- 1Departamento de Biotecnología Marina, Centro de Investigación Científica y de Educación Superior de Ensenada, Ensenada, Mexico
- 2Departamento de Oceanografía Biológica, Centro de Investigación Científica y de Educación Superior de Ensenada, Ensenada, Mexico
- 3Centro de Investigacións Mariñas, Vilanova de Arousa, Spain
- 4Centro de Investigación Científica de Yucatán, Unidad de Ciencias del Agua, Cancún, Mexico
- 5Alfred-Wegener-Institut, Helmholtz Zentrum für Polar-und Meeresforschung, Helmholtz-Gemeinschaft Deutscher Forschungszentren, Bremerhaven, Germany
Spirolides are polyether cyclic imines considered as “fast acting toxins.” Long-term human health consequences of spirolide ingestion are uncertain, and hence regulatory limits for human consumption have not been established. Nevertheless, monitoring these toxins in shellfish is essential because they can interfere with detection by mouse bioassay of lipophilic regulated toxins. Todos Santos Bay (TSB), in the northwest of the Baja California Peninsula, is an important shellfish cultivation and fish-farming area in Mexico. The toxin analog 13-desmethyl spirolide C has been reported in cultivated mussels (Mytilus galloprovincialis) from TSB, but the causative species associated with accumulation of this toxin has not been previously identified. We assessed the occurrence of Alexandrium ostenfeldii, the unique known producer of spirolides, by inverted light microscopy and by PCR with species-specific oligonucleotides designed for the ITS and 18S rDNA. We determined the presence and abundance of this species at the surface and at the thermocline from samples collected over two annual sampling periods (2013–2014 and 2016–2017). During the 2013–2014 period, A. ostenfeldii was found in 50% of the samples analyzed by light microscopy. The highest cell abundance (about 3.6 × 103 cells L-1) occurred in October 2013. During 2016–2017 the dinoflagellate was present in low cell abundances (<5 × 102 cells L-1) and was detected in only 20.9% of the samples. Cells of this species were usually found when sea surface temperature ranged from 17 to 20∘C. We also evaluated spirolide accumulated in cultivated mussels from TSB by tandem mass spectrometry (LC-MS/MS). The only spirolide detected was 13-desmethyl spirolide C, found mainly during the 2013–2014 sampling period, with the highest concentration (1.05 μg kg-1) in June 2014. During winter, toxin concentration was at or below the detection limit. During 2016–2017, spirolides were below the detection limit, coinciding with the absence of the causative species. Cell abundance of A. ostenfeldii and spirolide concentration in mussels did not present a clear correlation. This study represents the first record of A. ostenfeldii in TSB and provides evidence that this species is the primary origin of spirolides accumulated in mussels.
Introduction
Spirolides are naturally occurring polyether cyclic imine compounds with a spiro-group attached to the tricyclic ethers. These toxins were first isolated from mussel digestive glands and toxicity was determined by intraperitoneal mouse bioassay during chemical investigations of polar bioactive molecules from microalgae and shellfish from Nova Scotia (Canada) (Hu et al., 1995). The intact cyclic imine moiety is the pharmacophore that confers biological activity (Hu et al., 1996, 2001). Many spirolides, including those belonging to the A, B, C, D groups and their respective desmethyl derivatives, act as “fast-acting toxins” (FAT) characterized by rapid onset of neurological symptoms followed by death after intraperitoneal injection into mice (Cembella et al., 1999; Richard et al., 2001). Spirolides are not considered in the sanitary legislation for human consumption of shellfish since a toxic effect in humans has not been proven and no poisoning symptoms associated with these toxins have been confirmed (Otero et al., 2011). A few complaints of gastric distress and tachycardia were recorded after consumption of mussels containing high spirolide concentrations, but these non-specific symptoms could not be definitively ascribed to spirolides (Richard et al., 2001). Cholinergic receptors (muscarinic and nicotinic) are proposed main targets for spirolides, and the toxic effects could be their activity as irreversible antagonists of the muscarinic acetylcholine receptor (Gill et al., 2003) and weak L-type transmembrane calcium channel activators (Sleno et al., 2004).
Spirolides are coextracted in the methanolic extract of the regulated polyether shellfish toxins, such as okadaic acid, dinophysistoxins, azaspiracids, and yessotoxins. The FAT activity of spirolides in methanolic or lipophilic fractions can therefore interfere with and cause artifacts in the intraperitoneal mouse bioassay (Trainer et al., 2013). False toxicity positives associated with spirolides can cause unnecessary shellfish sanitary closures that will negatively affect economic activities for coastal aquaculture and harvest of wild shellfish.
The causative species associated with spirolide accumulation in cultured shellfish from Nova Scotia was identified as the mixotrophic dinoflagellate Alexandrium ostenfeldii (Paulsen) Balech and Tangen (Cembella A. et al., 2000). This species is widely distributed in coastal waters around the globe (Cembella, 2018), from the coast of Washington State, United States (Balech, 1995), the northeastern coast of North America (Hargraves and Maranda, 2002), and including north Atlantic and sub-Arctic waters, as well as the Mediterranean Sea and the brackish Baltic Sea (Suikkanen et al., 2013). Interest in the study of A. ostenfeldii and associated toxicity has increased due to the reports of coastal blooms of this species in the Peruvian coast (Sánchez et al., 2004), Narragansett Bay and the New River Estuary along the United States east coast (Borkman et al., 2012), and the Baltic Sea coast of Finland (Hakanen et al., 2012). Blooms of this species occurred recently in Oosterschelde, Netherlands, representing a major concern for public health for poisonings associated with paralytic shellfish toxins (PSTs), but these blooms were not related to human intoxication by spirolides (Van de Waal et al., 2015). The toxin regulatory situation is complicated by the fact that certain A. ostenfeldii populations can also produce other neurotoxins, such as PSTs, and/or the cyclic imines known as gymnodimines. The toxin composition and content of A. ostenfeldii depends upon genotypic strain differences and geographic origin (Suikkanen et al., 2013), and is therefore difficult to predict and assess.
The Pacific coast of North America from Alaska to Baja California is relatively underrepresented in studies of the biogeographical distribution of A. ostenfeldii and associated spirolides (Cembella, 2018). Todos Santos Bay (TSB), in the northwest of the Baja California peninsula, is the region with the highest production of cultivated mussels in Mexico (Maeda Martínez, 2008). Sanitary closures have been implemented in the region due to the presence of lipophilic toxins in shellfish harvested from this bay. Sánchez-Bravo (2013) demonstrated a high percentage of non-specificity in the detection of toxicity with the lipophilic mouse bioassay in comparison with measurements of toxin composition determined by liquid chromatography coupled with tandem mass spectrometry (LC-MS/MS) in mussel samples from TSB that could be attributed to the presence of spirolides among other “fast acting” toxins. Particularly in 2012, 13-desmethyl spirolide C was detected during almost the whole year with no apparent temporal pattern of appearance (García-Mendoza et al., 2014). Unfortunately, the causative species associated with this spirolide accumulation in shellfish was not identified.
The inability to reliably identify the toxigenic species that produces spirolides is often associated with the complexity in the identification of Alexandrium taxa (Touzet et al., 2008), especially by morphological criteria alone. A. ostenfeldii has been detected and identified by polymerase chain reaction (PCR) probes involving specific primers to amplify the Internal Transcribed Spacer (ITS) of the rDNA gene (Schwarz, 2011). This represents a useful technique to unequivocally evaluate the presence of this species in the phytoplankton community. In the present work, we evaluated the temporal distribution of A. ostenfeldii in TSB as causative agent for the accumulation of spirolides in cultivated bivalve molluscs from the bay. The presence and temporal abundance of this species in the phytoplankton community was evaluated by PCR and by morphological examination of cells from water samples by light microscopy, and combined with analysis of accumulated spirolides in cultivated mussels (Mytilus galloprovincialis). The combination of these alternative techniques allowed the detection and confirmation of A. ostenfeldii in TSB and provided the first report of this species in the northwest Pacific of Mexico.
Materials and Methods
Field Sampling
Todos Santos Bay, located on the northwestern coast of the Baja California peninsula, Mexico, is subject to a regular monitoring program for marine biotoxins in shellfish and associated toxigenic plankton by the FICOTOX laboratory of Centro de Investigación Científica y de Educación Superior de Ensenada, Baja California (CICESE). From July 2013 to June 2014 water and mussel samples were collected periodically from the mussel cultivation area in TSB (St 13; Figure 1). Water samples for taxonomic analysis and enumeration were collected at the surface and at 10 m depth with a 2 L Niskin bottle. In addition, plankton samples were analyzed from vertical net tows with 20 μm mesh plankton net conducted through the upper 20 m of the water column. In 2016, the regular monitoring program of FICOTOX was extended to include evaluation of the phytoplankton community where caged tuna aquaculture is located in the region. From 2016, plankton samples were analyzed from Salsipuedes Bay, where some tuna enclosures are located (St 1, 3, 4 and 6; Figure 1). Sampling at St 13 continued throughout this period and two more stations (St 11 and 12; Figure 1) in the southern sector of TSB were also monitored on a monthly basis from August 2016 to August 2017. Water samples were collected with a 2 L Niskin bottle at the surface and at the thermocline. The vertical temperature profile of the water column was measured with a conductivity, temperature and pressure profiler (CTD, CastAway, YSI, Yellow Springs, OH, United States). The depth of the thermocline was determined from the temperature profile before the samples were collected.
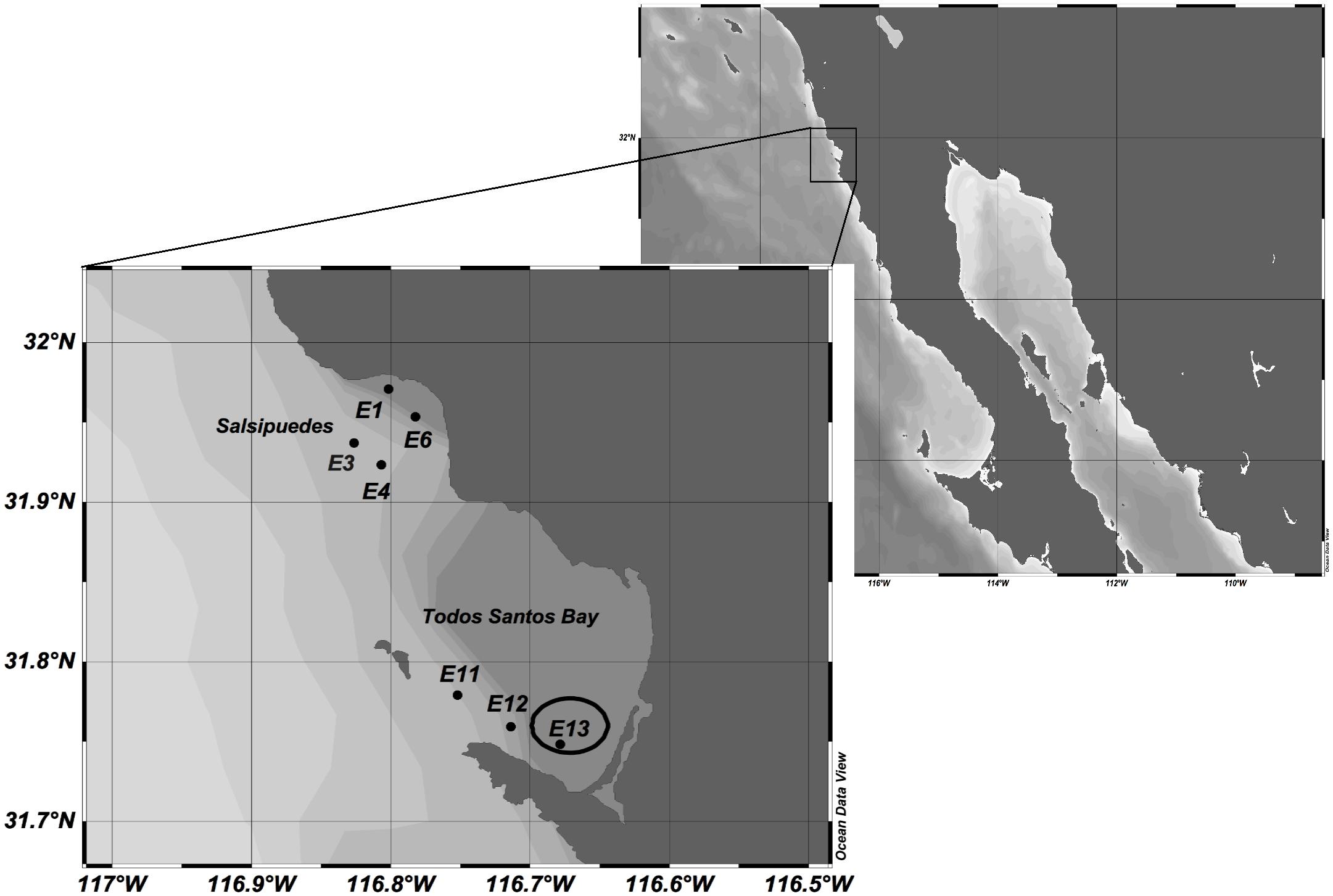
Figure 1. Sampling stations located in Todos Santos Bay (TSB) region. Station 13 enclosed in the circle is inside the mussel cultivation area.
Microscopic Evaluation of A. ostenfeldii Abundance
A 250 mL water subsample from the Niskin bottle was fixed with Lugol’s iodine-acetate solution. These samples were analyzed with an inverted microscope (Leica DM13000B, Leica Microsystems, Wetzlar, Germany) according to the Utermöhl method (Lund et al., 1958). A 50 mL sample was sedimented and the complete surface of the sedimentation chamber was analyzed to quantify the cell abundance of A. ostenfeldii. For net samples only 10 mL were sedimented and the results were presented as the relative abundance (in %) of A. ostenfeldii to total phytoplankton cells collected with the 20 μm plankton net.
The thecal plate arrangement and morphology of cells considered provisionally as A. ostenfeldii was evaluated by epifluorescence microscopy. Fixed cells from seawater samples were separated by a capillary, and rinsed twice with deionized water to get rid of salts and residual Lugol’s solution. Then, 4 μL of Fluorescent Brightener 28 (Sigma-Aldrich, St. Louis, MO, United States) at a concentration of 1 mg mL-1 was added to stain the cellulose thecal plates (Fritz and Triemer, 1985). Plate arrangement was characterized under an Axio Imager A2 microscope system and digitally photographed with an AxioCam ICc 1 camera and Axiovision software (Carl Zeiss AG, Oberkochen, Germany).
Molecular Identification of A. ostenfeldii
DNA Extraction
Seawater (500 mL to 1 L) was filtered through 8 μm pore-size polycarbonate filters (Nucleopore, Whatman, Darmstadt, Germany), under gentle vacuum to avoid cell disruption. Filters were stored in a 50 mL centrifuge tubes at -80°C until analysis. The DNA was extracted with a DNeasy Plant Mini kit (Qiagen, Hilden, Germany) according to the manufacturer’s instructions but with modification of the volume of the lysis buffer to 600 μL. Homogenization of cells was performed with a mini-Bead Beater 24 (BioSpec Products, Bartlesville, OK, United States) at maximum speed (6.5 m s-1) for 45 s (Wohlrab et al., 2010), with 170 μm3 of zirconium beads of 0.1 mm diameter to disrupt the cells. The DNA was resuspended in sterile Milli-Q water. The purity and concentration of DNA was measured by UV-spectroscopy with a NanoDrop 2000 (Thermo Scientific, Wilmington, DE, United States) and the integrity of the DNA was verified on a 1.2% agarose gel via electrophoresis.
Polymerase Chain Reaction (PCR) Amplification
The primers AOF4 (5′-TGTCAATGCGTGTGCATTCG-3′) and AOR3 (5′-CATTGCAACCAATGCACATGA-3′) were used for identification of A. ostenfeldii during the first monitoring period (2013–2014). These primers produce an amplicon of 99 bp of the ITS1, 5.8S and ITS2 region (Schwarz, 2011). The primers were originally designed specifically to differentiate A. ostenfeldii from A. peruvianum (4 bp), but A. peruvianum is now recognized as a heterotypic synonym of the former species (Kremp et al., 2014). For identification of A. ostenfeldii in the 2016–2017 samples the primers AOST213F (5′-GGGAAGGGTTGTGGTCATTAGTTA-3′) and AOST213R (5′-TTGCCATGTAATTCATTCAGTCATT-3′), which produce an amplicon of 89 bp of the 18S region were used (Elferink et al., 2017).
PCR reactions (30 μL) were performed in a Veriti thermal cycler (Applied Biosystems, United States) using 100 ng of DNA. For ITS amplifications, the master mix was prepared with the following concentrations: 0.4 mM of each dNTP, 0.12 μM of each primer, 4 mM of MgCl2, 1 X GoTaq® Flexi buffer (Promega, Madison, WI, United States), and 1.25 U of GoTaq® DNA polymerase. The PCR was carried out at 94°C for 4 min, followed by 40 cycles of 94°C for 30 s, 60°C for 45 s, 72°C for 1 min, and a final extension step of 72°C for 7 min.
For the 18S rDNA amplifications the master mix contained the following concentrations: 0.2 mM of each dNTP, 0.30 μM of each primer, 3 mM of MgCl2, 1 X GoTaq® Flexi buffer (Promega, Madison, WI, United States), and 0.6 U of GoTaq® DNA polymerase. The amplification was carried out under the following conditions: 94°C for 3 min, followed by 30 cycles of 94°C for 30 s, 60°C for 45 s, 72°C for 1 min and a final extension step of 72°C for 7 min. The amplicons were analyzed by electrophoresis on 2% agarose gel.
The specificity of the reaction was confirmed with a DNA template from a monoclonal reference culture of A. ostenfeldii. Strain CCMP 1773 was acquired from the Provasoli Guillard Center for Marine Microalgae (CCMP, Boothbay Harbor, ME, United States) and cultivated under optimal growth conditions at 15°C and photon flux density of 100 μmol quanta m2 s-1. The DNA of this strain was used as a positive control. For the negative control the DNA was replaced with ultrapure water in the PCR reaction.
Sequencing
PCR products of ITS and 18S amplifications were sent for sequence analysis to SeqXcel, San Diego, CA, United States. The analysis of the sequences was carried out using the BLAST tool on the NCBI website and the sequence alignments were performed using the CLUSTAL X software (Thompson et al., 1997) and the Biological sequence alignment editor and analysis program (Bioedit) (Hall, 1999).
Compositional Analysis and Quantification of Spirolides
Spirolides were determined from particulate plankton by filtration of 300–800 mL seawater from the surface and thermocline through Nucleopore polycarbonate filters (3.0 μm pore-size; Whatman, Darmstadt, Germany) under low vacuum. Filters were stored in 50 mL centrifuge tubes at -70°C until extraction. During extraction, filters were repeatedly rinsed with 1 mL 100% MeOH until complete discoloration of the filters. The methanolic extract was filtered through spin-filters (0.45 μm pore-size; Millipore Ultrafree, Eschborn, Germany) and centrifuged for 30 s at 800 ×g. The filtrate was transferred to an autosampler vial and 5 μL were injected into the LC-MS/MS system. The protocol for the detection of spirolides was as described by Krock et al. (2008) for lipophilic toxins. Toxin analyses were performed on a liquid chromatograph (Agilent 1100, Waldbronn, Germany) coupled to triple-quadrupole mass spectrometer (ABI-SCIEX-4000 Q Trap, Applied Biosystems, Darmstadt, Germany) equipped with a Turbo V ion source. Separation of the lipophilic toxins was performed by reverse-phase chromatography on a C8 column (50 mm × 2 mm) packed with 3 μm Hypersil BDS 120 Å (Phenomenex, Aschaffenburg, Germany). The mobile phase comprised two eluents, where eluent A was water and eluent B was acetonitrile-water (95:5 v:v), both containing 2.0 mmol L-1 ammonium formate and 50 mmol L-1 formic acid. The limit of detection for SPX-1 (13-desmethyl spirolide C) was 0.3 ng mL-1, determined with reference to an external standard solution of 10 ng mL-1 SPX-1 obtained from the CRMP, IMB, National Research Council, Halifax, NS, Canada.
Spirolides in mussels from the cultivation area at St 13 were determined from whole mussel samples provided by Acuacultura Oceanica Co., Ensenada, BC, Mexico. Evaluation of lipophilic toxins in mussels was performed according to the standardized procedure of the European Union for the determination of marine lipophilic biotoxins in molluscs (European Union Reference Laboratory for Marine Biotoxins [EURLMB], 2015). Complete soft tissues of at least 20 commercial-size mussels were homogenized for the determination of phycotoxins. Toxin extraction was performed with 100% methanol on 2.0 ± 0.01 g of shellfish tissue (European Union Reference Laboratory for Marine Biotoxins [EURLMB], 2015). The detection and quantification of lipophilic phycotoxins was performed with the analytical methodology of liquid chromatography coupled to a tandem mass spectrometer (LC-MS/MS). A triple quadrupole Thermo Scientific TSQ Quantum Access Max LC-MS/MS system (San Jose, CA, United States) was used for analysis of mussel samples collected from July 2013 to June 2014, according to the method of Martín-Morales et al. (2013). After extraction, an aliquot of the methanolic extract was filtered through a 0.22-μm-syringe filter and 5 μL were injected into the LC-MS/MS system. The mass spectrometer was operated in positive-ion mode by multiple reaction monitoring (MRM). The Regueiro et al. (2011) method was employed for chromatographic separation with minor modifications for use of a shorter column. Chromatography was performed in reverse-phase mode with a Gemini-NX C18 (Phenomenex, Torrance, CA, United States) (50 × 2 mm; 3 μm) analytical column. The mobile phase was described by Gerssen et al. (2009) to yield alkaline chromatographic conditions; phase A = ammonium hydroxide 6.7 mM, phase B = acetonitrile in ammonium hydroxide 6.7 mM in proportion 9:1 (v:v). A calibration curve of four concentration levels of a certified solution obtained from CIFGA (Lugo, Spain) was used to quantify 13-desmethyl spirolide C. The limit of detection of the method was 0.04 ng mL-1.
For the mussel samples from 2016 to 2017, toxin analyses were performed by the method of Krock et al. (2008), as described above for analysis of spirolides in plankton samples. An aliquot of methanolic extract of mussel tissue was transferred to a spin-filter (pore-size 0.45 μm; Millipore Ultrafree, Eschborn, Germany) and centrifuged for 30 s at 800 ×g. The filtered sample was transferred to an autosampler vial and 5 μL were injected into the LC-MS/MS system.
Results
Occurrence of A. ostenfeldii in Todos Santos Bay
Light microscopic analysis of the phytoplankton community in 134 water samples collected from August 2016 to August 2017 revealed that A. ostenfeldii occurred in 20.9% of the samples. The species was detected in the same number of samples from the surface and the thermocline, indicating that A. ostenfeldii was present throughout the mixed layer. Nevertheless, the cell abundance was higher at the surface than at the thermocline. There was no apparent seasonality for the species presence, and the low cell abundances did not allow for definition of a clear pattern. The only discernable trend was the low cell abundance during the winter (November–February), when cell densities were consistently low or below the detection limit (Figures 2A,B).
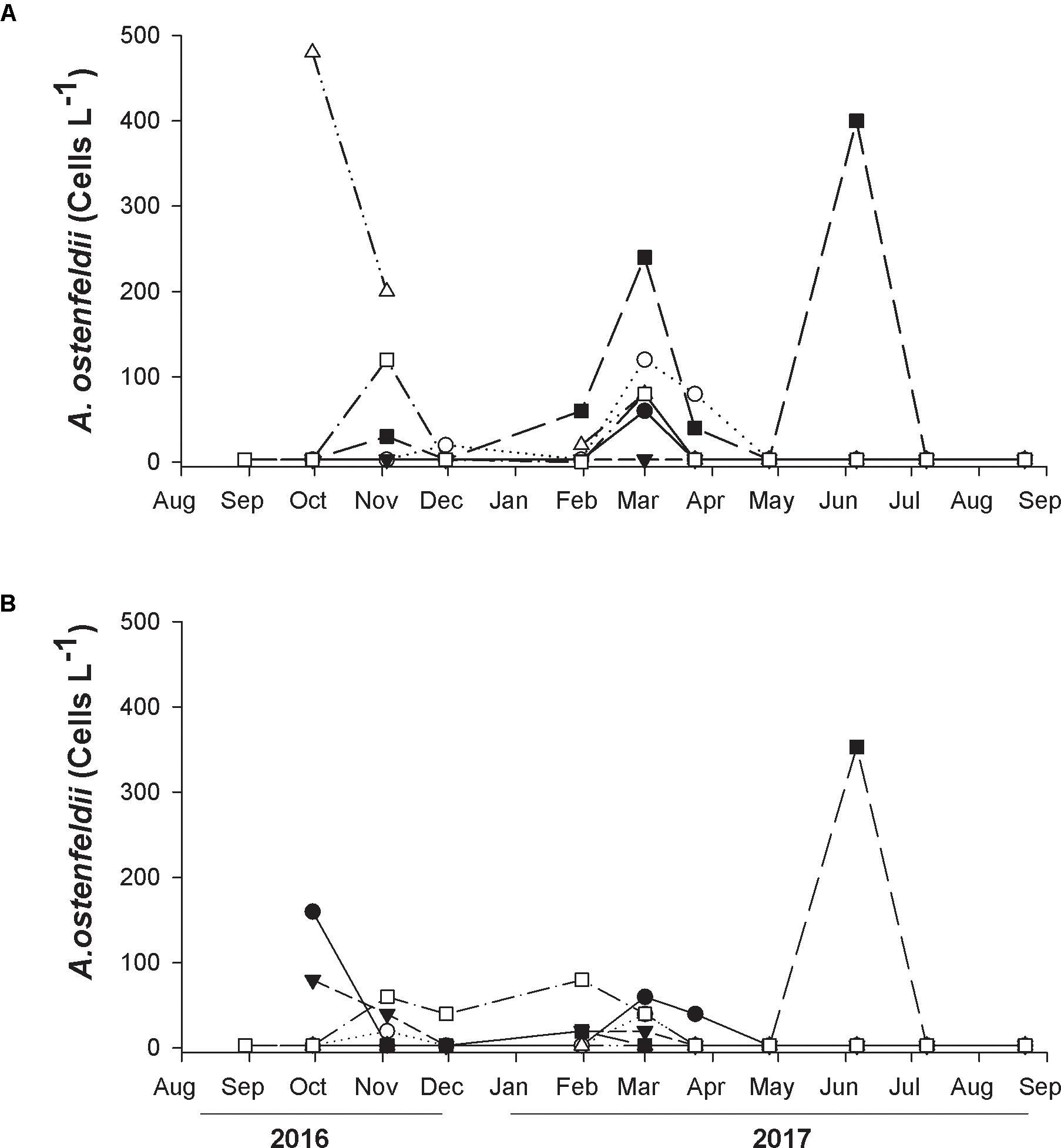
Figure 2. Temporal variation of cell abundance of A. ostenfeldii at seven sampling stations located in Todos Santos Bay region: Station 1 (closed circles), Station 3 (open circles), Station 4 (closed triangles), Station 6 (open triangles), Station 11 (closed square), and Station 12 (open square). Samples were collected at surface (A) and at the thermocline (B) from August 2016 to August 2017.
At the surface A. ostenfeldii was detected at all stations except St 4, but at low abundance throughout the sampling period (Figure 2A). The maximum abundance of 480 cells L-1 was registered at St 6 (Salsipuedes) at the end of September 2017 (Figure 2A). A relatively high abundance of 400 cells L-1 in June 2017 and 240 cells L-1 at the beginning of March, 2017 were also recorded from St 11 (Rincón de Ballenas). The species was not observed at the thermocline from St 6 and at the other stations the abundance was low (20–80 cells L-1) or absent (Figure 2B). The highest abundance of A. ostenfeldii in the thermocline was 353 cells L-1 detected at St 11 in June (Figure 2B). An abundance of 160 cells L-1 was found at the end of September from the same station.
Morphological Characterization of A. ostenfeldii
The cells of A. ostenfeldii presented a globose appearance with slightly rounded margins (Figures 3A,G). The theca is very thin and not easily detected with light microscopy (Figure 3H). The specimens from TSB were typically observed as solitary cells varying in size from 25 to 35 μm in diameter, in some water samples both vegetative cells and cysts were found (Figure 3I). The cingulum is equatorial and slightly excavated with no presence of cingular lists (Figures 3B,C). The hypotheca and epitheca have the same height. The tabulation of the cells is typical of A. ostenfeldii showing an insert-type tabulation characterized by the contact of the plate 1’ with 1”, 6”’, 2’, 4’ and the apical pore complex APC (Figures 3B,C). This complex is completely occupied by a big comma-shaped pore (Figure 3B). The sixth precingular plate (6”) is wider than tall. The 1’ plate is elongated and asymmetric, with a prominent ventral pore in the inflection (Figure 3C). The sulcal plate series is clearly distinguished. The key plate for describing the sulcus is S.p. This plate contacts with s.d.p, s.sp, 5”’, 4”’, 2”” and 1””’ (Figures 3D–F).
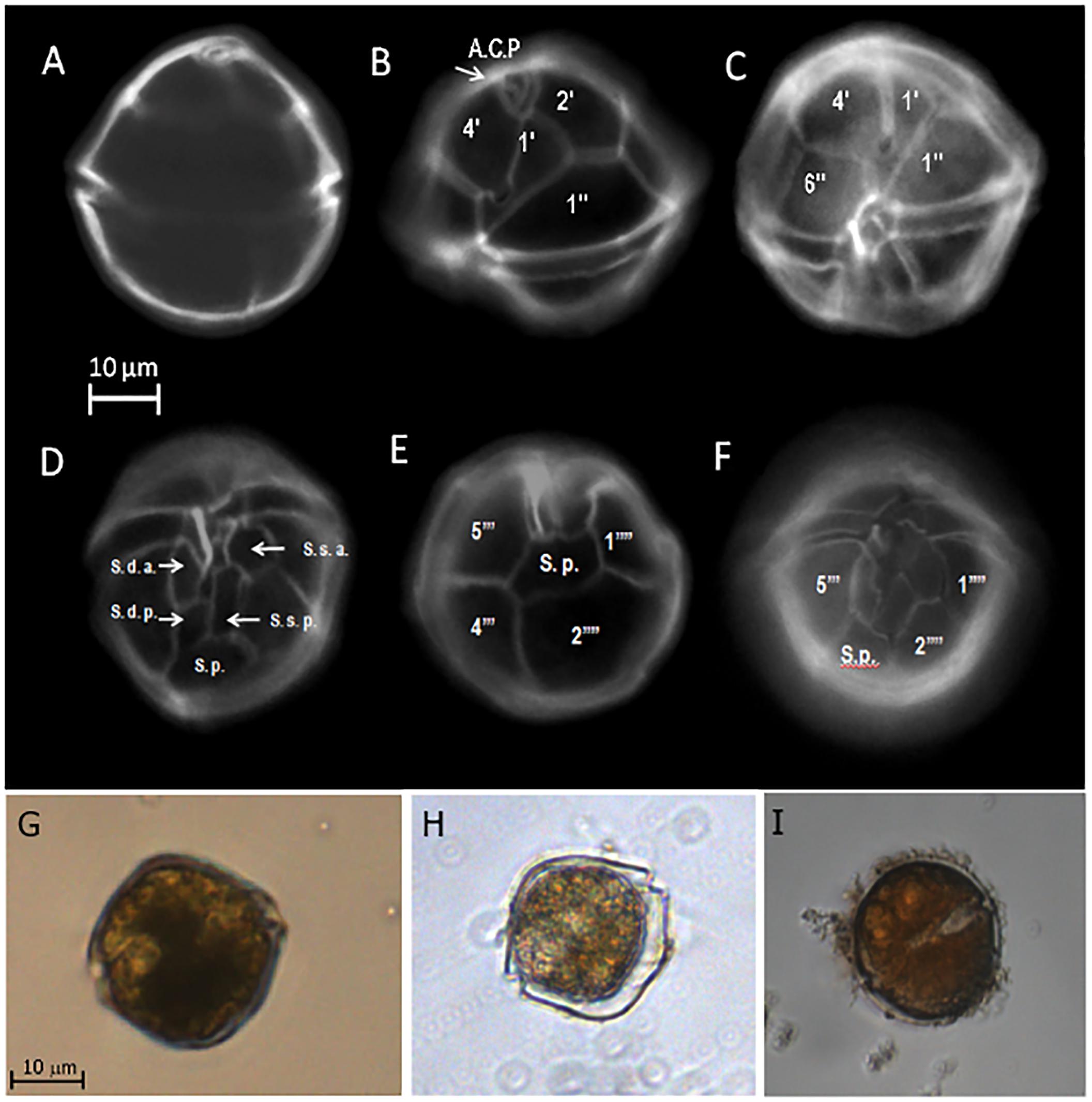
Figure 3. Morphological characteristics of Alexandrium ostenfeldii collected in water samples from Todos Santos Bay region. The cells were stained with calcofluor to evaluate the thecal plate arrangement with epifluorescence microscopy. (A) Shape of the cells; (B) apical pore complex (APC) complex occupied by a prominent comma-shaped pore; (C) first precingular (1’) plate with a ventral pore; (D) ventral view showing the sulcal plates; (E) antapical view showing the sulcal posterior plate; (F) ventral view; (G,H) vegetative cells observed by inverted optical microscopy; (I) presence of cysts in the water samples.
Molecular Identification of A. ostenfeldii
Molecular identification of A. ostenfeldii was conducted on 57 samples analyzed by PCR from the south of TSB (Rincón de Ballenas, St 13) where mussel farms are located. With the AOF4 and AOR3 primers, the 99 bp ITS fragment was detected in 52.6% of the samples collected from July 2013 to June 2014, confirming the presence of A. ostenfeldii in the study area. As expected, given the higher plankton biomass collected among the three alternative samples (at surface, from 10 m depth and vertical net haul) the highest number of positive results (68.4%) were obtained from net haul samples, Figure 4 shows an example of the PCR results. The expected size amplicon was detected in all three fractions of samples from April 8, 2014 collected at surface, from 10 m and by net haul (Figure 4), suggesting that this species was present throughout the mixed layer of the water column. In contrast, the absence of an amplicon in the samples from April 22, 2014 indicates that A. ostenfeldii was not present on this date (Figure 4).
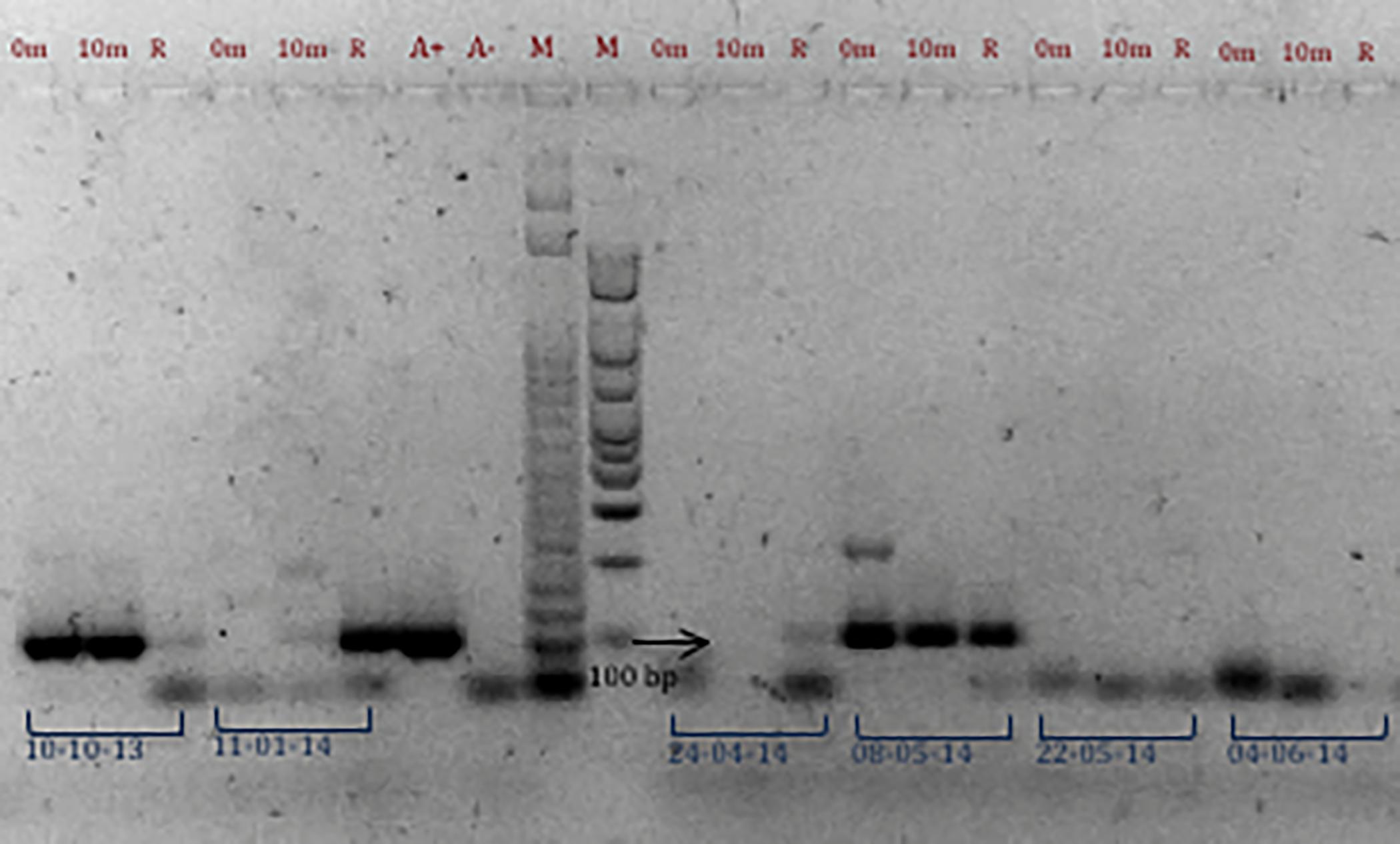
Figure 4. PCR amplification of samples from Station 13 using AOF4 and AOR3 primers. The 99 bp ITS fragment indicates the presences of A. ostenfeldii. Results of samples collected at different dates are presented (October 10, 2013; November 1, 2014; April 24, 2014; May 8 and 22, 2014, and June 4, 2014). Samples were collected at 0 and 10 m discrete depth in the water column and by vertical phytoplankton net tows (R). A+: positive PCR control and A–: negative PCR control. M: molecular marker of 50 and 100 bp bioline.
The samples collected from August 2016 to August 2017 at seven stations and analyzed by PCR with the alternative primers AOST213F and AOST213R also confirmed the presence of A. ostenfeldii within the TSB region. The 18S amplification fragment of 89 bp was detected in 23.7% of 156 samples processed. For example, in samples of March 1, 2017, the expected amplification fragment was observed in samples from all stations, except in the surface sample from St 4, St 6 at the thermocline, and from St 13 at both surface and thermocline (Figure 5).
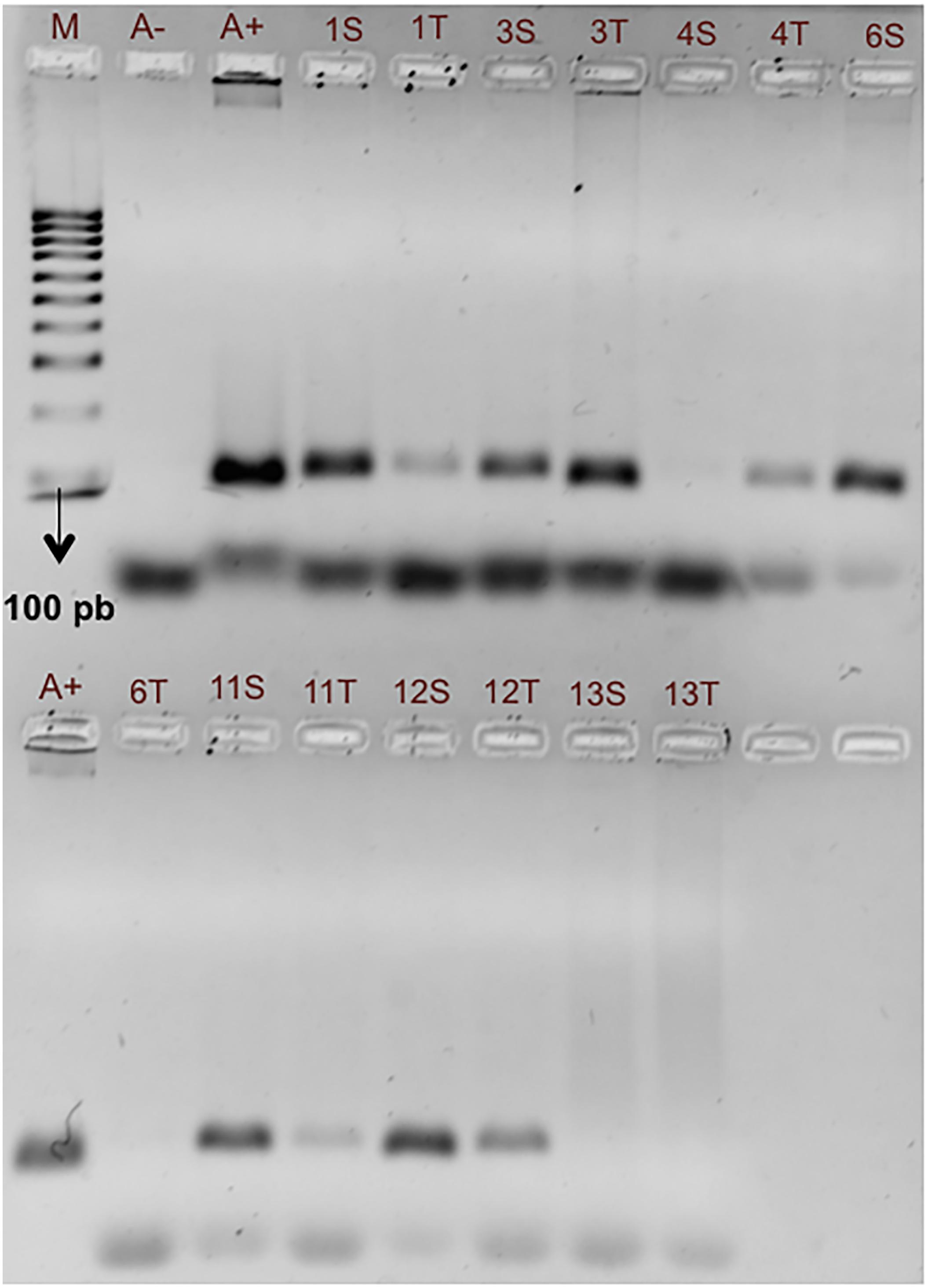
Figure 5. PCR amplification of samples from seven different stations in Todos Santos Bay region using AOST123F and AOST123R primers. The 89 bp fragment indicates the presence of A. ostenfeldii. Results of samples collected on March 1, 2017 are presented. The samples were collected at surface (S) and thermocline (T) in the water column. A+: positive PCR control and A–: negative PCR control. M: molecular marker of 100 bp bioline.
A. ostenfeldii was detected in 70.5% of the 213 samples from the two sampling periods analyzed by PCR and light microscopy. The PCR method yielded a higher positive detection (by 26.7%) compared to light microscopy. In contrast, PCR results were negative for only 1.88% of the samples in which A. ostenfeldii was registered by light microscopy.
PCR products were sequenced with the different primers for specific dates. For example, the alignments and blast analysis conducted with the 99 bp ITS fragment sequence from the sample of August 8, 2013 (St 13) showed 98% identity with A. ostenfeldii sequences found in the NCBI GenBank (JX841278.1).
Spirolide Detection in Plankton and Mussels
The presence of spirolide was confirmed in mussel samples collected at Rincón de Ballenas (St 13), but the analog 13-desmethyl spirolide C was the only analog detected by LC-MS/MS in mussels. The chromatogram presented in Figure 6 shows the quantitative detection of 13-desmethyl spirolide C in a mussel sample of September, 2014. The retention time (RT) (4.25 min) of the 164 m/z ion that coincided with the RT of the 692.501>444.30 m/z transition used as the confirmatory ion for 13-desmethyl spirolide C. The chromatogram of analogs 13-19 didesmethyl spirolide C and the spirolide B are also presented in Figure 6, but these analogs were not detected in mussels; the corresponding signals for these analogs were absent or below the detection limit and/or the retention times did not align with those of the expected transitions.
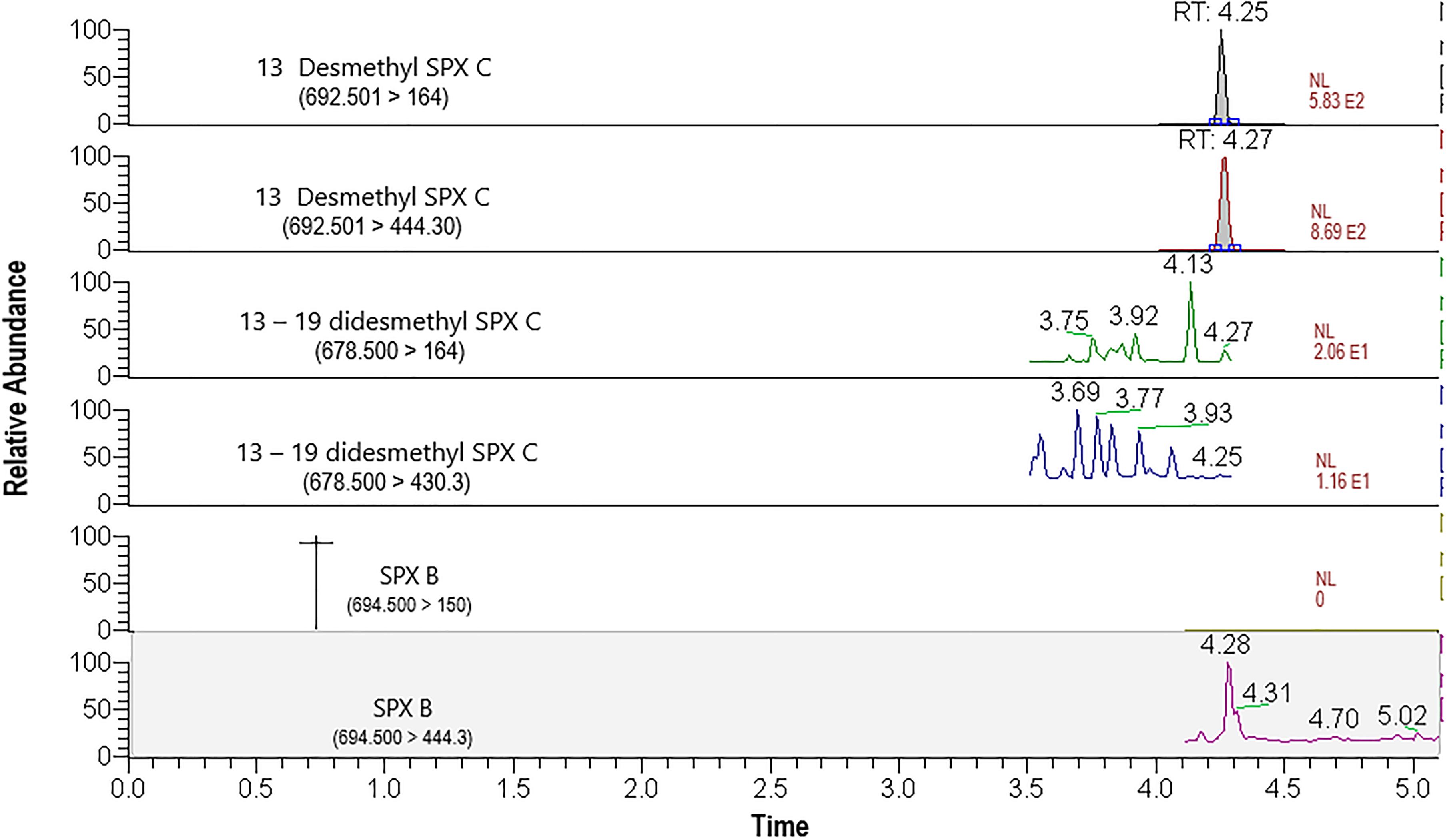
Figure 6. LC-MS/MS chromatograms (in MRM mode) of 13-desmethyl spirolide (SPX) C transitions (692.501>164 m/z and 692.501>444.30 m/z). Mass transitions of 13-19-didesmethyl spirolide (SPX) C and spirolide (SPX) B are also shown but were not detected in this sample of Mediterranean mussels (M. galloprovincialis) from TSB collected on September 12, 2014.
The cell abundances of A. ostenfeldii detected in Rincón de Ballenas area (St 13) at the surface and at 10 m or the thermocline depth were compared with the spirolide accumulation in mussels (Figure 7). A. ostenfeldii was detected in 50% of the samples from 2013 to 2014. The species was heterogeneously distributed from surface to 10 m depth since the detection and abundances coincided in samples from both depths. There was no clear seasonality pattern of occurrence for A. ostenfeldii or for the spirolides in mussels. The cell abundance of A. ostenfeldii varied throughout the sampling period of 2013–2014 (from 0 to 3.6 × 103 cells L-1). Nevertheless, these variations are not reflected in the concentration of 13-desmethyl spirolide C in mussels, which varied from below the detection limit (0.04 μg kg-1) and remained <1.05 μg kg-1 throughout the sampling period (2013–2014) (Figure 7). The maximum A. ostenfeldii cell abundance was found in October 2013 (3.62 × 103 and 3.59 × 103 cells L-1 in surface waters and at 10 m depth, respectively); the concentration of 13-desmethyl spirolide C was 0.54 μg kg-1. From August 2016 to August 2017, A. ostenfeldii was absent from all samples, except for a sample obtained at the end of August 2016 (280 cells L-1) at the thermocline. According to the LC-MS/MS results, the concentration of 13-desmethyl spirolide C remained below the detection limit in all of the samples. During the sampling period from 2016 to 2017, no spirolides were detected in the 154 samples of plankton particulate matter.
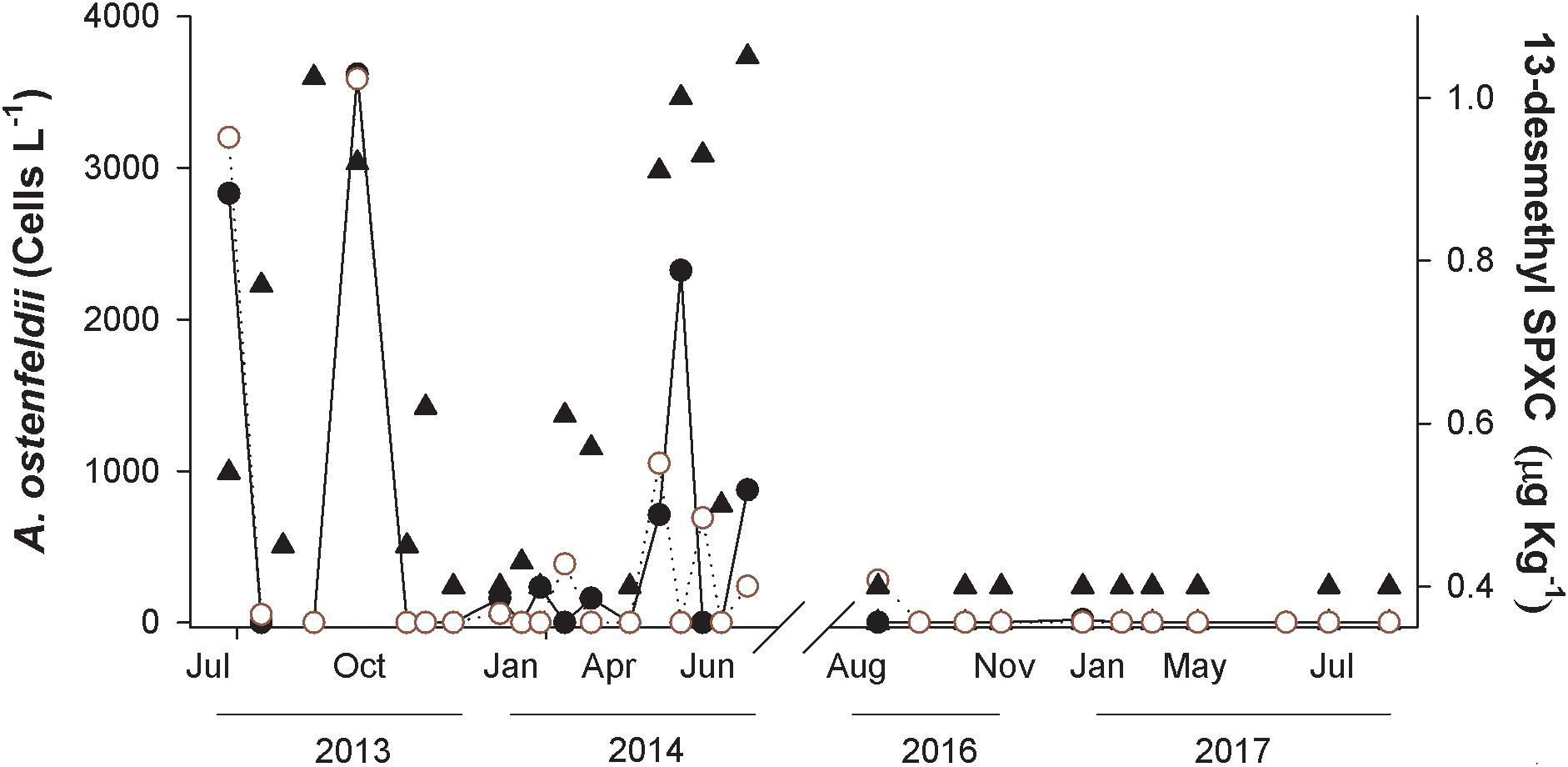
Figure 7. Concentration of 13-desmethyl spirolide (SPX) C (closed triangles) in the Mediterranean mussel Mytilus galloprovincialis collected from July 2013 to June 2014 and from August 2016 to August 2017 in Rincón de Ballenas (Todos Santos Bay). The abundance of A. ostenfeldii cells at surface (closed circles) and at 10 m or thermocline depth (open circles) is also shown.
Certain populations of A. ostenfeldii are capable of producing PSP toxins (saxitoxin and derivatives) in addition to or instead of spirolides (Cembella, 2018). As a confirmation, for three samples (from July 8, 2013; August 22, 2013; October 10, 2013) where the cell abundance of A. ostenfeldii was high, but the concentration of spirolides in mussels was low, analysis of PSP toxins was carried out on mussels samples. No PSP toxins were detected in these samples.
Water Temperature Associated With the Presence of A. ostenfeldii
In an attempt to establish trends of A. ostenfeldii cell abundance in relation to water temperature, water temperatures at surface, 5 m and 10 m depth at St 13 are presented together with A. ostenfeldii cell abundance during July 2013 to June 2014 and from August 2016 to August 2017 (Rincón de Ballenas) (Figure 8). The highest cell abundance of A. ostenfeldii was associated with temperatures between 17 and 20°C (Figure 8). During 2013–2014, maximum surface temperature of 22.1°C was registered in September 2013. The water column was stratified as evidenced by a decrease of 5°C from surface waters at 5 m (17°C) and 8°C at 10 m depth (14°C). Under these conditions, A. ostenfeldii was not detected. Abundance of A. ostenfeldii was <500 cells L-1 during the winter period with lower water temperature (November to April 2013–2014). Strong water stratification was present during the period 2016–2017, with a decrease of 8.5°C from the surface to 10 m depth. During this period, a higher maximum surface temperature of 22.9°C was detected in August 2016 and August 2017. The species was not detected during this period (Figure 8). When the water column was homogeneous with respect to temperature and during periods of lower water temperature between November, 2013 and April, 2014 cell abundance was reduced or remained below detection.
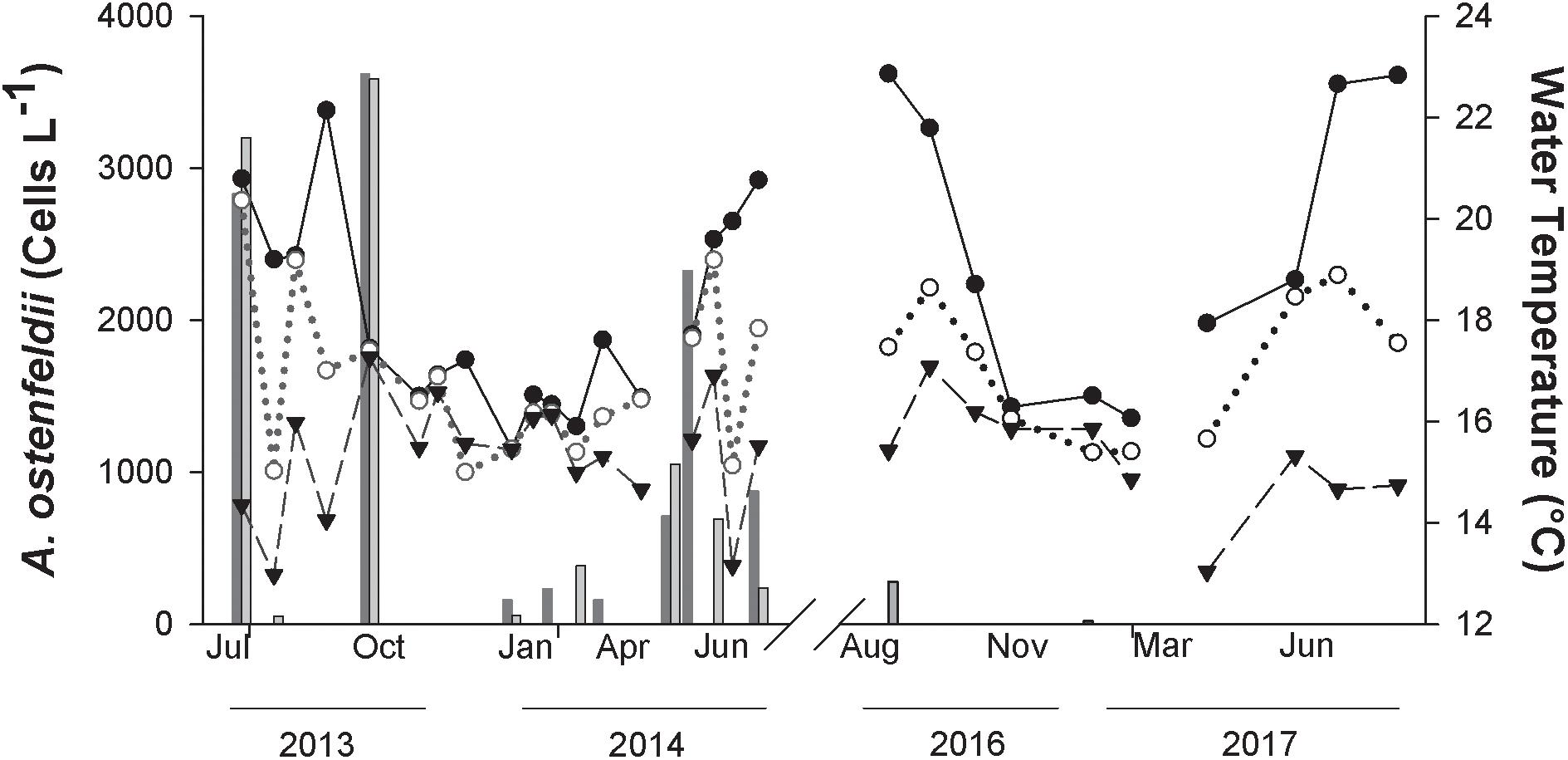
Figure 8. Temperature at surface (closed circles), 5 m (open circles) and 10 m depth (closed triangles) at Station 13 (Rincón de Ballenas) in Todos Santos Bay. The cell abundance of A. ostenfeldii in samples at surface (dark gray bar) and 10 m or thermocline (light gray bar) is also presented.
Discussion
Dynamics of A. ostenfeldii in Todos Santos Bay
The dinoflagellate A. ostenfeldii was found in TSB with variable abundances and as a common component of the phytoplankton community, but this species did not exhibit a clear seasonal occurrence pattern during the sampling period. The maximum cell density (3 × 103 cells L-1) registered at St 13 during late summer to fall 2013 was typical for this species from coastal North Atlantic waters and Scandinavian fjords. In such low water temperature and moderate salinity environments the species tends not to form high biomass blooms, but rather is commonly found as a non-dominant component of the phytoplankton community at low maximal cell abundances ranging typically from 102 to 103 cells L-1 (Balech and Tangen, 1985; Moestrup and Hansen, 1988; Gribble et al., 2005). For example, A. ostenfeldii in coastal waters of Nova Scotia tends to form sub-surface aggregations at relatively low cell abundances of less than 4 × 103 cells L-1 (Cembella et al., 2001). In Casco Bay, along the Gulf of Maine, United States, a maximum abundance of 244 cells L-1 was registered during June 2001 (Gribble et al., 2005). These low abundances contrast with the high bloom concentrations reported from certain locations in northern Europe, typically characterized by a low salinity regime. Maximum cell abundances as high as 2.1 × 105 cells L-1 have been recorded from the coast of Finland in the northern Baltic Sea (Hakanen et al., 2012) and in the virtually freshwater Ouwerkerkse Creek, Netherlands, an extreme bloom of A. ostenfeldii yielded maximal cell densities of 4.5 × 106 cells L-1 (Van de Waal et al., 2015). In any case, the association of extreme blooms of A. ostenfeldii with low salinity environments is clearly not applicable to seasonal dynamics of this species in TSB. In comparison with habitats of A. ostenfeldii in the North Atlantic, TSB represents an environment characterized by higher seasonal surface water temperatures but only slightly higher salinity, which does not vary dramatically on an annual basis.
Temperature is one of the most important environmental variables that determine the biogeography of phytoplankton species (Butterwick et al., 2005), and which has a direct physiological influence and consequences on metabolic rates and hence growth rate (Anderson, 1998). This suggests a possible regulatory role of temperature in determining the temporal distribution of A. ostenfeldii in TSB. In spite of the lack of a definitive seasonal cell abundance pattern, and absence of a clear relationship between the presence of A. ostenfeldii and the ambient temperature, the species was apparently not present in the water column during the winter. The cell abundance of A. ostenfeldii in TSB decreased from August 2016 to August 2017 to the comparable period in 2013–2014. This indicates that the temporal cell distribution and abundance may be related to prior temperature anomalies. Under this scenario, a low cell abundance of A. ostenfeldii could be related to shifts in the phytoplankton community associated with the abnormally warm water conditions in the region, that initiated 3 years before 2016, associated with el Niño events, with temperature anomalies above 3°C (García-Mendoza et al., 2018). These anomalies were accompanied by low phytoplankton biomass. In the California Current System status report these changes are also described for the phytoplankton community, for which the productivity and cell concentrations of diatoms were below average for the southern California coast during 2016 (McClatchie et al., 2016). Jacox et al. (2018) reported that the water temperature anomalies caused changes in the structure and composition of the phytoplankton community, perhaps disadvantageous to A. ostenfeldii, while favoring an increase in cell abundances of species better adapted to these conditions. These warm water conditions recorded in TSB in the last few years have caused blooms of other genera such Cochlodinium and Chattonella that had been previously registered only in low densities in the bay.
Alexandrium ostenfeldii is often considered a “cold-water” species, but “blooms” (or at least maximal cell abundance) consistently occur during the warm period of the annual cycle in the respective environments. In TSB, two peaks of high cell abundance were detected in October, 2013 and May, 2014, when the surface water temperature was 17°C, but also when surface water was around 20°C. This suggests an optimal temperature window of 17–20°C for A. ostenfeldii in TSB. By comparison, massive blooms of A. ostenfeldii in the northern Baltic Sea occurred at water temperatures close to 20°C (Hakanen et al., 2012). In Narragansett Bay, Gulf of Maine, United States, maximum cell abundances were found within a temperature range from 14.0 to 17.0°C and the species (cited as synonym A. peruvianum) was not detected from the water column during autumn when the temperatures were between 13 and 17°C (Borkman et al., 2012). In contrast, in the Beagle Channel, Argentina the species was recorded at lower temperatures (7.5–10°C) during austral spring (Almandoz et al., 2014). The fact that A. ostenfeldii is globally distributed (Cembella, 2018) indicates that temperature-adapted variants are common and that comparisons of temperature tolerance windows among geographically disjunct populations are not strictly valid.
Due to the increasing global temperature the surface ocean is anticipated to become more stratified (Stocker et al., 2013). Garneau et al. (2011) recorded the maximum cell abundance for A. catenella in summer–autumn along the coast of Oregon and northern California, during the dry season (reduced freshwater runoff) with weak upwelling and low nutrients in surface waters. Stratification of the water column forming a superficial stable layer may favor the growth of Alexandrium over other phytoplankton species during low nutrient conditions in this stratified layer (Horner et al., 1997). Under such circumstances, the influence of the ambient water temperature on the temporal and spatial distribution of A. ostenfeldii may also be indirect, via contribution to stratification of the water column. More stratification of the water column should favor growth and persistence of species such as A. ostenfeldii, a facultatively mixotrophic dinoflagellate with vertical migration capabilities. This interpretation is consistent with the evidence that cells were absent from the water column at TSB during winter (the 2013–2014 monitoring period) and remained <500 cells L-1 during 2016–2017, when the water column was well mixed and homogeneous. Yet a simple association of A. ostenfeldii with high stratification is not supported by the data on the apparent lack of cells in the upper water column during August 2016, during a period of high surface water temperature (22.9°C) and strong stratification.
Nutrients are known to influence the dynamics of phytoplankton communities in rivers, estuaries and coastal zones (Anderson et al., 2002). In the same way, inorganic macronutrients could directly influence growth of A. ostenfeldii but there were no massive blooms recorded during the sampling periods in TBS. Furthermore, the high mixotrophic potential of this species renders it unlikely that inorganic macronutrient concentrations were directly correlated with or driving cell growth or biomass yield in TSB. This suggestion agrees with both Collos et al. (2007) and Brandenburg et al. (2017) who reported that proliferation of cells of Alexandrium species were independent of the inorganic nutrient concentrations because of mixotrophic feeding of the species. During a bloom in the Baltic Sea, the abundance of A. ostenfeldii cells was related with warm water, but was not significantly correlated with dissolved inorganic nutrient concentrations, however, nutrient concentration were related to the high concentration of resting cyts in the sediment (Hakanen et al., 2012).
Species Detection and Identification
The presence of A. ostenfeldii in TSB was independently confirmed by both light microscopy and positive PCR assays applied to 213 field samples during the two multi-annual periods. There were significant discrepancies between the results of the alternative methods, e.g., the molecular approach yielded 27.6% higher positive detection of the species than microscopy. By comparison, positive registration of toxic dinoflagellate species in the Mediterranean Sea by microscopy did not coincide with detection of the species by PCR. These differences were especially evident with members of the genus Alexandrium, and varied among species; the PCR method gave 38% higher detection for A. catenella, 36% for A. minutum and 20% for undefined Alexandrium species (Penna et al., 2007).
These discrepancies are related to differences in cell detection limits for the respective methods. The nominal detection limit of the Utermöhl method is 20 cells L-1 in a sedimentation volume of 50 mL when the whole chamber is counted (Edler and Elbrächter, 2010). In comparison, with a reported PCR amplification with 1 pg of genomic DNA for Alexandrium (Penna et al., 2007), and an estimated 115 pg per cell nuclear DNA content for A. ostenfeldii (Kremp et al., 2009), it is then theoretically possible to detect a single cell of this species in a field sample by PCR technique. Therefore, the possibility of detecting species that are poorly represented in the phytoplankton community, like A. ostenfeldii, increased significantly due the use of the PCR technique.
In a few cases (1.88%), A. ostenfeldii cells were identified from TSB samples by microscopy but were not detected by PCR. This may be attributable to misidentification of Alexandrium taxa due to the difficulty of morphological discrimination among closely related species within this genus (Touzet et al., 2009; Cembella, 2018). In TSB, A. ostenfeldii may be present in different life history (vegetative cells, gametes, zygotes) and growth stages of variable morphology. Furthermore, other species of Alexandrium genus, such as A. catenella (Peña-Manjarrez et al., 2005), may be present in TSB, and could be misidentified as A. ostenfeldii in the absence of critical taxonomic analysis.
Accumulation of Spirolides in Mussels
The dinoflagellate A. ostenfeldii was likely responsible for the accumulation of spirolides in mussels collected in the TSB region during 2012 (García-Mendoza et al., 2014). Among the diverse structural sub-types and known analogs of spirolides, only 13-desmethyl spirolide C was detected in cultivated mussels from TSB. This indicates that A. ostenfeldii populations in the region produce primarily or exclusively this spirolide analog and not other toxins such as PSTs associated with certain A ostenfeldii strains from global locations. In a phylogenetic study comparing multiple A. ostenfeldii strains from widely biogeographically distributed populations, six groups were defined that cluster genetically and according to toxin profile (Kremp et al., 2014). Group 1 strains produce both 13-desmethyl spirolide C and PSTs (Van Wagoner et al., 2011). In contrast, Group 2 members produce mostly or exclusively 13-desmethyl spirolide C (Franco et al., 2006; Touzet et al., 2008). The strain present in TSB therefore seems to belong to this latter group. Populations of A. ostenfeldii Group 2 have been registered mainly from eastern Canada, northeast United States, Spain and Irish coasts (Cembella A. D. et al., 2000; MacKinnon et al., 2004; Percy et al., 2004). However, it is necessary to isolate local strains to characterize their toxin profile and to confirm their phylogenetic relation with other populations of A. ostenfeldii. In addition, it is necessary to evaluate the toxin content of vegetative cells and cyts since this might influence the concentrations of spirolides in mussels.
From 2013 to 2014 13-desmethyl spirolide C was detected in 78.9% of the samples analyzed. There was no apparent seasonality in the presence of this toxin, as typified by the time-series data from 2012 when 13-desmethyl spirolide C was detected in most (80%) of the mussel samples analyzed and was present all year long without a clear pattern of appearance (García-Mendoza et al., 2014). In contrast, during the period from 2016 to 2017, 13-desmethyl spirolide C was below the detection limit and this was related with the absence of the causative species.
Concentrations of 13-desmethyl spirolide C in this study were lower than the concentrations recorded in shellfish from other regions. In Catalonia, NW Mediterranean Sea, 2–16 μg kg-1 of 13-desmethyl spirolide C was detected in mussels and oysters (García-Altarez et al., 2014). In shellfish samples from the Chinese coast the highest concentration of 13-desmethyl spirolide C was 8.96 μg kg-1 (Wu et al., 2015) and in Norwegian blue mussels the concentration of this toxin reached 226 μg kg-1 (Rundberger et al., 2011). The concentration of this spirolide previously reported in mussels from TSB was higher during the year 2012 (3 μg kg-1) (García-Mendoza et al., 2014) than recorded in this present study (1.05 μg kg-1).
In July, 2013 among the highest abundance for the species was recorded (2.8 and 3.2 × 103 cells L-1), but the concentration of the 13-desmethyl spirolide C was low (0.54 μg kg-1). The toxin cell quota is variable and dependent upon environmental conditions; the physiological state of the cell and the growth phase influence the cellular concentration of the toxin (Boczar et al., 1998; Anderson et al., 2012). This variability could explain the fact that even though one of the highest abundance of A. ostenfeldii was recorded and presence of the species was confirmed by PCR, the concentration of 13-desmethyl spirolide C was near the detection limit. Environmental conditions during this sampling period could influence the spirolide production in A. ostenfeldii present in the bay, and this could explain why there was not a clear correlation between the abundance of the species and the accumulation of spirolide in mussels.
At the end of August, 2013 and middle of September, 2013 A. ostenfeldii was not detected in the water samples, but, nevertheless, one of the highest concentration of 13-desmethyl spirolide C in this study (1.02 μg kg-1) was recorded from mussels. Jensen and Moestrup (1997) have proposed that the wide variation in cell concentrations of this species can also be due to rapid shifts between pelagic and benthic stages in shallow waters. Furthermore, A. ostenfeldii has been found to actively produce temporary cysts in cultures (Hakanen and Kremp, unpubl. data) and live field samples, although the trigger mechanisms are not always understood. It has been reported that when there is only low abundance of A. ostenfeldii vegetative cells in the water column, the population may have already undergone a life history transition by forming resting cysts deposited to benthic sediments as “seedbeds” for subsequent blooms (MacKenzie et al., 1996). These resting cysts remain toxic and can be ingested by shellfish directly from the sediments or via resuspension events driven by wind and other hydrodynamic mechanisms disrupting the sediments. In such cases, the relatively high concentration of 13-desmethyl spirolide C in the absence of vegetative cells in the water column could be associated with a rapid proliferation of cysts and remobilization from the sediments. A routine operation conducted at shellfish aquaculture farms is the lifting and physical displacement of lines that sink by the weight of the product (oyster modules, mussel socks, etc.), and which thereby causes sediment disruption and resuspension. We cannot therefore discard the possibility that A. ostenfeldii cysts were available for the mussels during previous days before sampling the water column and that the mussels accumulated the toxin from such cysts, even when vegetative cells could not be detected.
Conclusion
Integration of analytical techniques, i.e., optical microscopy, specific primers for species identification by PCR and LC-MS/MS for toxin determination in plankton and shellfish tissue matrices, allowed the confirmation of A. ostenfeldii as the primary and perhaps exclusive source of spirolide in TSB, and is the first record of the species in the northwest Pacific coast of Mexico. The species is a common component of the phytoplankton community but, typically occurs in low cell abundances. The analog 13-desmethyl spirolide C was the only spirolide detected in mussels from TSB, which suggests a very restricted toxin profile is produced by the dinoflagellate. The species was not consistently detected when strong stratification and higher temperatures were recorded in the water column, indicating that these environmental conditions are not favorable for the accumulation of this species in the region.
Author Contributions
PP-B determined the abundance of A. ostenfeldii cells, extracted the spirolide toxins, conducted the PCR reactions, analyzed and interpreted the data, and wrote the primary draft manuscript. EG-M and EP-R analyzed, interpreted, and reviewed the data for accuracy and integrity, and co-wrote and edited the manuscript. AC did a critical review of the manuscript, and co-authored and edited it. JB performed LC-MS/MS spirolide analysis. AA-B performed the morphological description of the species and CG-S helped with the molecular work.
Funding
This work was funded by CONACyT scholarship 280225 – CV 468796; FORDECYT – CONACyT project number 260040-2015; and Red Temática CONACyT (RedFAN) 2015-2017 projects.
Conflict of Interest Statement
The authors declare that the research was conducted in the absence of any commercial or financial relationships that could be construed as a potential conflict of interest.
Acknowledgments
We thank Acuacultura Oceanica Co. for providing the cultivated mussels for toxin analysis, and Bernd Krock and Annegret Müller (AWI) for analysis of mussels and filtered plankton samples for spirolides by LC-MS/MS for the period 2016–2017. The participation and financing of Allan Cembella and AWI co-workers was within the PACES II Research Program (Topic II Coast: WP3) of the Alfred-Wegener-Institut, Helmholtz Zentrum für Polar und Meeresforschung under the general theme Earth and Environment, Helmholtz Gemeinschaft, Germany. We also thank CONACYT for financial support of the PhD scholarship 280225 to PP-B, and the FORDECYT project 260040-2015, as well as Red Temática RedFAN 2015-2017 projects for travel funding for the morphological identification in CICY.
References
Almandoz, G., Montoya, N., Hernando, M., Benavides, H., Carignan, M., and Ferrario, M. (2014). Toxic strains of the Alexandrium ostenfeldii complex in southern South America (Beagle Channel, Argentina). Harmful Algae 37, 100–109. doi: 10.1016/j.hal.2014.05.011
Anderson, D., Glibert, P. J., and Burkholder, J. (2002). Harmful algal blooms and Eutrophication: nutrient sources, composition and consequences. Estuaries 25, 704–706. doi: 10.1007/BF02804901.
Anderson, D. M., Alpermann, T. J., Cembella, A. D., Collos, Y., Masseret, E., and Montresor, M. (2012). The globally distributed genus alexandrium: multifaceted roles in marine ecosystems and impacts on human health. Harmful Algae 14, 10–35. doi: 10.1016/j.hal.2011.10.012
Anderson, D. M. (1998). “Physiology and bloom dynamics of toxic Alexandrium species, with emphasis on life cycle transitions,” in Physiological Ecology of Harmful Algal Blooms eds D. M. Anderson, A. D. Cembella, and G. M. Hallegraeff (Heidelberg: Springer-Verlag), 29–40.
Balech, E. (1995). The Genus Alexandrium Halim (Dinoflagellata). Sherkin Island: Sherkin Island Marine Station.
Balech, E., and Tangen, K. (1985). Morphology and taxonomy of toxic species in the tamarensis group (Dinophyceae): Alexandrium excavatum (Braarud) comb. nov. and Alexandrium ostenfeldii (Paulsen) comb. nov. Sarsia 70, 333–343. doi: 10.1080/00364827.1985.10419687
Boczar, B., Beitler, M., Liston, J., Sullivan, J., and Cattolico, R. (1998). Paralytic shellfish toxins in Protogonyaulax tamarensis and Protogonyaulax catenella in axenic culture. Plant Physiol. 88, 1285–1290. doi: 10.1104/pp.88.4.1285
Borkman, D. G., Smayda, T. J., Tomas, C. R., York, R., Strangman, W., and Wright, J. (2012). Toxic Alexandrium peruvianum (Balech and de Mendiola) Balech and Tangen in Narragansett bay, Rhode Island (USA). Harmful Algae 19, 92–100. doi: 10.1016/j.hal.2012.06.004
Brandenburg, K., de Senerpont Domis, L., Wohlrab, S., Krock, B., Uwe, J., Van Scheppingen, Y., et al. (2017). Combined physical, chemical and biological factors shape Alexandrium ostenfeldii blooms in the Netherlands. Harmful Algae 64, 146–153. doi: 10.1016/j.hal.2017.02.004
Butterwick, C., Heaney, S. I., and Talling, J. F. (2005). Diversity in the influence of temperature on the growth rates of freshwater algae, and its ecological relevance. Freshw. Biol. 50, 29–300. doi: 10.1111/j.1365-2427.2004.01317.x
Cembella, A., Lewis, N., and Quilliam, M. (2000). The marine dinoflagellate Alexandrium ostenfeldii (Dinophyceae) as the causative organism of spirolide shellfish toxins. Phycologia 39, 67–74. doi: 10.2216/i0031-8884-39-1-67.1
Cembella, A. D., Bauder, A. G., Lewis, N. I., and Quilliam, M. A. (2000). “Population dynamics and spirolide composition of the toxigenic dinoflagellate Alexandrium ostenfeldii,” in Coastal Embayments of Nova Scotia, eds G. M. Hallegraeff, S. I., Blackburn, J. S. Bolch, and R. J. Lewis (Hobart: Intergovernmental Oceanographic Commission), 173–176.
Cembella, A. D. (2018): “Chapter 16 harmful algal species fact sheets,” in Harmful Algal Blooms: A Compendium Desk Reference, eds S. Alexandrium, J. Shumway, and S. Morton (Hoboken, NJ: Wiley-Blackwell).
Cembella, A. D., Bauder, A. G., Lewis, N. I., and Quilliam, M. A. (2001). Association of the gonyaulacoid dinoflagellate Alexandrium ostenfeldii with spirolide toxins in size-fractionated plankton. J. Plankton Res. 23, 1413–1419. doi: 10.1093/plankt/23.12.1413
Cembella, A. D., Lewis, N. I., and Quilliam, M. A. (1999). Spirolide composition in micro-extracted pooled cells isolated from natural plankton assemblages and from cultures of the dinoflagellate Alexandrium ostenfeldii. Nat. Toxins 8, 197–206. doi: 10.1002/1522-7189(200009/10)7:5<197::AID-NT62>3.0.CO;2-H
Collos, Y., Vaquer, A., Laabir, M., Abadie, E., Laugier, T., Pastoureaud, A., et al. (2007). Contribution of several nitrogen sources to growth of Alexandrium catenella during blooms in Thau lagoon, southern France. Harmful Algae 6, 781–789. doi: 10.1016/j.hal.2007.04.003
Edler, L., and Elbrächter, M. (2010). “The utermöhl method for quantitative phytoplankton analysis,” in Microscopic and Molecular Methods for Quantitative Phytoplankton Analysis eds B. Karlson, C. Cusack, and E. Bresnan (Paris: UNESCO).
Elferink, S., Neuhaus, S., Wohlrab, S., Toebe, K., Voß, D. Gottschling, M., Lundholm, N., et al. (2017). Molecular diversity patterns among various phytoplankton size-fractions in West Greenland in late summer. Deep Sea Res. Part I Oceanogr. Res. Pap. 121, 54–69. doi: 10.1016/j.dsr.2016.11.002
European Union Reference Laboratory for Marine Biotoxins [EURLMB] (2015). EU Harmonized Standard Operating Procedure for Determination of Lipophilic Marine Biotoxins in Molluscs by LC-MS/MS. Version 5. Available at: http://www.aecosan.msssi.gob.es/CRLMB/docs/docs/metodos_analiticos_de_desarrollo/EU-Harmonised-SOP-LIPO-LCMSMS_Version5.pdf
Franco, J. M., Paz, B., Riobo, P., Pizarro, G., Figueroa, R., Fraga, S., et al. (2006). “First report of the production of spirolides by Alexandrium peruvianum (Dinophyceae) from the Mediterranean Sea,” in Proceedings of the Abstracts 12th International Conference on Harmful Algae, Copenhagen, Denmark
Fritz, L., and Triemer, R. E. (1985). A rapid simple technique utilizing Calcofluor White M2R for the visualization of dinoflagellate thecal plates. J. Phycol. 21, 662–664. doi: 10.1111/j.0022-3646.1985.00662.x
García-Altarez. M., Casanova, A., Bane, V., Diogène, J., Furey, A., and De la Iglesia, P. (2014). Confirmation of pinnatoxins and.spirolides in shellfish and passive samplers from Catalonia (Spain) by liquid chromatography coupled with tripe quadrupole and high resolution hybrid tandem mass spectrometry. Mar. Drugs 12, 3706–3732. doi: 10.3390/md12063706
García-Mendoza, E., Cáceres-Martínez, J., Rivas, D., Fimbres-Martínez, M., Sánchez-Bravo, Y., Vásquez-Yeomans, R., et al. (2018). Mass mortality of cultivated northern bluefin tuna Thunnus thynnus orientalis associated with Chattonella marina in Baja California, Mexico. Front. Mar. Sci. 5:454. doi: 10.3389/fmars.2018.00454
García-Mendoza, E., Sánchez-Bravo, Y., Turner, A., Blanco, J., O’Neil, A., Mancera-Flores, J., et al. (2014). Lipophilic toxins in cultivated mussels (Mytilus galloprovincialis) from Baja California, México. Toxicon 90, 111–123. doi: 10.1016/j.toxicon.2014.07.017
Garneau, M. E., Schnetzer, A., Counway, P. D., Jones, A. C., Seubert, E. L., and Caron, D. A. (2011). Examination of seasonal dynamic of the toxic dinoflagellate Alexandrium catenella at Redondo beach, California by quantitative PCR. Appl. Environ. Microbiol. 77, 7669–7680. doi: 10.1128/AEM.06174-1
Gerssen, A., Mulder, P. P., McElhinney, M. A., and Boer, J. (2009). Liquid chromatography-tandem massspectrometry method for the detection of marine lipophilic toxins under alkaline conditions. J. Chromatogr. 1216, 421–430. doi: 10.1016/j.chroma.2008.12.099
Gill, S., Murphy, M., Clausen, J., Richard, D., Quilliam, M., MacKinnon, S., et al. (2003). Neural injury biomarkers of novel shellfish toxins, spirolides: a pilot study using immunochemical and transcriptional analysis. Neurotoxicology 24, 593–604. doi: 10.1016/S0161-813X(03)00014-7
Gribble, K., Keafer, B., Quilliam, M., Cembella, A., Kulis, D., Manahan, A., et al. (2005). Distribution and toxicity of Alexandrium ostenfeldii (Dinophyceae) in the Gulf of Maine, USA. Deep Sea Res. Part II Top. Stud. Oceanogr. 52, 2745–2763. doi: 10.1016/j.dsr2.2005.06.018
Hakanen, P., Suikkanen, Frazén, J., and Frazén, H. (2012). Bloom and toxin dynamics of Alexandrium ostenfeldii in shallow embayment at the SW coast of Finland, northern Baltic Sea. Harmful Algae 15, 91–99. doi: 10.1016/j.hal.2011.12.002
Hall, T. (1999). BioEdit: a user friendly biological sequence alignment editor and analysis program for Windows 95/98/NT. Nucleic Acids Sym. Ser. 41, 95–98.
Hargraves, P. E., and Maranda, L. (2002). Potentially toxic of harmful microalgae from the northeast coast. Northeastern Nat. 9, 81–120. doi: 10.1656/1092-6194(2002)009[0081:PTOHMF]2.0.CO;2
Horner, R. A., Garrison, D. L., and Plumley, F. G. (1997). Harmful algal blooms and red tide problems on the U.S west coast. Limnol. Oceanogr. 42, 1076–1088. doi: 10.4319/lo.1997.42.5_part_2.1076
Hu, T., Burton, I. W., Cembella, A. D., Curtis, J. M., Quilliam, M. A., Walter, J. A., et al. (2001). Characterization of spirolides A, C, and 13-desmethyl C, new marine toxin isolated from toxic plankton and contaminated shellfish. J. Nat. Prod. 64, 308–312. doi: 10.1021/np000416q
Hu, T., Curtis, J. M., Walter, J. A., and Wright, J. L. (1996). Characterization of biologically inactive spirolides E and F: identification of the spirolide pharmacophore. Tetrahedron Lett. 37, 7671–7674. doi: 10.1016/0040-4039(96)01721-2
Hu, T., Curtis, J. M., Oshima, Y., Quilliam, M. A., Walter, J. A., Watson-Wright, W. M., et al. (1995). Spirolides B and D, two novel macrocycles isolated from the digestive glands of shellfish. J. Chem. Soc. Chem. Commun. 2159–2161. doi: 10.1039/C39950002159
Jacox, M. G., Alexander, M. A., Mantua, N. J., Scott, J. D., Hervieux, G., Webb, R. S., et al. (2018). Forcing of multiyear extreme ocean temperatures that impacted California current living marine resources in 2016. Bull. Amer. Meteor. Soc. 99, S27–S33. doi: 10.1175/BAMS-D-17-0119.1
Jensen, M, Ø., and Moestrup, Ø. (1997). Autoecology of the toxic dinoflagellate Alexandrium ostenfeldii: life history and growth at different temperatures and salinities. Eur. J. Phycol. 32, 9–312. doi: 10.1080/09541449710001719325
Kremp, A., Lundholm, T., Drebler, N., Erler, K., Gerdts, G., Eirtovaara, S., et al. (2009). Bloom forming Alexandrium ostenfeldii (Dinophyceae) in shallow waters of the Aland Archipelago Northern Baltic Sea. Harmful Algae 8, 318–328. doi: 10.1016/j.hal.2008.07.004
Kremp, A., Tahvanainen, P., Litaker, W., Krock, B., Suikkanen, S., Leaw, C. P., et al. (2014). Phylogenetic relationships, morphological variation and toxin patterns in the Alexandrium ostenfeldii (Dinophyceae) complex: implications for species boundaries and identities. J. Phycol. 50, 81–100. doi: 10.1111/jpy.12134
Krock, B., Tillman, U, John, U., and Cembella, A. (2008). LC-MS/MS aboard ship: tandem mass spectrometry in the search for phycotoxins and novel toxigenic plankton from the North Sea. Anal. Bioanal. Chem. 392, 797–803. doi: 10.1007/s00216-008-2221-7
Lund, J. W. G., Kipling, C., and Le Cren, E. D. (1958). The inverted microscope method of estimating algal numbers and the statistical basis of estimations by counting. Hydrobiology 11, 143–170. doi: 10.1007/BF00007865
MacKenzie, L., White, D., Oshima, Y., and Kapa, J. (1996). The resting cyst and toxicity of Alexandrium ostenfeldii (Dinophyceae) in New Zealand. Phycologia 35, 148–155. doi: 10.2216/i0031-8884-35-2-148.1
MacKinnon, S., Cembella, A., Quilliam, M., LeBlanc, P., Lewis, N., Hardstaff, W., et al. (2004). “The characterization of two new spirolides isolated from Danish strains of the toxigenic dinoflagellate Alexandrium ostenfeldii,” in Proceedings of the 10th International Conference on Harmful Algae, Florida Fish and Wildlife Conservation Commission, eds K. A. Steidinger, J. H. Landsberg, C. R. Tomas, and G. A, Vargo (Paris: Intergovernmental Oceanographic Commission of UNESCO).
Maeda Martínez, A. N. (2008). “Estado actual del cultivo de bivalvos en México,” in Estado Actual del Cultivo y Manejo de Moluscos Bivalvos y su Proyección Futura: Factores que Afectan su Sustentabilidad en América Latina, eds A. Lovatelli, A. Farías, and I. Uriarte (Puerto Montt: Taller Técnico Regional de la FAO),91–100.
Martín-Morales, E., Mariño, C., Arévalo, F., Correa, J., Moroño, A., and Blanco, J. (2013). “Marine and freshwater toxins analysis,” in Proceedings of the Fourth Joint Symposium and AOAC Task Force Meeting, Baiona. doi: 10.13140/RG.2.1.2912.6647
McClatchie, S., Goericke, R., Leising, A., Auth, T. D., Bjorkstedt, E., Robertson, R., et al. (2016). State of the California current 2015–16: comparisons with the 1997–98 El Niño. Calif. Coop. Ocean Fish. Invest. Rep. 57, 5–61.
Moestrup,Ø., and Hansen, P. J. (1988). On the occurrence of the potentially toxic dinoflagellates Alexandrium tamarense (=Gonyaulax excavata) and A. ostenfeldii in Danish and Faroese waters. Ophelia 28, 195–213. doi: 10.1080/00785326.1988.10430813
Otero, A., Chapela, M. J., Atanassoya, M., Vieites, J. M., and Cabado, A. G. (2011). Cyclic imines: chemistry and mechanism of action: a review. Chem. Res. Toxicol. 11, ox1817–ox1829. doi: 10.1021/tx200182m.
Peña-Manjarrez, J. L., Helenes, J., Gaxiola-Castro, G., and Orellana-Cepeda, E. (2005). Dinoflagellate cysts and bloom events at Todos Santos Bay, Baja California, México, 1999–2000. Continental Shelf Res. 25, 1375–1393. doi: 10.1016/j.csr.2005.02.002
Penna, A., Bertozzini, E., Battocchi, C., Galluzzi, L., Giacobbe, M., Vila, M., et al. (2007). Monitoring of HAB species in the Mediterranean sea through molecular methods. J. Plankton Res. 29, 19–38. doi: 10.1093/plankt/fbl053
Percy, L., Morris, S., Higman, W., Stone, D., Hardstaff, W. R., Quilliam, M. A., et al. (2004). “Identification of Alexandrium ostenfeldii from the Fal Estuary, UK; morphology, molecular taxonomy and toxin composition,” in Proceedings of the XI International Conference on Harmful Algal Blooms, Cape Town, 209.
Regueiro, J., Rossignoli, A. E., Alvarez, G., and Blanco, J. (2011). Automated online solid-phase extraction coupled to liquid chromatography tandem mass spectrometry for determination of lipophilic marine toxins in shellfish. Food Chem. 129, 533–540. doi: 10.1016/j.foodchem.2011.04.054
Richard, D., Arsenault, E., Quilliam, M.A., and Cembella, A.D. (2001). “Investigations into the toxicology and pharmacology of spirolides, a novel group of shellfish toxins,” in Harmful Algal Blooms 2000, eds G. M. Hallegraeff, S. I. Blackburn, C. J. Bolch, and R. J. Lewis (Paris: International Oceanographic Commission), 383–386.
Rundberger, T., Aasen, J. A., Selwood, A. L., and Miles C. O. (2011). Pinnatoxins and spirolides in Norwegian blue mussels and seawater. Toxicon 58, 700–711. doi: 10.1016/j.toxicon.2011.08.008
Sánchez, S., Villanueva, P., and Carbajo, L. (2004). “Distribution and concentration of Alexandrium peruvianum (Balech and de Mendiola) in the Peruvian coast (03°24’–18°20’ LS) between 1982–2004,” in Proceedings of the XI International Conference on Harmful Algal Blooms, Cape Town.
Sánchez-Bravo, Y. (2013). Concentración de Toxinas Lipofílicas en el Mejillón Mediterráneo (Mytilus galloprovincialis) Asociadas a la Presencia del Dinoflagelado del Género Dinophysis Durante Enero a Diciembre del 2012, en la Bahía de Todos Santos, B.C, México. Tesis de licenciatura, Universidad Autónoma de Baja California, México City.
Schwarz, E. (2011). Molecular and Morphological Characterization of Alexandrium species (Dinophyceae) from the East Coast, USA. Master’s thesis, University of North Carolina Wilmington, Wilmington, NC.
Sleno, L., Chalmers, M. L., and Volmer, D. A. (2004). Structural study of spirolide marine toxins by mass spectrometry. Part II. Mass spectrometric characterization of unknown spirolides and related compounds in a cultured phytoplankton extract. Anal. Bioanal. Chem. 378, 977–986. doi: 10.1007/s00216-003-2296-0
Stocker, T. F., Qin, D., Plattner, G. K., Tignor, M., Allen, S. K., Boschung, J., et al. (2013). Climate change 2013: the physical science basis. Contribution of Working Group I to the Fifth Assessment Report of the Intergovernmental Panel on Climate Change. Cambridge: Cambridge University Press, 1535.
Suikkanen, S., Kremp, A., Hautala, H., and Krock, B. (2013). Paralytic shellfish toxins or spirolides? The role of environmental and genetic factors in toxin production of the Alexandrium ostenfeldii complex. Harmful Algae 26, 52–59. doi: 10.1016/j.hal.2013.04.001
Thompson, J. D., Gibson, T. J., Plewniak, F., Jeanmougin, F., and Higgins, D. G. (1997). The ClustalX windows interfase: flexible strategies for multiple sequence alignment aided by quality analysis tools. Nucleic Acid Res. 25, 4876–4882. doi: 10.1093/nar/25.24.4876
Touzet, N., Franco, J. M., and Raine, R. (2008). Morphogenetic diversity and biotoxin composition of Alexandrium (Dinophyceae) in Irish coastal waters. Harmful Algae 7, 782–797. doi: 10.1016/j.hal.2008.04.001
Touzet, N., Keady, E., Raine, R., and Maher, M. (2009). Evaluation of taxa-species real time PCR, whole-cell FISH and morphotaxonomy analyses for the detection and quantification of the toxic microalgae Alexandrium minutum (Dinophyceae), global clade ribotype. FEMS Microbiol. Ecol. 67, 329–341. doi: 10.1111/j.1574-6941.2008.00627.x
Trainer, V., Moore, L., Bill, B., Adams, N., Harrington, N., Borchert, J., et al. (2013). Diarrheic shellfish toxins and other lipophilic toxins of human health concern in Washington state. Mar. Drugs 11, 1816–1817. doi: 10.3390/md11061815
Van de Waal, D. B., Tillmann, U., Martens, H., Krock, B., Van Scheppingen, Y., and John, U. (2015). Characterization of multiple isolates from an Alexandrium ostenfeldii bloom in the Netherlands. Harmful Algae 49, 94–104. doi: 10.1016/j.hal.2015.08.002
Van Wagoner, R. M., Misner, I., Tomas, C. R., and Wright, J. L. C. (2011). Occurrence of 12- methylgymnodimine in a spirolide producing dinoflagellate Alexandrium peruvianum and the biogenic implications. Tetrahedron Lett. 52, 4243–4246. doi: 10.1016/j.tetlet.2011.05.137
Wohlrab, S., Iversen, M. H., and John, U. (2010). A molecular and co-evolutionary context for grazer induced toxin production in Alexandrium tamarense. PLoS One 5:e15039. doi: 10.1371/journal.pone.0015039
Keywords: polyether toxins, Mediterranean mussel, 13-desmethyl spirolide-C, LC-MS/MS, marine dinoflagellates, harmful algal blooms
Citation: Paredes-Banda P, García-Mendoza E, Ponce-Rivas E, Blanco J, Almazán-Becerril A, Galindo-Sánchez C and Cembella A (2018) Association of the Toxigenic Dinoflagellate Alexandrium ostenfeldii With Spirolide Accumulation in Cultured Mussels (Mytilus galloprovincialis) From Northwest Mexico. Front. Mar. Sci. 5:491. doi: 10.3389/fmars.2018.00491
Received: 01 September 2018; Accepted: 04 December 2018;
Published: 18 December 2018.
Edited by:
Marius Nils Müller, Federal University of Pernambuco, BrazilReviewed by:
Mariângela Menezes, Universidade Federal do Rio de Janeiro, BrazilMohamed Laabir, Université de Montpellier, France
Copyright © 2018 Paredes-Banda, García-Mendoza, Ponce-Rivas, Blanco, Almazán-Becerril, Galindo-Sánchez and Cembella. This is an open-access article distributed under the terms of the Creative Commons Attribution License (CC BY). The use, distribution or reproduction in other forums is permitted, provided the original author(s) and the copyright owner(s) are credited and that the original publication in this journal is cited, in accordance with accepted academic practice. No use, distribution or reproduction is permitted which does not comply with these terms.
*Correspondence: Patricia Paredes-Banda, ZXBhcmVkZXNAY2ljZXNlLmVkdS5teA== Ernesto García-Mendoza, ZXJnYXJjaWFAY2ljZXNlLm14