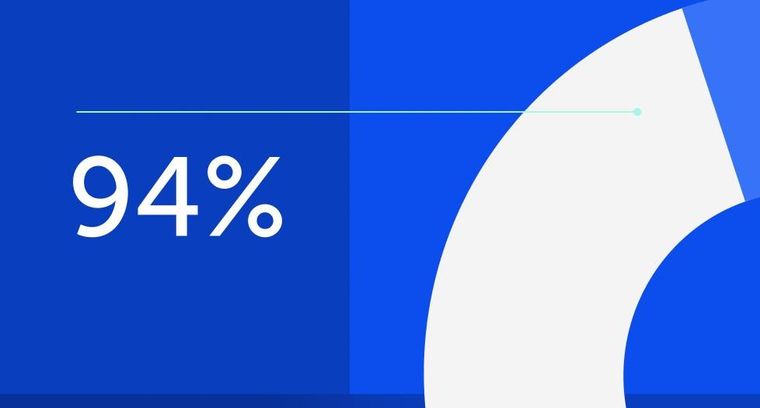
94% of researchers rate our articles as excellent or good
Learn more about the work of our research integrity team to safeguard the quality of each article we publish.
Find out more
REVIEW article
Front. Mar. Sci., 18 February 2019
Sec. Ocean Observation
Volume 6 - 2019 | https://doi.org/10.3389/fmars.2019.00031
This article is part of the Research TopicOceanobs'19: An Ocean of OpportunityView all 136 articles
This paper reviews the design of the Tropical Pacific Observing System (TPOS) and its governance and takes a forward look at prospective change. The initial findings of the TPOS 2020 Project embrace new strategic approaches and technologies in a user-driven design and the variable focus of the Framework for Ocean Observing. User requirements arise from climate prediction and research, climate change and the climate record, and coupled modeling and data assimilation more generally. Requirements include focus on the upper ocean and air-sea interactions, sampling of diurnal variations, finer spatial scales and emerging demands related to biogeochemistry and ecosystems. One aim is to sample a diversity of climatic regimes in addition to the equatorial zone. The status and outlook for meeting the requirements of the design are discussed. This is accomplished through integrated and complementary capabilities of networks, including satellites, moorings, profiling floats and autonomous vehicles. Emerging technologies and methods are also discussed. The outlook highlights a few new foci of the design: biogeochemistry and ecosystems, low-latitude western boundary currents and the eastern Pacific. Low latitude western boundary currents are conduits of tropical-subtropical interactions, supplying waters of mid to high latitude origin to the western equatorial Pacific and into the Indonesian Throughflow. They are an essential part of the recharge/discharge of equatorial warm water volume at interannual timescales and play crucial roles in climate variability on regional and global scales. The tropical eastern Pacific, where extreme El Niño events develop, requires tailored approaches owing to the complex of processes at work there involving coastal upwelling, and equatorial cold tongue dynamics, the oxygen minimum zone and the seasonal double Intertropical Convergence Zone. A pilot program building on existing networks is envisaged, complemented by a process study of the East Pacific ITCZ/warm pool/cold tongue/stratus coupled system. The sustainability of TPOS depends on effective and strong collaborative partnerships and governance arrangements. Revisiting regional mechanisms and engaging new partners in the context of a planned and systematic design will ensure a multi-purpose, multi-faceted integrated approach that is sustainable and responsive to changing needs.
Sustained and systematic observation of the tropical Pacific Ocean has been a priority for nations around the basin since the 1980s, driven principally by the global climate effects of the El Niño/Southern Oscillation (ENSO), and by the demonstrated prediction skill based on ocean and air-sea interface observations. Increasingly planned and based on defined science goals, this Tropical Pacific Observing System (TPOS) has served the community well as a long-term and routine contribution to the Global Climate Observing System [GCOS] (2014a,b), delivering measurements that advance our capability to describe, understand and predict ENSO and climate variability more generally (McPhaden et al., 1998, 2010).
The TPOS supports research that underpins improvements in prediction and contributes to detecting climate change. Benefits of the TPOS manifest over multiple sectors and into regions remote from the tropical Pacific; agriculture and water management (Sassone and Weiher, 1997; Lazo et al., 2011; World Meteorological Organization [WMO], 2012; Centre for International Economics, 2014), marine ecosystems and fisheries (Chavez et al., 2014; Tommasi et al., 2016), human health and well-being (Fischer et al., 2010; World Meteorological Organization [WMO], 2012), disaster preparedness (Hoeke et al., 2013; Cashin et al., 2014; Harrison et al., 2014) and health/well-being of terrestrial living systems (Intergovernmental Panel on Climate Change, 2014) are just some of the sectors that benefit directly and indirectly from the TPOS, often via sophisticated El Niño prediction systems.
The TPOS came under pressure during the period 2012–2014 when logistical and other support for the Pacific tropical moored buoy array (TMA) was reduced, leading to inferior data returns (around 40% compared with the usual 80–90%; see Figure 1-1 in Cravatte et al., 2016) and, in the western Pacific, a phased decommissioning of around thirteen sites. The central and eastern Pacific data returns were back to normal by 2015, but there are now only two TRITON sites west of 165E. Several studies suggest this degradation compromised our ability to monitor climate variability and consequently to model and predict ENSO (Tollefson, 2014; Fujii et al., 2015, 2019; Chiodi and Harrison, 2017a,b; Xue et al., 2017). This challenging period should be seen in the context of a TPOS capability that had grown significantly over the last two decades and highlighted points of vulnerability that were not well understood or managed (Ando et al., 2017; Serra, 2018). The TPOS 2020 Project was initiated to improve our understanding of the collective TPOS capability, beyond its individual parts, and to provide a stronger, more capable integrated approach with improved governance and management of risk (see the TPOS 2020 First Report, Cravatte et al., 2016, hereafter referred to as the First Report). In doing so, improved knowledge of relevant phenomena and spatiotemporal variability are considered for the design, and emerging technologies and new techniques will form part of the recommended response to requirements.
The TPOS 2020 Project does not directly consider regional and local requirements and observing system responses. Global Climate Observing System [GCOS] (2014b) included two papers that addressed Pacific Island (Wiles et al., 2014) and eastern Pacific (Takahashi et al., 2014) regional needs and these were considered by Cravatte et al. (2016). The priority given to the western Pacific (see The TPOS 2020 First Report and Low-Latitude Western Boundary Current Systems) partly reflects the demand for improved severe weather and climate outlooks. Section “Eastern Pacific” discusses some of the eastern Pacific coastal aspects.
The TPOS 2020 approach to the initial design (Figure 1) followed the Framework for Ocean Observing (Lindstrom et al., 2012; Tanhua et al., 2019, this issue), beginning with an analysis of user demand, then the driving phenomena, and followed by specification of the required sampling (essential variables, spatiotemporal characteristics, quality, etc.). With this approach, Essential Ocean Variable (EOV) requirements do not tell us which platforms or techniques are needed but establish a target for the collective observational capability (the general and specific responses). A variable requirement may be responding to multiple needs and uses, including those underpinning basic scientific research.
Figure 1. Schematic of the process deployed by TPOS 2020 in the First Report (an adaptation of the Framework for Ocean Observing, Lindstrom et al., 2012). The design is user driven and some form of stakeholder “market” analysis is required to define needed products, including resolution, accuracy and so on. In some cases (e.g., research) the users may provide this specification in terms of variables. The second level is a set of requirements expressed in terms of characteristics (scales, etc.) of Essential Ocean/Climate Variables. At the next level the TPOS 2020 considered a general response in the form of recommendations; these may be thought of as observing system requirements. The specific response (level four) is expressed as a set of actions and recommendations at the level of networks and platforms. Finally, and most importantly, partners in the TPOS (a 2nd set of stakeholders) contribute the needed observational capability and capacity. Feedback occurs as part of the process, and directly between the final level (partners) and users/beneficiaries (the arrows from bottom right to top left).
The design stage, and establishing requirements in particular, is critical for priority setting. The user and product requirements determine essential inputs which are expressed in terms of EOVs. Priorities are established for the general response and specific actions based on these higher-level requirements. It is a subjective process, relying on experts and stakeholder feedback.
End-user engagement is built into the TPOS 2020 approach, through its governance and through its review process, whereby both experts and general stakeholders (users of data; providers of data; beneficiaries) are invited to critique the design.
The recommended general response and specific actions of the TPOS 2020 initial design comprises a mix of experimental and sustained approaches, and it is the latter that constitutes the Backbone of TPOS: the fundamental and core contributions to the sustained observing system (Cravatte et al., 2016). The recommendations of the First Report for the Backbone are generally not platform-specific, nor do they refer to any single nation or individual effort. They are written in a form that prioritizes need but otherwise leaves flexibility in the hands of the providers and operators of measurement networks (see Section “Phased Changes to the Backbone” for further detail).
The recommended response is generally an ambitious target and, if fully met, represents the ideal observing system strategy for the tropical Pacific.
The First Report (Cravatte et al., 2016) takes advantage of developments in both satellite and in situ networks over the last 20 years, including multiple scatterometers, multiple altimeters, global Argo and advances in mooring and other technology. It advocates for an integrated, multi-faceted approach in which in situ systems support satellite measurements, and vice versa, and the strengths of particular platforms are brought together to produce an observing system that is stronger than the sum of its individual contributions.
The new Backbone TPOS design aims to deliver to five key functions:
(1) Provide data in support of, and to evaluate, validate and initialize, ENSO prediction and other forecasting systems and to foster their advancement;
(2) Provide observations to quantify the evolving state of the surface and subsurface ocean;
(3) Support integration of satellite and in situ approaches including calibration and validation;
(4) Advance understanding and modeling of the climate system in the tropical Pacific, including through the provision of observing system infrastructure for process studies; and
(5) Maintain and extend the tropical Pacific climate record.
Present and future elements have been assessed against their ability to deliver to these functions, with high priority given to those that contribute to many uses. This framework, along with the development of the capabilities of Argo and space-based sensors, leads to a reassessment of the role of the TMA. Moorings’ unique capabilities provide excellent temporal resolution of many different oceanographic, meteorological, and biogeochemical EOVs, enabling diagnosis of the spectral characteristics of phenomena and specification of the errors inherent in sparser-in-time sampling. They can provide collocated ocean and atmosphere observations, with the full suite of variables to estimate air-sea fluxes. However, today Argo greatly improves the vertical resolution of subsurface features, and samples between the TMA lines, while satellites provide unprecedented spatial coverage and resolution, capturing the synoptic atmospheric variability that partly drives the net surface fluxes.
In the new TPOS Backbone, we have sought to exploit the complementarities among platforms to underpin a major advance in our capability to monitor the tropical Pacific Ocean and surface atmosphere. We approach the observing system as a network, where individual components, each with their strengths, combine to provide robustness, reinforcement, cross-checking, and greater overall resilience. We see state estimation and its exploitation of this complementary between satellite and in situ observations as fundamental, empowering both research and operational users of the observing system. Beyond the goal of tracking the system state, TPOS 2020 has also targeted observations needed to challenge and help improve model physics (Cravatte et al., 2016; their section 2.6.8), particularly for mixing and ageostrophic dynamics on the equator and upper ocean-atmosphere coupling at sub-daily timescales. Associated with this latter challenge is the need to describe these processes across key weather and ocean regimes.
Maintaining a credible climate record across a redesign of the observing system has been a challenging and at times controversial aspect of this project. It was risk to the climate record which drove the creation of the TPOS 2020 project, with deterioration of the TMA in the early 2010s giving a concrete demonstration of the precariousness of long-term observations. Changing locations and sampling patterns of longstanding measurements may cause a loss of some information but insisting that time series of every variable or site be maintained in the context of changing observing technology, science issues and user needs is not compelling. A climate record is more than a time series at a point (Cravatte et al., 2016); in reality it comprises any systematic observations that accurately describe the evolution of phenomena.
For TPOS 2020, balancing the requirements of maintaining a credible and robust climate record against new requirements and opportunities occupied many months of study and debate, and indeed, has directed much of our work. We are striving for a balance between detecting long term change across the full TPOS and making new observations that will fuel novel insights into key processes and challenge the next generation of coupled models.
In the new TPOS Backbone the function of monitoring the state of the ocean and air-sea interface will be largely delivered through a combination of satellite-based sensors, autonomous floats and a reconfigured moored array, along with traditional elements of the observing system (e.g., drifters, high-resolution XBT, tide gauges, hydrographic sections), while the function of driving forward our understanding of key regimes and poorly understood physical processes will be delivered through enhancements that might inform on potentially missing elements. The special role that some in situ measurements play for the calibration and validation of space-based observations, including multiple mission products, will also be preserved or enhanced.
The major recommendations from the First Report are given below, with focus on those that represent a specific TPOS response (refer to the Executive Summary http://tpos2020.org/first-report/ for details; recommendations are referenced in [brackets]).
• Improve coverage and reduce diurnal cycle aliasing of vector winds with a constellation of multi-frequency scatterometer missions and complementary wind speed measurements from microwave sensors and in situ to enable all-weather wind retrievals over 90% of the tropical Pacific Ocean every 6 h [Recommendations 1, 2]. In situ wind and surface current data play a vital role as reference data and in the climate record.
• Continue and enhance space-based precipitation measurements complemented by expansion of the in situ moored siphon rain gauges covering diverse rainfall climate regimes [Recommendations 8, 9].
• Enhance in situ observations of the state variables needed to estimate the full surface heat and freshwater fluxes, with emphasis given to key regimes and promoting pilot studies of new platforms. This will be accompanied by the promotion and support of studies to improve wind stress products and to understand the sensitivity and impact of observed air-sea flux variables in forecast and reanalysis systems [Recommendation 15 and Actions 6–11].
• Continuation of high-frequency, moored time series and broad spatial scale underway surface ocean pCO2 observations across the Pacific from 10°S to 10°N [Recommendation 12].
• Continuity of complementary satellite and in situ sea surface salinity (SSS) measurement networks, with a focus on improved satellite accuracy [Recommendation 10]. Recent results (e.g., Boutin et al., 2018; Hasson et al., 2018) show improvements in the quality of SSS retrievals, encouraging TPOS 2020 to review this recommendation.
• Recommendations for other surface variables (sea surface temperature (SST), sea surface height, ocean color, mean sea level pressure) follow those of other global observing system plans (for example, Global Climate Observing System [GCOS], 2016) [Recommendation 3–7, 13]; the importance of passive microwave SST measurements is highlighted.
• Existing technologies cannot meet surface current velocity requirements. The First Report encourages efforts to measure surface velocity from space [Recommendation 11].
• Broad-scale sampling of subsurface temperature and salinity is required, with enhanced resolution through the tropics, and better meridional spacing and increased vertical resolution in the equatorial region. Additional targets are to resolve near-surface salinity stratification and to improve sampling of equatorial currents [Recommendations 16–20]. More specifically:
◦ Target the fast, coupled upper ocean physics across key regimes: enhance mooring resolution in the upper layer and expand the moored array poleward to give broader coverage and better temporal resolution of faster processes and the parameters needed to estimate total surface fluxes and the mixed layer response;
◦ Double Argo profile returns between 10°N – 10°S, starting in the western Pacific;
◦ Restore the equatorial moored array in the west;
◦ Target the circulation and physics on the equator: maintain and expand direct measurements of velocity on the equator across the basin; and
◦ Expand direct velocity sampling, initially at 140°W, to better resolve the meridional structure of the Equatorial Undercurrent (EUC) and the advection and mixing processes where the EUC interacts with the mixed layer.
The value added from the proposed TPOS 2020 Backbone is sketched in Figure 2 while Figure 3 shows the proposed changes to the core TMA mooring configuration. Mooring sampling is returned and enhanced to the equatorial west Pacific west of 165°E and the TMA is reconfigured and enhanced elsewhere (Figure 2, top panels). Surface mooring enhancements provide the full flux variables needed for bulk flux estimation. Velocity measurements are increased from TMA near the surface and subsurface (Figure 2, 2nd and 3rd panels down; Figure 3) while doubling Argo enhances the sampling of temperature and salinity in the equatorial region (bottom two panels). The reconfiguration of the moored array and Argo from its present form should be staged in a way that allows testing and evaluation and an orderly transition. Discussions have commenced on the technical detail and on how the transition should be managed (see Phased Changes to the Backbone).
Figure 2. Schematic showing data returns from the TMA and Argo for key variables of interest between the present observing system (left) and the proposed TPOS 2020 Backbone (right). Observations from the TMA are shown in large circles and Argo in smaller dots. Improvements in surface flux, velocity and subsurface sampling are expected. Further extensions of the TMA are under consideration.
Figure 3. Schematic of recommended enhancements to standard TAO moorings. Standard measurement suite shown in red; enhancements in blue. Depths (m) of the subsurface instruments are listed by each symbol. The enhancements are designed to provide quantities for bulk flux estimation and to take advantage of moorings’ high temporal sampling rates for the rapid-evolving, short-vertical-scale phenomena of the surface mixed layer, including velocity. Shallow velocity measurements are also needed to reference satellite scatterometer winds to the surface current, a particular problem in the tropics where currents are strong and winds relatively weak.
Autonomous observing and forecast development has expanded beyond physical parameters. Past TMA physical data have been of great value for the development of next generation ecosystem and operational fisheries forecasts, but biogeochemical observations are necessary to make further improvements in these models. The region’s large carbon signal and economic reliance on diverse and productive ecosystems emphasize the need to advance understanding of tropical Pacific biogeochemical variability and predictability.
The tropical Pacific is a region with unique and highly variable biogeochemical signatures over space and time (Figure 4). This region is the largest oceanic source of CO2 to the atmosphere, supplying up to 1 petagram of carbon annually with considerable ENSO-driven interannual variability (e.g., Chavez et al., 1999; Feely et al., 2006). Poor constraint of these large interannual signals could double the uncertainty in annual carbon budgeting of CO2 emissions and their redistribution among the atmosphere, ocean, and terrestrial biosphere (Le Quéré et al., 2018). Globally, ocean warming and stratification are causing a decline in oceanic dissolved oxygen. Models suggest that further expansion of the already extensive Oxygen Minimum Zone (OMZ) in the tropics could reduce habitat, shift distributions of marine species, and change ecosystem structure (Stramma et al., 2012).
Figure 4. Summary of long term trends and example El Niño (January 1998) vs La Niña (July 1998) conditions for important biogeochemical parameters. (A) Long term trends in surface ocean and atmospheric pCO2. The colored lines indicate surface ocean pCO2 from tropical Pacific moorings, all at 0° (Sutton et al., 2018). The black line is atmospheric pCO2 (in ppm) from a station on Easter Island (Dlugokencky et al., 2018). (B,C) Gridded surface ocean pCO2 from Landschützer et al. (2017). (D,E) SeaWiFS satellite monthly mean surface chlorophyll concentration. The filled circles represent the magnitude of the skipjack tuna catch for El Niño (D, January–June 1992) and La Niña (E, January–June 1989), adapted from Lehodey et al. (1997). (F,G) Surface nitrate concentrations from the NASA Ocean Biogeochemical Model (NOBM; Gregg and Casey, 2007; https://giovanni.gsfc.nasa.gov/giovanni/). The latitude/longitude domain in (B) through (G) is 20°N to 20°S, 120°E to 80°W.
Despite being iron-limited, tropical Pacific waters are moderately productive above the OMZ and serve as globally significant regions of biologically fueled carbon sequestration (Mathis et al., 2014). The organic matter produced in the eastern and central tropical Pacific that escapes upper ocean remineralization is exported to the deep ocean. Conversely, in the warm pool thermal stratification restricts subsurface nutrients from entering the euphotic zone, resulting in low surface nutrients, primary productivity and carbon export.
The western boundary currents feeding the EUC partially regulate biological productivity through entrainment of iron from the shelves (Ryan et al., 2006). Further upstream, these feeder currents also derive pre-formed inorganic carbon from the surface of the north and south Pacific and dissolved inorganic carbon, due to remineralization, as they transit toward the EUC. Both of these processes contribute to the upwelling and degassing of CO2 in the central and eastern Pacific. Quantifying the volume fluxes into the EUC is important for understanding the relative importance of local versus remote forcing to the observed variability in biological productivity and air-sea CO2 fluxes. In addition to these applications to biogeochemistry, inflow to the EUC is also central to understanding the heat and freshwater budget of the tropical Pacific, which was discussed at length in the First Report.
Primary productivity in the southeastern tropical Pacific supports the largest single species fishery in the world, the Peruvian anchoveta, where real-time TMA data are used to make present-day fisheries management decisions. The western and central tropical Pacific supports over 50% of the global tuna industry, a significant resource (as much as 40% of the Gross Domestic Product) for the region’s island nations (Lehodey et al., 1997; Chavez et al., 2003). Abundance and distribution of these fish populations correlate with ENSO (and in some cases Pacific Decadal Oscillation); however, it is not clear how the coupling between physics and biogeochemistry drive changes at these higher trophic levels (Chavez et al., 2014). Tropical Pacific physical fluctuations have a deep impact on these and other living marine resources, including protected species such as coral reefs, sea turtles, and cetaceans.
Initial recommendations for integrating biogeochemistry into TPOS focused on (1) sustaining and expanding established observations, such as air-sea CO2 fluxes and satellite ocean color and (2) pursuing data synthesis and new technology pilot projects to refine the critical time and space scales for biogeochemistry (Cravatte et al., 2016). The final backbone observing system design must provide additional key observations that will underpin research to better understand both climate impacts and connections to higher trophic levels. The biogeochemical and ecosystem processes of the tropical Pacific driving this design are:
• tropical Pacific biogeochemical and ecosystem response to climate change, including consequences of OMZ variability and change to higher trophic level habitat;
• seasonal to decadal variability of the Tropical Pacific biological pump to allow biogeochemical model/forecast development and assessment of ecosystem impact;
• seasonal to decadal variability of tropical Pacific CO2 flux and implications for the global carbon cycle;
• upper ocean carbon budget including carbon export below the mixed layer and sources of anthropogenic carbon to equatorial Pacific upwelled water; and
• volume and nutrient fluxes into the EUC to understand how this variability modulates biological variability in the central and eastern Pacific.
These processes drive the major biogeochemical requirements in TPOS to maintain a climate record of air-sea CO2 flux and to resolve seasonal to interannual water column variability of inorganic carbon, oxygen, chlorophyll, particles, and nutrients, which are all EOVs. These requirements can be met by maintaining air-sea CO2 records on ships of opportunity and buoys (including new sites in the western Pacific), enhancing at least one third of Argo enhancements in the region with biogeochemical sensors, re-establishing CTD and bottle sampling on mooring servicing cruises to 1000 m, and continued biogeochemical-related satellite programs.
Biogeochemical (BGC)-Argo observations in the tropical Pacific have the potential to characterize seasonal to interannual variability of dissolved oxygen and the OMZ, inorganic carbon, chlorophyll, and nitrate, as well as the mechanisms driving the biological pump. BGC-Argo data combined with ship-based validation data and surface ocean CO2 flux measurements could also be used to estimate vertical gradients in carbon chemistry, to calculate upper ocean carbon budgets and export. Maintaining and expanding high-quality observations (surface pCO2 and ship-based bottle samples) will be required to validate new and emerging platforms and biogeochemical sensors like BGC-Argo and autonomous surface vehicles.
Sustained, repeat hydrographic surveys of biogeochemical observations would provide the opportunity to measure biogeochemical phenomena that cannot currently be measured autonomously and provide a climate-quality time series to validate autonomous sensors with larger measurement uncertainties. On mooring servicing vessels, the CTD package should be equipped with dissolved oxygen and optical sensors. Water samples for chlorophyll and nutrients should be routine, while other GO-SHIP parameters such as dissolved trace elements, inorganic carbon, particulate organic carbon, transient tracers, N2O, C isotopes and dissolved organic carbon should be accommodated where possible, probably through the involvement of funded investigators. These measurements should continue on GO-SHIP voyages (on average, one GO-SHIP voyage per year intersects the tropical Pacific), as well as process study cruises.
These backbone observations combined with process studies and continued observing technology development would be foundational to biogeochemical model development and assessments of current and future ecosystem impact. Occasional quantification of volume fluxes into the EUC in conjunction with biological pump process studies and modeling efforts, for example, would help to identify how iron sources drive biological production and carbon export. This would address how coupling between physics and biogeochemistry drives changes at higher trophic levels and how sensitive biological carbon drawdown is to variability and change. This would also constrain anthropogenic signals in the dissolved inorganic carbon content of the EUC and its source waters.
If implementation of biogeochemical observations needs to be geographically targeted, the areas of greatest interest for subsurface oxygen measurements are the eastern and central tropical Pacific, because of the key role that OMZs play there. For water mass properties and provenance, the low-latitude boundary currents and the western EUC are most important because of the role they play in delivering natural and anthropogenic inorganic carbon to the central and eastern Pacific. For air-sea CO2 fluxes and nutrient variability, it is important to map the entire tropical Pacific likely with a combination of ship transects, BGC-Argo and autonomous surface vehicles.
In summary, the observing system should:
• provide air-sea CO2 observations on buoys, including new sites in the western Pacific
• map basin-wide surface CO2 variability with ships or autonomous surface vehicles
• instrument at least one third of Argo float enhancements in the region with biogeochemical sensors
• re-establish CTD and bottle sampling for biogeochemical parameters on mooring servicing cruises
• incorporate biogeochemical observations on repeat hydrography voyages
• continue advocacy for biogeochemical satellite programs
• provide a backbone for biogeochemical process studies and ecosystem observations
The long-term vision is that through coupled physical and biogeochemical observations, process studies, and model development, TPOS can better serve the research community and ultimately inform marine resource management and protection.
The western equatorial Pacific is a principal conduit from the subtropics to the equator for thermocline and intermediate waters (Fine et al., 1994, see Figure 5). Low-Latitude Western Boundary Currents (LLWBCs) – the Mindanao Current (MC) in the northern hemisphere and the complex current system in the Solomon Sea in the southern hemisphere – transport waters of subtropical or higher-latitude origin toward the equator (Hu et al., 2015). These waters either exit the basin through the Indonesian seas via the Indonesian Throughflow (ITF), or feed the equatorial current system (Tsuchiya et al., 1989; Lukas et al., 1996; Fukumori et al., 2004; Grenier et al., 2011). At interannual ENSO timescales, LLWBC transport variations are comparable in magnitude to the interior transport variations: they are thus an essential part of the recharge/discharge of equatorial warm water volume (Lee and Fukumori, 2003; Izumo, 2005; Ishida et al., 2008; Qin et al., 2015) so measurements of their transport and properties are important for understanding, diagnosing and predicting ENSO events. LLWBC transports can also vary at decadal timescales, possibly contributing to decadal modulation of the background state of the equatorial band (Lee and Fukumori, 2003), although this decadal variability is largely unconstrained by observations. Finally, despite very different representation of these currents across climate models, major long-term changes are consistently predicted, including an acceleration in the southern hemisphere of the New Guinea Coastal Undercurrent and deceleration of both the MC and ITF (Hu et al., 2015; Sen Gupta et al., 2016). As such, the LLWBCs and the ITF represent major contributions to the mass, heat, and freshwater budget of the tropical Pacific (Roemmich et al., 2014; Cravatte et al., 2016).
Figure 5. Schematic showing the main circulation features for (A) the tropical Pacific, (B) the Indonesian Seas and (C) the tropical western Pacific. In (A) color shading shows mean salinity at thermocline level (20°C isotherm depth is used as a proxy) based on the BRAN2016 reanalysis (Oke et al., 2012); scale at right. Arrows highlight the flow pathways: Black at thermocline depth (thicker arrows are stronger), red for very shallow flows, dashed for postulated flows. Current names are listed in the legend at bottom. In (B,C) the brown shading shows the bathymetry (scale for both at right). Again arrows highlight the flow pathways, with purple arrows for undercurrents. Other observational arrays, including moorings, glider and XBT lines are added (legend).
The western Pacific is a primary source of macro and micro-nutrient (particularly iron) enrichments through land-sea exchanges including river discharges, hydrothermal sources, sediment inputs from the shelves and slopes, and through aerosol (notably volcanic) deposition (Mackey et al., 2002; Ryan et al., 2006; Slemons et al., 2009, 2010; Gorgues et al., 2010; Radic et al., 2011; Grenier et al., 2013; Labatut et al., 2014; Qin et al., 2016; Ganachaud et al., 2017). LLWBCs are thus also conveyor belts for micro-nutrients from the western continental margins to the central and eastern Equatorial Pacific upwelling regions, via the EUC; these remote nutrient supplies are critical for maintaining the high levels of biological productivity in this region (Mackey et al., 2002; Slemons et al., 2010, 2012; Grenier et al., 2013; Qin et al., 2016). Better understanding of the enrichment processes and transport variability is important to infer the changes in primary productivity in the eastern equatorial basin. This productivity affects the regional food web that underpins critical tropical fisheries (Bell et al., 2011) and also plays a role in the levels of pCO2 in surface waters and transferring carbon to the deep ocean.
LLWBCs are deep, narrow, powerful, and variable currents, flowing within a few 10 km from the coast, and are therefore challenging to observe. They require specialized instrumentation and integration across multiple platforms to adequately describe the connected fluctuations. Developing a LLWBC monitoring plan requires addressing several issues: identifying the users of a sustained monitoring system; assessing the relative importance of real-time and long-term sampling; determining the priority variables to be measured, their sampling accuracy and spatial and temporal timescales.
LLWBC and ITF heat transports are a first-order term in the evolution of equatorial warm water volume in all phases of ENSO. This suggests that simultaneous real-time monitoring of the combined LLWBC/ITF heat transport variability would enable a qualitative advance in ENSO and tropical Pacific climate diagnostics. The result, if implemented, would serve climate diagnostics and outlooks for expert assessments at climate and seasonal forecast centers. In addition, LLWBC observations could serve for constraining, validating and improving model simulations (Balmaseda et al., 2014) in this region where model resolution is often too coarse to resolve the narrow straits and complex bathymetry and be key to better understanding decadal/longer term modulation of ENSO.
For this purpose, monitoring the heat transport on monthly/seasonal timescales should be the target with vertical profiles of velocity and temperature observations. Ideally, monthly temporal resolution is needed for forecasting purposes and seasonal for change detection; given the high-frequency transport variability, this would imply a sampling at higher temporal resolution than monthly. We should strive to measure the entirety of the LLWBCs heat transport, coast to coast when it is constrained by bathymetry, or at locations appropriate to capture its horizontal extent. As much of the variability occurs in the upper 0–500 m this is the early priority, if possible extending deeper to capture the intermediate water transport; while monitoring the transport to the sill depth of the passages would be an ultimate goal from long-term climate perspectives.
In the following, we briefly discuss the main LLWBC features, learnt from the past or current process studies in the southern and northern hemispheres and in the ITF. We present a monitoring strategy with appropriate locations and platforms to respond to the above requirements.
In the southern hemisphere, the New Guinea Coastal Current (NGCC) system is composed of several branches that split within the Solomon Sea, a semi-enclosed sea acting as a bottleneck. The main branch flows as an undercurrent through the southern entrance within a narrow band closely tied to the coast, carrying mostly subtropical waters that flow up the coast of Australia in the Gulf of Papua Current (GPC; Kessler and Cravatte, 2013). The GPC/NGCC mixes with tropical waters that flow directly into the Solomon Sea. Inside the Solomon Sea, westward and eastward branches of the NGCC exit northward through Vitiaz Strait, St. George’s Channel and Solomon Strait (Figure 5).
Transport flowing through the Solomon Sea is highly variable at intraseasonal, seasonal and interannual timescales (see Ganachaud et al., 2014, 2017; Germineaud et al., 2016, for reviews). Large interannual transport variations, strongly modulated by ENSO, occur mainly in the top 250 m.
During the past decade since OO’09, international field campaigns in the Solomon Sea as part of the CLIVAR-SPICE program, have provided a testbed for sampling strategies. The main difficulty in observing the heat transport in this region is due to bathymetry splitting the LLWBCs into numerous branches, the narrowness of some passages (rendering the use of altimetry as a first-order proxy for mass transport inadequate), and the narrow current widths close to coastlines (and so not well sampled by broadscale Argo profiles). Integration across various observational networks in this region is essential. Full-depth subsurface moorings have been deployed in the straits for periods of 1.5–3 years (Ganachaud et al., 2017); repeated glider transects across the southern entrance of the Sea have proven to be an adequate solution for providing estimates of coast-to-coast equatorward heat and freshwater transport to 1000 m, though transects can be aliased by eddies (Davis et al., 2012); Pressure Inverted Echo Sounders (PIES) and high density XBT transects have been combined with altimetry and Argo floats to infer the mass transport interannual variability through the Solomon Sea (Melet et al., 2010; Zilberman et al., 2013). They are capable of quantifying many time scales of the volume transport of the NGCC system.
At present, the mass and heat and freshwater transports across the Solomon Sea southern entrance are sustainably monitored, with PIES/altimetry providing high resolution estimates of the mass transport, and repeated glider transects (better than monthly) providing in real-time vertical structure and property measurements. These should be maintained. Glider sampling must be frequent due to vigorous mesoscale eddies that result in high-frequency transport variability. Resolving monthly variability would require several transects per month, which seems as yet unrealistic.
Beyond monitoring transport and properties entering the Solomon Sea from the South Pacific, additional value would come from monitoring the exits to the equator, for two reasons. First, important mixing and water mass modification occurs inside the Solomon Sea (Melet et al., 2011; Alberty et al., 2017). Second, the partitioning between the three exit straits leads to three distinct pathways connecting the Solomon Sea to the equator, that models indicate have highly disparate transit times and ultimate destinations (Grenier et al., 2011; Qin et al., 2015). Resolving this partitioning appears to be important for the timescale of the impacts on the equatorial region, resulting in feedback processes, and biogeochemical enrichment.
Moorings are currently the only viable platforms for a sustained observing system in the narrow passages where the flow is fast and sufficiently confined by the bathymetry. However, while they provide excellent time series resolution, the mooring shortcomings (potential lack of cross-channel resolution of the current structure, poor coverage of the temperature-salinity (T-S) in the upper ∼100 m of the water column because of lack of a surface expression, heavy shipping traffic) might be complemented by HF radars to provide real-time surface current measurements. Gliders, providing near-real-time measurements, could be tested for measuring the transport through the wider Solomon Strait where the currents are not accelerated by confinement in narrow channels. It is worth noting that maintaining instrumentation in such remote areas will require dedicated personnel efforts and international coordination.
The North Pacific LLWBC system originates from the bifurcation of the North Equatorial Current (NEC) near the Philippine coast, resulting in a complex and time-varying distribution of its mass and properties among the Kuroshio, MC, Mindanao Eddy (ME), and North Equatorial Counter Current (NECC) (see Figure 5 for locations). High-salinity North Pacific Tropical Water is redistributed north and south, with the southward MC the primary source of the ITF (e.g., Gordon and Fine, 1996). Unlike the Solomon Sea, where the western boundary flow is primarily an undercurrent (see The Southern LLWBCs: The Solomon Sea), the MC is surface-intensified with the bulk of its transport above 250 m (Wijffels et al., 1995; Schonau and Rudnick, 2017; Figure 6).
Figure 6. Mean absolute geostrophic velocity on two transects across the LLWBCs of the Northern (top) and Southern (bottom) Hemispheres, from glider data. Red (positive) is equatorward in both panels, but the contour levels are different (scales in cm/s at right of each panel). The (top) panel is redrawn from Figure 4 of Schonau and Rudnick (2017). The green lines in the bottom panel separate regions with different sampling periods and were contoured separately. The western boundary currents are the red areas tight to the western edge, in each case about 100 km wide; note that the northern LLWBC (Mindanao Current) is surface-intensified with its maximum above 100 m, while the southern LLWBC has its core near 400 m, and little surface expression.
Opposite-direction undercurrents below both the Kuroshio and MC (e.g., Figure 6) converge to feed eastward subsurface jets (Qiu et al., 2013; Cravatte et al., 2017); the northward Mindanao Undercurrent also carries intermediate water from the South Pacific into the northern hemisphere (Qu and Lindstrom, 2004; Figure 5). Strong intraseasonal variability of these undercurrents has been identified from subsurface moorings (e.g., Wang et al., 2016). Recirculations offshore of the LLWBCs and the presence of both permanent and transient eddies complicate the understanding of current connectivity and blur the boundaries that define the LLWBC transport itself.
As is the case for the Solomon Sea (see The Southern LLWBCs: The Solomon Sea), the several possible destinations of the MC flow matter a great deal in diagnosing the downstream effects on the tropical Pacific as a whole. While one part of the MC flows directly into the ITF, an unknown amount enters the equatorial current system; since ITF transport varies strongly with ENSO (see Links to Indian Ocean: The Indonesian Seas), it is likely that this distribution changes with time. Determining the partitioning along these pathways and their variability is the key goal of observing this region.
The importance of the northern LLWBCs and their connection to the ITF has motivated several modern programs using diverse techniques to sample the region, effectively testing possible strategies for long-term monitoring. Investigators working under the Northwestern Pacific Ocean Circulation and Climate Experiment project have deployed moorings across the zonal currents east of the Philippines and spanning the bifurcation of the NEC, especially focusing on the undercurrents. Some of these sites include real-time data transmission. Gliders deployed under the Origins of the Kuroshio and Mindanao Currents program (Rudnick et al., 2015) produced 16 transects across the complete MC-ME-MUC system near 8°N during 2009 to 2013, measuring temperature, salinity and referenced geostrophic velocity. These allowed estimate of the mean and the seasonal and interannual variability of the system as a whole (Schonau and Rudnick, 2017; see Figure 5). Recently, investigators from the Korea Institute of Ocean Science and Technology (KIOST) and Inha University deployed a line of bottom Current and Pressure Recording Inverted Echo Sounders and subsurface Acoustic Doppler Current Profiler (ADCP) moorings across nearly the entire system at 8°N. While the data will not be available until the moorings are serviced, the array will provide highly resolved time series, invaluable in evaluating possibilities for sampling these currents. Although these individual research efforts have described important aspects of the variability, none have yet pointed to a sustainable monitoring strategy.
The complex flow and various pathways for the northern LLWBC, with no defined boundary to the east, make the design of a credible monitoring strategy for the MC more challenging than for either of the other two LLWBC regions. As in the case of the other LLWBC systems, the goal will be monthly resolution, and a multi-platform approach seems most appropriate. Such an approach might include a line of moorings to sample subsurface temperature and velocity, combined with Argo floats and glider transects to provide the real-time fields. A near-term goal should also investigate whether global observing programs (such as Argo combined with satellite altimetry) can produce useful estimates of the MC mass and property transport without dedicated in situ observations; the fact that the MC is shallow and surface-intensified makes this a realistic possibility. Two issues should be studied: First, how far offshore of the Philippine coast does Argo geostrophy give a reliable measure of the meridional currents? Second, can altimeter-derived surface velocity anomalies, combined with Argo temperature and salinity sampling, resolve the flow close to the coast? These can be tested with the present KIOST line of mooring data when those become available.
The Maritime Continent of Indonesia forms the leaky western boundary of the equatorial Pacific Ocean. The fresher water within the upper thermocline of the Indonesian seas is primarily derived from the North Pacific LLWBC entering via the Sulawesi Sea that then mostly flows into Makassar Strait. A smaller quantity of saltier lower thermocline and intermediate waters are derived from the South Pacific LLWBC (likely originating from Vitiaz Strait) and enter via the Maluku Channel and the Halmahera Sea. The North and South Pacific water masses mix within the various basins in the Indonesian seas to form the isohaline profile that is exported into the tropical Indian Ocean and beyond.
The mean ITF transport of ∼15 Sv from the Pacific and its associated heat transport to the Indian Ocean must be accounted for to close the equatorial Pacific heat budget. The transport varies considerably on interannual to multi-decadal timescales largely associated with ENSO and Indo-Pacific decadal variability (see Sprintall et al., 2019, this issue). The flow is maintained by a pressure gradient between the Western Pacific and Eastern Indian ocean that is modulated by wind and buoyancy changes over the tropical Indo-Pacific (Wyrtki, 1987), primarily tied to variations in the strength and location of the Walker circulation, whose center of convection is located over the Indonesian Seas. Recent observations have demonstrated a large multi-decadal intensification of the ITF and its heat transport in tandem with anomalously strong Pacific trade winds (see Sprintall et al., 2014, 2019 for a review). Understanding the changes in the vertical profile of velocity is critical for understanding changes in the biogeochemical properties that are linked to changes in the behavior and species distribution of the rich ecosystems within the Indonesian seas.
At present there are only a few long-term direct measurements of the ITF within the Indonesian seas (e.g., Gordon et al., 2012; see Sprintall et al., 2019, this issue). To better understand future ITF changes and their links to the western Pacific LLWBCs and tropical Pacific, we need to develop a sustained array that measures the ITF vertical profiles of not only velocity, but also temperature, salinity and other properties. The design of a suitable monitoring array for the ITF is challenging since we do not yet have a full understanding of the various transport streams and how partitioning through the various entry and exit channels change on interannual and longer time scales. Sustained measurement within the western Pacific entrance passages are also complicated by recirculation features in the energetic Halmahera and Mindanao Retroflections (Figure 5) that can influence the transport of water into the Indonesian seas. In addition, near surface measurements are hampered by damage from fishing and vandalism. For these reasons, an ITF monitoring strategy for the entrance ITF channels from the Pacific might best be achieved by focusing on mooring deployments in the relatively narrow constrictions that are not influenced by recirculations (e.g., the 680 m Labani Channel in southern Makassar Strait that capture 80% of the upper thermocline Pacific inflow and the deeper 1890 m Lifamatola Passage). As in other LLWBCs, at present the moorings do not report in real-time and only resolve full-depth velocity and so additional T-S measurements, such as from long-term XBT and glider transects or Argo profiles (e.g., Wijffels et al., 2008; Sprintall et al., 2009; Gruenberg and Gordon, 2018) are needed to develop proxy measurements of heat and freshwater fluxes. The wider eastern passages of the Seram Sea have never had adequate transport resolving coverage before, but water mass observations suggest that they provide an important source of South Pacific water. Although the flows may be swift, it could be that (multiple) glider transects might offer the best strategy to obtain a transport mass, heat and freshwater time series in these eastern pathways. ITF transport proxy measurements have also been successfully derived from altimetry, Argo profiles and reanalysis data. However, this typically only gives information about the total mass transport changes.
Final recommendations for designing a Pilot study able to develop an optimal sampling strategy for resolving the mass, heat, and freshwater transports of the LLWBC and ITF are given here.
- The ultimate target, as stated above, is to monitor LLWBCs heat transport to 500m at monthly/seasonal timescales. Ideally this should include simultaneous heat transport measurements in the northern and southern LLWBCs and the ITF.
- Further analyses are still needed to compare the existing different transport estimates in the northern and southern LLWBCs and in the ITF, and to determine the scales of intrinsic variability. In particular, comparisons of transport estimates at the entrance and exits of the Solomon Sea by different platforms should be planned.
- A single platform approach is not adequate, rather combining multiple and complementary platforms will be required for a sustained observing system of the LLWBCs. The ability of altimetry combined with Argo to produce useful estimates of the Mindanao mass and property transport should be investigated.
- International and regional cooperation, and dedicated personnel efforts will be necessary to sustain an observational network in these remote areas, and for coordinated data sharing.
- Monitoring the LLWBCs micronutrient content variations is currently restricted to careful ship-based measurements of iron, however, the timescales of release of iron from likely sources (river, hydrothermal, etc.) highlight the need for autonomous observing technology development. Further process studies are needed to better understand the locations and processes of the land to ocean inputs. This also requires understanding the effect of these properties on the downstream productivity and their interaction with other forcing terms. A first achievable step toward a sustained monitoring effort might be to monitor the micro-nutrients concentrations downstream in the EUC east of 156°E since this will ultimately impact the productivity of the eastern Pacific.
- In addition to these recommendations for sustained monitoring, a better understanding of the processes controlling the vigorous mixing occurring in the LLWBCs and ITF is needed. Mixing is known to be important for the transformation of water masses, but it is localized, highly variable, and difficult to estimate in these regions (Koch-Larrouy et al., 2015; Alberty et al., 2017; Bouruet-Aubertot et al., 2018; Sprintall et al., 2019); more targeted process studies are needed.
The Eastern Pacific (EPAC) region is arguably among the most problematic in the world for climate modeling, as oceanic processes, low-cloud physics, and convective rainfall processes have complex interactions that have eluded adequate representation for several decades, contributing to severe biases in even the best of the state-of-the-art climate models (Figure 7). Global coupled climate models suffer in particular from the so-called double ITCZ, which refers to the strong positive rainfall biases in the southeastern Pacific throughout the year (Bellucci et al., 2010), as well as a too diffuse thermocline that weakens the thermocline feedback, a main deterministic process for El Niño development. At the southern edge of the tropical EPAC is the stratus region, where coupled models and reanalysis consistently underestimate the frequency of low cloud, resulting in overestimation of the net surface heat flux in the region (e.g., Cronin et al., 2006; de Szoeke et al., 2012). Observing the EPAC from the ITCZ through the stratus region on sub-seasonal to interannual time scales is important for resolving such long-standing issues.
Figure 7. Mean SST bias (colors) of an ensemble of 39 coupled GCMs from the CMIP5 historical experiments over 1982–2005 relative to Reynolds SST (Reynolds et al., 2007). The contours indicate the standard deviation of the mean bias amongst the model ensemble (in °C).
Recent research also highlights the existence of two ENSO regimes, one associated with extreme El Niño events in the EPAC, and the other with moderate events centered in the Central Pacific (also called a Modoki or Warm Pool El Niño event). Such differences in the amplitude and spatial patterns of ENSO events, referred to as ENSO diversity (Capotondi et al., 2015), result from variations in the balance between the thermodynamic feedback across the equatorial Pacific and the activation of the non-linear Bjerknes feedback, when the far eastern Pacific warms above a temperature threshold corresponding to the convective threshold (Takahashi and Dewitte, 2016; Takahashi et al., 2018). Thus, monitoring the heat content in the far eastern Pacific is key for ENSO forecasting. While heat content can be approximated by the zonal average of equatorial thermocline anomalies, which the current TMA permits, it is also influenced by diabatic processes including diapycnal mixing, eddy-induced transport, and short-wave penetration (Lengaigne et al., 2012) that cannot be constrained by the observing system. In particular, the sloping thermocline induces a modal dispersion of equatorial Kelvin waves, which is associated with mixing processes that modulate heat content in the far eastern Pacific (Mosquera-Vásquez et al., 2014). Tropical instability waves also yield eddy-induced meridional heat transport that can influence the growth of ENSO (Holmes et al., 2018), while eddy-induced heat transport originating off the coast of Peru is important for the heat-budget at regional scales (Colas et al., 2012). Overall, these studies indicate that the perceived role the EPAC plays in ENSO has evolved since the era when the TMA was first implemented. The recommendations for the EPAC observing system must reflect this evolution in thinking.
Since the inception of the TMA, maintaining observations within the TMA along 95°W has been challenging due to the high exposure to vandalism and consequent loss of data. Data loss at these sites, as well as a lack of observations east of 95°W, limit our ability to pinpoint the causes of model deficiencies, despite the additional data from the Argo system and satellites (Xue et al., 2017). In particular, uncertainties in the atmospheric forcing from models in this region remain a concern for oceanic reanalysis, especially regarding their impact on regional air-sea coupled modes (Dewitte and Takahashi, 2017; Takahashi and Martínez, 2017) and their potential upscale effects on the tropical Pacific circulation (Toniazzo, 2010). Some of the main issues in atmospheric modeling of the EPAC are related to uncertainties in the surface heat fluxes and PBL parameterizations (Tapiador et al., 2018), as well as the vertical heating structure of the EPAC ITCZ. Satellite based measurements indicate that the maximum in convective heating is in the mid-upper troposphere (Schumacher et al., 2004), but reanalysis products indicate that the ascent is shallow (Back and Bretherton, 2006), consistent with the direct observations of a strong shallow overturning circulation in this region (Zhang et al., 2004). The vertical structure of the latent heating is of particular importance to ENSO, since it has a substantial influence on the surface wind response that is key for the Bjerknes feedback (Nigam et al., 2000; Nigam and Chung, 2000; Wu, 2003). In addition, the coupling between the ITCZ and SST provides non-linear feedbacks into the ENSO system (Dommenget et al., 2012; Lloyd et al., 2012; Takahashi and Dewitte, 2016).
The far EPAC also contains the most productive oceanic ecosystems in the world, and many livelihoods depend on a variety of marine resources that contribute significantly to local and international economies, as well as local cultures (Chavez et al., 2014). This relatively high biological productivity associated with the sluggish circulation in the subthermocline means the EPAC hosts the most extended OMZ in the world (Paulmier and Ruiz-Pino, 2009), important to climate feedbacks through the release of potent greenhouse gasses (see Biogeochemistry and Ecosystem Contributions). While open ocean deoxygenation during the second half of the 20th century (Breitburg et al., 2018) represents a threat to the marine ecosystems of the EPAC, the forcing mechanisms of the OMZ remain poorly known (Oschlies et al., 2017), mostly because oxygen data are very scarce and the transport of oxygenated waters by the equatorial undercurrent to the coast at subsurface is through fine meridional scale zonal jets (i.e., Tsuchiya jets) (Montes et al., 2014), poorly simulated by global models. The extension of the observing system to the far EPAC thus offers an opportunity to monitor key biological parameters (e.g., O2 and fluorescence) for improving our understanding of the OMZ dynamics.
Further progress in our understanding of the mechanisms governing climate variability and predictability in the EPAC and of its impacts, including ENSO, requires enhanced observing capabilities in this region, through a combination of existing technologies (i.e., Argo floats, satellite observations, autonomous vehicles) and appropriate network design (e.g., Cravatte et al., 2016). In particular, this new design will need to consider the coastal transition zone (coastal upwelling system), equatorial upper ocean non-linear processes, the vertical structure of the ITCZ and associated air-sea fluxes, the OMZ (Takahashi et al., 2014), and external ENSO forcing such as the South Pacific Meridional Mode (SPMM: Zhang et al., 2014) that can modulate the amplitude of ENSO (Larson et al., 2018).
To this end, the existing TMA line along 95°W should be maintained and expansions of the TMA into the northeast Pacific ITCZ and seasonal southeast Pacific ITCZ are necessary. Oceanic and biological conditions in the coastal regions of the EPAC are monitored by the countries along the west coast of South America for ecosystem management. However, there is currently no regional coordination in the framework of TPOS 2020, although the Permanent Commission for the South Pacific coordinated several annual cruises for El Niño monitoring and the GOOS Regional Alliance for the southeast Pacific (GRASP) provides a framework for data sharing. Such coordination would represent a significant advance for the observing system in the EPAC.
The Argo network is another key component of the observing system in this region, although this would require participation by all South American countries to meet the objective of doubling the density of coverage. Argo floats combined with satellite data (SST, altimetry) will address some of the knowledge gap by providing measurements of variability in upper ocean vertical structure. However, the Argo network does not allow monitoring of subsurface mesoscale ocean variability over a region due to its irregular sampling. Thus, regular hydrographic sections from ships or autonomous vehicles operated by national institutions in South America would represent an important contribution to the observing system. A section between the Galapagos islands and Ecuador or northern Peru would allow complementary measurements as well as monitor oxygen conditions and transport of water mass properties by the zonal jets to the Peru-Chile undercurrent, the conduit by which the OMZ is influenced by the equatorial variability (Montes et al., 2014). The monitoring of the coastal upwelling conditions (temperature, salinity, current and O2 profiles at historical sections) would also allow detection of intraseasonal Kelvin waves and validate the assimilation products from these independent observations. Such monitoring is now done by national institutions in South America; thus, its implementation within TPOS 2020 would require regional coordination for sharing data and standardized protocols for making measurements.
The requirement to characterize the atmospheric state within the ITCZ, atmospheric meridional circulation across the equator and surface forcing under the stratocumulus cloud deck on monthly timescales in the EPAC, needed to validate atmospheric structure in models across seasons and during ENSO, could be met through the instrumentation of new sites associated with well-located islands, including:
Clipperton (10°18′N 109°13′W, France) and Galapagos (Ecuador) Islands: Monitoring vertical profiles of atmospheric temperature, humidity and winds during the seasonal migration of the ITCZ to obtain the vertical atmospheric structure in the region on intra-seasonal to seasonal time scales. Over longer time periods, monitoring of vertical structure over the Galapagos Islands during El Niño events (see Huaman and Takahashi, 2016) would enable better understanding of the processes associated with the non-linear amplification of the Bjerknes feedback.
Hormigas island (11°58′S 77°46′W, Peru): This small island is located 30 miles from the coast in the blind zone of satellite scatterometers (Astudillo et al., 2017). This site would allow monitoring of changes in winds along the coast of Peru that are not seen by satellites. This, in turn, will allow better understanding of coastal upwelling dynamics through estimate of the near-shore decrease of the winds (i.e., wind curl) and air-sea interactions along the coast of Peru, thought to play a role on the evolution of EPAC El Niño (Dewitte and Takahashi, 2017) and the development of coastal El Niño events (Takahashi and Martínez, 2017).
St Felix Islands of the Desventuradas Archipelago (26°17′S 80°06′W, Chile), along with the existing OceanSITES Stratus mooring (20°S, 85°W), are ideally located for monitoring the stratocumulus cloud deck, important for moderating local SST and surface buoyancy forcing, as well as being a key region for understanding the interhemispheric surface energy balance important to the seasonal double ITCZ in the eastern Pacific (Kang et al., 2008, 2009; Li and Xie, 2014; Zhang et al., 2015). This site is also well located for monitoring the SPMM.
In summary, the EPAC design should include:
• The ability to capture intraseasonal to interannual variability in the coastal transition zone;
• Improved observation of equatorial upper ocean non-linear processes on intraseasonal time scales;
• Observations of atmospheric vertical structure within the ITCZ and associated air-sea fluxes;
• Observations of the OMZ and associated advection of oxygen by the surface and sub-surface current systems of the EPAC; and
• Capability to monitor modes of external ENSO forcing such as the South Pacific Meridional Mode.
While the TPOS backbone must rely upon proven technology, with systematic engineering and development and pilot studies, it is possible for new technologies to transition through multiple Technical Readiness Levels (TRL) (Lindstrom et al., 2012). With this in mind, we see a number of trends in technology and sampling techniques that may be ready to adopt into the TPOS backbone in the next decade.
One of the great legacies of OceanObs’99 was the initiation of the Argo float array (Argo Science Team, 1999). Ten years later, a legacy of OceanObs’09 was the consensus that these platforms should serve multidisciplinary functions. This has led to the development of a global BGC-Argo community (Claustre et al., 2010; Gruber et al., 2010)1 with miniaturization of numerous sensors previously used only within carefully controlled shipboard experiments. Among these biogeochemical enhancements, oxygen measurements have the longest history, but through several large research programs, most notably SOCCOM2, a standard suite of biogeochemical parameters is emerging. These are temperature, salinity, dissolved oxygen, pH, nitrate, chlorophyll fluorescence, particulate backscatter, and downwelling irradiance. More than 100 such floats have been deployed in the Southern Ocean but tropical Pacific deployments remain scarce.
Acoustic-based sensors of physical measurements also show great promise. Measuring wind and rain from passive aquatic listening device below the ocean surface could have the advantage of avoiding detection from the fishing fleet and thus having reduced data loss from vandalism. Likewise, depending upon the depth of the sensor, the footprint of the acoustic signal may be 100’s of meters in radius and therefore may be more comparable to a satellite footprint than a buoy point measurement.
New sensors are also being developed for satellite applications. As described by Villas Bôas et al. (2019), both NASA and ESA are developing potential sensors for satellite application that could measure wave height and surface currents from a single sensor. These are presently at a prototype TRL, with field tests from aircraft, but by 2030 may be operational. If so, this could play an important role, filling a gap in the surface current observing capabilities of the TPOS. Because currents at the equator are strongly ageostrophic, relying upon geostrophic methods for computing currents introduces large errors. Direct measurements of currents are needed for TPOS and the capability of doing so from space adds important spatial coverage and horizontal resolution capability.
An exciting new trend in technology is the growth of autonomous vehicles: underwater gliders, autonomous surface vehicles (e.g., Wave Gliders and Saildrone), and airborne drones. Unlike floats, drifters and radiosondes, autonomous vehicles can be navigated by a pilot. Surface EOV and ECVs that have been observed from autonomous surface vehicles include wind speed and direction, wind stress, air temperature and humidity, solar and longwave radiation, barometric pressure, air and sea pCO2, SST, SSS, Chl, O2, and upper ocean velocity. For many of these vehicles, the endurance is sufficiently long – several months or even approaching a year – it is more efficient to have the pilot shore-based, even if the vehicle is deployed and recovered from a ship. However, for many of the applications and for some vehicles like Saildrone, the autonomous surface vehicle can travel thousands of kilometers and thus can be deployed and recovered from a seaside port, rather than a ship. In general, autonomous vehicles rely upon aerodynamics for propulsion from wind, waves, or buoyancy. As the impacts of anthropogenic global warming begin to increase, these fossil-free solutions for observing the climate will become more and more important.
While autonomous vehicles can potentially hold station, much like moorings, an important advantage of these vehicles is that they can be navigated. Thus they can perform repeat sections and surveys, which can adapt to conditions at hand. For example, these vehicles may provide an alternative to TPOS moorings in high vandalism regions and could provide surface EOV and ECV along transects previously monitored by TMA moorings and the mooring tender research vessel. They also may focus on fronts between different regimes, such as the front that separates the equatorial “cold tongue” from the warm water to the north (and south) where ITCZs and convection form. Likewise, the vehicles may focus on the front of the eastern edge of the equatorial Pacific warm pool. During El Niño, this front shifts eastward so that warm water extends across much of the equatorial Pacific.
With the strong currents and weak winds and waves found in the tropics, the tropical environment is a surprisingly difficult environment for autonomous vehicles. To become effective components of the TPOS backbone, these new technologies must continue to be tested in the field and in the operational environment. Doing so may have enormous payoff for the TPOS in added capability, efficiency and resilience.
We envisage a staged implementation of the new Backbone with ongoing assessment through to full maturity. Many elements are connected through to global systems and will evolve accordingly, but with recognition of and advocacy from the TPOS community (users, sponsors, research). Others will require specific actions from the TPOS community and these are discussed in more detail below.
Figure 8 shows an assessment and a projection of the status of TPOS measured against requirements at the EOV level (see Figure 1). For SST, SSH and ocean color the situation and future prospects are strong. Increased assurance for passive microwave measurements of SST is desired, and the in situ networks need future improvement. The requirements for wind are being met adequately, but as noted in Section “Recommendations for an Integrated Backbone,” at least one more scatterometer is required to achieve the desired coverage. The First Report highlighted areas for improvement in the in situ vector wind record over the coming 5 years. SSS has benefited from experimental satellite missions during the last decade but these are expected to cease during the coming 3–5 years. The quality of SSS retrievals now fully justify a follow-on mission(s) and TPOS will argue for this in its 2nd Report. The situation for flux variables remains marginal with a strong reliance on high-quality in situ data; there are prospects for improving this situation as reflected in the figure. TPOS is advocating improvements for subsurface temperature and salinity with the prospect of both reaching the adequate level or better by 2025. Surface and subsurface ocean velocity requirements are considerably more difficult to satisfy. Remote measurements are being planned (see Trends in Technology and Sampling Techniques) and provide some potential for meeting needs at the surface at least. The First Report identifies actions to strengthen subsurface in situ sampling. Surface seawater pCO2 relies on sparsely distributed in situ networks (marginal) but BGC-Argo may be a game changer for subsurface biogeochemical processes. Ocean surface precipitation remains at the marginal to good range, principally due to the uncertain representativeness of buoy point measurements of rainfall.
Figure 8. Status of the observing system in the tropical Pacific with respect to the main variables and requirements as set out in Section 3.4 of Cravatte et al. (2016). The variables are SST, sea surface height (SSH), wind/ocean vector wind, SSS, ocean color, heat and freshwater flux, vertical temperature, salinity and velocity, pCO2 and precipitation. The assessment of status is broad and not at the detailed level. The four status levels are (a) red: requirement not met or only in part; (b) gold: requirement partially met (useful/threshold in the language of WMO Rolling Review of Requirements http://www.wmo.int/pages/prog/www.OSY/GOS-RRR.html); (c) lime: requirement met adequately (breakthrough); and (d) green: fully met or exceeded (ideal/goal).
A possible transition timing is shown in Figure 9 with most activities beginning in 2019–2020, and serious implementation and change spread over 2020–2023. Note that this schedule is only indicative since there are many variables in the implementation that are currently unresolved. In this schema, full implementation would not be achieved until 2024. The transition rate is resource dependent, but a steady and slower implementation allows assessment of the changes to occur, and particularly allows learnings on the use of new platforms and sensors.
Figure 9. Schematic providing detail of the schedule for change in the Backbone involving Argo, the TMA, and so-called super-sites (here denoted by TMA∗2). The symbol denotes a pilot mode. The symbol | | refers to review/evaluate points. For Argo the numbers show the annual seeding rate. For the TMA the numbers usually refer to enhancement levels. In anticipation of changes to the TMA (denoted by ΔTMA), we assume three phases Δ1, Δ2, and Δ3, following the priorities outlined in Section “The TPOS 2020 First Report.” The shading indicates the degree to which requirements will be met through this schedule (approximate) except for the supersites. “TMA west” currently consists of the TAO line at 165°E and 3 JAMSTEC sites. The former State Oceanic Administration have outlined plans to develop the so-called “ding” array (denoted by “
”) which extends the TMA to the northwest while at the same time supporting some of the key equatorial sites of the TMA (Chen et al., 2018).
For Argo, the deployment rates per year (over current numbers) will depend on the residence time in the tropics and the western and eastern Pacific in particular; the deployment strategy will have dual goals of maintaining the core Argo distribution while at the same time achieving the TPOS enhancement. We assume that around 30% of all enhancements will be BGC-Argo floats. The priority is in the order shown. Increased vertical sampling (Argo Δz) is already underway with Argo’s use of high bandwidth satellite communications in new floats.
The schedule for enhancing the western Pacific part of the TMA is initially dependent on Chinese plans for the so-called “Ding” array (“”), that will implement broader western Pacific coverage to enhance observations of the Warm Pool, Asian Monsoon, western boundary currents, and for typhoons (Chen et al., 2018). Here we assume a pilot will be deployed in 2019 (for cross-calibration and testing) and that the full array will follow over 3–4 years. We also assume JAMSTEC will continue to occupy three sites, including a super-site (see below). This ‘TMA west’ schedule will deliver partial restoration of the core equatorial TMA (4 out of 7) and enhancements in the northwest which will be discussed in the 2nd Report of the TPOS 2020 Project.
Several sensor and platform configurations required for the TPOS 2020 Backbone are currently being trialed (pilot experiments), including improved vertical sampling of currents (TMA Δz); subject to positive evaluation these will be implemented at the equatorial mooring sites. The proposed 1oN/S enhancements to the TMA at 140°W (see the First Report) also fall into this category, but long-term implementation has some dependency on the TMA reconfiguration (ΔTMA).
The “full flux at all moorings” recommendation (see Air-Sea Interface Surface Variables and Figure 3) involves a largely proven approach but we expect it will be prudent to phase this change in so that assessment and evaluation can take place. As noted in the First Report, this change does not require a significant injection of resources, but it is nevertheless of a scale that will require careful planning and coordination, including for data management needs. The roll-out should begin with the established TMA sites before new sites are instrumented.
The transformation of the TMA (assumed to be in three phases Δ1, Δ2, and Δ3) will undoubtedly pose the greatest challenge since it will be undertaken in an environment where resources are a strong consideration and full implementation requires coordination and cooperation between three or more agencies (see discussion in Section “The Rationale Behind the Reconfiguration of the Backbone”). The changes should be staged, and review and assessment should accompany each stage (denoted in the figure by ||). Sensitivity studies should be conducted to test the assumptions behind the design and to evaluate any anticipated impact. However, it should be noted that any such study will not be conclusive since the studies themselves are impacted by assumptions and parameterizations, and sensitivity experiments often have to be interpreted in the presence of significant systematic errors (see Fujii et al., 2019, for a fuller discussion).
The TPOS 2020 Project is currently considering options at the detailed level, such as the preferred longitudes and latitudes for extensions, and any priorities within the extensions themselves.
In addition to these Backbone changes, several so-called TMA supersites have been proposed (denoted TMA∗2). At these sites direct covariance flux measurements (DCFS) may be trialed, as well as new approaches such as hygrometers and infrared radiometers (for true satellite SST validation). They also provide opportunity to trial biogeochemistry and biological sensors.
To help work through the inevitable challenges these data streams will present and to assess their efficacy and refine their costings, pilots and improved technical coordination among those supporting TPOS are required. The pilots should be implemented as soon as possible, and indeed some of them have already began, as noted above. The outcomes from pilots should be assessed and shared regularly across the TPOS implementation teams and user groups. In addition, to ensure uniform data quality, sharing of technical and data processing practices across implementing teams is also required, and needs to be established where this does not exist.
A set of process studies have been proposed to aid the design and the evolution of TPOS (see the First Report, Chapter 6). Some of these studies have been initiated, some are close to completion, while others await investment. All are important for the phased implementation and improvement of TPOS. Section “Biogeochemistry and Ecosystem Contributions” refers to biogeochemical studies that are important for the design of that component of the observing system, some parts of which are underway. Section “Eastern Pacific” makes the case for an East Pacific ITCZ/warm pool/cold tongue/stratus system study. A Pacific upwelling and mixing physics process study aims to understand the complex of processes connecting the thermocline and the surface in the eastern equatorial upwelling region that is a principal control on SST and thus enables the large-scale air-sea feedbacks crucial to ENSO. The National Oceanic and Atmospheric Administration has funded the initial work on this study, including modeling. Two air-sea interaction studies (northern and eastern edge of the Warm Pool) have been the subject of initial exploratory work but are still to be initiated as a coherent and coordinated piece of work.
Assessments are a crucial activity as pilots transition to full implementation and changes are undertaken, to help quickly diagnose issues and feed them back to the implementing teams. It is unlikely we will have the luxury of mandating overlapping periods, but to the extent possible change will be in accordance with GCOS Monitoring Principles (Global Climate Observing System [GCOS], 2010). Fujii et al. (2019) provide several examples of how such work might be done, but it will also be important that future governance arrangements take this need into account, including the need for ongoing oversight (see the following section).
The TPOS 2020 Project effectively takes the design and support of the TPOS “offline” from global governance (that is, not directly coordinated by) to undertake reevaluation of the requirements and the design, and to begin the process of change to achieve a more effective and efficient system. The TPOS 2020 Steering Committee is responsible for scientific and technical aspects, while the Resource Forum provides representation for stakeholders (user, providers, beneficiaries); see Figure 10. TPOS 2020 has a fixed-term and deploys standard project management techniques to support its activities.
Figure 10. (A) TPOS 2020 Project governance arrangements. The TPOS 2020 Steering Committee has six Task Teams (as shown) and shares a seventh (the Transition and Implementation Task Team) with the JCOMM Management Group. The Resource Forum is a key element of the structure. Further details are available from tpos2020.org. (B) Possible post-2020 TPOS arrangements. It is likely a science/steering committee will continue and that at least some of the Task Teams will have renewed mandates. A regional TPOS Forum is proposed to facilitate coordination and cooperation between partners, and with users/beneficiaries.
At the creation of the Project, there was no dedicated governance arrangement for the TPOS; some elements fell within the responsibilities of the Joint WMO/Intergovernmental Oceanographic Commission Technical Commission for Oceanography and Marine Meteorology (JCOMM) and its Observation Program Area (e.g., drifters, ships of opportunity) while others, such as Argo, enjoyed independent governance arrangements but otherwise were fully coordinated with JCOMM. The Tropical Mooring Implementation Panel (TIP3), which provided the original oversight and scientific and technical leadership for TAO/TRITON now falls within the Data Buoy Cooperation Panel of JCOMM and in principle retains the intergovernmental lead for the global TMA. The TIP were among the first to draw attention to the deterioration of the TMA in 2012/2013, however, as the events unfolded during that period it was clear TPOS lacked coordination among the network providers and active oversight and management of risk was lacking (Global Climate Observing System [GCOS], 2014a,b).
At the most recent meeting of the Resource Forum4, each of the 14 participating organizations expressed strong support for the TPOS 2020 plans and recognized the need for strong and effective future coordination. The Forum supported the TPOS 2020 First Report and its specific short- and near-term actions, noting that they will benefit the strategic goals for TPOS, the global ocean observing community and decision makers who rely on knowledge of the tropical Pacific for livelihoods, public safety, and commerce. The Forum supported the establishment of a task team to guide implementation of the recommendations and actions.
Given the importance of the Tropical Pacific for the provision of seasonal-interannual predictions, and the importance of strengthened engagement with Meteorological Services in the future of the observing system, it was important to engage JCOMM, and WMO through the WMO Integrated Global Observing System (WIGOS) in discussions on future governance. The implementation task team, now known as the TPOS/JCOMM Transition and Implementation Task Team (T&I TT) (Figure 10A), is an early manifestation of the type of coordination and cooperation that will be needed for maintenance of the TPOS in the future. WMO WIGOS recognizes the activities of the T&I TT as a regional Pilot and the joint sponsorship by JCOMM is an acknowledgment by JCOMM that such coordination might be needed in the post-TPOS 2020 era (see the decision in World Meteorological Organization [WMO], 2017). Discussion of post-2020 governance arrangements have commenced and must consider scientific and technical oversight and leadership (the legacy of the Steering Committee) as well as regional coordination, as discussed above. Strengthened regional coordination approaches are also being considered in other regions (e.g., the Atlantic Blueprint process, the Southern Ocean Observing System); and a number of approaches will probably be tested, to address particular scientific and geopolitical contexts.
A central theme in the governance discussions in the Tropical Pacific (and other regions) is that active and mutually supportive partnerships among TPOS stakeholders are vital for its robustness and sustainability. The TMA was put at risk in 2012–2013 because such partnerships were not in place or effective. This paper proposes that it be in the form of a TPOS Forum where partners (providers of observing capability) and users/beneficiaries can assist JCOMM in coordination and evolution of the TPOS (Figure 10B). It is further proposed that the TPOS 2020 (project) Steering Committee be replaced by a TPOS Scientific Advisory Committee, advising the TPOS Forum and other sponsors (e.g., GOOS and/or the World Climate Research Program) of the evolving scientific requirements (for example EOV and observing system requirements) and assessing potential technical solutions (options among the mix of platforms).
As Section “Phased Changes to the Backbone” notes, there is also a role for a TPOS Scientific Advisory Committee in the review and assessment of implementation post 2020, and in providing adjustments to strategy and/or the design as a consequence of these reviews, as appropriate.
While it is best to assess the need for scientific and technical task teams post-2020, our expectation based on the current level and expected duration of scientific activities is that some of the existing teams may continue (but perhaps with revised mandates) while others may be created. Further discussion with the scientific community will be needed to finalize the best and most efficient arrangement.
TPOS 2020 has undertaken pioneering work on observing design, including elaboration of the Framework for Ocean Observing process. The TPOS 2020 process delineates the different steps of requirement assessment, from users through to the observing system itself. This process together with the goals agreed for the TPOS 2020 Backbone provide the framing for the TPOS 2020 First Report and for priority setting. The significant sustained (operational) use for climate prediction is one of the primary factors.
The TPOS 2020 Report builds on the strengths of the observing system, including many new capabilities that have become available over recent decades. We present a combination of enhancements and changes that play to the strengths of different observing techniques, both remote and in situ, to provide robustness, reinforcement and cross-checking, and greater overall resilience.
A new Backbone is outlined that enhances our capability to track long-term change, but also delivers unprecedented in situ observations of surface fluxes and velocity, equatorial currents and subsurface hydrography, along with robust and better validated satellite data streams. Further enhancements to capture the diurnal cycle of ocean and atmosphere coupling are also being considered.
Biogeochemistry, low-latitude western boundary currents and the Eastern Pacific are highlighted as three areas for future evolution and change in TPOS. The major biogeochemical requirements relate to the climate record of air-sea CO2 flux, and to seasonal to interannual water column variability of inorganic carbon, oxygen, chlorophyll, particles, and nutrients. BGC-Argo observations have the potential to respond to many of these requirements.
We present a roadmap for a future integrated observing system for the LLWBCs and ITF, in the form of design elements for a Pilot study that will provide guidance on the optimal sampling strategy for resolving the mass, heat, and freshwater transports. The ultimate target is to routinely monitor LLWBCs heat transport to 500 m at monthly-to-seasonal timescales, ideally including simultaneous heat transport measurements in the northern and southern extensions of LLWBCs and the ITF. Such an approach will require international cooperation and collaboration and involve multiple and complementary platforms, such as Argo and altimetry.
It is now clear that further progress in our understanding of the mechanisms governing climate variability and predictability in the eastern Pacific, including ENSO, requires enhanced observing capabilities, through a combination of existing technologies (i.e., Argo floats, satellite observations, autonomous vehicles) and appropriate network design. This design will need to consider the coastal transition zone (coastal upwelling system), the vertical structure of the ITCZ and associated air-sea fluxes, and the OMZ. Regular sections between the Galapagos Islands and Ecuador or northern Peru would be a valuable contribution, but enhanced cooperation and data sharing will be needed. The need to characterize the atmospheric vertical structure in the ITCZ and in the stratocumulus cloud deck could be met through the instrumentation of new ocean sites on well-located islands.
We examined the status of important elements of TPOS and identified good prospects for altimetry, SST, ocean color and wind. SSS and rainfall require development and we support experimental missions to measure ocean surface currents from space. An initial timetable for implementation was provided, but subject to further discussion with the sponsors of the observing system. We contend that change in the observing system is a healthy and necessary part of the design and for maintaining engagement and investment.
This paper does not address aspects such as modeling and data assimilation, air-sea fluxes, data management or subseasonal variability. These topics are discussed in detail elsewhere in this issue. Coupled data assimilation and prediction looms as one the major developments over the next decade; already coupled numerical weather prediction and reanalysis systems are reaching into the traditional domains of oceanography. The input requirements on these shorter time scales are unknown but the First Report anticipated greater demands for upper ocean and atmosphere/ocean boundary layer data. The priority attached to the climate record has been one of the more controversial aspects of the TPOS 2020, with views ranging from fully embracing an integrated and multi-faceted and changing set of inputs to the climate record, to views that see existing data records as sacrosanct and not to be changed under any circumstances. This paper argues for a middle ground where climate records are valued, but change is also embraced.
Persistent systematic errors and difference in some data-based products and in models and reanalyses remain a point of significant concern. The power of the observing system is reduced by such factors, often in a significant and compromising way. Such issues will be a focus for the 2nd Report. Data sharing and adherence to the FAIR Principles (see Tanhua et al., 2019, this issue) are also major concerns, with many data having restricted usage.
Modeling and data assimilation systems are also an important tool for understanding the effectiveness (impact) of observations and for testing and evaluating changes to the observing system. As noted in Cravatte et al. (2016) (and reviewed in Fujii et al., 2019) observing system sensitivity and simulation experiments can provide guidance on the relative impact of (for example) moorings, floats and satellites on ocean state estimates (analyses) and forecasts. Chapter 6 of the First Report discussed some possible avenues for experimentation, however, we note such guidance is sensitive to the details of the modeling/assimilation systems and can be compromised by systematic errors.
The governance of the TPOS 2020 Project has broken new ground. The sponsors agreed to review and redesign the observing system through a project of their own creation and under their own support. The governance follows common Project Management arrangements but is somewhat unusual in the intergovernmental world, for example for GOOS, JCOMM and the World Climate Research Program. It is, however, a modus operandi that is often used to support space missions. The experience from TPOS 2020 suggests such approaches should be more widely used for ocean observations.
NS, WK, SC, JS, SW, MC, AS, and YS contributed text, review and revisions to all sections of the paper. BD and YS led the Eastern Pacific discussion and contributed review. KT provided input to and review of the Eastern Pacific section. AS led the biogeochemistry contribution with input from PS. KH contributed to the opening sections and the section on governance. AS and XL contributed to the low-latitude western boundary current section. DC contributed to Sections “The TPOS 2020 First Report” and “Phased Changes to the Backbone.” SB contributed comments, review and editing.
BD thanks LEFE-GMMC for financial support. JS participation in this study was supported by NOAA’s Global Ocean Monitoring and Observing Program through Award NA15OAR4320071. NOAA’s Ocean Observing and Monitoring Division has supported NS and WK and the TPOS 2020 Distributed Project Office.
The authors declare that the research was conducted in the absence of any commercial or financial relationships that could be construed as a potential conflict of interest.
We wish to acknowledge contributions from M. Alberty, W. Anutalya, R. Davis, G. Eldin, C. Germineaud, A. Gordon, L. Gourdeau, C. Jeandel, F. Lacan, A. Melet, and U. Send. We also acknowledge the authors of the First Report and the members of the TPOS 2020 Steering Committee and its Task Teams, and the TPOS Resource Forum members, who have supported the TPOS 2020 Project. Lucia Upchurch (PMEL) provided valuable editing support, especially for the figures. Hristina Hristova of PMEL assisted with Figure 2. This is PMEL contribution # 4869. The members of the EP Task Team are acknowledged for discussions.
Alberty, M. S., Sprintall, J., MacKinnon, J., Ganachaud, A., Cravatte, S., Eldin, G., et al. (2017). Spatial patterns of mixing in the Solomon Sea. J. Geophys. Res. 122:5. doi: 10.1002/2016JC012666
Ando, K., Kuroda, Y., Fujii, Y., Fukuda, T., Hasegawa, T., Horii, T., et al. (2017). Fifteen years progress of the TRITON array in the Western Pacific and Eastern Indian Oceans. J. Oceanogr. 73, 403–426. doi: 10.1007/s10872-017-0414-4
Argo Science Team (1999). “Argo: the global array of profiling floats,” in Proceedings of OceanObs99 International Conference on the Ocean Observing System for Climate, St. Raphael, France.
Astudillo, O., Dewitte, B., Mallet, M., Frappart, F., Ruttlant, J., Ramos, M., et al. (2017). Surface winds off Peru-Chile: observing closer to the coast from radar altimetry. J. Remote Sens. Environ. 191, 179–196. doi: 10.1016/j.rse.2017.01.010
Back, L. E., and Bretherton, C. S. (2006). Geographic variability in the export of moist static energy and vertical motion profiles in the tropical Pacific. Geophys. Res. Lett. 33:L17810. doi: 10.1029/2006GL026672
Balmaseda, M., Kumar, A., Andersson, E., Takaya, Y., Anderson, D., Janssen, P., et al. (2014). Operational Forecasting Systems. Report of the Tropical Pacific Observing System 2020 Workshop (TPOS 2020), Vol II. San Diego, CA: Scripps Institution of Oceanography.
Bell, J. D., Johnson, J. E., and Hobday, A. J. (eds.) (2011). Vulnerability of(Fisheries)and Aquaculture in the Tropical Pacific to Climate Change. Nouméa: Secretariat of the Pacific Community (SPC) FAME Digital Library.
Bellucci, A., Gualdi, S., and Navarra, A. (2010). The double-ITCZ syndrome in coupled general circulation models: the role of large-scale vertical circulation regimes. J. Clim. 23, 1127–1145. doi: 10.1175/2009JCLI3002.1
Bouruet-Aubertot, P., Cuypers, Y., Ferron, B., Dausse, D., Menage, O., Atmadipoera, A., et al. (2018). Contrasted turbulence intensities in the Indonesian Throughflow: a challenge for parameterizing energy dissipation rate. Ocean Dynam. 68, 779–800. doi: 10.1007/s10236-018-1159-3
Boutin, J., Vergely, J. L., Marchand, S., D’Amico, F., Hasson, A., Kolodziejczyk, N., et al. (2018). New SMOS sea surface salinity with reduced systematic errors and improved variability. Remote Sens. Environ. 214, 115–134. doi: 10.1016/j.rse.2018.05.022
Breitburg, D., Levin, L. A., Oschlies, A., Grégoire, M., Chavez, F. P., Conley, D. J., et al. (2018). Declining oxygen in the global ocean and coastal waters. Science 359:6371. doi: 10.1126/science.aam7240
Capotondi A., Wittenberg, A., Newman, M., Di Lorenzo, E., Yu, J. -Y., Braconnot, P., et al. (2015). Understanding ENSO diversity. Bull. Amer. Met. Soc. 96, 921–936. doi: 10.1175/BAMS-D-13-00117.1
Cashin, P., Mohaddes, K., and Raissi, M. (2014). Fair Weather or Foul? The Macroeconomic Effects of El Niño. Cambridge Working Papers in Economics #1418. Available at: http://www.econ.cam.ac.uk/research/repec/cam/pdf/cwpe1418.pdf.
Centre for International Economics (2014). Analysis of the Benefits of Improved Seasonal Climate Forecasting for Agriculture, Prepared for the Managing Climate Variability Program, March 2014. Available at: http://www.managingclimate.gov.au/publications/benefits-of-improved-forecasts/.
Chavez, F. P., Hobday, A. J., Strutton, P., Gierach, M., Radenac, M. H., Chai, F., et al. (2014). White Paper #7— A Tropical Pacific Observing System (TPOS) in Relation to Biological Productivity and Living Resources. Report of the Tropical Pacific Observing System 2020 Workshop (TPOS 2020), GCOS-184/GOOS-206/WCRP-6/2014, Vol. 2. San Diego, CA: Scripps Institution of Oceanography.
Chavez, F. P., Ryan, J., Lluch-Cota, S. E., and Ñiquen, M. C. (2003). From anchovies to sardines and back: multidecadal change in the Pacific Ocean. Science 299, 217–221. doi: 10.1126/science.1075880
Chavez, F. P., Strutton, P. G., Friederich, G. E., Feely, R. A., Feldman, G. C., Foley, D. G., et al. (1999). Biological and chemical response of the equatorial Pacific Ocean to the 1997-98 El Nino. Science 286, 2126–2131. doi: 10.1126/science.286.5447.2126
Chen, D., Smith, N., and Kessler, W. (2018). The evolving ENSO observing system. Natl. Sci. Rev. 5, 805–807. doi: 10.1093/nsr/nwy137
Chiodi, A. M., and Harrison, D. E. (2017a). Observed El Niño SSTA development and the effects of easterly and westerly wind events in 2014/15. J. Clim. 30, 1505–1519. doi: 10.1175/JCLI-D-16-0385.1
Chiodi, A. M., and Harrison, D. E. (2017b). Simulating ENSO SSTAs from TAO/TRITON winds: the impacts of 20 years of buoy observations in the Pacific waveguide and comparison with reanalysis products. J. Clim. 30, 1041–1059. doi: 10.1175/JCLI-D-15-0865.1
Claustre, H., Bishop, J., Boss, E., Bernard, S., Berthon, J., Coatanoan, C., et al. (2010). “Bio-optical profiling floats as new observational tools for biogeochemical and ecosystem studies: Potential synergies with ocean color remote sensing,” in Proceedings of OceanObs’09: Sustained Ocean Observations and Information for Society, Venice, Italy, 21-25 September 2009, Vol. 1, eds J. Hall, D. E. Harrison, D. Stammer (Auckland: ESA Publication). doi: 10.5270/OceanObs09.cwp.17
Colas, F., McWilliams, J. C., Capet, X., and Kurian, J. (2012). Heat balance and eddies in the Peru-Chile current system. Clim. Dyn. 39, 509–529. doi: 10.1007/s00382-011-1170-6
Cravatte, S., Kessler, W. S., Smith, N., and Wijffels, S. E. (2016). First Report of TPOS 2020. GOOS-215, 200. Available at: http://tpos2020.org/first-report/
Cravatte, S., Kestenare, E., Marin, F., Dutrieux, P., and Firing, E. (2017). Subthermocline and intermediate zonal currents in the tropical Pacific Ocean: paths and vertical structure. J. Phys. Oceanogr. 47, 2305–2324. doi: 10.1175/JPO-D-17-0043.1
Cronin, M. F., Bond, N. C., Fairall, C. W., and Weller, R. A. (2006). Surface cloud forcing in the east pacific stratus deck/cold tongue/ITCZ complex. J. Clim. 3, 392–409. doi: 10.1175/JCLI3620.1
Davis, R. E., Kessler, W. S., and Sherman, J. T. (2012). Gliders measure western boundary current transport from the South Pacific to the Equator. J. Phys. Oceanogr. 42, 2001–2013. doi: 10.1175/JPO-D-12-022.1
de Szoeke, S. P., Yuter, S., MechemFairall, D., Burleyson, C. D., and Zuidema, P. (2012). Observations of stratocumulus clouds and their effect on the eastern pacific surface heat budget along 20°S. J. Clim. 25, 8542–8567. doi: 10.1175/JCLI-D-11-00618.1
Dewitte, B., and Takahashi, K. (2017). Diversity of moderate El Niño events evolution: role of air-sea interactions in the eastern tropical Pacific. Clim. Dyn. doi: 10.1007/s00382-017-4051-9
Dlugokencky, E. J., Lang, P. M., Mund, J. W., Crotwell, A. M., Crotwell, M. J., and Thoning, K. W. (2018). Atmospheric Carbon Dioxide Dry Air Mole Fractions from the NOAA ESRL Carbon Cycle Cooperative Global Air Sampling Network, 1968-2017, Version: 2018-07-31. Available at: Path: ftp://aftp.cmdl.noaa.gov/data/trace_gases/co2/flask/surface/
Dommenget, D., Bayr, T., and Frauen, C. (2012). Analysis of the non-linearity in the pattern and time evolution of El Niño southern oscillation. Clim Dyn. 40, 2825–2847. doi: 10.1007/s00382-012-1475-0
Feely, R. A., Takahashi, T., Wanninkhof, R., McPhaden, M. J., Cosca, C. E., Sutherland, S. C., et al. (2006). Decadal variability of the air-sea CO2 fluxes in the equatorial Pacific Ocean. J. Geophys. Res. 111:C08S90. doi: 10.1029/2005JC003129
Fine, R. A., Lukas, R., Bingham, F. M., Warner, M. J., and Gammon, R. H. (1994). The western equatorial Pacific: a water mass crossroads. J. Geophys. Res. Oceans 12, 25063–25080. doi: 10.1029/94JC02277
Fischer, A. S., Hall, J., Harrison, D. E., Stammer, D., and Benveniste, J. (2010). “Conference summary-Ocean information for society: sustaining the benefits, realizing the potential,” in Proceedings of OceanObs’09: Sustained Ocean Observations and Information for Society, Venice, Italy, September 21–25, 2009, Vol. 1, eds J. Hall, D. E. Harrison, and D. Stammer (Auckland: ESA Publication WPP-306).
Fujii, Y., Cummings, J., Xue, Y., Schiller, A., Lee, T., Balmaseda, M. A., et al. (2015). Evaluation of the tropical pacific observing system from the ocean data assimilation perspective. Q. J. R. Meteorol. Soc. 141, 2481–2496. doi: 10.1002/qj.2579
Fujii, Y., Rémy, E., Zuo, H., Oke, P., Halliwell, G., Gasparin, F., et al. (2019). Observing system evaluation based on ocean data assimilation and prediction systems: on-going challenges and future vision for designing/supporting ocean observational networks. Submitted to Frontiers in Marine Science, this issue.
Fukumori, I., Lee, T., Cheng, B., and Menemenlis, D. (2004). The origin, pathway, and destination of Nino-3 water estimated by a simulated passive tracer and its adjoint. J. Phys. Oceanor. 34, 582–604. doi: 10.1175/2515.1
Ganachaud, A., Cravatte, S., Melet, A., Schiller, A., Holbrook, N. J., Sloyan, B. M., et al. (2014). The southwest pacific ocean circulation and climate experiment (SPICE). J. Geophys. Res. Oceans 11, 7660–7686. doi: 10.1002/2013JC009678
Ganachaud, A., Cravatte, S., Sprintall, J., Germineaud, C., Alberty, M., Jeandel, C., et al. (2017). The Solomon Sea: its circulation, chemistry, geochemistry and biology explored during two oceanographic cruises. Elem. Sci. Anth 5:33. doi: 10.1525/elementa.221
Germineaud, C., Ganachaud, A., Sprintall, J., Cravatte, S., Eldin, G., Alberty, M. S., et al. (2016). Pathways and water mass properties of the thermocline and intermediate waters in the Solomon Sea. J. Phys. Oceanogr. 46, 3031–3049. doi: 10.1175/JPO-D-16-0107.1
Global Climate Observing System [GCOS] (2010). Implementation Plan for the Global Observing System for Climate in Support of the UNFCCC (2010 Update). GCOS–138 (GOOS–184, GTOS–76, WMO–TD/No. 1523). Geneva: World Meteorological Organization.
Global Climate Observing System [GCOS] (2014a). Report of the Tropical Pacific Observation System 2020 (TPOS 2020) Workshop, Vol. 1. La Jolla, CA: GCOS-184, 66.
Global Climate Observing System [GCOS] (2014b). Report of the Tropical Pacific Observation System 2020 (TPOS 2020) Workshop, Vol. II. La Jolla, CA: GCOS-184, 340.
Global Climate Observing System [GCOS] (2016). The Global Observing System for Climate: Implementation Needs, GCOS-200. Geneva: World Meteorological Organization, 325.
Gordon, A. L., and Fine, R. A. (1996). Pathways of water between the Pacific and Indian oceans in the Indonesian seas. Nature 379, 146–149. doi: 10.1038/379146a0
Gordon, A. L., Huber, B. A., Metzger, E. J., Susanto, R. D., Hurlburt, H. E., and Adi, T. R. (2012). South China sea throughflow impact on the indonesian throughflow. Geophys. Res. Lett. 39:L11602. doi: 10.1029/2012GL052021
Gorgues, T., Menkes, C., Slemons, L., Aumont, O., Dandonneau, Y., Radenac, M. H., et al. (2010). Revisiting the La Niña 1998 phytoplankton blooms in the equatorial Pacific. Deep Sea Res. I 57, 567–576. doi: 10.1016/j.dsr.2009.12.008
Gregg, W. W., and Casey, N. W. (2007). Modeling coccolithophores in the global oceans. Deep Sea Res. II 54, 447–477. doi: 10.1016/j.dsr2.2006.12.007
Grenier, M., Cravatte, S., Blanke, B., Menkes, C., Koch-Larrouy, A., Durand, F., et al. (2011). From the western boundary currents to the Pacific Equatorial undercurrent: modeled pathways and water mass evolutions. J. Geophys. Res. 116:C12. doi: 10.1029/2011JC007477
Grenier, M., Jeandel, C., Lacan, F., Vance, D., Venchiarutti, C., Cros, A., et al. (2013). From the subtropics to the central equatorial Pacific Ocean: neodymium isotopic composition and rare earth element concentration variations. J. Geophys. Res. Oceans. 118, 592–618. doi: 10.1029/2012JC008239
Gruber, N., Doney, S., Emerson, S., Gilbert, D., Kobayashi, T., Körtzinger, A., et al. (2010). “Adding oxygen to Argo: Developing a global in situ observatory for ocean deoxygenation and biogeochemistry,” in Proceedings of OceanObs’09: Sustained Ocean Observations and Information for Society, Venice, Italy, 21-25 September 2009, Vol. 2, eds J. Hall, D. E. Harrison, D. Stammer (Auckland: ESA Publication WPP-306), doi: 10.5270/OceanObs09.cwp.39
Gruenberg, L., and Gordon, A. L. (2018). Variability in Makassar Strait heat flux and its effect on the eastern tropical Indian Ocean. Oceanography 31, 80–87. doi: 10.5670/oceanog.2018.220
Harrison, D. E., Bond, N., Goddard, L., Martinez, R., and Yamagata, T. (2014). Some Societal Impacts of ENSO, White Paper #2, Report of the Tropical Pacific Observing System 2020 Workshop (TPOS 2020), Vol. II. San Diego, CA: Scripps Institution of Oceanography, 4–26.
Hasson, A., Puy, M., Boutin, J., Guilyardi, E., and Morrow, R. (2018). Northward propagation across the tropical north Pacific Ocean revealed by surface salinity: how do El Niño anomalies reach Hawaii? J. Geophys. Res. 123:4. doi: 10.1002/2017JC013423
Hoeke, R. K., McInnes, K. L., Kruger, J. C., McNaught, R. J., Hunter, J. R., and Smithers, S. G. (2013). Widespread inundation of Pacific islands triggered by distant-source wind-waves. Global Planet. Change 108, 1–11. doi: 10.1016/j.gloplacha.2013.06.006
Holmes R. M., McGregor, S., Santoso, A., and England, M. H. (2018). Contribution of tropical instability waves to ENSO irregularity. Clim. Dyn. doi: 10.1007/s00382-018-4217-0
Hu, D., Wu, L., Cai, W., Sen Gupta, A., Ganachaud, A., Qiu, B., et al. (2015). Pacific western boundary currents and their roles in climate. Nature 522, 299–308. doi: 10.1038/nature14504
Huaman, L., and Takahashi, K. (2016). The vertical structure of the eastern Pacific ITCZs and associated circulation using the TRMM Precipitation Radar and in situ data. Geophys. Res. Lett. 43, 8230–8239. doi: 10.1002/2016GL068835
Intergovernmental Panel on Climate Change (2014). “Summary for policymakers,” in Climate Change 2014: Impacts, Adaptation, and Vulnerability. Part A: Global and Sectoral Aspects. Contribution of Working Group II to the Fifth Assessment Report of the Intergovernmental Panel on Climate Change, eds C. B. Field, et al. (Cambridge: Cambridge University Press), 1–32.
Ishida, A., Kashino, Y., Hosoda, S., and Ando, K. (2008). North-south asymmetry of warm water volume transport related with El Niño variability. Geophys. Res. Lett. 35:L18612. doi: 10.1029/2008GL034858
Izumo, T. (2005). The equatorial undercurrent, meridional overturning circulation, and their roles in mass and heat exchanges during El Nino events in the tropical Pacific Ocean. Ocean Dyn. 55, 110–123. doi: 10.1007/S10236-005-0115-1
Kang, S. M., Frierson, D. M. M., and Held, I. M. (2009). The tropical response of extratropical thermal forcing in an idealized GCM: the importance of radiative feedbacks and convective parameterization. J. Atmos. Sci. 66, 2812–2827. doi: 10.1175/2009JAS2924.1
Kang, S. M., Held, I. M., Frierson, D. M. M., and Zhao, M. (2008). The response of the ITCZ to extratropical thermal forcing: idealized slab-ocean experiment with a GCM. J. Clim. 21, 3521–3532. doi: 10.1175/2007JCLI2146.1
Kessler, W. S. and Cravatte, S. (2013). Mean circulation of the Coral Sea. J. Geophys. Res. Oceans. 118, 6385-6410 doi: 10.1002/2013JC009117
Koch-Larrouy, A., Atmadipoera, A., Van Beek, P., Madec, G., Aucan, J., Lyard, F., et al. (2015). Estimates of tidal mixing in the Indonesian archipelago from multidisciplinary INDOMIX in situ data, Deep Sea Res. Part I 106, 136–153. doi: 10.1016/j.dsr.2015.09.007
Labatut, M., Lacan, F., Pradoux, C., Chmeleff, J., Radic, A., Murray, J. W., et al. (2014). Iron sources and dissolved- particulate interactions in the seawater of the Western Equatorial Pacific, iron isotope perspectives. Global Biogeochem. Cycles 28, 1044–1065. doi: 10.1002/2014GB004928
Landschützer, P., Gruber, N., and Bakker, D. C. E. (2017). An updated Observation-Based Global Monthly Gridded Sea Surface pCO2 and Air-sea CO2 Flux Product from 1982 Through 2015 and Its Monthly Climatology. (NCEI Accession 0160558). Version 2.2. Asheville, NA: NOAA National Centers for Environmental Information.
Larson, S. M., Pegion, K., and Kirtman, E. P. (2018). The South Pacific meridional mode as a thermally-driven source of ENSO amplitude modulation and uncertainty. J. Clim. 31, 3927–3944. doi: 10.1175/JCLI-D-17-0722.1
Lazo, J. K., Lawson, M., Larsen, P. H., and Waldman, D. M. (2011). United States economic sensitivity to weather variability. Bull. Am. Meteor. Soc. 92, 147–167. doi: 10.1175/2011BAMS2928.1
Le Quéré, C., Andrew, R. M., Friedlingstein, P., Sitch, S., Pongratz, J., Manning, A. C., et al. (2018). Global carbon budget 2017. Earth Syst. Sci. Data 10, 405–448. doi: 10.5194/essd-10-405-2018
Lee, T., and Fukumori, I. (2003). Interannual-to-decadal variations of tropical-subtropical exchange in the Pacific Ocean: boundary versus interior pycnocline transports. J. Clim. 16, 4022–4042. doi: 10.1175/1520-0442(2003)016<4022:IVOTEI>2.0.CO;2
Lehodey, P., Bertignac, M., Hampton, J., Lewis, A., and Picaut, J. (1997). El Nino Southern Oscillation and tuna in the western Pacific. Nature 389, 715–718. doi: 10.1038/39575
Lengaigne, M., Hausmann, U., Madec, G., Menkes, C., Vialard, J., and Molines, J. M. (2012). Mechanisms controlling warm water volume interannual variations in the equatorial Pacific: diabatic versus adiabatic processes. Clim. Dyn. 38, 1031–1046. doi: 10.1007/s00382-011-1051-z
Li, G., and Xie, S.-P. (2014). Tropical biases in CMIP5 multimodel ensemble: the excessive equatorial Pacific cold tongue and double ITCZ problems. J. Clim. 27, 1765–1780. doi: 10.1175/JCLI-D-13-00337.1
Lindstrom, E. J., Gunn, A., Fischer, A., McCurdy, A., and Glover, L. K. (2012). A Framework for Ocean Observing. By the Task Team for an Integrated Framework for Sustained Ocean Observing. Paris: UNESCO. doi: 10.5270/OceanObs09-FOO
Lloyd, J., Guilyardi, E., and Weller, H. (2012). The role of atmosphere feedbacks during ENSO in the CMIP3 models. Part III: the shortwave flux feedback. J Clim. 25, 4275–4293. doi: 10.1175/JCLI-D-11-00178.1
Lukas, R., Yamagata, T., and McCreary, J. P. (1996). Pacific low-latitude western boundary currents and the Indonesian throughflow. J. Geophys. Res. 101, 12209–12216. doi: 10.1029/96JC01204
Mackey, D. J., O’Sullivan, J. E., and Watson, R. J. (2002). Iron in the Western Pacific: a riverine or hydrothermal source for iron in the equatorial undercurrent? Deep Sea Res. I 49, 877–893. doi: 10.1016/S0967-0637(01)00075-9
Mathis, J. T., Feely, R. A., Sutton, A., Carlson, C., Chai, F., Chavez, F., et al. (2014). White Paper #6- Tropical Pacific Biogeochemistry: Status, Implementation and Gaps. Report of the Tropical Pacific Observing System 2020 Workshop (TPOS 2020), GCOS-184/GOOS-206/WCRP-6/2014, Vol. 2. San Diego, CA: Scripps Institution of Oceanography.
McPhaden, M. J., Busalacchi, A. J., and Anderson, D. L. T. (2010). A TOGA retrospective. Oceanograph 23, 86–103. doi: 10.5670/oceanog.2010.26
McPhaden, M. J., Busalacchi, A. J., Cheney, R., Donguy, J.-R., Gage, K. S., Halpern, D., et al. (1998). The tropical ocean-global atmosphere observing system: a decade of progress. J. Geophys. Res. 103, 14169–14240. doi: 10.1029/97JC02906
Melet, A., Gourdeau, L., Kessler, W. S., Verron, J., and Molines, J. M. (2010). Thermocline circulation in the Solomon Sea: a modeling study. J. Phys. Oceanogr. 40, 1302–1319. doi: 10.1175/2009JPO4264.1
Melet, A., Verron, J., Gourdeau, L., and Koch-Larrouy, A. (2011). Equatorward pathways of Solomon Sea water masses and their modifications. J. Phys. Oceanogr. 41, 810–826. doi: 10.1175/2010JPO4559.1
Montes I., Dewitte, B., Gutknecht, E., Paulmier, A., Dadou, I., and Garçon, V. (2014). High-resolution modeling the oxygen minimum zone of the Eastern Tropical Pacific: sensitivity to the tropical oceanic circulation. J. Geophys. Res. Oceans 119, 5515–5532. doi: 10.1002/2014JC009858
Mosquera-Vásquez, K., DeWitte, B., and Illig, S. (2014). The central pacific El Niño intraseasonal kelvin wave. J. Geophs. Res. Oceans 119, 6605–6621. doi: 10.1002/2014JC010044
Nigam, S., and Chung, C. (2000). ENSO surface winds in CCM3 simulation: diagnosis of errors. J. Clim. 13, 3172–3186. doi: 10.1175/1520-04422000013<3172:ESWICS<2.0.CO;2
Nigam, S., Chung, C., and DeWeaver, E. (2000). ENSO diabatic heating in ECMWF and NCEP–NCAR reanalyses, and NCAR CCM3 simulation. J. Clim. 13, 3152–3171. doi: 10.1175/1520-0442(2000)013<3152:EDHIEA>2.0.CO;2
Oke, P. R., Sakov, P., Cahill, M. L., Dunn, J. R., Fiedler, R., Griffin, D. A., et al. (2012). Towards a dynamically balanced eddy-resolving ocean reanalysis: BRAN3. Ocean Modell. 67, 52–70. doi: 10.1016/j.ocemod.2013.03.008
Oschlies, A., Duteil, O., Getzlaff, J., Koeve, W., Landolfi, A., and Schmidtko, S. (2017). Patterns of deoxygenation: sensitivity to natural and anthropogenic drivers. Philos. Trans. R. Soc. 375:20160325. doi: 10.1098/rsta.2016.0325
Paulmier, A., and Ruiz-Pino, D. (2009). Oxygen minimum zones (OMZs) in the modern ocean. Prog. Oceanogr. 80, 113–128. doi: 10.1029/j.pocean.2008.08.001
Qin, X., Menviel, L., Sen Gupta, A., and van Sebille, E. (2016). Iron sources and pathways into the pacific equatorial undercurrent. Geophys. Res. Lett. 43:18. doi: 10.1002/2016GL070501
Qin, X., Sen Gupta, A., and van Sebille, E. (2015). Variability in the origins and pathways of pacific equatorial undercurrent water. J. Geophys. Res. 120, 3113–3128. doi: 10.1002/2014JC010549
Qiu, B., Rudnick, D. L., Chen, S., and Kashino, Y. (2013). Quasi-stationary north equatorial undercurrent jets across the tropical North Pacific Ocean. Geophys. Res. Lett. 40, 2183–2187. doi: 10.1002/grl.50394
Qu, T., and Lindstrom, E. J. (2004). Northward intrusion of antarctic intermediate water in the western pacific. J. Phys. Oceanogr. 34, 2104–2118. doi: 10.1175/1520-0485(2004)034<2104:NIOAIW>2.0.CO;2
Radic, A., Lacan, F., and Murray, J. W. (2011). Isotopic composition of dissolved iron in the equatorial Pacific Ocean: new constraints for the oceanic iron cycle. Earth Planet. Sci. Lett. 306, 1–10. doi: 10.1016/j.epsl.2011.03.015
Reynolds, R. W., Smith, T. M., Liu, C., Chelton, D. B., Casey, K. S., and Schlax, M. G. (2007). Daily high-resolution-blended analyses for sea surface temperature. J. Clim. 20, 5473–5496. doi: 10.1175/2007JCLI1824.1
Roemmich, D., Cravatte, S., Delcroix, T., Gasparin, F., Hu, D., Johnson, G. C., et al. (2014). White Paper #10—In situ Temperature, Salinity, and Velocity Observations. Report of the Tropical Pacific Observing System 2020 Workshop (TPOS 2020), Vol. II. San Diego, CA: Scripps Institution of Oceanography, 243–271.
Rudnick, D. L., Jan, S., and Lee, C. M. (2015). A new look at circulation in the western North Pacific: Introduction to the special issue. Oceanograph 28, 16–23. doi: 10.5670/oceanog.2015.77
Ryan, J. P., Ueki, I., Chao, Y., Hang, H., Polito, P. S., and Chavez, F. P. (2006). Western Pacific modulation of large phytoplankton blooms in the central and eastern equatorial Pacific. J. Geophys. Res. 111:G02013. doi: 10.1029/2005JG000084
Sassone, P. G., and Weiher, R. F. (1997). “Cost benefit analysis of TOGA and the ENSO observing system,” in Operational Oceanography: The Challenge for European Co-Operation, eds J. H. Stel et al. (Amsterdam: Elsevier Science B.V), 36–50.
Schonau, M. C., and Rudnick, D. L. (2017). Mindanao current and undercurrent: thermohaline structure and transport from repeat glider observations. J. Phys. Oceanogr. 47, 2055–2075. doi: 10.1175/JPO-D-16-0274.1
Schumacher, C., Houze, R. A. Jr., and Kraucunus, I. (2004). The tropical dynamical response to latent heating estimates derived from the TRMM precipitation radar. J. Atmos. Sci. 61, 1341–1358. doi: 10.1175/1520-0469(2004)061<1341:TTDRTL>2.0.CO;2
Sen Gupta, A., McGregor, S., van Sebille, E., Ganachaud, A., Brown, J., and Santoso, A. (2016). Future changes to the indonesian Throughflow and Pacific circulation: the differing role of wind and deep circulation changes. Geophys. Res. Lett. 43, 1669–1678. doi: 10.1002/2016GL067757
Serra, Y. L. (2018). Precipitation measurements from the tropical moored array: a review and look ahead. Q. J. Roy. Meteor. Soc. 144, 221–234. doi: 10.1002/qj.3287
Slemons, L. O., Murray, J. W., Gorgues, T., Aumont, O., and Menkes, C. (2009). Biogeochemical impact of a model western iron source in the Pacific Equatorial Undercurrent. Deep Sea Res. I 56, 2115–2128. doi: 10.1016/j.dsr.2009.08.005
Slemons, L. O., Murray, J. W., Resing, J., Paul, B., and Dutrieux, P. (2010). Western Pacific coastal sources of iron, manganese, and aluminum to the equatorial undercurrent. Global Biogeochem. Cycles 24:3. doi: 10.1029/2009GB003693
Slemons, L. O., Paul, B., Resing, J., and Murray, J. W. (2012). Particulate iron, aluminum, and manganese in the Pacific equatorial undercurrent and low latitude western boundary current sources. Mar. Chem. 14, 54–67. doi: 10.1016/j.marchem.2012.08.003
Sprintall, J., Gordon, A. L., Koch-Larray, A., Lee, T., Potemra, J., Pujiana, K., et al. (2014). The Indonesian seas and their role in the coupled ocean climate system. Nat. Geosci. 7, 487–492. doi: 10.1038/ngeo2188
Sprintall, J., Wijffels, S., Molcard, R., and Jaya, I. (2009). Direct estimates of the Indonesian Throughflow entering the Indian Ocean. J. Geophys. Res. 114:C07001. doi: 10.1029/2008JC005257
Sprintall, J., Gordon, A. L., Wijffels, S. E., Feng, M., Hu, S., Koch-Larrouy, A., et al. (2019). Detecting change in the Indonesian Seas. Submitted to Frontiers in Marine Science, this issue.
Stramma, L., Prince, E. D., Schmidtko, S., Luo, J., Hoolihan, J. P., Visbeck, M., et al. (2012). Expansion of oxygen minimum zones may reduce available habitat for tropical pelagic fishes. Nat. Clim. Change 2, 33–37. doi: 10.1038/nclimate1304
Sutton, A. J., Feely, R. A., Maenner-Jones, S., Musielewicz, S., Osborne, J., Dietrich, C., et al. (2018). Autonomous Seawater Partial Pressure of Carbon Dioxide (pCO2) and pH Time Series from 40 Surface Buoys Between 2004 and 2017 (NCEI Accession 0173932). Version 1.1. Asheville, NA: NOAA National Centers for Environmental Information.
Takahashi, K., and Dewitte, B. (2016). Strong and moderate nonlinear El Niño regimes. Clim. Dyn. doi: 10.1007/s00382-015-2665-3
Takahashi, K., Karamperidou, C., and Dewitte, B. (2018). A theoretical model of strong and moderate El Niño regimes. Clim. Dyn. doi: 10.1007/s00382-018-4100-z
Takahashi, K., and Martínez, A. (2017). The very strong coastal El Niño in 1925 in the far-eastern Pacific. Clim. Dyn. doi: 10.1007/s00382-017-3702-1
Takahashi, K., Martínez, R., Montecinos, A., Dewitte, B., Gutiérrez, D., Rodríguez-Rubio, E. (2014). TPOS White Paper #8a – Regional Applications of Observations in the Eastern Pacific: White Paper #8a- Report of the Tropical Pacific Observing System 2020 Workshop (TPOS 2020) GCOS-184, Vol 2. Geneva: World Meteorological Organization, 171–205.
Tanhua, T., Appeltans, W., Bax, N. J., Currie, K., DeYoung, B., Dunn, D. C., et al. (2019). GOOS, FOO and Governance – Assessments and Strategies. Submitted to Frontiers in Marine Science, this issue.
Tapiador, F. J., Roca, R., Del Genio, A., Dewitte, B., Petersen, W., and Zhang, F. (2018). Is precipitation a good metric of model performance? Bull. Amer. Meteor. Soc. doi: 10.1175/BAMS-D-17-0218.1
Tollefson, J. (2014). El Niño monitoring system in failure mode. Nature. doi: 10.1038/nature.2014.14582
Tommasi, D., Stock, C. A., Pegion, K., Vecchi, G. A., Methot, R. D., Alexander, M. A., et al. (2016). Improved management of small pelagic fisheries through seasonal climate prediction. Ecol. Appl. 27, 378–388. doi: 10.1002/eap.1458
Toniazzo, T. (2010). Climate variability in the south-eastern tropical Pacific and its relation with ENSO: a GCM study. Clim. Dyn. 34, 1093–1114. doi: 10.1007/s00382-009-0602-z
Tsuchiya, M., Lukas, R., Fine, R. A., Firing, E., and Lindstrom, E. (1989). Source waters of the Pacific Equatorial Undercurrent. Progr. Oceanogr. 23, 101–147. doi: 10.1016/0079-6611(89)90012-8
Villas Bôas, A. B., Ardhuin, F., Ayet, A., Bourassa, M. A., Brandt, P., Chapron, B., et al. (2019). Integrated observations and modeling of winds, currents, and waves: requirements and challenges for the next decade. Submitted to Frontiers in Marine Science, this issue.
Wang, F., Wang, J., Guan, C., Ma, Q., and Zhang, D. (2016). Mooring observations of equatorial currents in the upper 1000 m of the western Pacific Ocean during 2014. J. Geophys. Res. Oceans 121, 3730–3740. doi: 10.1002/2015JC011510
Wijffels, S., Firing, E., and Toole, J. (1995). The mean structure and variability of the Mindanao Current at 8°N. J. Geophs. Res. Oceans. 100, 18421–18435. doi: 10.1029/95JC01347
Wijffels, S. E., Meyers, G. M., and Godfrey, J. S. (2008). A 20-year average of the indonesian throughflow: regional currents and the interbasin exchange. J. Phys. Oceanogr. 38, 1965–1978. doi: 10.1175/2008JPO3987.1
Wiles, P., Murphy, B., Ngari, A., White, R., Berdon, J., Hampton, J., Pahalad, J., et al. (2014). The Tropical Pacific Observing System and the Pacific Islands, White Paper #8b. Report of the Tropical Pacific Observing System 2020 Workshop (TPOS 2020), Vol. II. San Diego, CA: Scripps Institution of Oceanography.
World Meteorological Organization [WMO] (2012). Proceedings of the WMO Regional Association VI Conference on Social and Economic Benefits of Weather, Climate and Water Services. Geneva: World Meteorological Organization, 86.
World Meteorological Organization [WMO] (2017). Executive Council – Sixty-Ninth Session: Abridged Final Report with Resolutions and Decisions. Geneva: World Meteorological Organization.
Wu, Z.H. (2003). A shallow CISK, deep equilibrium mechanism for the interaction between large-scale convection and large-scale circulations in the tropics. J. Atmos. Sci. 60, 377–392. doi: 10.1175/1520-04692003060<0377:ASCDEM<2.0.CO;2
Wyrtki, K. (1987). Indonesian throughflow and the associated pressure gradient. J. Geophys. Res. 92, 12941–12946. doi: 10.1029/JC092iC12p12941
Xue, Y., Wen, C., Yang, X., Behringer, D., Kumar, A., Vecchi, G., et al. (2017). Evaluation of tropical Pacific observing systems using NCEP and GFDL ocean data assimilation systems. Clim. Dyn. 49, 843–868. doi: 10.1007/s00382-015-2743-6
Zhang, C., McGauley, M., Bond, N.A. (2004). Shallow meridional circulation in the tropical eastern pacific. J. Clim. 17, 133–139. doi: 10.1175/1520-04422004017<0133:SMCITT<2.0.CO;2
Zhang, H. H., Clement, A., and Di Nezio, P. (2014). The South Pacific meridional mode: a mechanism for ENSO-like variability. J. Clim. 27, 769–783. doi: 10.1175/JCLI-D-13-00082.1
Zhang, X., Liu, H., and Zhang, M. (2015). Double ITCZ in coupled Ocean-atmosphere models: from CMIP3 to CMIP5. Geophys. Res. Lett. 42, 8651–8659. doi: 10.1002/2015GL065973
Keywords: ocean observing, tropical Pacific, TPOS 2020, user requirements, variable requirements, design, tropical moorings
Citation: Smith N, Kessler WS, Cravatte S, Sprintall J, Wijffels S, Cronin MF, Sutton A, Serra YL, Dewitte B, Strutton PG, Hill K, Sen Gupta A, Lin X, Takahashi K, Chen D and Brunner S (2019) Tropical Pacific Observing System. Front. Mar. Sci. 6:31. doi: 10.3389/fmars.2019.00031
Received: 28 October 2018; Accepted: 21 January 2019;
Published: 18 February 2019.
Edited by:
John Siddorn, Met Office, United KingdomReviewed by:
Oscar Schofield, Rutgers, The State University of New Jersey, United StatesCopyright © 2019 Smith, Kessler, Cravatte, Sprintall, Wijffels, Cronin, Sutton, Serra, Dewitte, Strutton, Hill, Sen Gupta, Lin, Takahashi, Chen and Brunner. This is an open-access article distributed under the terms of the Creative Commons Attribution License (CC BY). The use, distribution or reproduction in other forums is permitted, provided the original author(s) and the copyright owner(s) are credited and that the original publication in this journal is cited, in accordance with accepted academic practice. No use, distribution or reproduction is permitted which does not comply with these terms.
*Correspondence: Neville Smith, bnNtaTMxMThAb3V0bG9vay5jb20=
Disclaimer: All claims expressed in this article are solely those of the authors and do not necessarily represent those of their affiliated organizations, or those of the publisher, the editors and the reviewers. Any product that may be evaluated in this article or claim that may be made by its manufacturer is not guaranteed or endorsed by the publisher.
Research integrity at Frontiers
Learn more about the work of our research integrity team to safeguard the quality of each article we publish.