- 1School of Environment and Science, Australian Rivers Institute, Griffith University, Brisbane, QLD, Australia
- 2Department of Chemistry, University of Cambridge, Cambridge, United Kingdom
- 3Scripps Institution of Oceanography, University of California, San Diego, La Jolla, CA, United States
- 4Marine Biological Section, Department of Biology, University of Copenhagen, Helsingør, Denmark
- 5Laboratory for Biological Geochemistry, School of Architecture, Civil and Environmental Engineering, Ecole Polytechnique Fédérale de Lausanne, Lausanne, Switzerland
- 6Climate Change Cluster, University of Technology Sydney, Ultimo, NSW, Australia
- 7ARC Centre of Excellence for Coral Reef Studies, Townsville, QLD, Australia
Crustose coralline algae (CCA) are key organisms in coral reef ecosystems, where they contribute to reef building and substrate stabilization. While ocean acidification due to increasing CO2 can affect the biology, physiology and ecology of fully developed CCA, the impacts of elevated CO2 on the early life stages of CCA are much less explored. We assessed the photosynthetic activity and growth of 10-day-old recruits of the reef-building crustose coralline alga Porolithon cf. onkodes exposed to ambient and enhanced CO2 seawater concentration causing a downward shift in pH of ∼0.3 units. Growth of the CCA was estimated using measurements of crust thickness and marginal expansion, while photosynthetic activity was studied with O2 microsensors. We found that elevated seawater CO2 enhanced gross photosynthesis and respiration, but significantly reduced vertical and marginal growth of the early life stages of P. cf. onkodes. Elevated CO2 stimulated photosynthesis, particularly at high irradiance, likely due to increased availability of CO2, but this increase did not translate into increased algal growth as expected, suggesting a decoupling of these two processes under ocean acidification scenarios. This study confirms the sensitivity of early stages of CCA to elevated CO2 and identifies complexities in the physiological processes underlying the decreased growth and abundance in these important coral reef builders upon ocean acidification.
Introduction
Crustose coralline algae (CCA) are abundant in tropical and temperate reef ecosystems, where they provide important ecosystem functions (Littler, 1972; Littler and Doty, 1975). CCA contribute to the stabilization of coral reef frameworks by consolidating and cementing loose rubble and by sealing porous dead corals skeleton against mechanical erosion (Littler, 1972; Adey, 1998). CCA also provide food and habitat for a range of reef organisms such as parrot fish and sea urchins (Littler and Doty, 1975; Steneck, 1983) and are preferred settlement substrates for larvae of corals and other invertebrates (Heyward and Negri, 1999; Harrington et al., 2004). The presence of CCA is thus crucial for the health and recovery of coral reefs following disturbances.
The current and projected increase in atmospheric pCO2 and the derived ocean acidification (OA) due to a decrease in seawater pH and calcium carbonate (CaCO3) saturation state (Ω) is critical for the integrity and fitness of calcifying organisms (Anthony et al., 2008; Kroeker et al., 2010). CCA are among the marine organisms most sensitive to OA as their skeleton is predominantly formed by high-magnesium calcite, a highly soluble form of CaCO3 (Morse et al., 2007). Rates of net calcification and growth of CCA are generally negatively affected by OA (Anthony et al., 2008; Kuffner et al., 2008; Martin and Gattuso, 2009) due to reduced availability of carbonate ions (CO32-) for calcification (Feely et al., 2004) and increased skeletal dissolution of existing calcium carbonate (Diaz-Pulido et al., 2014). On the other hand, increased atmospheric CO2 increases the concentration of dissolved CO2 and bicarbonate ions (HCO3-) in seawater potentially alleviating inorganic carbon limitation (Larkum et al., 2003) and thus stimulating algal photosynthesis. However, the available experimental evidence shows that the responses of CCA photosynthesis to OA are highly variable, including positive (Semesi et al., 2009) or negative effects (Anthony et al., 2008; Gao and Zheng, 2010; Martin et al., 2013), as well as no significant response (Noisette et al., 2013; Johnson et al., 2014; Comeau et al., 2017). This variability in response to OA may be due to a number of reasons, including use of different experimental setups and time scales (e.g., 1 year experiment with Lithophyllum cabiochae vs. 3 months experiment with Lithophyllum incrustans Martin et al., 2013; Noisette et al., 2013), different life history stages (e.g., recruits vs. adults), and flexibility of different CCA species in their use of inorganic carbon uptake strategies, i.e., use of carbon concentrating mechanisms (CCM) vs. diffusive CO2 uptake (Cornwall et al., 2012, 2015; Diaz-Pulido et al., 2016).
Increased availability of dissolved inorganic carbon (DIC) is expected to enhance algal photosynthesis and consequently algal growth rates (Roleda et al., 2012). However, growth responses to OA have been less explored in coralline algae compared to fleshy macroalgae (e.g., Kroeker et al., 2013), and in most studies changes in CCA growth rates have been based on changes in crust weight rather than on a direct measurement of algal growth (e.g., Anthony et al., 2008; Johnson and Carpenter, 2012). Changes in crust weight have the innate difficulty of not allowing distinction between new growth and skeletal dissolution (Lewis et al., 2017). The relationship between photosynthesis and growth rates has been previously studied in fleshy macroalgae (e.g., Koch et al., 2013), where elevated CO2 enhanced photosynthetic activity and tissue growth in two species of red algae Gracilaria (Gao et al., 1993), but similar relations in CCA remain unexplored.
Existing knowledge on the physiological responses of elevated CO2 on tropical CCA comes from studies on adult crusts (Anthony et al., 2008; Johnson et al., 2014; Comeau et al., 2017). In our previous studies using the reef building CCA Porolithon onkodes, we explored the impacts of OA and warming on spore germination and germling growth and found these processes to be highly sensitive to elevated CO2 (Ordoñez et al., 2017). Roleda et al. (2015) tested the effects of OA on growth of temperate coralline algae recruits and found a direct negative response of crust size upon exposure to OA conditions. Studies of the early life stages of tropical (Kuffner et al., 2008; Fabricius et al., 2015; Ordoñez et al., 2017) and temperate (Bradassi et al., 2013; Roleda et al., 2015; Guenther et al., 2018) CCA have demonstrated a high vulnerability to elevated CO2, as well as other environmental stressors (Santelices, 1990). However, very little is known about the effects of OA on photosynthesis and the coupling between photosynthesis and growth rates in the early life stages of CCA (but see Cornwall et al., 2013). In the present study, we explore the effect of elevated CO2 conditions on the photosynthetic activity and growth rates of recruits of a dominant crustose coralline alga in the Great Barrier Reef, Australia. We used O2 microsensors due to the small size of the CCA recruits (around 0.004–002 mm2 surface area), and because microsensors can decouple light respiration from gross oxygen production, as compared to chamber based incubation measurements (Revsbech and Jorgensen, 1983; Larkum et al., 2003).
Materials and Methods
Experimental Approach
The effects of ocean acidification on rates of growth and photosynthesis of CCA recruits were investigated by exposing Porolithon cf. onkodes spores to elevated and ambient CO2 conditions in the laboratory during a 4 week experiment. Dynamics of O2 concentration (measured using O2 microsensors) and crust thickness (measured using scanning electron microscopy, SEM) of 10-day-old recruits were estimated at the end of the experiment. The experiment was conducted in an indoor laboratory at Heron Island Research Station (HIRS), Great Barrier Reef (GBR), Australia during the summer of 2014 (February–March 2014). The species P. cf. onkodes was used for this experiment due to its important role as a reef builder and cementer (Littler, 1972), and because it is a common alga in tropical reefs, especially in the Great Barrier Reef (Ringeltaube and Harvey, 2005; Dean et al., 2015). Identification of P. cf. onkodes (Heydrich) Foslie was obtained with field and laboratory observations. Microscope techniques such as Scanning Electron Microscopy and histology were used to examine morphological and anatomical characteristics. The encrusting coralline alga P. cf. onkodes used for this experiment is usually found on the reef crest (approx. 3–5 m depth), has a pink-orange color surface with granular appearance due to the presence of numerous tightly packed mega cells in horizontal fields (trichocytes fields), reproductive structures (conceptacles) are unipored, small, flush or slightly raised, cell fusions are present and thallus has a non-coaxial organization. DNA sequences of individuals of this species can be found in GenBank (Accession No. MF979936, see Gabrielson et al., 2018).
Cultivation of Recruits and Experimental Setup
To obtain CCA spores for the experiment, spore release from reproductive P. cf. onkodes fragments was induced in the laboratory following a combination of methods described in Jones and Moorjani (1973); Ichiki et al. (2000), Roleda et al. (2012), and Ordoñez et al. (2017). Adult P. cf. onkodes fragments (3 cm × 3 cm) were collected from the reef crest (ca 5 m depth at highest tide) at Harry’s Bommie (Heron Island, GBR, 23°27′631″S, 151°55′798″E) using hammer and chisel. After collection, fragments were cleaned from epiphytes with a soft brush and rinsed with filtered sterilized seawater. Seawater was filtered twice: first using a hand-made sand filter containing a cotton filter mat and then a house water filter with a 5 μm cartridge (house water filter OMNI OPAQUE), seawater was then sterilized using an aquarium ultra violet sterilizer (Pro Aqua UV sterilizer). Spore release was then induced by placing the fragments on a tray with no water and in a dark, cold (18°C) room for 30 min. Subsequently, sterilized ambient seawater was added to the tray, which was then placed under an artificial metal-halide lamp (Aqua Medic Ocean Light Plus equipped with one 150 W aqualine bulb and two 24 W T5 blue fluorescent bulbs) for 7 h. Immediately after the illumination period, adults commenced spore release and were carefully transferred to the experimental containers (1 L plastic containers) where spore release continued under experimental conditions. Three replicate containers (n = 3) were used for each CO2 treatment (6 experimental containers in total, 3 for ambient CO2 and 3 for high CO2). One transparent polystyrene Petri dish (94 mm × 16 mm diameter) was placed on the bottom of each experimental container to provide substratum for spore settlement and growth. Three adult fragments were randomly assigned to each experimental container to obtain sufficient spores for analysis. However, adults were removed after 4 h, when the first spore attachment was detected, for the following two reasons: (1) To avoid having individuals at different growth stages and (2) to have sufficient number of spores to get a good estimate of their individual growth, but only enough spores to avoid those that had settled close to each other to coalesce. The margin of coalesced spores are difficult to distinguish making individual measurements hard to perform.
Spores were cultured for a period of 10 days in seawater under (i) ambient CO2 (400.8–448.1 μatm) and pH 8.00–8.03 (National Bureau of Standards/National Institute of Standards and Technology) and (ii) high CO2 (998.9–1070.6 μatm) and pH 7.67–7.70. The high CO2 treatment corresponded to the representative concentration pathway 8.5 scenario (RCP 8.5), i.e., the worst case OA scenario predicted by the end of the century (year 2100) according to the Intergovernmental Panel on Climate Change (IPCC, 2014). To achieve the target pH for the high CO2 treatment, analytical grade CO2 gas (BOC Limited Australia) was injected into a 120 L mixing sump using an aquarium control system (Aquatronica, AEB Technologies, Italy). Seawater pH was monitored in the mixing sump by temperature compensated pH electrodes (inPro4501VP, Mettler-Toledo, Switzerland). When seawater pH exceeded the desired threshold, the control system opened solenoid valves to inject CO2 into the mixing sump as previously described in Diaz-Pulido et al. (2011). CO2 conditioned seawater was pumped continuously into experimental containers at a rate of 500 mL min-1. The same set up was used for ambient CO2 treatment, but with untreated seawater in the mixing sump. Mixing sumps were constantly fed with seawater from the Heron Island reef flat. pH probes were calibrated daily to 0.01 pH units with three NIST-certified pH buffers (Mettler-Toledo, Switzerland). Temperatures in both sumps (ambient CO2 and high CO2) and in one container of each treatment was constantly monitored every 30 s and recorded by the aquarium control system. In addition, pH and temperature measurements were frequently taken on experimental containers with a portable pH and temperature meter (SG98-B-SevenGo Duo Pro, Mettler-Toledo, Switzerland) to ensure the pH and temperature were kept constant. Illumination was provided with metal-halide lamps (Aqua Medic Ocean Light Plus equipped with one 150 W aqualine bulb and two 24 W T5 blue fluorescent bulbs) over a 12 h light: 12 h dark photoperiod under an irradiance of 160 μmol photons m-2 s-1, as measured by cosine corrected quantum sensor connected to a light meter (Li-COR, United States). Total alkalinity, pH and salinity values were used to estimate the concentration of dissolved inorganic carbon (pCO2, HCO3- and CO32-) using Microsoft Excel CO2SYS version 2.1 (Pierrot et al., 2006). The saturation state of seawater with respect to high-Mg-calcite was calculated for a 16.4 mol% MgCO3, following a protocol described in (Diaz-Pulido et al., 2012). Carbonate chemistry parameters are shown in Table 1.
O2 Microsensor Measurements
Microscale O2 concentration measurements were done with Clark-type O2 microsensors (Revsbech, 1989) connected to a pA-meter (PA2000, Unisense A/S, Aarhus, Denmark) interfaced to a PC via an A/D converter (Figure 1). The O2 microsensors (OX25; Unisense, Denmark) had a measuring tip diameter of 25 μm, a t90 response time of <0.5 s, a stirring sensitivity of the measuring signal of <2–3%, and a detection limit of ∼0.3 μM1. The O2 microsensors were linearly calibrated from signal readings in air saturated seawater and anoxic seawater (flushed with N2). The O2 concentration (μmol O2 L-1) in air saturated seawater at experimental temperature and salinity was determined using tabulated values of O2 solubility in water at defined temperature and salinity1.
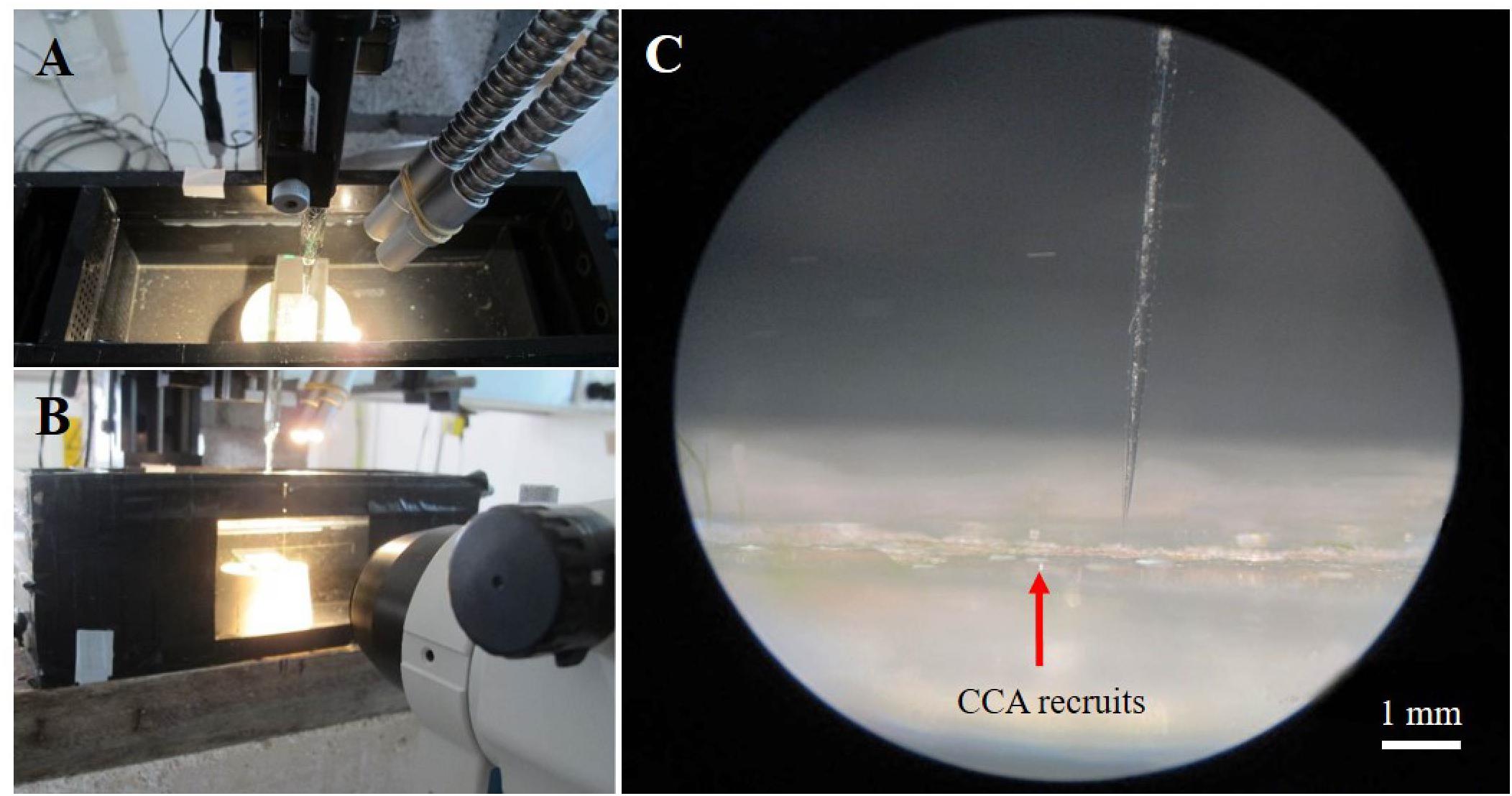
Figure 1. Setup for O2 microsensor measurements on crustose coralline algae. General overview of the set-up showing a flow-chamber with a mounted CCA sample (A,B), with an O2 microsensor mounted in a manual micromanipulator, illumination via a fiber-optic halogen lamp, and observation of the sample via a dissection microscope (B). (C) Close up image (via the dissection microscope) of an O2 microsensor performing O2 measurements on CCA recruits (pink crust).
The O2 microsensors were mounted on a PC-controlled motorized micromanipulator for automatic profiling (Pyro Science GmbH, Germany) at an angle of 15° relative to the vertically incident collimated light from a fiber-optic tungsten-halogen lamp (KL-2500, Schott GmbH, Germany), equipped with a heat filter and a collimating lens. Positioning of the microsensor as well as data acquisition of microsensor signals was facilitated by customized software (Profix, Pyro-Science GmbH, Germany). Experiments were conducted with CCA recruits placed in a custom-made black acrylic flow chamber supplied with seawater at a flow velocity of ∼1–2 cm s-1 (Brodersen et al., 2014). We used the same seawater as in the different CO2 treatments. The downwelling photon irradiance (PAR, 400–700 nm) was measured for defined lamp settings with a calibrated cosine corrected quantum sensor connected to a light meter (LI-250A, Li-COR). For the high CO2 treatment, the seawater taken from the treatment sumps was exchanged every 20–30 min in order to account for changes in pH related to atmospheric CO2 exchange. The pH in the water was monitored constantly with a portable pH meter (SG98-B-SevenGo Duo Pro, Mettler-Toledo, Switzerland) calibrated with NIST-certified pH buffers (Mettler-Toledo, Switzerland) to 0.01 pH units. Recruits from 3 ambient and 2 high CO2 experimental tanks were used for O2 microsensor measurements. Only two replicates could be used from high CO2 treatments due to unexpected experimental constrains. However, around 6–9 measurements were taken on different spots within a single recruit with the objective of including and understanding spatial variations within the recruit.
After measurements of steady-state O2 concentrations at the surface of CCA samples under defined photon irradiance levels, the microsensor tip was positioned at the CCA surface, where the local volume-specific gross photosynthesis rate (in units of nmol O2 cm-3 s-1) was measured from the immediate O2 depletion rate during a brief 1–3 s darkening according to the microsensor light–dark shift technique (Revsbech and Jorgensen, 1983); for these measurements, microsensor signals were recorded on a fast-responding strip-chart recorder (BD25, Kipp & Zonen, Netherlands).
The diffusive flux of O2 between CCA and the overlaying water across the diffusive boundary layer (DBL), J, was determined from steady state O2 concentration profiles using Fick’s first law of diffusion: , where dC/dz is the slope of the O2 concentration profile in the DBL, and D0 is the molecular diffusion coefficient of O2 in seawater at experimental temperature and salinity (2.1707 × 10-5 cm2 s-1), as taken from tabulated values2.
To examine the effects of elevated CO2 on the photosynthetic performance of the CCA, we measured gross and net photosynthesis as a function of increasing photon irradiance (P-E curves). Each specimen was incubated for about 15 min at each irradiance level before microsensor measurements commenced to provide steady state O2 conditions. Gross photosynthesis data was fitted to an exponential function (Webb et al., 1974): , where Pmax is the maximum photosynthetic rate and α is the photosynthetic efficiency, i.e., the initial slope of the P-E curve. The saturation irradiance (Ek) was calculated as Ek = Pmax/α, and describes the photon irradiance above which photosynthesis approaches saturation. Net photosynthesis data were fitted to the modified exponential function used in Roberts et al. (2002): + R. This function considers the term of respiration (R) and assumes absence of photoinhibition.
Vertical and Marginal Growth Measurements
Vertical growth of the juvenile CCA samples was estimated at the end of the experiment by measuring the crust thickness using a scanning electron microscope (JSM-6510 series, JEOL). To obtain cross sections, recruits attached to the petri dish were carefully detached from the substrate and sliced into sections using a razor blade. The resulting fragments were mounted on a metal stub with adhesive. Samples were carbon coated using a sputter coater (JFC-1600 auto fine coater, JEOL) for SEM analyses. Images were taken with a magnification of ×3000 at a high voltage of 5 kV with a spot size of 50 nm and a working distance (WD) of 12–16 mm. We measured a total of 15 points along the crust from different fragments. To estimate thickness of the crust, the distance from the surface of the crust to the bottom was measured using the SEM software (JEOL Scanning electron microscope software) (Figures 2B,D). Growth rates in units of μm crust increment day-1 were obtained by normalizing crust thickness to the duration of the experiment, i.e., 10 days. Marginal growth was estimated by measuring the change in surface area. Final and initial photographs of individual recruits were taken and images were analyzed using Image J software (University of Wisconsin-Madison).
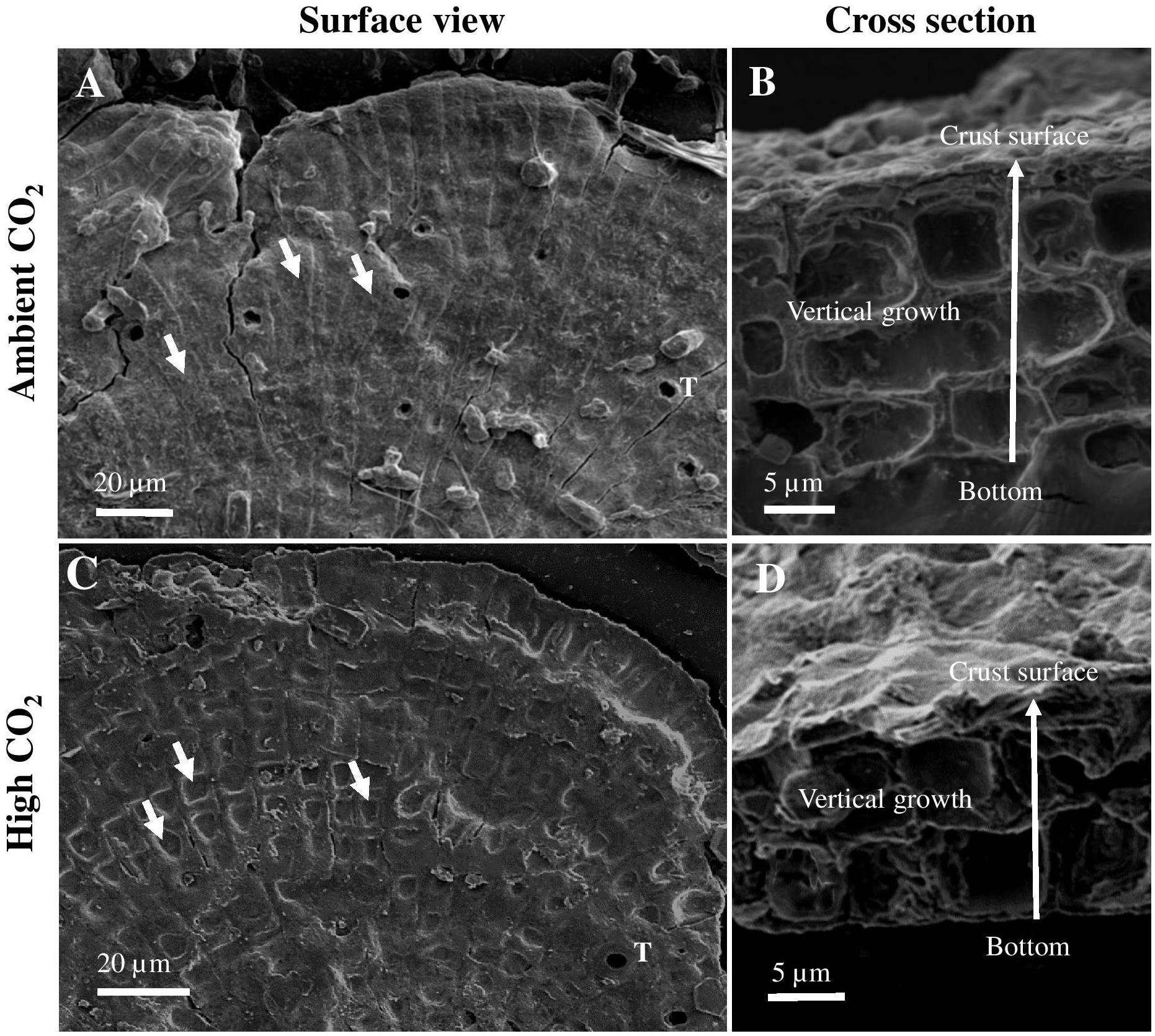
Figure 2. Scanning electron microscopy images of surface view (A,C) and cross section (B,D) of 10-day-old Porolithon cf. onkodes recruits developed under ambient CO2 (CO2 between 400.8 and 448.1 μatm, pH 8.0–8.03) and high CO2 (CO2 between 998.9 and 1070.6 μatm, pH 7.67–7.70) conditions. Arrows in panels (B,D) indicate vertical growth direction. Short white arrows in panel (A) indicate non-collapsed epithallial/perithallial cells and in panel (C) collapsed epithallial/perithallial cells. White T in panels (A,C) indicate first appearance of mega cells (trichocytes).
Statistical Analyses
Photosynthetic parameters were compared between treatments using one-way ANOVA. Pearson correlation was used to test the relationships between vertical growth and gross photosynthesis. Normal distribution of data and homogeneity of variances were tested using Kolmogorov-Smirnov and Cochran’s test and data was arc-sin transformed when needed. All statistical analyses were performed using SPSS (version 25).
Results
Photosynthesis and Respiration
The measured P-E curves showed a significant difference between recruits growing under elevated CO2 and recruits growing under ambient CO2 conditions (Figures 3A,B). Maximum gross photosynthesis was 50% higher under elevated CO2 [mean Pmax = 75.36 nmol O2 cm-3 s-1 (±9.7 SE)] as compared to ambient CO2 conditions [mean Pmax = 37.36 nmol O2 cm-3 s-1 (±7.8 SE)], ANOVA p = 0.044). Photosynthetic efficiency and saturation irradiance were slightly higher under high CO2 when compared to ambient CO2, but the difference was not statistically significant (ANOVA, α: p = 0.22, Ek: p = 0.12). At the maximum photon irradiance used in the experiment (340 μmol photons m-2 s-1), gross photosynthesis was 39% higher under high CO2 than under ambient CO2 conditions but the difference was not significant (ANOVA p = 0.081, Figure 4A).
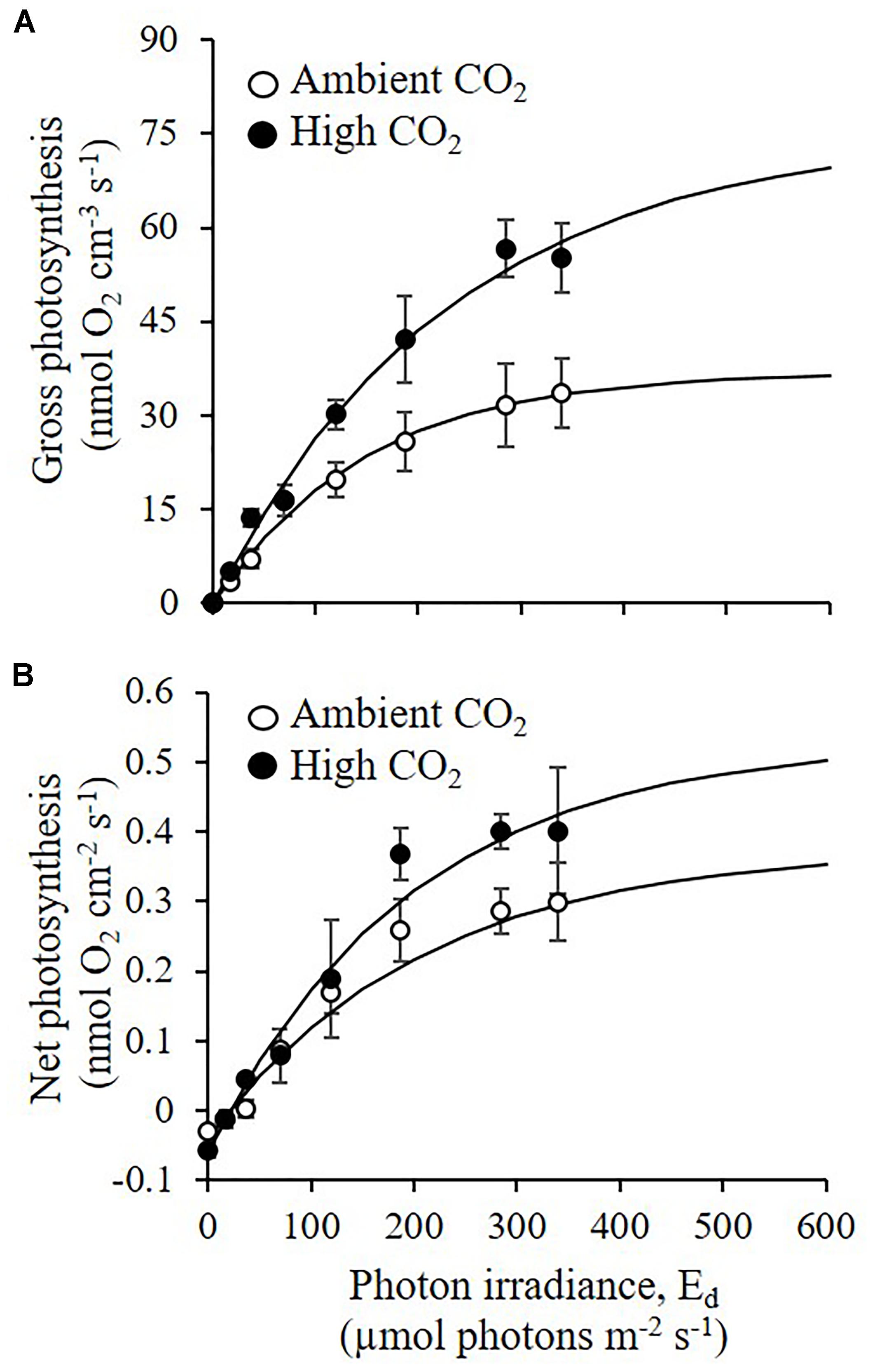
Figure 3. Effects of elevated CO2 on net and gross photosynthesis of CCA recruits. (A) Gross photosynthesis (nmol O2 cm-3 s-1), and (B) net photosynthesis (nmol O2 cm-2 s-1) were measured under ambient CO2 (CO2 between 400.8 and 448.1 μatm, pH 8.0–8.03) and high CO2 (CO2 between 998.9 and 1070.6 μatm, pH 7.67–7.70) conditions, for a range of incident downwelling photon irradiance regimes (Ed: μmol photons m-2 s-1). Symbols with error bars represent means ± SE (n = 3 for ambient CO2 and n = 2 for High CO2; biological replicates). Solid lines represent curve fits (R2 > 0.9) of the equation for gross photosynthesis and the equation + R for net photosynthesis.
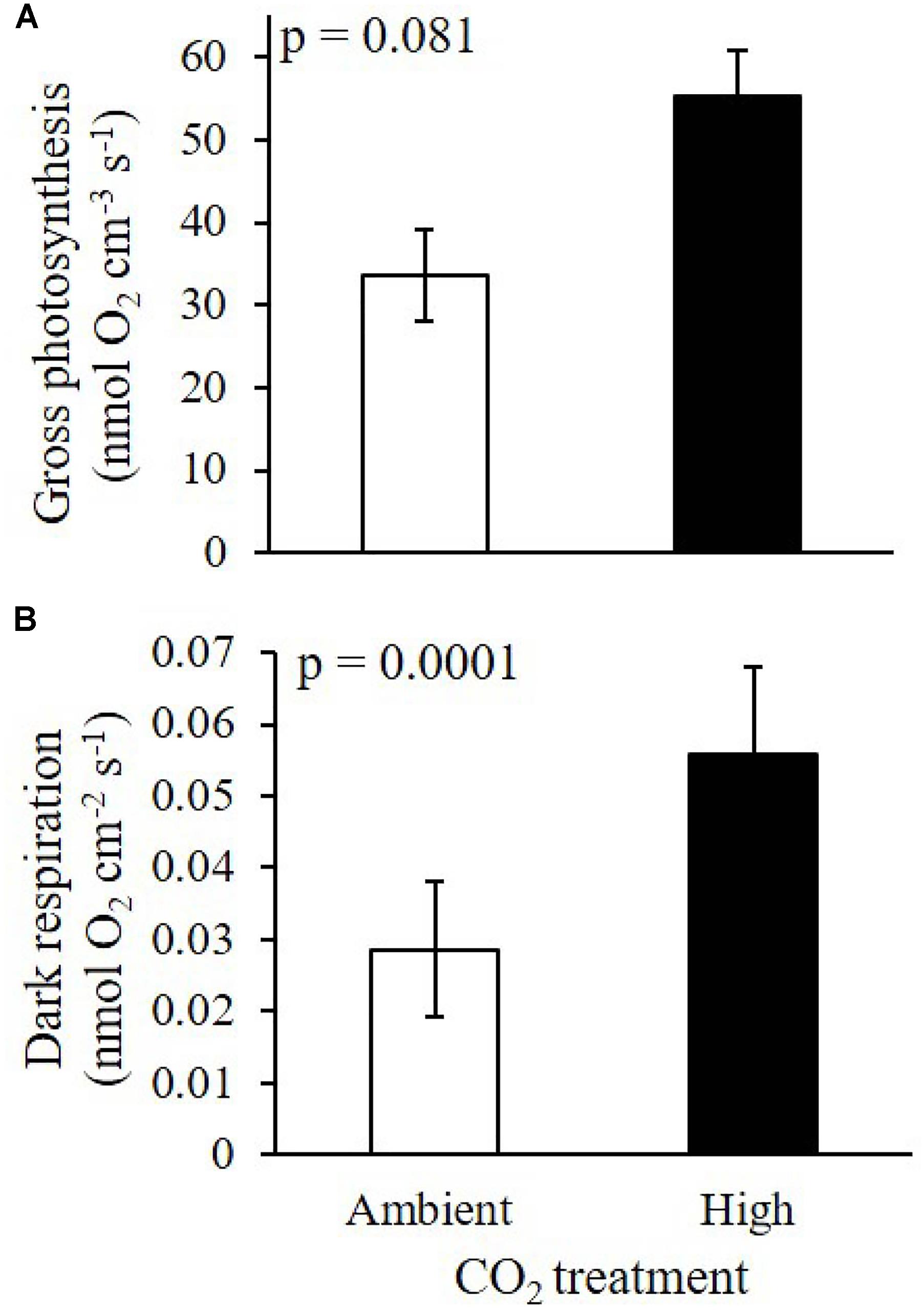
Figure 4. Effects of elevated CO2 on (A) surface gross photosynthesis, and (B) dark respiration of 10-day-old CCA recruits under two treatments: ambient CO2 (CO2 between 400.8 and 448.1 μatm, pH 8.0–8.03) and high CO2 (CO2 between 998.9 and 1070.6 μatm, pH 7.67–7.70). Gross photosynthesis was measured at the CCA surface at a photon irradiance of 340 μmol photons m-2 s-1. Data on panel (A) are means ± SE (n = 3 for ambient CO2 and n = 2 for high CO2; biological replicates). Data on panel (B) are means ± SE (n = 9 for each CO2 treatment).
The responses of net photosynthetic rates to the CO2 treatments followed a similar trend as the gross photosynthesis, with increased Pmax, and slightly higher α and Ek values under elevated CO2 than under ambient CO2 conditions. However, the difference across CO2 treatments was less pronounced and not statistically significant (ANOVA, p = 0.12, p = 0.78, p = 0.35, for Pmax, α and Ek, respectively, Figure 3B).
Dark respiration was significantly enhanced by elevated CO2, with recruits showing a 48.7% increase in respiration rates compared to recruits from ambient CO2 (ANOVA, p = 0.0001, Figure 4B). In addition, surface dynamics of O2 concentration during experimental dark-light shifts showed higher O2 production and faster response of CCA recruits under elevated CO2 compared to measurements under ambient CO2 (Figure 5).
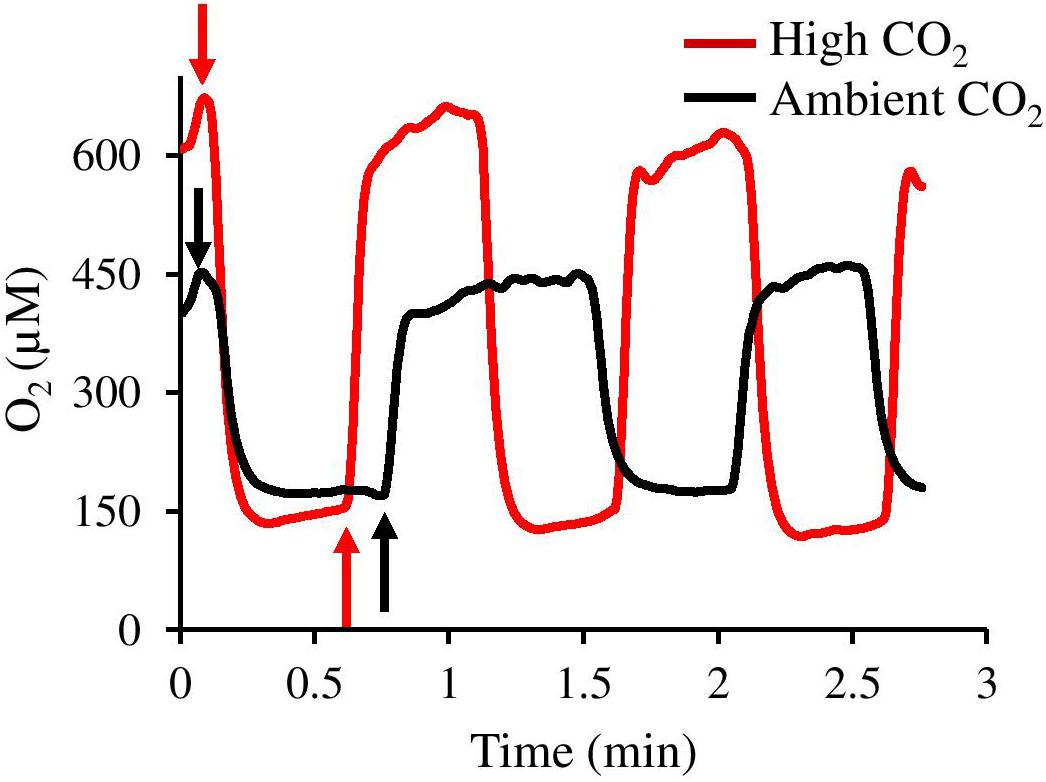
Figure 5. Surface O2 concentration dynamics of CCA recruits during light-dark shifts under ambient CO2 (black line, CO2 between 400.8 and 448.1 μatm, pH 8.0–8.03) and high CO2 (red line, CO2 between 998.9 and 1070.6 μatm, pH 7.67–7.70). Upward arrows and downward arrows exemplify onset of light and dark periods, respectively.
Growth Rates
Vertical and marginal growth rates were significantly affected by high CO2 (ANOVA, p = 0.01 and 0.03, respectively). P. cf. onkodes recruits grown under ambient CO2 conditions showed thicker and larger crusts as compared to recruits exposed to high CO2 conditions (Figures 6A,B). Crust vertical growth rates were reduced from 2.8 ± 0.24 μm d-1 under ambient CO2 to 2.05 μm d-1 (±0.04 SE) under high CO2, i.e., a 28.7% change. Marginal growth rates were also reduced by 19%. Structural damage of the cells under high CO2 was observed on the crust surface, where epithallial/perithallial cells seemed to have collapsed (Figure 2C) as opposed to observations of the crust surface of germlings growing under ambient conditions where cells seem to preserve their structure (Figure 2A). Furthermore, cross sections of CCA recruits showed cells with irregular shapes and thinner cell walls in samples exposed to elevated CO2 (Figure 2D) compared to those under ambient conditions (Figure 2B).
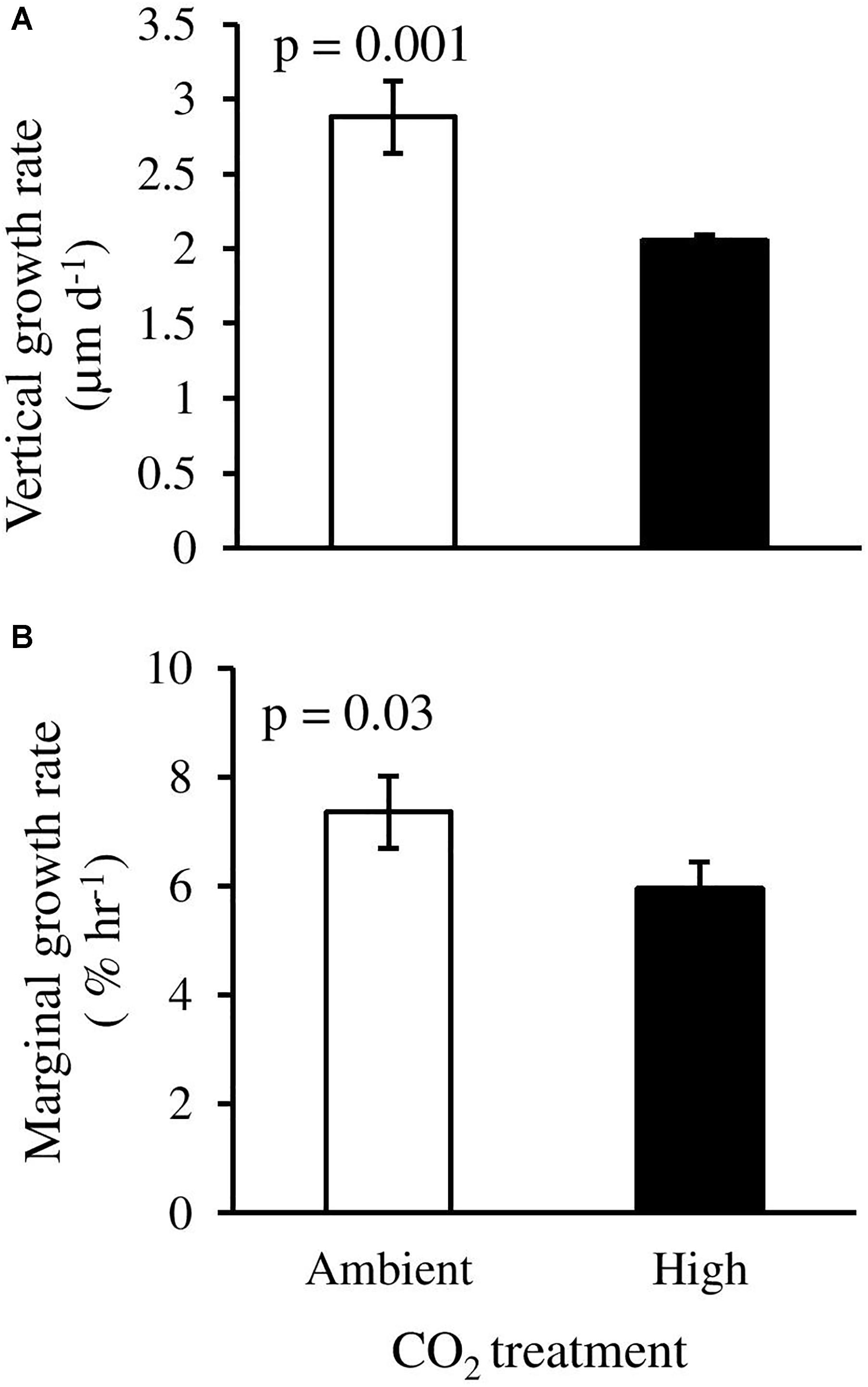
Figure 6. Effects of elevated CO2 on (A) vertical growth rate, and (B) marginal growth rate of CCA recruits in response to two treatments: ambient CO2 (CO2 between 400.8 and 448.1 μatm, pH 8.0–8.03) and high CO2 (CO2 between 998.9 and 1070.6 μatm, pH 7.67–7.70). Columns with error bars represent means ± SE [(A): n = 3 for ambient CO2 and n = 2 for high CO2; (B) n = 6, biological replicates].
Growth vs. Photosynthesis
We found a negative correlation between gross photosynthesis and crust thickness of P. cf. onkodes recruits at an incident irradiance of 340 μmol photons m-2 s-1 (Pearson correlation coefficient 0.89, p = 0.01, n = 5, Figure 7). The highest gross photosynthetic rate (55.16 nmol O2 cm-3 s-1) was found on thinnest recruits (1.93 μm d-1) growing under elevated CO2, whereas the lowest gross photosynthetic rate (3.29 nmol O2 cm-3 s-1) was measured on the thickest recruits (3.35 μm d-1) from ambient CO2 condition.
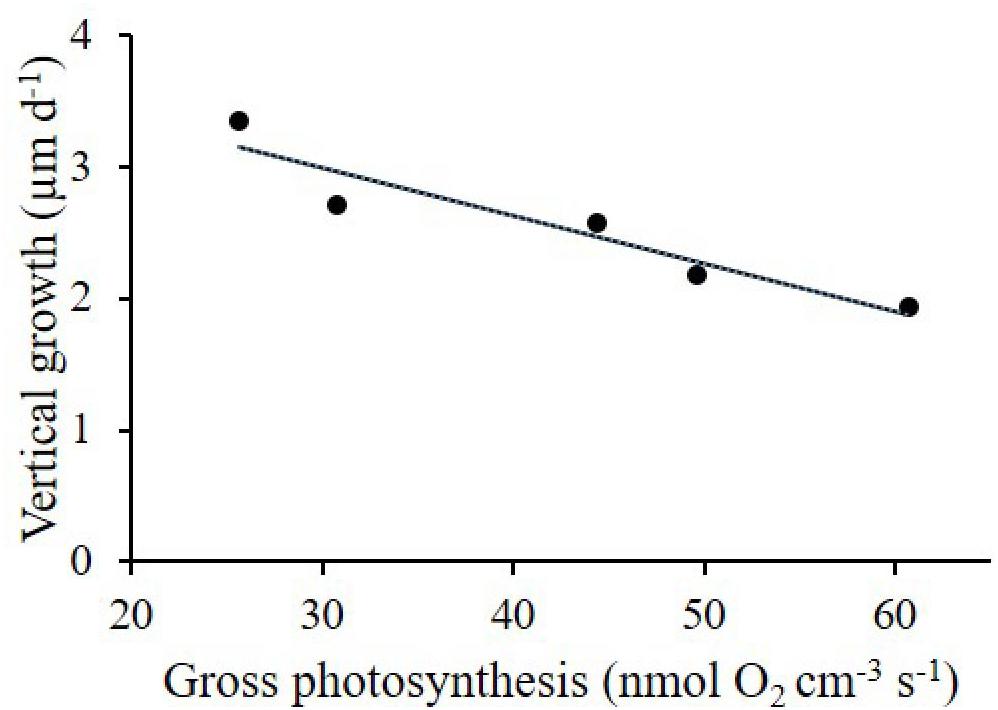
Figure 7. Negative linear correlation (R2 = 0.89; p = 0.016) between vertical growth (μm d-1) and gross photosynthesis (nmol O2 cm-3 s-1) of CCA recruits grown under ambient CO2 (CO2 between 400.8 and 448.1 μatm, pH 8.0–8.03) and high CO2 (CO2 between 998.9 and 1070.6 μatm, pH 7.67–7.70).
Discussion
The impacts of ocean acidification (OA) on adult coralline algae have been relatively well documented (Hofmann and Bischof, 2014), and there is a growing body of evidence supporting the sensitivity of the early life history stages of coralline algae to OA. In particular, recent studies have shown reduced spore germination (Bradassi et al., 2013; Ordoñez et al., 2017) and attachment (Guenther et al., 2018) and declined germling abundance [e.g., % cover, Kuffner et al. (2008); Ordoñez et al. (2014); Fabricius et al. (2015); Ordoñez et al. (2017)] and growth (Roleda et al., 2015; Ordoñez et al., 2017) under elevated CO2 conditions. However, whether metabolic processes such as photosynthesis and respiration of early life stages of CCA also show a negative response to elevated CO2 has not been well documented. Our study tests for the first time the effects of elevated CO2 on the photosynthesis and respiration of recruits of a major reef building coralline alga. We demonstrate that increased CO2 can potentially enhance gross photosynthesis and dark respiration, but can reduce the growth of P. cf. onkodes recruits. Our findings also reveal a possible decoupling between photosynthesis and growth rates in CCA recruits, suggesting that the carbon fixed during the photosynthetic process does not necessarily translate into enhanced algal growth. Understanding the relationships between physiological and vital processes under OA, in particular on vulnerable early life stages, is essential to improve our understanding of OA impacts on coral reef organisms and the important ecosystem services they support.
Effects of Elevated CO2 on Photosynthesis
Porolithon cf. onkodes recruits growing under high CO2 conditions showed significantly higher maximum gross photosynthetic rates at the thallus surface compared to those under ambient CO2 conditions, suggesting that photosynthesis in our experimental CCA was carbon limited under ambient CO2 conditions, in line with, e.g., measurements on epilithic algal layers on coral tiles (Larkum et al., 2003). The photosynthetic responses of coralline algae to elevated CO2 (reduced pH) documented in the literature are highly variable and depend on the nature of the experiments, the techniques employed to quantify O2 dynamics, and the species of algae considered in the experiments. For example, two studies using adult Porolithon onkodes from French Polynesia found no effect of elevated CO2 on gross photosynthesis and respiration (Johnson et al., 2014; Comeau et al., 2017), although in the Johnson et al. (2014) study, high CO2 decreased net photosynthesis, similar to results from the Great Barrier Reef using the same CCA species (Anthony et al., 2008). Studies using different taxa from temperate regions found gross photosynthesis to remain constant under elevated CO2 [e.g., articulated algae Corallina officinalis (Hofmann et al., 2012), Corallina elongata (Noisette et al., 2013), CCA Lithophyllum incrustans, Lithothamnion corallioides (Noisette et al., 2013), Lithothamnion glaciale (Kamenos et al., 2013)]. Enhanced productivity in response to elevated CO2 has been observed for some adult tropical corallines (Semesi et al., 2009; Yildiz et al., 2014). Using O2 microsensors on adult CCA, Hofmann et al. (2016) found elevated photosynthetic rates under reduced pH, similar to the findings in our study.
Enhanced photosynthesis in response to elevated CO2 concentrations may indicate that photosynthesis in early life stages of P. onkodes is limited by intracellular inorganic carbon availability. There are two main mechanisms by which algae acquire CO2 for carbon fixation within the cell: (1) the active uptake of HCO3- via a number of carbon concentrating mechanisms (CCMs), and (2) the diffusive uptake of CO2 (Giordano et al., 2005; Raven et al., 2005). These mechanisms could be altered by elevated seawater CO2 concentrations. Coralline algae have CCMs that facilitate active transport of HCO3- across cellular membranes leading to higher concentrations of CO2 at the site of RuBisCO (Comeau et al., 2013; Hofmann and Heesch, 2018) and given the high availability of HCO3- in seawater, enhanced CO2 may thus not significantly enhance DIC transport to the site of photosynthesis. However, since CCM activity is energetically costly, it is likely that elevated seawater CO2 alleviates the use of CCMs, effectively downregulating CCM activity upon enhanced and energetically less costly diffusive CO2 uptake under OA conditions. Flexibility in the use of carbon acquisition strategies under OA has been suggested for a number of articulated coralline algae including C. officinalis (Cornwall et al., 2012) and Arthrocardia corymbosa (Cornwall et al., 2013). Our study cannot discern the mechanistic basis for the increased rates of photosynthesis observed in our recruits under OA, but could suggest that photosynthesis in our early life stages of CCA is carbon limited. The majority of studies examining the effects of elevated CO2 on CCA photosynthesis have used incubation chambers with larger O2 sensors and have shown negative or no response of OA on photosynthetic activity (Anthony et al., 2008; Gao and Zheng, 2010; Hofmann et al., 2012; Cornwall et al., 2013; Martin et al., 2013; Noisette et al., 2013; Johnson et al., 2014; Comeau et al., 2017). However, when microsensors have been used to test CO2 effects on coralline algae photosynthesis, increased O2 production has been detected (e.g., Hofmann et al., 2016, but see Cornwall et al., 2013). Microsensors measure O2 dynamics directly at the surface of the CCA, with minimum interference from epiphytic algae (e.g., diatoms, Roleda et al., 2015) or bacteria, or endolithic algae (e.g., Anthony et al., 2008), presumably providing a more local and direct measurement of the effects of CO2 on CCA physiology.
Declined Growth and Relationship With Photosynthesis
Photosynthesis is the major source of carbon for CCA algal tissue construction, therefore, it is generally expected that increased photosynthetic rates and consequently the amount of fixed carbon would be positively correlated with algal growth. As detailed earlier, photosynthetic rates of the experimental recruits increased with increased CO2 concentration, however, this increase in CO2 did not translate directly in enhanced algal growth. On the contrary, both vertical and marginal growthrates of the algal crusts were depressed under high CO2 (a 28 and 19% decrease under high compared to ambient CO2, Figures 6A,B), and we additionally observed a significant inverse relationship between algal photosynthesis and growth (Pearson correlation R2 = 0.89, p = 0.01, Figure 7). The decreased growth of CCA under elevated CO2 shown in our study agrees with findings from several studies using both adult and early life stages of CCA (Kuffner et al., 2008; Bradassi et al., 2013; Ordoñez et al., 2017). The decoupling between increased photosynthetic rates and algal growth rates may be explained by two key processes. First, and perhaps the most important process, high CO2 enhances skeletal dissolution of the coralline algal crusts. In fact, SEM images showed distorted crust tissues, collapsed epithelial cells (Figure 2C), and apparently a thinning of calcified cell walls (Figure 2D), similar to observations on Lithothamnion glaciale from the Mediterranean (Ragazzola et al., 2012) and Hydrolithon samoense from Japan exposed to high CO2 (Kato et al., 2014). Several studies have attributed such reduction in growth of CCA recruits (e.g., Bradassi et al., 2013; Roleda et al., 2015) and adults (Diaz-Pulido et al., 2014) to increased skeletal dissolution due to undersaturation of seawater with respect to the dominant form of calcium carbonate in the CCA, i.e., high-magnesium-calcite. Table 1 shows a high Mg-calcite saturation state <1 in the high CO2 treatment, indicating undersaturation of seawater calcium carbonate and hence conditions for skeletal dissolution. Undersaturation may also affect deposition of new calcium carbonate in the algal skeleton. Secondly, since algal growth is a function of photosynthesis minus algal respiration and excretion of photosynthates, this suggest that carbon losses due to respiratory and excretion/secretion processes are higher than carbon gains from the photosynthetic process (Falkowski and Raven, 2013). In this regard, respiration rates were indeed higher under high CO2, which may explain the observed reduced growth, albeit net photosynthesis was also slightly higher under elevated CO2. It is also likely that a significant fraction of photosynthetically fixed carbon is excreted/secreted as dissolved organic carbon (DOC) to the external environment, leaving less carbon readily available for algal growth (e.g., Iñiguez et al., 2016, 2017) and potentially for calcification. This situation may be particularly important when nutrients limit algal growth, as shown in symbiotic algae (Yellowlees et al., 2008) and polar algae (Iñiguez et al., 2016). Recent work shows that high CO2 concentrations stimulated the release of DOC in a range of tropical reef algae, particularly red algae (Diaz-Pulido and Barron, personal observation). The mechanisms explaining the decoupling of algal photosynthesis and growth under OA conditions require further investigations.
Although our study did not directly quantify the calcification rate of the early life stage of P. cf. onkodes, we expect that the observed reduction in both vertical and marginal crust growth is associated with a lowering in the amount of calcium carbonate deposited by the algal crusts. On the other hand, a direct positive relationship between photosynthesis and calcification has also been postulated (Borowitzka, 1981; Hofmann et al., 2016), although decoupling between photosynthesis and calcification on adult coralline algae has also been shown (Semesi et al., 2009). Our finding of increased photosynthetic rate under elevated CO2 does not seem to be coupled with elevated rates of calcification, given the reduced growth observed when exposed to high CO2, and the considerable alterations in crust thickness and ultrastructure. Any positive effect of elevated DIC (both CO2 and HCO3-) on CCA photosynthesis and potentially on calcification may thus not be sufficient to compensate for the reduction in high-Mg-calcite saturation state and resulting skeletal dissolution, as discussed by Comeau et al. (2013) and Bradassi et al. (2013). The very thin crusts of the early life stages of P. cf. onkodes could contribute to some extent to the high vulnerability of these recruits to OA, thus CCA recruits may represent one of the most sensitive life stages to OA. A further understanding of the metabolic processes underpinning decreased growth and calcification is fundamental for understanding the underlying causes for decreased abundance in key reef building coralline algae.
Data Availability Statement
Data are available at the Australian Rivers Institute – Coast and Estuaries repository system at Griffith University.
Author Contributions
AO and GD-P designed the experiments, collected and analyzed the growth data, and drafted the manuscript. AO performed the experiments. DW and NL collected and analyzed the photosynthesis data. All authors contributed with manuscript writing and gave final approval for publication.
Funding
This project was supported by the Australian Research Council (DP-120101778), the Great Barrier Reef Foundation, a Sapere-Aude Advanced grant from the Independent Research Fund Denmark (MK), and the Carlsberg Foundation (DW). Samples for this study were collected under the permit G12/34877.1 granted by Great Barrier Reef Marine Park Authority.
Conflict of Interest Statement
The authors declare that the research was conducted in the absence of any commercial or financial relationships that could be construed as a potential conflict of interest.
Acknowledgments
We thank P. Gartrell, C. Barron, B. Lewis, L. Gomez, E. Kennedy, and HIRS staff who assisted with the experiments.
Footnotes
References
Adey, W. H. (1998). Coral reefs: algal structured and mediated ecosystems in shallow, turbulent, alkaline waters. J. Phycol. 34, 393–406. doi: 10.1046/j.1529-8817.1998.340393.x
Anthony, K. R. N., Kline, D. I., Diaz-Pulido, G., Dove, S., and Hoegh-Guldberg, O. (2008). Ocean acidification causes bleaching and productivity loss in coral reef builders. Proc. Natl. Acad. Sci. U.S.A. 105, 17442–17446. doi: 10.1073/pnas.0804478105
Borowitzka, M. A. (1981). Photosynthesis and calcification in the articulated coralline red algae Amphiroa anceps and A. foliacea. Mar. Biol. 62, 17–23. doi: 10.1007/BF00396947
Bradassi, F., Cumani, F., Bressan, G., and Dupont, S. (2013). Early reproductive stages in the crustose coralline alga Phymatolithon lenormandii are strongly affected by mild ocean acidification. Mar. Biol. 160, 2261–2269. doi: 10.1007/s00227-013-2260-2
Brodersen, K. E., Lichtenberg, M., Ralph, P. J., Kühl, M., and Wangpraseurt, D. (2014). Radiative energy budget reveals high photosynthetic efficiency in symbiont-bearing corals. J. Royal Soc. Interface 11:20130997. doi: 10.1098/rsif.2013.0997
Comeau, S., Carpenter, R. C., and Edmunds, P. J. (2013). Coral reef calcifiers buffer their response to ocean acidification using both bicarbonate and carbonate. Proc. R. Soc. B 280:1753. doi: 10.1098/rspb.2012.2374
Comeau, S., Carpenter, R. C., and Edmunds, P. J. (2017). Effects of pCO2 on photosynthesis and respiration of tropical scleractinian corals and calcified algae. ICES J. Mar. Sci. 74, 1092–1102. doi: 10.1093/icesjms/fsv267
Cornwall, C. E., Hepburn, C. D., McGraw, C. M., Currie, K. I., Pilditch, C. A., Hunter, K. A., et al. (2013). Diurnal fluctuations in seawater pH influence the response of a calcifying macroalga to ocean acidification. Proc. R. Soc. B 280:1772. doi: 10.1098/rspb.2013.2201
Cornwall, C. E., Hepburn, C. D., Pritchard, D., Currie, K. I., McGraw, C. M., Hunter, K. A., et al. (2012). Carbon-use strategies in macroalgae: differential responses to lowered pH and implications for ocean acidification. J. Phycol. 48, 137–144. doi: 10.1111/j.1529-8817.2011.01085.x
Cornwall, C. E., Revill, A. T., and Hurd, C. L. (2015). High prevalence of diffusive uptake of CO2 by macroalgae in a temperate subtidal ecosystem. Photosynth. Res. 124, 181–190. doi: 10.1007/s11120-015-0114-0
Dean, A. J., Steneck, R. S., Tager, D., and Pandolfi, J. M. (2015). Distribution, abundance and diversity of crustose coralline algae on the Great Barrier Reef. Coral Reefs 34, 581–594. doi: 10.1007/s00338-015-1263-5
Diaz-Pulido, G., Anthony, K. R. N., Kline, D. I., Dove, S., and Hoegh-Guldberg, O. (2012). Interactions between ocean acidification and warming on the mortality and dissolution of coralline algae. J. Phycol. 48, 32–39. doi: 10.1111/j.1529-8817.2011.01084.x
Diaz-Pulido, G., Cornwall, C., Gartrell, P., Hurd, C., and Tran, D. V. (2016). Strategies of dissolved inorganic carbon use in macroalgae across a gradient of terrestrial influence: implications for the Great Barrier Reef in the context of ocean acidification. Coral Reefs 35, 1327–1341. doi: 10.1007/s00338-016-1481-5
Diaz-Pulido, G., Gouezo, M., Tilbrook, B., Dove, S., and Anthony, K. R. N. (2011). High CO2 enhances the competitive strength of seaweeds over corals. Ecol. Lett. 14, 156–162. doi: 10.1111/j.1461-0248.2010.01565.x
Diaz-Pulido, G., Nash, M. C., Anthony, K. R. N., Bender, D., Opdyke, B. N., Reyes-Nivia, C., et al. (2014). Greenhouse conditions induce mineralogical changes and dolomite accumulation in coralline algae on tropical reefs. Nat. Commun. 5:3310. doi: 10.1038/ncomms4310
Fabricius, K. E., Kluibenschedl, A., Harrington, L., Noonan, S., and De’ath, G. (2015). In situ changes of tropical crustose coralline algae along carbon dioxide gradients. Sci. Rep. 5:9537. doi: 10.1038/srep09537
Falkowski, P. G., and Raven, J. A. (2013). Aquatic Photosynthesis. Princeton, NJ: Princeton University Press. doi: 10.1515/9781400849727
Feely, R. A., Sabine, C. L., Lee, K., Berelson, W., Kleypas, J., Fabry, V. J., et al. (2004). Impact of anthropogenic CO2 on the CaCO3 system in the oceans. Science 305, 362–366. doi: 10.1126/science.1097329
Gabrielson, P. W., Hughey, J. R., and Diaz-Pulido, G. (2018). Genomics reveals abundant speciation in the coral reef building alga Porolithon onkodes (Corallinales, Rhodophyta). J. Phycol. 54, 429–434. doi: 10.1111/jpy.12761
Gao, K., Aruga, Y., Asada, K., and Kiyohara, M. (1993). Influence of enhanced CO2 on growth and photosynthesis of the red algae Gracilaria sp. and G. chilensis. J. Appl. Phycol. 5, 563–571. doi: 10.1007/BF02184635
Gao, K., and Zheng, Y. (2010). Combined effects of ocean acidification and solar UV radiation on photosynthesis, growth, pigmentation and calcification of the coralline alga Corallina sessilis (Rhodophyta). Glob. Change Biol. 16, 2388–2398. doi: 10.1111/j.1365-2486.2009.02113.x
Giordano, M., Beardall, J., and Raven, J. A. (2005). CO2 concentrating mechanisms in algae: mechanisms, environmental modulation, and evolution. Annu. Rev. Plant Biol. 56, 99–131. doi: 10.1146/annurev.arplant.56.032604.144052
Guenther, R., Miklasz, K., Carrington, E., and Martone, P. T. (2018). Macroalgal spore dysfunction: ocean acidification delays and weakens adhesion. J. Phycol. 54, 153–158. doi: 10.1111/jpy.12614
Harrington, L., Fabricius, K., De’Ath, G., and Negri, A. (2004). Recognition and selection of settlement substrata determine post-settlement survival in corals. Ecology 85, 3428–3437. doi: 10.1890/04-0298
Heyward, A. J., and Negri, A. P. (1999). Natural inducers for coral larval metamorphosis. Coral Reefs 18, 273–279. doi: 10.1007/s003380050193
Hofmann, L. C., and Bischof, K. (2014). Ocean acidification effects on calcifying macroalgae. Aquat. Biol. 22, 261–279. doi: 10.3354/ab00581
Hofmann, L. C., and Heesch, S. (2018). Latitudinal trends in stable isotope signatures and carbon concentrating mechanisms of northeast Atlantic rhodoliths. Biogeosciences 15, 6139–6149. doi: 10.5194/bg-2017-399
Hofmann, L. C., Koch, M., and de Beer, D. (2016). Biotic control of surface pH and evidence of light-induced H+ pumping and Ca2+-H+ exchange in a tropical crustose coralline alga. PLoS One 11:e0159057. doi: 10.1371/journal.pone.0159057
Hofmann, L. C., Yildiz, G., Hanelt, D., and Bischof, K. (2012). Physiological responses of the calcifying rhodophyte, Corallina officinalis (L.), to future CO2 levels. Mar. Biol. 159, 783–792. doi: 10.1007/s00227-011-1854-9
Ichiki, S., Mizuta, H., and Yamamoto, H. (2000). Effects of irradiance, water temperature and nutrients on the growth of sporelings of the crustose coralline alga Lithophyllum yessoense Foslie (Corallinales, Rhodophyceae). Phycol. Res. 48, 115–120. doi: 10.1046/j.1440-1835.2000.00193.x
Iñiguez, C., Carmona, R., Lorenzo, M. R., Niell, F. X., Wiencke, C., and Gordillo, F. J. L. (2016). Increased CO2 modifies the carbon balance and the photosynthetic yield of two common Arctic brown seaweeds: Desmarestia aculeata and Alaria esculenta. Polar Biol. 39, 1979–1991. doi: 10.1007/s00300-015-1724-x
Iñiguez, C., Heinrich, S., Harms, L., and Gordillo, F. J. L. (2017). Increased temperature and CO2 alleviate photoinhibition in Desmarestia anceps: from transcriptomics to carbon utilization. J. Exp. Bot. 68, 3971–3984. doi: 10.1093/jxb/erx164
IPCC (2014). “Climate change 2013: the physical science basis,” in Contribution of Working Group I to the Fifth Assessment Report of the Intergovernmental Panel on Climate Change, eds T.F. Stocker, D. Qin, G.-K. Plattner, M. Tignor, S. K. Allen, J. Boschung et al. (Cambridge: Cambridge University Press).
Johnson, M. D., and Carpenter, R. C. (2012). Ocean acidification and warming decrease calcification in the crustose coralline alga Hydrolithon onkodes and increase susceptibility to grazing. J. Exp. Mar. Biol. Ecol. 43, 94–101. doi: 10.1016/j.jembe.2012.08.005
Johnson, M. D., Moriarty, V. W., and Carpenter, R. C. (2014). Acclimatization of the crustose coralline alga Porolithon onkodes to variable pCO2. PLoS One 9:e87678. doi: 10.1371/journal.pone.0087678
Jones, W. E., and Moorjani, A. S. (1973). Attachment and Early Development of the Tetraspores of Some Coralline Red Algae. Cochin: MBAI Special Publication dedicated to Dr. N K Panikkar 1, 293–304.
Kamenos, N. A., Burdett, H. L., Aloisio, E., Findlay, H. S., Martin, S., Longbone, C., et al. (2013). Coralline algal structure is more sensitive to rate, rather than the magnitude, of ocean acidification. Glob. Change Biol. 19, 3621–3628. doi: 10.1111/gcb.12351
Kato, A., Hikami, M., Kumagai, N. H., Suzuki, A., Nojiri, Y., and Sakai, K. (2014). Negative effects of ocean acidification on two crustose coralline species using genetically homogeneous samples. Mar. Environ. Res. 94, 1–6. doi: 10.1016/j.marenvres.2013.10.010
Koch, M., Bowes, G., Cliff, R., and Xing-Hai, Z. (2013). Climate change and ocean acidification effects on seagrasses and marine macroalgae. Glob. Change Biol. 19, 103–132. doi: 10.1111/j.1365-2486.2012.02791.x
Kroeker, K. J., Kordas, R. L., Crim, R., Hendriks, I. E., Ramajo, L., Singh, G. S., et al. (2013). Impacts of ocean acidification on marine organisms: quantifying sensitivities and interaction with warming. Glob. Change Biol. 19, 1884–1896. doi: 10.1111/gcb.12179
Kroeker, K. J., Kordas, R. L., Crim, R. N., and Singh, G. G. (2010). Meta-analysis reveals negative yet variable effects of ocean acidification on marine organisms. Ecol. Lett. 13, 1419–1434. doi: 10.1111/j.1461-0248.2010.01518.x
Kuffner, I. B., Andersson, A. J., Jokiel, P. L., Rodgers, K. U. S., and Mackenzie, F. T. (2008). Decreased abundance of crustose coralline algae due to ocean acidification. Nat. Geosci. 1, 114–117. doi: 10.1038/ngeo100
Larkum, A. W. D., Koch, E.-M. W., and Kühl, M. (2003). Diffusive boundary layers and photosynthesis of the epilithic algal community of coral reefs. Mar. Biol. 142, 1073–1082. doi: 10.1007/s00227-003-1022-y
Lewis, B., Kennedy, E. V., and Diaz-Pulido, G. (2017). Seasonal growth and calcification of a reef-building crustose coralline alga on the Great Barrier Reef. Mar. Ecol. Prog. Ser. 568, 73–86. doi: 10.3354/meps12074
Littler, M. M., and Doty, M. S. (1975). Ecological components structuring the seaward edges of tropical pacific reefs: the distribution, communities and productivity of Porolithon. J. Ecol. 63, 117–129. doi: 10.2307/2258846
Martin, S., Cohu, S., Vignot, C., Zimmerman, G., and Gattuso, J. P. (2013). One-year experiment on the physiological response of the Mediterranean crustose coralline alga, Lithophyllum cabiochae, to elevated pCO2 and temperature. Ecol. Evol. 3, 676–693. doi: 10.1002/ece3.475
Martin, S., and Gattuso, J. P. (2009). Response of Mediterranean coralline algae to ocean acidification and elevated temperature. Glob. Change Biol. 15, 2089–2100. doi: 10.1111/j.1365-2486.2009.01874.x
Morse, J. W., Arvidson, R. S., and Lüttge, A. (2007). Calcium carbonate formation and dissolution. Chem. Rev. 107, 342–381. doi: 10.1021/cr050358j
Noisette, F., Egilsdottir, H., Davoult, D., and Martin, S. (2013). Physiological responses of three temperate coralline algae from contrasting habitats to near-future ocean acidification. J. Exp. Mar. Biol. Ecol. 448, 179–187. doi: 10.1016/j.jembe.2013.07.006
Ordoñez, A., Doropoulos, C., and Diaz-Pulido, G. (2014). Effects of ocean acidification on population dynamics and community structure of crustose coralline algae. Biol. Bull. 226, 255–268. doi: 10.1086/BBLv226n3p255
Ordoñez, A., Kennedy, E. V., and Diaz-Pulido, G. (2017). Reduced spore germination explains sensitivity of reef-building algae to climate change stressors. PLoS One 12:e0189122. doi: 10.1371/journal.pone.0189122
Pierrot, D., Lewis, E., and Wallace, D. W. R. (2006). MS Excel Program Developed for CO2 System Calculations. ORNL/CDIAC-105a. Oak Ridge, TN: Carbon Dioxide Information Analysis Center. doi: 10.3334/CDIAC/otg.CO2SYS_XLS_CDIAC105a
Ragazzola, F., Foster, L. C., Form, A., Anderson, P. S. L., Hansteen, T. H., and Fietzke, J. (2012). Ocean acidification weakens the structural integrity of coralline algae. Glob. Change Biol. 18, 2804–2812. doi: 10.1111/j.1365-2486.2012.02756.x
Raven, J. A., Ball, L. A., Beardall, J., Giordano, M., and Maberly, S. C. (2005). Algae lacking carbon-concentrating mechanisms. Can. J. Bot. 83, 879–890. doi: 10.1139/b05-074
Revsbech, N. P. (1989). An oxygen microsensor with a guard cathode. Limnol. Oceanogr. 34, 474–478. doi: 10.4319/lo.1989.34.2.0474
Revsbech, N. P., and Jorgensen, B. B. (1983). Photosynthesis of benthic microflora measured with high spatial resolution by the oxygen microprofile method: capabilities and limitations of the method. Limnol. Oceanogr. 28, 749–756. doi: 10.4319/lo.1983.28.4.0749
Ringeltaube, P., and Harvey, A. (2005). Non-geniculate coralline algae (Corallinales, Rhodophyta) on heron reef, Great Barrier Reef (Australia). Bot. Mar. 43, 409–498.
Roberts, R. D., Kühl, M., Glud, R. N., and Rysgaard, S. (2002). Primary production of crustose coralline red algae in a high artic fjord. J. Phycol. 38, 273–283. doi: 10.1046/j.1529-8817.2002.01104.x
Roleda, M. Y., Cornwall, C. E., Feng, Y., McGraw, C. M., Smith, A. M., and Hurd, C. L. (2015). Effect of ocean acidification and pH fluctuations on the growth and development of coralline algal recruits, and an associated benthic algal assemblage. PLoS One 10:e0140394. doi: 10.1371/journal.pone.0140394
Roleda, M. Y., Morris, J. N., McGraw, C. M., and Hurd, C. L. (2012). Ocean acidification and seaweed reproduction: increased CO2 ameliorates the negative effect of lowered pH on meiospore germination in the giant kelp Macrocystis pyrifera (Laminariales. Phaeophyceae). Glob. Change Biol. 18, 854–864. doi: 10.1111/j.1365-2486.2011.02594.x
Santelices, B. (1990). Patterns of reproduction dispersdal and recruitment in seaweeds. Oceanogr. Mar. Biol. Annu. Rev. 28, 177–276.
Semesi, I. S., Kangwe, J., and Björk, M. (2009). Alterations in seawater pH and CO2 affect calcification and photosynthesis in the tropical coralline alga, Hydrolithon sp. (Rhodophyta). Estuar. Coast. Shelf Sci. 84, 337–341. doi: 10.1016/j.ecss.2009.03.038
Steneck, R. S. (1983). Escalating herbivory and resulting adaptive trends in calcareous algal crusts. Paleobiology 9, 44–61. doi: 10.2307/2400629
Webb, W. L., Newton, M., and Starr, D. (1974). Carbon dioxide exchange of Alnus rubra. Oecologia 17, 281–291. doi: 10.1007/bf00345747
Yellowlees, D., Rees, T. A. V., and Leggat, W. (2008). Metabolic interactions between algal symbionts and invertebrate hosts. Plant Cell Environ. 31, 679–694. doi: 10.1111/j.1365-3040.2008.01802.x
Keywords: ocean acidification, global warming, early stages, red algae, algal physiology, photosynthesis, respiration
Citation: Ordoñez A, Wangpraseurt D, Lyndby NH, Kühl M and Diaz-Pulido G (2019) Elevated CO2 Leads to Enhanced Photosynthesis but Decreased Growth in Early Life Stages of Reef Building Coralline Algae. Front. Mar. Sci. 5:495. doi: 10.3389/fmars.2018.00495
Received: 29 August 2018; Accepted: 11 December 2018;
Published: 07 January 2019.
Edited by:
Laurie Carol Hofmann, Max Planck Institute for Marine Microbiology (MPG), GermanyReviewed by:
Maggy Nugues, Université de Sciences Lettres de Paris, FranceRaphael Ritson-Williams, California Academy of Sciences, United States
Copyright © 2019 Ordoñez, Wangpraseurt, Lyndby, Kühl and Diaz-Pulido. This is an open-access article distributed under the terms of the Creative Commons Attribution License (CC BY). The use, distribution or reproduction in other forums is permitted, provided the original author(s) and the copyright owner(s) are credited and that the original publication in this journal is cited, in accordance with accepted academic practice. No use, distribution or reproduction is permitted which does not comply with these terms.
*Correspondence: Guillermo Diaz-Pulido, Zy5kaWF6LXB1bGlkb0BncmlmZml0aC5lZHUuYXU=