slow down and trap particles in the water column through sediment baffling (Huvenne et al., 2009). This mechanism not only adapts the corals to their ecosystems but could also increase ingestion of microplastics by cold-water coral reef organisms. A broad spectrum of feeding guilds is represented at the MRC; suspension feeders, filter feeders, deposit feeders, grazers, omnivores, and predators (Henry et al., 2013). The shallower, westernmost areas are located closer to the inhabited islands of Barra and Benbecula, and experience, on average, stronger currents than those in the east (Navas et al., 2014). Western sites are also characterized by higher numbers of species inhabiting the reefs (Henry et al., 2010), with higher proportions of filter and suspension feeders than in the deeper more remote eastern sites (Henry et al., 2013). Thus, not only was it expected that litter and microplastics would be found in the East Mingulay MPA, but that the frequency of occurrence would vary across the reef complex and between feeding guilds.
Use of Historic Surveys to Assess Marine Litter
Past underwater camera and remotely operated vehicle (ROV) surveys of the MRC were used to overcome the absence of marine litter monitoring deep on Scotland's continental shelf (the exception being offshore private sector data from post-debris field surveys). Cruise reports from several research surveys conducted between 2003 and 2012 were systematically reviewed to identify, categorize, and map marine litter occurrences (Table 1).
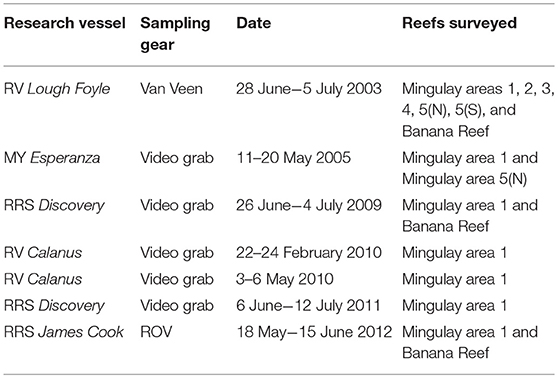
Table 1. List of historic research cruises for which the systematic review of marine litter was conducted for the East Mingulay MPA.
Two of these past research cruises also collected video, which was also systematically reviewed in case litter items were missed in the original cruise reports. In 2003, footage collected by a Van Veen grab equipped with video were obtained to assess biotope types (Roberts et al., 2005). For the present study, approximately 60 min of video from 56 stations were reviewed on Windows Media Player for evidence of litter (Table 2). In 2012, video footage collected by the Irish Marine Institute's Holland-I ROV was originally collected to assess fish communities on the MRC (Milligan et al., 2016) and to locate deep-sea shark nursery grounds (Henry et al., 2013). For the present study, 34 h of Holland-I ROV footage from 10 dive transects from the Mingulay 1 reef were reviewed in Windows Media Player to assess marine litter (see Supplementary Material S1).
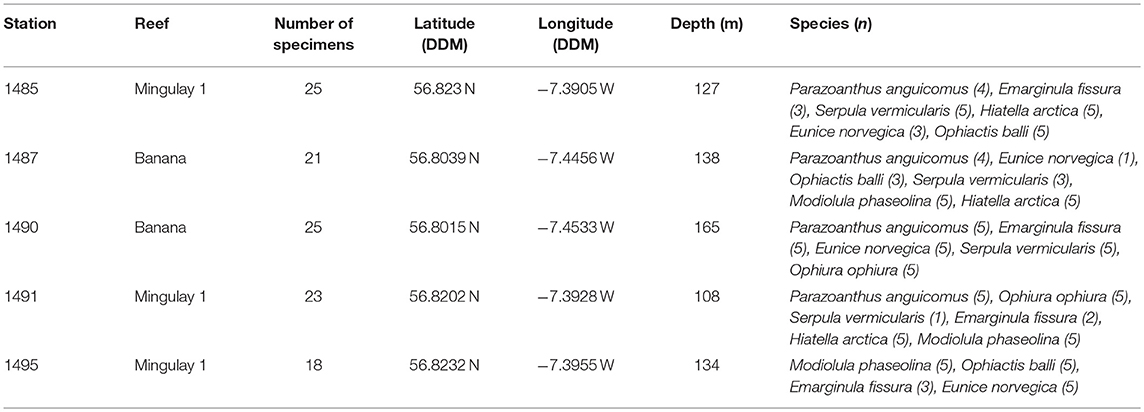
Table 2. Video grab stations and selected species for the assessment of microparticles at the East Mingulay MPA where n equals the amounts of specimens dissected.
In an effort to standardise the reporting of litter, the United Nations Environment Program/Intergovernmental Oceanographic Commission (UNEP-IOC) Guidelines on Survey and Monitoring of Marine Litter were adopted (see Supplementary Material S2), in which items within each litter category were counted (Cheshire et al., 2009).
Use of Historic Samples for Microparticle Assessments
Harmonised protocols for microparticle assessments in benthos are needed to facilitate comparison across ecosystems (Miller et al., 2017). Therefore, the present study adapted methods developed by Courtene-Jones et al. (2017a) in the adjacent deep-sea Rockall Trough to assess microparticles in historic benthic samples collected from cold-water coral ecosystems at the MRC.
Benthic faunal samples were originally collected by video-assisted benthic grabs in 2009 during the RRS Discovery cruise 340b in June and July (Inall, 2009). Samples were sieved on board over a 1 mm mesh and washed with seawater, preserved in buffered 4% formalin, and then transferred to a 70% industrial methylated solution (IMS) for long-term storage. For the present study, 112 specimens already sorted and identified to species level were selected from across five stations on the MRC (Table 2). Station selection ensured a spatial gradient in environmental settings between Banana Reef (located closer to the nearest island) and Mingulay Reef 1 (further from the inhabited islands). All selected taxa were known to be abundant at the MRC (Henry et al., 2010, 2013; Kazanidis and Witte, 2016; Kazanidis et al., 2016), making them useful indicator taxa for the future assessment of microparticles. Taxa included suspension feeders: the zoanthid Parazoanthus anguicomus Norman, 1869 and the tubiculous polychaete Serpula vermicularis Linnaeus, 1767, filter feeders: the bivalves Hiatella arctica Linnaeus, 1767 and Modiolula phaseolina Philippi, 1844, a deposit feeder: the limpet Emarginula fissura Linnaeus, 1758, omnivores: the ophiuroids Ophiactis balli Thompson, 1840 and Ophiura ophiura Linnaeus, 1758, and a predator and important symbiont with Lophelia pertusa: the carnivorous polychaete Eunice norvegica Linnaeus, 1767 (Figure 1).
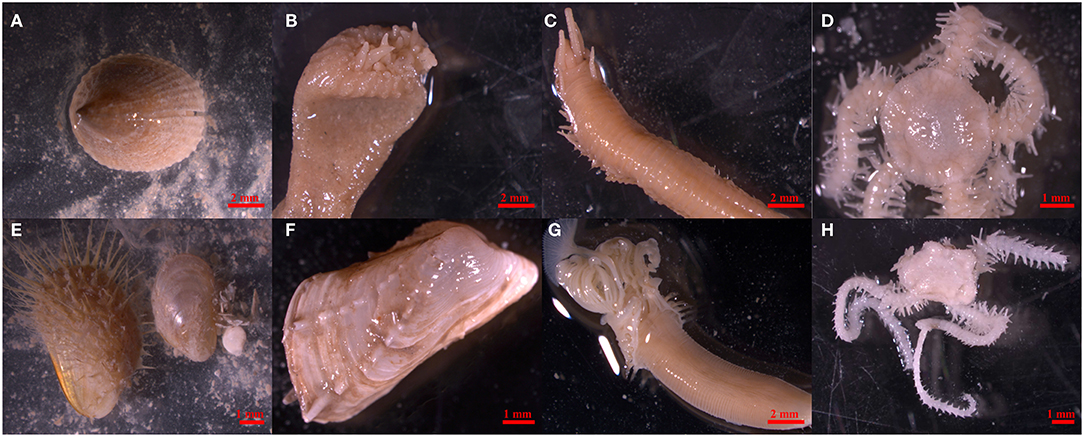
Figure 1. Study organisms Emarginula fissure (A), Parazoanthus anguicomus (B), Eunice norvegica (C), Ophiactis balli (D), Modiolula phaseolina (E), Hiatella arctica (F), Serpula vermicularis (G), and Ophiura ophiura (H) (From left to right, top to bottom).
Following a protocol developed by Courtene-Jones et al. (2017b), an enzymatic 30% StableCell™ Trypsin and deionized water solution was chosen to dissociate organic material from the benthic specimens from any synthetic microparticle polymers. It is unlikely that trypsin would enzymatically degrade the robust synthetic polymers and rubbers to a significant extent under these conditions. However, due to the specimens being fixed in formalin and preserved in IMS for nine years, initial trials showed incomplete digestion even after 24 h. To aid digestion and to ensure any microparticles had been ingested and not artificially introduced during reef sampling and processing, the gut cavity of each specimen was dissected and digested instead. Guts were placed in the trypsin/deionized solution in a 50 mL covered glass jar and placed in a water bath of 38–42°C to digest for 25 min (Courtene-Jones et al., 2017a). All contents were then strained through a 0.075 mm metal gauze to separate biological material from potential non-biological materials (microparticles). The gauze was rinsed with deionised water into a glass petri dish and the contents were analysed and photographed under a Zeiss SteREO Discovery V20 stereomicroscope. Microparticles were stored in 20 ml glass vials for Raman spectroscopy analyses to identify potential microplastic candidates.
Microparticle Laboratory Quality Assurance and Quality Control (QA/QC)
Further to digesting only the animal's digestive system, additional precautionary measures were taken to ensure processing in the laboratory did not artificially introduce microparticles into the samples (Woodall et al., 2015). Clothing and laboratory coats were 100% cotton for the duration of the study. The microscope cover was used whenever the microscope was not in use, and gloves were always worn (Torre et al., 2016). The lab area was cleaned with an IMS solution three times prior to beginning any analysis. All glassware and tools were triple rinsed with deionised water (Courtene-Jones et al., 2017a). A control petri dish with damp filter paper was placed on the lab bench and analysed at the end of the study to identify any potential air contamination (Courtene-Jones et al., 2017a). Protocols to ensure a clean laboratory environment are more effective than excluding certain microparticles in analysis (Torre et al., 2016). Therefore, no particles were excluded in the analysis. Additionally, specimen vials were pre-screened prior to digestion to identify microparticles also stored in the IMS but which had not been ingested by the reef animals.
Statistical Analyses
Chi-squared analyses were used to test the null hypotheses that microparticle ingestion was equally likely across feeding guilds, reef stations, and the reefs themselves. Statistics were performed using IBM SPSS® statistics software version 24.
Raman Spectroscopy
Raman spectroscopy was used in an attempt to identify microplastics found during this study. Raman spectroscopy uses an inelastic scattering of light that vibrates off the molecular structure of an object (Araujo et al., 2018). The vibrational signatures can then be matched to known chemical structures. Raman spectroscopy was used to tentatively identify samples, referenced against existing literature and libraries. Raman data was collected on a Renishaw inVia Raman Spectrometer equipped with a Leica DM 2,500 M microscope and 785 nm diode laser (Toptica Photonics, Xtra I). The excitation beam was focussed on to the sample using a 20x/0.4 N.A objective lens (Leica, NPLAN) providing an excitation spot of a 2.5 μm diameter on the sample. Spectra were acquired between 100 and 3400 cm−1 in extended scan mode using a 600 lines/mm grating and collected with a cooled CCD. Due to high background fluorescence from the samples, the acquisition parameters were optimised per sample using excitation powers between 0.75 and 37.25mW and exposure times between 10 and 30s. The exposure parameters were limited by detector saturation due to the fluorescence background. Spectra were then processed using a modified multi-polynomial fitting to remove the fluorescence background and were then smoothed using a Savitzky-Golay filter (Ghosal et al., 2018).
Results
Historic Litter Assessment
The systematic review of cruise reports recorded six instances of litter out of 217 benthic stations sampled by different gear (ROV dive transects and video-assisted grabbing), one of which was outside the MPA boundary but the other five were recorded from Mingulay Reef 1 and Mingulay Reef 5 North. An analysis of historic video from the 2003 survey for the present study recorded an instance of litter that had not been logged either in the main body of the cruise report or the accompanying video catalogue, but which had been reported in the cruise report's appendix. This reflects the critical need for a more rigorous and systematic approach to reviewing historic documents. It was also notable that cruise reports from surveys conducted in 2009 and 2011 recalled that lost fishing gear had directly impeded the deployment of equipment, and in 2011, caused significant damage to sampling gear.
Marine litter observed during the present study was reported in accordance with the Guidelines on Survey and Monitoring of Marine Litter (Cheshire et al., 2009), as standardised reporting is critical to promoting knowledge of litter pertaining to GES and the MSFD. The three observed litter items reported during the 2003 survey and identified during accompanying video footage analysis were best designated as “Plastic Sheet or Tarpaulin” (litter code RL16), but whether these were fisheries-related or, e.g., boating, is unclear. In the 2005 and 2009 cruise reports, observed litter was classified under UNEP-IOC's General Fishing and Boating category, which includes buoys, nets, monofilament line, and rope (litter codes RL05 and RL08 for 2005 and 2009, respectively). The 2011 survey reported litter that was best categorised as fishing-related litter, which includes sinkers, lures, hooks, traps, pots, and baskets or trays (litter code RL08). No litter was observed from ROV footage collected during the 2012 survey (Table 3).
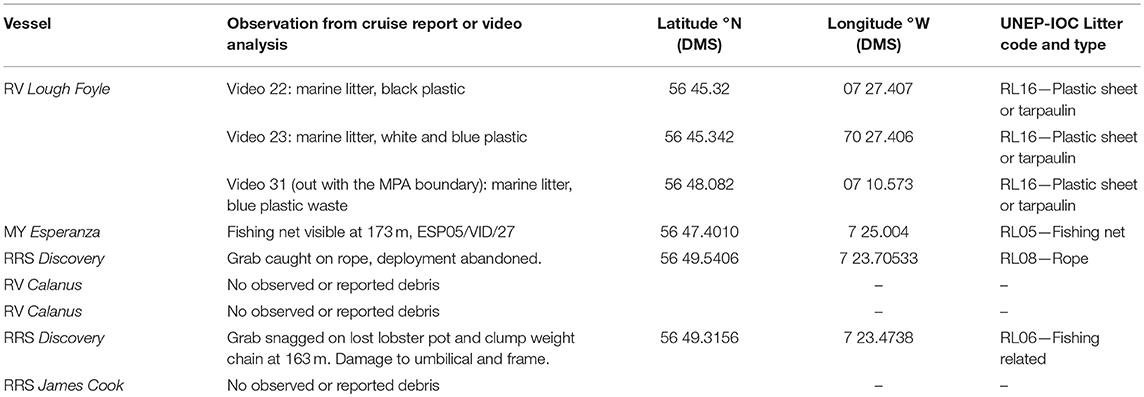
Table 3. Categories of marine litter observed from video footage or reported in cruise reports from the East Mingulay MPA and an adjacent area.
Microplastic Analysis
Out of the total 112 organisms sampled (Figure 2), 10 specimens had ingested 1 microparticle, all of which were classified as microfibres (images of all microfibres are provided in Supplementary Material S3) and in one case, the omnivorous ophiuroid Ophiactis balli had ingested both a microfibre and a microplastic particle (La Beur et al., 2019). Frequency of ingestion varied disproportionately across feeding guilds: of the 10 specimens that had ingested microparticles, five were suspension feeders, while only two filter feeders, two omnivores, and one deposit feeder had ingested microparticles. Suspension-feeding taxa (the zoanthid Parazoanthus anguicomus and the polychaete Serpula vermicularis) ingested approximately the same number of microfibres as the deposit-feeding limpet Emarginula fissura), with 16% and 15% of specimens having ingested microparticles, respectively. No microparticles were retrieved from the guts of the predatory worm Eunice norvegica.
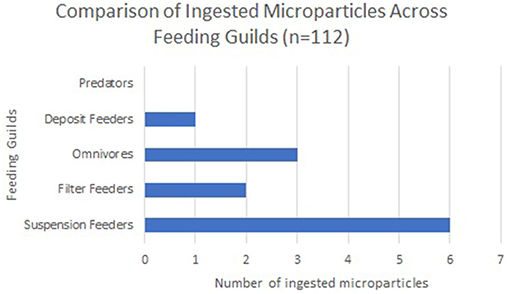
Figure 2. Number of ingested microparticles across feeding guilds shows that suspension feeders most commonly ingested microparticles, followed by omnivores, and then filter feeders.
Microparticle ingestion varied across the MPA, but all five stations showed evidence of specimens ingesting microparticles. Frequency of ingestion was highest at Station 1,485 on Mingulay Reef 1, with microfibres found in 20% of the specimens sampled here, and lowest at Station 1,490 on Banana Reef (Figure 3). Notably, Station 1,485 was located in a topographic seafloor depression in a gently sloping area with strong, but variable, ocean currents. In general, frequency of ingestion was three times higher on Mingulay Reef 1 (12%) than on Banana Reef (4%). Although the number of ingested microparticles clearly differed between feeding guilds, stations, and between the two reefs (Figure 4), none of these differences were statistically significant at α = 0.05 (χ2 = 3.85, df = 4, critical value = 9.49 across feeding guilds; χ2 = 4.63, df = 4, critical value = 9.49 across stations; χ2 = 1.98, df = 1, critical value = 3.84 between reefs). These results are not statistically significant but may be biologically significant. More research in this area is needed.
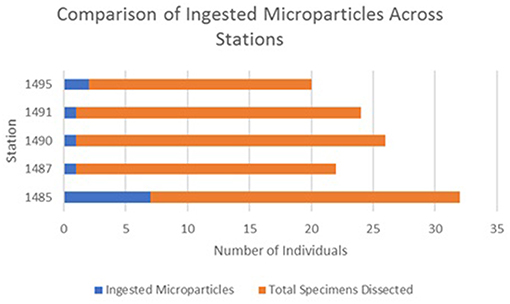
Figure 3. Number of ingested microparticles across stations shows how many individual specimens were found to have ingested microparticles out of all the specimens dissected.
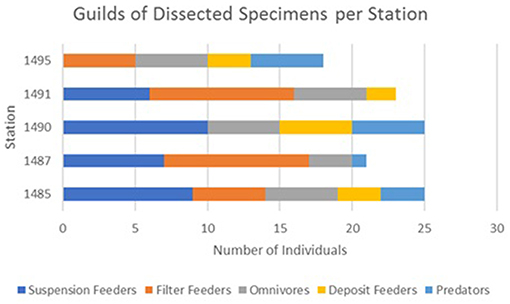
Figure 4. A comparison of the individual specimen feeding guilds dissected for this experiment across reef stations.
The present study documented marine litter, including microplastics, at three of the reefs in the East Mingulay MPA, including Mingulay Reef 1, Mingulay Reef 5 North, and Banana Reef. Most records fell within the zoned managed area that now prohibits most forms of fishing, with a majority of litter (categorised mostly as associated with fisheries activity) being found at Mingulay Reef 1 (Figure 5).
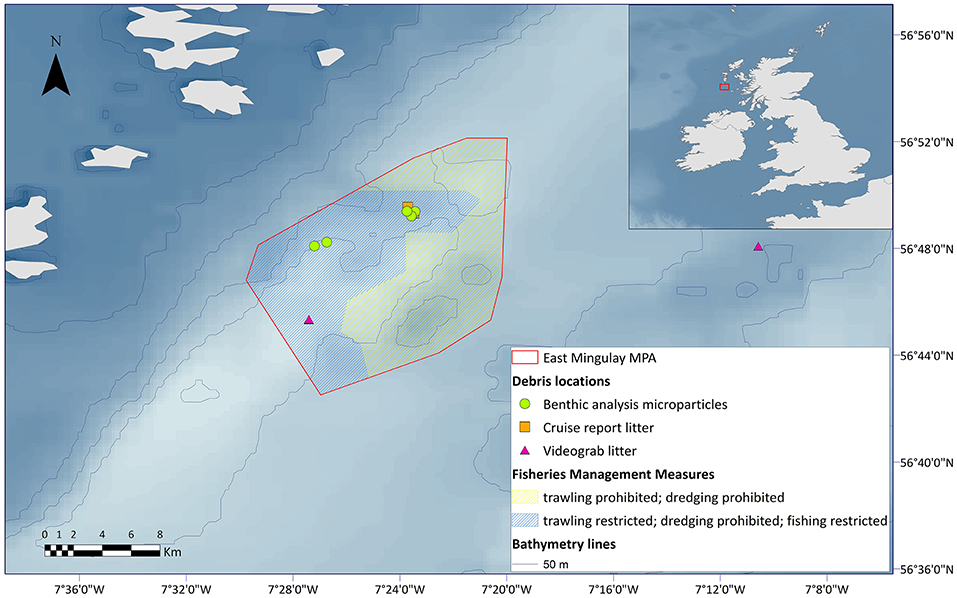
Figure 5. Locations of marine litter and microparticles identified from historic cruise reports and benthic samples from the East Mingulay MPA.
Raman Spectroscopy Results
Raman spectroscopy yielded a unique spectrum for all samples found (Supplementary Material S4). Samples were baseline corrected (Supplementary Material S5); however, spectra could not be identified definitively, as many had significant noise, or appeared to have additional peaks to known reference samples, potentially indicative of sample degradation or biofouling. Physical degradation, biofilm, and photodegradation can all contribute to the mis-identification of polymer structures. Tentative sample identification was only possible for a few samples, and included variations of potential polystyrene, polyurethane, and poly (ethylene terephthalate).
QA/QC Laboratory Contamination Assessment
The damp Whatman filter paper was evaluated after 26 days of air contamination of the lab station. There were 37 fibres on the filter paper from the whole of the study. It is worth noting that the fibres observed on the filter were of a different size, colour, and shape than the fibres found in the specimens of the benthic analysis. When precautionary measures are taken, background contamination can be negligible (Torre et al., 2016). Therefore, it was concluded that there was no air contamination of the dissected specimens, and thus, this study did not exclude any microfibres in quantification or interpretation (Taylor et al., 2016). Additionally, in studies of marine microfibres, contamination of microscope covers has been found to be statistically insignificant, and in fact, using microscope covers drastically reduces contamination of microfibres being identified (Torre et al., 2016).
Discussion
The present study on marine litter and microparticle ingestion demonstrated that archived data can be reviewed and used in new ways to obtain baseline levels of anthropogenic debris and microparticle ingestion. This “collect once, use many times” approach can help inform marine policy including regional and sub-regional GES assessments for the MSFD, without the need for costly investment in new surveys to obtain environmental baselines. This first assessment of marine litter and microparticles on the deep continental shelf to the west of Scotland showed that most debris was lost fishing gear. The benthic fauna inhabiting the cold-water coral reef ecosystems of this area recorded the legacy of marine litter, with 11% of organisms across a range of feeding strategies having ingested microparticles of degraded anthropogenic debris. Fine-scale interactions between bathymetry and hydrography may have played a role in trapping and focusing these microparticles, with the highest ingestion rates occurring in a topographic hollow along a gentle slope in an area with strong variable ocean currents. Ingested particles were almost entirely microplastic fibres 2 to 5 mm in length, with several types of polymers tentatively identified by Raman spectroscopy, including polypropylene (PP), polyurethane (PU), polystyrene (PS), and polyethylene terephthalate (PET). Along with other related studies on marine litter and microplastics in deep Scottish waters, including the Rockall Trough (Courtene-Jones et al., 2018), the present study on a continental shelf setting provides a first baseline from which progress towards achieving GES beyond 2020 can be measured. A series of methodological recommendations are also provided to aid future studies.
Marine Debris
Trends of macrolitter across the MRC were fishing-related litter in the form of plastic sheets or tarpaulin and discarded fishing gear, commonly referred to as “ghost fishing” due to the high possibility of organisms becoming entangled or ensnared. The MRC was designated an MPA in 2010, and since that time, fishing restrictions have been in place (Scottish Government, 2014). Therefore, any fishing-related litter identified should be from before 2010 or may have been transferred in by local currents. The ROV was equipped with an Insite Mini Zeus camera with direct HDSDI fibre output, as well as two Watt Deep-sea Power & Light SeaArc2 HMI lights and two 25,000 lument Cathx Ocean APHOS LED lights; these systems may influence the amount and type of litter observed in this study. Therefore, the marine litter reported in this study may be a conservative estimate of the amount of litter at Mingulay. Without collecting the litter items, it is difficult to source its origin. Microlitter identified at the MRC was primarily comprised of fibres. This corresponds with other studies' findings, as fibres are increasingly being reported as being ingested by deep-water organisms (Courtene-Jones et al., 2018).
Ecosystem Effects on Microparticle Ingestion
The present study supports other studies globally that microfibres are frequently found in the internal cavities of deep-water and deep-sea benthic fauna beyond the coasts (Woodall et al., 2014; Taylor et al., 2016; Courtene-Jones et al., 2017a). However, in contrast to Taylor et al.'s study (2016) that found that deposit feeders were more likely to ingest microfibres than suspension feeders, ingestion rates were as frequent for suspension feeders at the MRC as they were for the deposit feeder (16% vs. 15% ingestion rates, respectively). Furthermore, none of the predatory worms (Eunice norvegica) at the MRC had ingested microparticles, in contrast to consumption by predatory squat lobsters reported by Taylor et al. (2016), or by the predatory sea star Hymenaster pellucidus and gastropod Colus jeffreysianis (Courtene-Jones et al., 2017a). To summarise, the present study demonstrated no statistically significant effect of feeding type on frequency of particle ingestion (p > 0.05). This may be due to the rapid tidal cycling currents at the MRC (Davies et al., 2009); species at Mingulay may not have a chance to ingest microparticles as frequently as species at other sites with different hydrographical conditions.
These opposing findings could point to differences between focal ecosystems, the present study being located on a deep but inshore continental shelf setting, in contrast to bathyal open-ocean seamounts (Taylor et al., 2016) or the open-ocean bathyal plains (Courtene-Jones et al., 2017a). In addition, one of the reasons that Taylor et al. found more microplastics in deposit feeders than suspension feeders may relate to the fact that hydrographic conditions in their study regions may promote the settlement of litter on the seafloor, and thus, deposit feeders may be exposed to more particles than suspension feeders. Mingulay is a very hydrographically dynamic environment in which the settlement rates of particles are likely to be lower than those in (Taylor et al., 2016), and thus, the deposit feeders in Mingulay have smaller chances to capture these items.
Inter-related to contrasting ecosystem settings could be interspecific differences in life histories and biology, wherein the ingestion rate varies more strongly between species than they do between feeding guilds (Courtene-Jones et al., 2017a). Unlike bathyal seamounts and plains, the benthic macrofaunal community at the MRC comprises mixed assemblages of both coastal and deep-sea taxa. None of the species selected for the present study are exclusive to the deep-sea, and eurybathic species such as these may have wider environmental tolerances that adapt them to dynamic coastal zones. For example, these species may be faster-growing and feed more frequently than their stenobathic deep-sea counterparts if food supply is not limited or more continuous than the often-pulsed nature of food supply in the deep sea, factors that would all essentially equalise the opportunity to ingest food across feeding guilds. Courtene-Jones et al.'s (2017a) study from the abyssal ecosystem of the Rockall Trough also recorded ingestion rates that were four times higher than those found in the present study on the continental shelf and could also implicate ecosystem effects. For example, all three species examined from the bathyal zone were mobile taxa, in contrast to only three of eight species considered in the present study, the rest being truly sessile attached to substrata. Limited mobility may therefore reduce particle encounter rate if an organism cannot move to areas when food becomes more abundant. The handful of studies that currently exist on microparticle ingestion in deep-water marine ecosystems limits more firm conclusions, except to say that interspecific differences (and not feeding guilds) may hold the key to improving the understanding of ecosystem susceptibility to plastic consumption.
Possible Transport Routes for Marine Litter in the East Mingulay MPA
Our results provide complementary data for the deep seafloor to accompany Lusher et al.'s (2014) measurement of sea surface microplastics in the Sea of the Hebrides, which were recorded to be between one to five microplastic items per cubic meter water (relatively lower than the offshore regions, with 10 to 25 items per cubic meter). Without further analysis of surface microplastics over the MRC, it is not known whether microparticles ingested by reef organisms in the East Mingulay MPA derived entirely from downwelled surface waters, or from bottom currents. Previous studies by Duineveld et al. (2012), Kazanidis and Witte (2016), and Kazanidis et al. (2018) have shown that several species in Mingulay (including Parazoanthus anguicomus) depend more on surface fresh food particles, rather than on degraded bottom particles. This could be an indication about the role of downwelling of surface material vs. material transported from bottom currents.
The Raman spectroscopy analyses showed a complex mixture of anthropogenic compounds reaching the seafloor that seems to contrast with those found in the bathyal Rockall Trough further to the west of the MPA. Courtene-Jones et al. (2017a) reported that polyester dominated the water column fraction of microplastics in the deep Rockall Trough adjacent to the west of Scotland, but acrylic dominating the benthic fraction. Although definitive Raman spectroscopy results for ingested microparticles at the MRC were inconclusive, these first analyses point to a different type of microparticle assemblage being ingested by benthic fauna, including possibly polypropylene (PP), polyurethane (PU), polystyrene (PS), and polyethylene terephthalate (PET). Notably, instead of acrylics that could come from clothing fibres and washing/wastewater facilities, tentative sources of microparticles in the East Mingulay MPA could therefore derive from, e.g., marine ropes (PP), surfboards, boat hulls and decks (PU), protective packaging (PS), and food and beverage packaging (PET).
Original sources of plastics could begin to be inferred if stations closest to inhabited Outer Hebrides islands showed higher ingestion rates, but the present study demonstrated no statistically significant effect of either station, or reef. Identifying point or non-point sources of litter is important for management strategies, including source-reduction, retrieval, and mitigation (Rochman et al., 2016), and with regard to GES and the MSFD, this information would help inform management measures. In the meantime, while the present study could not identify a point source, the Raman spectroscopy results suggest that island management along the inner and outer Hebridean archipelago could take a more precautionary approach. Island communities could strive to minimise inputs from marine boating, fishing, and coastal development, e.g., by preventing the disposal of marine ropes and protective packaging, inspecting loose or chipped decks, and hulls, and offering recycling services for food packaging. Much of this litter input can be prevented by conducting regular beach clean-ups and increasing public awareness, particularly in the tourist season to prevent items from being carried offshore.
Bioindicator Candidates to Inform GES
A precautionary approach to management does not preclude the need to understand to what extent marine litter and microplastics harm deep-sea benthic organisms. Harm to these organisms, or ecosystem-scale impacts, cannot be deduced from this or other studies until long-term experiments are performed. Microparticles were most frequently observed in the zoanthid Parazoanthus anguicomus, which occurs in high current speed settings at the MRC (Roberts et al., 2009; Henry et al., 2010) and which may therefore be pre-adapted to processing coarse sediments and microparticles. However, microplastic ingestion could, for example, be accompanied by toxins that disrupt reproductive processes (Ryland, 2000; Cole et al., 2011).
Existing collections of MRC fauna and P. anguicomus in particular, could also be re-used to investigate such issues to compare zoanthid biomass, size, and reproductive traits between groups that ingested microparticles and those that did not. Parazoanthus anguicomus may also qualify as a good indicator species to assess abundance of microparticles for GES because the present study demonstrates it ingests microfibres and is very common across the MRC in high abundances (Henry et al., 2010).
Under the OSPAR Convention, the fulmar seabird was chosen as a sea surface interchange litter-monitoring species (OSPAR, 2018) as there are previous historical data that can be referenced as a baseline (Van Franeker and Law, 2015). For benthic fauna, no species has yet been identified as being a good indicator. Additionally, not all benthic species would make good indicators for investigating sea floor litter and it is important to understand what species are ingesting microparticles. This study demonstrates that suspension feeders and the readily abundant zoanthid Parazoanthus anguicomus in particular would make good sentinel species for GES assessments of D10.
Methodological Recommendations
Standardised approaches to identify and report litter during at-sea research and monitoring activities should be promoted. Standardisation will help integrate these data more easily into regional frameworks and to better inform policy, especially with regard to GES. The UNEP-IOC Guidelines on Survey and Monitoring of Marine Litter (Cheshire et al., 2009) adopted by the present study provided a simple framework by which to define litter and microparticles, for example. The UNEP does not require litter densities to be calculated, but this information, where possible, may prove valuable for assessing marine litter across larger scales.
In strong support of GES, it is recommended that, where possible, litter from the surface, water column, and seafloor be brought on deck and measured using criteria set forth by the EU Commission Decision 2010/477/EU, including: source and pathway of litter (if known); amount of litter per category in number of items (D10C1); amount of micro-litter per category in number of items and weight in grams (D10C2); amount of litter in grams and number of items per individual for each species in relation to size (as appropriate) (D10C3); and number of individuals affected per species (D10C4).
The present study demonstrates how the challenge of assessing GES in vulnerable marine ecosystems can be overcome by re-using data to obtain a baseline for GES, which then set the benchmark from which progress towards achieving GES can be established in a manner that is inclusive of such ecosystems. The re-use of historical surveys and samples in the present study demonstrated the utility to establish baseline levels for assessments and to further understand the extent of marine litter and microparticle pollution issues. However, limitations of using data collected for other purposes must be acknowledged: future shipboard collection and preservation of specimens should include best practice for avoiding contamination of litter and microplastics, for example. Some specimens had accumulated considerable biofilms on their surfaces, which may have contributed to the indeterminate signatures overserved through Raman spectroscopy. Polyamide and polyester were found in the highest abundances over time in the Courtene-Jones et al. (2018) study of a proximate area in the North East Atlantic.
Socioeconomic growth in key industry sectors (“blue growth”) including maritime and coastal tourism present challenges to achieving GES. Globally, blue growth may prioritise economic potential over environmental protection (Elliott et al., 2018). Publicly accessible media productions, such as the BBC's Blue Planet II, help to bridge the scientific community to wider society to coalesce abstract problems and specific solutions (Pahl et al., 2017). There was a strong audience demand for knowledge and action, to which the government responded to with the enactment of bans on single-use plastic items (Xanthos and Walker, 2017). In January 2018, the European Commission also announced a European-wide strategy to reduce plastics in the marine environment (European Commission, 2018). This plan includes strategies to restrict the unnecessary addition of microplastics in products such as personal care and hygiene, as well as to curb major sources of pollution such as vehicle tyres, textiles, and industrial plastic pellets (European Commission, 2018). These changes in social behaviours have minor significance in the global input of plastics, but these changes support a bottom-up approach to influencing the policies implemented by international frameworks, like the MSFD and Water Framework Directive (Galgani et al., 2013). In turn, this can generate momentum for large scale backs in industrial plastic consumption. Ultimately, these upper echelon policy changes have a lasting impact on the litter entering the world's oceans (Van Franeker et al., 2011).
Conclusion
The understanding of the extent of marine litter and the harm it causes continues to gain momentum. In Europe, Member States are obliged by the MSFD to work collectively to establish targets, criteria and indicators of the most poorly understood Descriptor, D10—Marine litter (Galgani et al., 2013). However, there are currently no coordinated national or regional monitoring programmes for marine litter in surface waters, the water column, or at the seabed within Europe, nor are there monitoring programs for microparticles (Galgani et al., 2010). The UK's position on GES and its adoption of the MSFD are unknown at the time of writing due to the “Brexit” implementation by March 2019. Yet, the present study demonstrated that the extent of marine litter and microplastics runs throughout a deep remote area on the UK continental shelf, characterised by vulnerable cold-water coral reef ecosystems, with many functional groups ingesting anthropogenic waste. With the socioeconomic push for blue growth, recommendations include adopting baseline assessments to reduce the frequency of microparticle ingestion from 11% and implementing standardized protocols for recording and analysing litter. Such recommendations can likewise be implemented with relatively little additional cost by re-using data collected for other purposes and harmonising new data collection practices across larger areas across all of the UK's different marine ecosystems.
Data Availability
All datasets generated for this study are included in the manuscript and/or the supplementary files.
Author Contributions
LL and L-AH designed the study. JMR provided historic video and benthic samples that LL reviewed. LL and GK analysed macrobenthos for microplastics. GK provided images of the macrofauna, SH, MS, and AM ran and helped interpret the Raman spectra. All authors contributed to writing the manuscript.
Funding
The analysis described in this paper received funding from the European Union's Horizon 2020 research and innovation program under grant agreement No. 678760 (ATLAS). This output reflects only the authors' views and the European Union cannot be held responsible for any use that may be made of the information contained therein. SH received funding from NERC through and IRF (NE/K009028/1 and NE/K009028/2).
Conflict of Interest Statement
The authors declare that the research was conducted in the absence of any commercial or financial relationships that could be construed as a potential conflict of interest.
Acknowledgments
The historic samples and offshore videos used in this study were collected during multiple projects with support from the MINCH project partnership (RV Lough Foyle cruise 2003), Greenpeace (MY Esperanza cruise 2005), the Oceans2025 programme (RRS Discovery 2009), The European Project on Ocean Acidification (RV Calanus 2010, grant agreement no. 211384), and the UK Ocean Acidification Programme (RRS Discovery 2011 & RRS James Cook cruises, NERC grant NE/H017305/1). We gratefully acknowledge all involved in supporting these expeditions over the last 15 years. We would also like to thank Freija Mendrik and Fiona Murray for assistance in the laboratory and Berta Ramiro Sanchez for assistance in GIS, James Cook cruises, NERC grant NE/H017305/1, with additional support by NE/K009028/2 to SJH.
Supplementary Material
The Supplementary Material for this article can be found online at: https://www.frontiersin.org/articles/10.3389/fmars.2019.00080/full#supplementary-material
References
Andrady, A. L. (2011). Microplastics in the marine environment. Mar. Pollut. Bull. 62, 1596–1605. doi: 10.1016/j.marpolbul.2011.05.030
Araujo, C. F., Nolasco, M. M., Ribeiro, A. M., and Ribeiro-Claro, P. J. (2018). Identification of microplastics using Raman spectroscopy: latest developments and future prospects. Water Res. 142, 426–440. doi: 10.1016/j.watres.2018.05.060
Barnes, D. K., Galgani, F., Thompson, R. C., and Barlaz, M. (2009). Accumulation and fragmentation of plastic debris in global environments. Philos. Trans. R. Soc. Lond. B. 364, 1985–1998. doi: 10.1098/rstb.2008.0205
Bergmann, M., Wirzberger, V., Krumpen, T., Lorenz, C., Primpke, S., Tekman, M. B., et al. (2017). High quantities of microplastic in Arctic deep-sea sediments from the HAUSGARTEN observatory. Environ. Sci. Technol. 51, 11000–11010. doi: 10.1021/acs.est.7b03331
Borja, A., Elliott, M., Carstensen, J., Heiskanen, A. S., and van de Bund, W. (2010). Marine management–towards an integrated implementation of the European Marine strategy framework and the water framework directives. Mar. Pollut. Bull. 60, 2175–2186. doi: 10.1016/j.marpolbul.2010.09.026
Cathalot, C., van Oevelen, D., Cox, T. J. S., Kutti, T., Lavaleye, M., Duineveld, G., et al. (2015). Cold-water coral reefs and adjacent sponge grounds: hot spots of benthic respiration and organic carbon cycling in the deep sea. Front. Mar. Sci. 2:37. doi: 10.3389/fmars.2015.00037
Chapron, L., Peru, E., Engler, A., Ghiglione, J. F., Meistertzheim, A. L., Pruski, A. M., et al. (2018). Macro-and microplastics affect cold-water corals growth, feeding and behaviour. Sci. Rep. 8:15299. doi: 10.1038/s41598-018-33683-6
Cheshire, A. C., Adler, E., Barbière, J., Cohen, Y., Evans, S., Jarayabhand, S., et al. (2009). UNEP/IOC Guidelines on Survey and Monitoring of Marine Litter. Technical report, UNEP Regional Seas Reports and Studies, No. 186; IOC Technical Series No. 83.
Chiba, S., Saito, H., Fletcher, R., Yogi, T., Kayo, M., Miyagi, S., et al. (2018). Human footprint in the abyss: 30 year records of deep-sea plastic debris. Mar. Policy. 96, 204–212. doi: 10.1016/j.marpol.2018.03.022
Cole, M., Lindeque, P., Fileman, E., Halsband, C., Goodhead, R., Moger, J., et al. (2013). Microplastic ingestion by zooplankton. Environ. Sci. Technol. 47, 6646–6655. doi: 10.1021/es400663f
Cole, M., Lindeque, P., Halsband, C., and Galloway, T. S. (2011). Microplastics as contaminants in the marine environment: a review. Mar. Pollut. Bull. 62, 2588–2597. doi: 10.1016/j.marpolbul.2011.09.025
Courtene-Jones, W., Quinn, B., Ewins, C., Gary, S. F., and Narayanaswamy, B. E. (2018). Consistent microplastic ingestion by deep-sea invertebrates over the last four decades (1976–2015), a study from the North East Atlantic. Environ. Pollut. 244, 503–512. doi: 10.1016/j.envpol.2018.10.090
Courtene-Jones, W., Quinn, B., Gary, S. F., Mogg, A. O. M., and Narayanaswamy, B. E. (2017a). Microplastic pollution identified in deep-sea water and ingested by benthic invertebrates in the Rockall Trough, North Atlantic Ocean. Environ. Pollut. 231, 271–280. doi: 10.1016/j.envpol.2017.08.026
Courtene-Jones, W., Quinn, B., Murphy, F., Gary, S. F., and Narayanaswamy, B. E. (2017b). Optimisation of enzymatic digestion and validation of specimen preservation methods for the analysis of ingested microplastics. Anal. Method. 9, 1437–1445. doi: 10.1039/C6AY02343F
Cózar, A., Echevarría, F., González-Gordillo, J. I., Irigoien, X., Ubeda, B., Hernández-León, S., et al. (2014). Plastic debris in the open ocean. Proc. Natl. Acad. Sci. U.S.A. 111, 10239–10244. doi: 10.1073/pnas.1314705111
Davies, A. J., Duineveld, G. C., Lavaleye, M. S., Bergman, M. J., van Haren, H., and Roberts, J. M. (2009). Downwelling and deep-water bottom currents as food supply mechanisms to the cold-water coral Lophelia pertusa (Scleractinia) at the Mingulay Reef Complex. Limnol. Oceanogr. 54, 620–629. doi: 10.4319/lo.2009.54.2.0620
Douarin, M., Elliot, M., Noble, S. R., Sinclair, D., Henry, L.-A., Long, D., et al. (2013). Growth of north-east Atlantic cold-water coral structures during the Holocene: a high resolution U-series and 14C chronology. Earth Planet. Sci. Lett. 375, 176–187. doi: 10.1016/j.epsl.2013.05.023
Douarin, M., Sinclair, D., Elliot, M., Henry, L.-A., Long, D., and Roberts, J. M. (2014). Changes in fossil assemblage in sediment cores from Mingulay Reef Complex (NE Atlantic): implications for coral reef build-up. Deep Sea Res. II 99, 286–296. doi: 10.1016/j.dsr2.2013.07.022
Duineveld, G. C., Jeffreys, R. M., Lavaleye, M. S., Davies, A. J., Bergman, M. J., Watmough, T., et al. (2012). Spatial and tidal variation in food supply to shallow cold-water coral reefs of the Mingulay Reef complex (Outer Hebrides, Scotland). Mar. Ecol. Prog. Ser. 444, 97–115. doi: 10.3354/meps09430
Elliott, M., Boyes, S. J., Barnard, S., and Borja, Á. (2018). Using best expert judgement to harmonise marine environmental status assessment and maritime spatial planning. Mar. Pollut. Bull. 133, 367–377. doi: 10.1016/j.marpolbul.2018.05.029
Ellison, K. (2007). The trouble with nurdles. Front. Ecol. Environ. 5, 396–396. doi: 10.1890/1540-9295(2007)5[396:TTWN]2.0.CO;2
European Commission (2018). Fibres from Polyester Clothes Could be More Damaging to Marine Life than Microbeads. Available online at: http://ec.europa.eu/environment/integration/research/newsalert/pdf/fibres_from_polyester_clothes_could_be_more_damaging_marine_life_than_microbeads_509na1_en.pdf (Accessed 7 Jul 2018).
Galgani, F., Fleet, D., Van Franeker, J. A., Katsanevakis, S., Maes, T., Mouat, J., et al. (2010). “Marine Strategy Framework Directive-Task Group 10 Report marine litter do not cause harm to the coastal and marine environment,” in Report on the identification of descriptors for the Good Environmental Status of European Seas regarding marine litter under the Marine Strategy Framework Directive. Office for Official Publications of the European Communities, ed N. Zampoukas (Luxembourg) doi: 10.2788/86941
Galgani, F., Hanke, G., Werner, S. D. V. L., and De Vrees, L. (2013). Marine litter within the European marine strategy framework directive. ICES J. Mar. Sci. 70, 1055–1064. doi: 10.1093/icesjms/fst122
Ghosal, S., Chen, M, Wagner, J., Wang, Z.-M., and Wall, S. (2018). Molecular Identification of polymers and anthropogenic particles extracted from oceanic water and fish stomach- a Raman micro-spectroscopy study. Environ. Pollut. 233, 1113–1124. doi: 10.1016/j.envpol.2017.10.014
Henry, L.-A., Davies, A., and Roberts, J. M. (2010). Beta diversity of cold-water coral reef communities off western Scotland. Coral Reefs 29, 427–436. doi: 10.1007/s00338-009-0577-6
Henry, L.-A., Navas, J. M., and Roberts, J. M. (2013). Multi-scale interactions between local hydrography, seabed topography, and community assembly on cold-water coral reefs. Biogeosciences 10, 2737–2746. doi: 10.5194/bg-10-2737-2013
Henry, L. A., and Roberts, J. M. (2007). Biodiversity and ecological composition of macrobenthos on cold-water coral mounds and adjacent off-mound habitat in the bathyal Porcupine Seabight, NE Atlantic. Deep Sea Res. Part I 54, 654–672. doi: 10.1016/j.dsr.2007.01.005
HM Government (2012). Marine Strategy Part One: UK Initial Assessment and Good Environmental Status. Department for Environment and Rural Affairs.
HM Government (2015). Marine Strategy Part Three: UK Programme of Measures. Department for Environment and Rural Affairs.
Huvenne, V. A. I., Masson, D. G., and Wheeler, A. J. (2009). Sediment dynamics of a sandy contourite: the sedimentary context of the Darwin cold-water coral mounds, Northern Rockall Trough. Intern. J. Earth Sci. 98, 865–884. doi: 10.1007/s00531-008-0312-5
Inall, M. (2009). “RRS discovery cruise 340b. dunstaffnage to govan via barra head and the surrounding shelf. 26th June to 4th July 2009,” in Scottish Association for Marine Science Internal Report No. 265, 124.
Jambeck, J. R., Geyer, R., Wilcox, C., Siegler, T. R., Perryman, M., Andrady, A., et al. (2015). Plastic waste inputs from land into the ocean. Sci. 347, 768–771. doi: 10.1126/science.1260352
Jamieson, A. J., Malkocs, T., Piertney, S. B., Fujii, T., and Zhang, Z. (2017). Bioaccumulation of persistent organic pollutants in the deepest ocean fauna. Nat. Ecol. Evol. 1:0051. doi: 10.1038/s41559-016-0051
Jefferson, R. L., Bailey, I., Richards, J. P., and Attrill, M. J. (2014). Public perceptions of the UK marine environment. Mar. Policy 43, 327–337. doi: 10.1016/j.marpol.2013.07.004
Kazanidis, G., Henry, L.-A., Roberts, J. M., and Witte, U. F. M. (2016). Biodiversity of Spongosorites coralliophaga (Stephens, 1915) on coral rubble at two contrasting cold-water coral reef settings. Coral Reefs 35, 193–208. doi: 10.1007/s00338-015-1355-2
Kazanidis, G., van Oevelen, D., Veuger, B., and Witte, U. F. M. (2018). Unravelling the versatile feeding and metabolic strategies of the cold-water ecosystem engineer Spongosorites coralliophaga (Stephens, 1915). Deep Sea Res. Part I. 141, 71–82. doi: 10.1016/j.dsr.2018.07.009
Kazanidis, G., and Witte, U. F. (2016). The trophic structure of Spongosorites coralliophaga-coral rubble communities at two northeast Atlantic cold water coral reefs. Mar. Biol. Res. 12, 932–947. doi: 10.1080/17451000.2016.1216569
La Beur, L., Henry, L.-A.;, Kazanidis, G., Hennige, S.;, McDonald, A., Shaver, M., and Roberts, J. M. (2019). Ingested Microplastics Identified from Macrobenthos Collected during the RRS Discovery D340b Research Cruise to the Mingulay Reef Complex (Outer Hebrides, western Scotland, Northeast Atlantic). PANGAEA, doi: 10.1594/PANGAEA.897250
Law, K. L., Morét-Ferguson, S., Maximenko, N. A., Proskurowski, G., Peacock, E. E., Hafner, J., et al. (2010). Plastic accumulation in the North Atlantic subtropical gyre. Science 329, 1185–1188. doi: 10.1126/science.1192321
Lobelle, D., and Cunliffe, M. (2011). Early microbial biofilm formation on marine plastic debris. Mar. Pollut. Bull. 62, 197–200. doi: 10.1016/j.marpolbul.2010.10.013
Lusher, A. L., Burke, A., O'Connor, I., and Officer, R. (2014). Microplastic pollution in the Northeast Atlantic Ocean: validated and opportunistic sampling. Mar. Pollut. Bull. 88, 325–333. doi: 10.1016/j.marpolbul.2014.08.023
Mato, Y., Isobe, T., Takada, H., Kanehiro, H., Ohtake, C., and Kaminuma, T. (2001). Plastic resin pellets as a transport medium for toxic chemicals in the marine environment. Environ. Sci. Technol. 35, 318–324. doi: 10.1021/es0010498
Miller, M. E., Kroon, F. J., and Motti, C. A. (2017). Recovering microplastics from marine samples: a review of current practices. Mar. Pollut. Bull. 123, 6–18. doi: 10.1016/j.marpolbul.2017.08.058
Milligan, R. J., Spence, G., Roberts, J. M., and Bailey, D. M. (2016). Fish communities associated with cold-water corals vary with depth and substratum type. Deep Sea Res. Part I. 114, 43–54. doi: 10.1016/j.dsr.2016.04.011
Miyake, H., Shibata, H., and Furushima, Y. (2011). “Deep-sea litter study using deep-sea observation tools” in Interdisciplinary Studies on Environmental Chemistry-Marine Environmental Modeling and Analysis, eds K. Omori, X. Guo, N. Yoshie, N. Fujii, I.C. Handoh, A. Isobe, and S. Tanabe (Tokyo: TERRAPUB), 261–269.
Moore, C. J. (2008). Synthetic polymers in the marine environment: a rapidly increasing, long-term threat. Environ. Res. 108, 131–139. doi: 10.1016/j.envres.2008.07.025
Navas, J. M., Miller, P. L., Henry, L. A., Hennige, S. J., and Roberts, J. M. (2014). Ecohydrodynamics of cold-water coral reefs: a case study of the Mingulay Reef Complex (Western Scotland). PLoS ONE 9:e98218. doi: 10.1371/journal.pone.0098218
OSPAR (2018). Marine Litter. Available online at: https://www.ospar.org/work-areas/eiha/marine-litter (Accessed Jul 20, 2018).
Pahl, S., Wyles, K. J., and Thompson, R. C. (2017). Channeling passion for the ocean towards plastic pollution. Nat. Hum. Behav. 1:697. doi: 10.1038/s41562-017-0204-4
Pham, C. K., Ramirez-Llodra, E., Alt, C. H., Amaro, T., Bergmann, M., Canals, M., et al. (2014). Marine litter distribution and density in European seas, from the shelves to deep basins. PLoS ONE 9:e95839. doi: 10.1371/journal.pone.0095839
Reisser, J. W., Slat, B., Noble, K. D., Plessis, K. D., Epp, M., Proietti, M. C., et al. (2015). The vertical distribution of buoyant plastics at sea: an observational study in the North Atlantic Gyre. Biogeosciences 12, 1249–1256. doi: 10.5194/bg-12-1249-2015
Roberts, C. M. (2003). Our shifting perspectives on the oceans. Oryx 37, 166–177. doi: 10.1017/S0030605303000358
Roberts, J. M., Brown, C. J., Long, D., and Bates, C. R. (2005) Acoustic mapping using a multibeam echosounder reveals cold-water coral reefs surrounding habitats. Coral Reefs 24, 654–669. doi: 10.1007/s00338-005-0049-6
Roberts, J. M., Davies, A. J., Henry, L.-A., Dodds, L. A., Duineveld, G. C. A., Lavaleye, M. S., et al. (2009). Mingulay reef complex: an interdisciplinary study of cold-water coral habitat, hydrography and biodiversity. Mar. Ecol. Prog. Ser. 397, 139–151. doi: 10.3354/meps08112
Roberts, J. M., Wheeler, A. J., and Freiwald, A. (2006). Reefs of the deep: the biology and geology of cold-water coral ecosystems. Science 312, 543–547. doi: 10.1126/science.1119861
Rocha-Santos, T., and Duarte, A. C. (2015). A critical overview of the analytical approaches to the occurrence, the fate and the behavior of microplastics in the environment. Trends Anal. Chem. 65, 47–53. doi: 10.1016/j.trac.2014.10.011
Rochman, C. M., Cook, A. M., and Koelmans, A. A. (2016). Plastic debris and policy: Using current scientific understanding to invoke positive change. Environ. Toxicol. Chem. 35, 1617–1626. doi: 10.1002/etc.3408
Rochman, C. M., Tahir, A., Williams, S. L., Baxa, D. V., Lam, R., Miller, J. T., et al. (2015). Anthropogenic debris in seafood: Plastic debris and fibres from textiles in fish and bivalves sold for human consumption. Sci. Rep. 5:14340. doi: 10.1038/srep14340
Ryland, J. S. (2000). Reproduction in British zoanthids, and an unusual process in Parazoanthus anguicomus. J. Mar. Biol. Assoc. UK 80, 943–944.
Scottish Government (2014). Consultation on the Management of Inshore Special Areas of Conservation and Marine Protected Areas Approaches. Available online at: http://www.gov.scot/Publications/2014/11/9361/7 (Accessed July 9,2018).
Scottish Government (2018). Marine Litter Strategy Group. Available online at: http://www.gov.sscot/Topics/marine/marine-environment/litter/MLS (Accessed June 27, 2018).
Sheavly, S. B., and Register, K. M. (2007). Marine debris and plastics: environmental concerns, sources, impacts and solutions. J. Polym. Environ. 15, 301–305. doi: 10.1007/s10924-007-0074-3
Taylor, M. L., Gwinnett, C., Robinson, L. F., and Woodall, L. C. (2016). Plastic microfibre ingestion by deep-sea organisms. Sci. Rep. 6:33997. doi: 10.1038/srep33997
Torre, M., Digka, N., Anastasopoulou, A., Tsangaris, C., and Mytilineou, C. (2016). Anthropogenic microfibres pollution in marine biota. A new and simple methodology to minimize airborne contamination. Mar. Pollut. Bull. 113, 55–61. doi: 10.1016/j.marpolbul.2016.07.050
Tubau, X., Canals, M., Lastras, G., Rayo, X., Rivera, J., and Amblas, D. (2015). Marine litter on the floor of deep submarine canyons of the Northwestern Mediterranean Sea: the role of hydrodynamic processes. Prog. Oceanogr. 134, 379–403. doi: 10.1016/j.pocean.2015.03.013
Van Cauwenberghe, L., Vanreusel, A., Mees, J., and Janssen, C. R. (2013). Microplastic pollution in deep-sea sediments. Environ. Pollut. 182, 495–499. doi: 10.1016/j.envpol.2013.08.013
Van Franeker, J. A., Blaize, C., Danielsen, J., Fairclough, K., Gollan, J., Guse, N., et al. (2011). Monitoring plastic ingestion by the northern fulmar Fulmarus glacialis in the North Sea. Environ. Pollut. 159, 2609–2615. doi: 10.1016/j.envpol.2011.06.008
Van Franeker, J. A., and Law, K. L. (2015). Seabirds, gyres and global trends in plastic pollution. Environ. Pollut. 203, 89–96. doi: 10.1016/j.envpol.2015.02.034
Vince, J., and Stoett, P. (2018). From problem to crisis to interdisciplinary solutions: Plastic marine debris. Mar. Policy 96, 200–203. doi: 10.1016/j.marpol.2018.05.006
Woodall, L. C., Gwinnett, C., Packer, M., Thompson, R. C., Robinson, L. F., and Paterson, G. L. (2015). Using a forensic science approach to minimize environmental contamination and to identify microfibres in marine sediments. Mar. Pollut. Bull. 95, 40–46. doi: 10.1016/j.marpolbul.2015.04.044
Woodall, L. C., Sanchez-Vidal, A., Canals, M., Paterson, G. L., Coppock, R., Sleight, V., et al. (2014). The deep sea is a major sink for microplastic debris. R. Soc. Open Sci. 1:140317. doi: 10.1098/rsos.140317
Wright, S. L., Rowe, D., Thompson, R. C., and Galloway, T. S. (2013). Microplastic ingestion decreases energy reserves in marine worms. Curr. Biol. 23, R1031–R1033. doi: 10.1016/j.cub.2013.10.068
Keywords: marine litter, microplastic, marine strategy framework directive, good environmental status, cold-water corals, Raman spectroscopy
Citation: La Beur L, Henry L-A, Kazanidis G, Hennige S, McDonald A, Shaver MP and Roberts JM (2019) Baseline Assessment of Marine Litter and Microplastic Ingestion by Cold-Water Coral Reef Benthos at the East Mingulay Marine Protected Area (Sea of the Hebrides, Western Scotland). Front. Mar. Sci. 6:80. doi: 10.3389/fmars.2019.00080
Received: 15 November 2018; Accepted: 11 February 2019;
Published: 05 March 2019.
Edited by:
Cinzia Corinaldesi, Polytechnical University of Marche, ItalyReviewed by:
Autun Purser, Alfred Wegener Institute Helmholtz Centre for Polar and Marine Research (AWI), GermanyChristine Ferrier-Pagès, Scientific Centre of Monaco, Monaco
Copyright © 2019 La Beur, Henry, Kazanidis, Hennige, McDonald, Shaver and Roberts. This is an open-access article distributed under the terms of the Creative Commons Attribution License (CC BY). The use, distribution or reproduction in other forums is permitted, provided the original author(s) and the copyright owner(s) are credited and that the original publication in this journal is cited, in accordance with accepted academic practice. No use, distribution or reproduction is permitted which does not comply with these terms.
*Correspondence: Lea-Anne Henry, bC5oZW5yeUBlZC5hYy51aw==