- Institute of Biological Sciences – Marine Biology, University of Rostock, Rostock, Germany
We present results on bioirrigation and reworking of sediments by benthic macrofauna in sediments of the Pomeranian Bay (southern Baltic Sea), that were obtained ∼22 years ago. The investigation took place at four stations ranging from 9 to 19 m water depth, which we observed between 1993 and 1995 over the course of 30 months. In order to assess exchange of solutes across and particles from the sediment–water interface with the underlying sediments, we used bromide as ex situ tracer for bioirrigation and chlorophyll a equivalents as in situ tracer for particle reworking. Using models to interpret tracer distributions in the sediment, we compare the magnitudes of small scale, diffusion-like, versus relatively large-scale, non-local, transport modes. Our results indicate a spatial differentiation of the bay: the coastal station most heavily impacted by eutrophication close to the Oder River mouth showed medium reworking and intense bioirrigation combined with lower chlorophyll concentrations throughout the sediment. This contrasts with high surface pigment concentrations at the shallow Oder Bank station, indicating benthic primary production and intense particle mixing. Medium local particle reworking at the northwestern station and medium mean solute transport rates characterized the two deeper stations in the northern Bay. The bromide tracer experiments, which exclusively depict animal activity, showed significant biological solute transport to ∼10 cm sediment depth within 3 days. Non-biological transport mechanisms in the field (resuspension, fishing activity, and pore water advection) might additionally affect the in situ tracer chlorophyll a – depth distributions. This tracer, too, indicates mixing within the uppermost 10 cm of the sediment within ∼2–3 months. In general, experimentally obtained solute transport constants were higher than most values reported previously (surface α: 155 year-1) and particle reworking was at the high end of reported values as well. Thus, benthic fauna is responsible for an intense bioturbation in Pomeranian Bay. Specifically, high bioirrigation rates were associated with high density and biomass of deep burrowing polychaetes. Recently published rates of particle reworking in the same area are on the same order of magnitude as ours obtained two decades ago. This finding is consistent with the species composition which generally remained the same.
Introduction
Bioturbation activity by benthic fauna influences early diagenetic processes, which are important for organic matter decomposition in benthic habitats. In estuarine regions with high nutrient inputs and intense matter cycling a well-developed macrofauna community plays a key role in organic matter degradation before final burial of the material in deeper sediment layers. Depending on the organisms’ densities, body size, mode of locomotion, and feeding type as well as the seasonal and interannual variability of benthic communities, the transport intensities vary considerably between sites (e.g., Lee and Swartz, 1980).
Transport processes in the solute phase (bioirrigation) primarily determine the oxidation status of the sediment by a downward transport of dissolved oxygen from the water column into the sediment and a release of reduced/adsorbed compounds like ammonia, phosphate, hydrogen sulfide, or methane (e.g., Aller, 1982). Particle reworking by fauna (particulate phase transport) often causes a rapid downward transport of labile fresh organic material derived from the water column (e.g., Graf, 1992), a slower transport of inorganic particles in the same direction, and may be responsible for the release of particle-associated contaminants due to changing redox conditions (e.g., Emerson et al., 1984; Kersten, 1988). Bioturbation is considered sensitive to anthropogenic drivers (Griffiths et al., 2017), but its effects on ecosystem functioning remain unclear. The present study adds to the understanding of variability and drivers of bioturbation in a comparatively shallow marine system.
Tracer studies using either artificial or natural tracer substances are used to quantify transport processes by benthic animals. In order to compare methods and transport mechanisms involved in different habitats, mathematical simulations of reworking and bioirrigation may be applied using diffusive and/or advective (non-local) transport modes (e.g., Berner, 1980; Aller, 1982; Aller and Yingst, 1985; Boudreau, 1986a,b; Martin and Banta, 1992; Sun et al., 1994; Soetaert et al., 1996; Gerino et al., 1998). Inert bromide ions are the most common solute tracers used. Still the number of studies with application to natural benthic communities is scarce (e.g., Martin and Banta, 1992; Forster et al., 1995, 2003b; Kauppi et al., 2018). Thus, both magnitude of solute exchange and the factors responsible for the transport still need further investigation. Particle tracer studies are more frequent and a whole suite of particles (minerals, various radionuclides, artificial particles, and chlorophyll) have been used (compare, e.g., Boudreau, 1997; Bradshaw et al., 2006). In the sediment chlorophyll is a highly attractive and labile substance and may therefore be particularly suited to trace bioturbation.
A few studies, mostly from the Baltic Sea, (Powilleit et al., 1994; Quintana et al., 2007; Hedman et al., 2011; Renz and Forster, 2013) combine particle and solute tracer studies. The combined studies of transport in these two phases representing the sediment has provided insight in the relative importance of solute versus particle movement, however, mostly in single species experiments. Studies using natural communities and both solute and particle tracers might reveal patterns of bioturbation (e.g., seasonal, geographic, external factors, other), since their common driver is the benthos. Bioturbation studies from the Baltic Sea are rare and often give information restricted to single species (e.g., Mahaut and Graf, 1987; Brey, 1989; Schaffner et al., 1992; Powilleit et al., 1994; Renz and Forster, 2013, 2014). The investigation presented here was conducted in the Pomeranian Bay, a coastal region in southern Baltic Sea influenced by the Oder River. A benthos sampling program started in April 1993 to generate an overview of the status of the macrozoobenthos. The communities were characterized by low species richness and a strong gradient in zoobenthic biomass with highest values in the coastal south western part (Powilleit et al., 1995; Kube, 1996; Kube et al., 1996a,c).
The aim of this study was to quantify particle reworking and bioirrigation at different sites within a relatively homogeneous area of the Pomeranian Bay. The study employed bromide as ex situ tracer for dissolved compounds (Martin and Banta, 1992) and sedimentary pigments as in situ tracer for organic particle movement (e.g., Sun et al., 1991, 1994; Boon and Duineveld, 1998; Morys et al., 2016). Different transports were compared on the basis of mixing coefficients derived from simulation processes with transport models. We identified dominant benthic species and modes of transport involved in different areas of the relatively homogeneous eutrophic shallow habitat to assess the importance of benthic fauna on biodegradation and transport processes. Finally, we compare our findings from the 90s to a recent investigation on particle reworking in this area by Morys et al. (2016, 2017).
The Study Area
The Pomeranian Bay, southern Baltic Sea, is a shallow bay situated north of the Oder river estuary between Germany and Poland. The total area covers approximately 6000 km2 taking the 20 m isobath as the northern boundary to the adjacent Arkona and Bornholm Basins. Water depth is <20 m with the central part the Oder Bank measuring water depths of only 7 to 9 m (Figure 1). Well to very well sorted fine sandy sediments prevail (Bobertz and Harff, 2004) with the exception of the Sassnitz Channel region in the north-western part where sediments are increasingly silty and less well sorted. Except for station 952, which is close but not in the channel, all of our station are located on the relatively homogeneous sandy grounds of the bay.
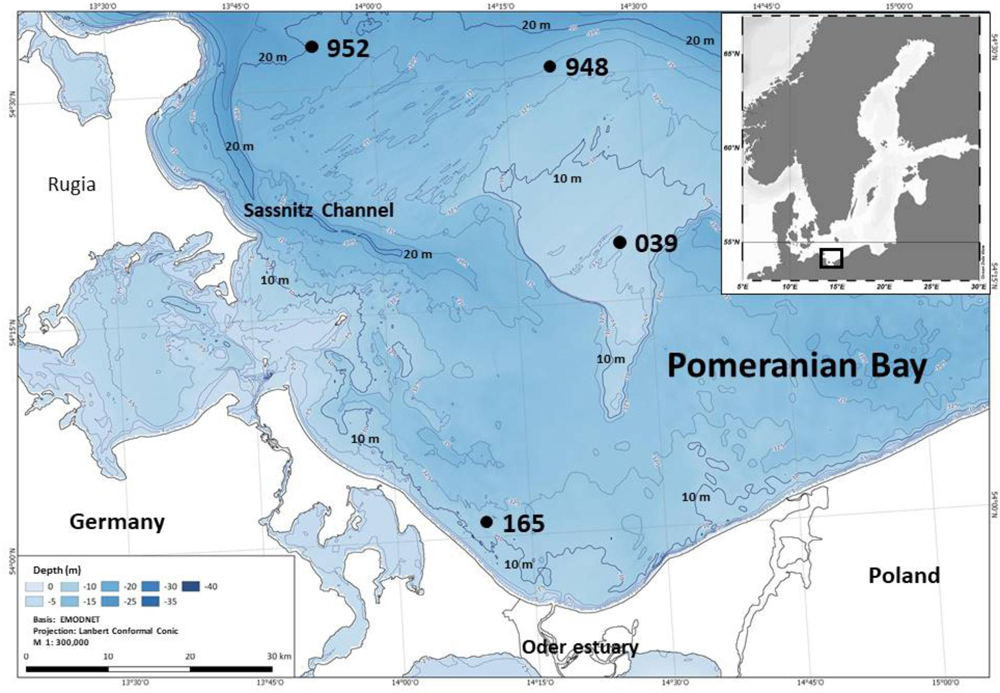
Figure 1. Map of the investigation area, Pomeranian Bay, and its location in the southern Baltic Sea. Stations are located at different water depths between 9 and 19 m across the shallow bay consisting of well sorted fine sands. The deeper channel system with more heterogeneous and considerably finer sediment is not part of the sampling scheme.
The major discharge of large freshwater input and nutrients into the Pomeranian Bay and source of eutrophication originates from the Oder River, which was one of the most contaminated rivers in Central Europe, through Szczecin Lagoon (Lampe, 1993; Siegel et al., 1996). Discharge loads follows the coast to the north-west or northeast direction depending on the predominant wind direction (Schernewski et al., 2001; Siegel et al., 2005). Tauber and Emeis (2005) substantiated that transport of fine material, nutrients and discharge from the Oder River predominantly occurs in the form of suspended fluffy material into the adjacent Arkona Basin in the north, while sandy bottoms remain stationary for many years.
Mean salinity varied from 7.8 near the Oder mouth to 8.5 near the Sassnitz Channel (Kube et al., 1996a) (Table 1). Sediments are typical of nutrient-poor sands, with median grain sizes ranging from 165 to 190 μm which were associated with low silt fractions (<1% of total mass) and values of loss on ignition (LOI) < 0.6% dry mass, reflecting low organic contents. We did not observe substantial deviations of median grain size at station 952 despite its location close the Sassnitz Channel.
The total number of benthic infauna species in the Pomeranian Bay was 35 (Powilleit et al., 1995). About 10 typical estuarine species (e.g., Peringia ulvae, Mya arenaria, Limecola balthica, Pygospio elegans, Nereis diversicolor, and Marenzelleria spp.) have an occurrence of nearly 90% and dominate the species inventory (Powilleit et al., 1995). Additionally these sediments often contain mobile clumps of the blue mussel Mytilus sp. and associated fauna, which resulted in benthic communities a bit more variable in terms of species diversity and biomass (Powilleit et al., 1995). Water depth, sediment characteristics, and the food supply in the bottom water were considered to be the most important factors controlling the distribution of macrofauna (Kube et al., 1996b). In the mid-nineties, when this study was conducted, highest macrofauna biomasses (up to 150 g AFDW m-2) were found in the estuarine region near the river mouth with dominance of suspension feeding bivalves. Pronounced gradients in macrofauna biomass existed from this coastal zone to outer parts of the Pomeranian Bay with biomasses of only 1 g AFDW m-2 (Powilleit et al., 1995).
The four stations of this study represent main macrofauna assemblages in the investigation area which could be separated by multivariate analyses (Powilleit et al., 1995) and basically these macrofauna distribution pattern still persist (e.g., Glockzin and Zettler, 2008; Darr et al., 2014a,b): the organically enriched southwestern coastal part close to the mouth of the Oder river (station 165, 11 m water depth), the shallow and exposed Oder Bank (station 039, 9 m), the northern part of Pomeranian Bay (station 948, 15 m) and the transition zone in the deep channel region in the northwestern part of the bay (station 952, 19 m), which is connected to the Arkona Basin (see Powilleit et al., 1995).
Materials and Methods
Collection and Sample Treatment
Between April 1993 and October 1995 sediment samples of four sampling locations were taken with a box corer (0.0225 m2) and additionally with a van Veen grab for macrofauna analyses in April 1993 (0.1 m2) in the Pomeranian Bay (Figure 1). Box core samples were subsampled on board for sediment pigment analyses (five or six sampling dates per station), for laboratory tracer experiments (up to 10 subcores per station and sampling date), and for sediment analyses using Plexiglas® cores of 10 cm inner diameter.
Bioirrigation Experiments
To determine the exchange of solutes at the sediment–water interface we performed laboratory tracer experiments of 3 days duration on land using sediment cores from the respective four sampling sites. The activity of organisms (with fauna, n = 3 replicate cores) was compared to the molecular diffusive transport of the tracer in previously deep frozen cores without living fauna (n = 1; i.e., defaunated) at each station.
Sediment cores were kept in a temperature controlled water tank in the dark and aerated individually. This aeration also maintained a well-mixed water column and no additional stirring was applied in order to not induce advective pore water flows. Sodium bromide (NaBr) was used as a chemical inert tracer for solute transport and added from a stock solution to the well-mixed overlying water (∼1 liter) to each sediment cores. The concentration at the start of the experiment was set to ∼10 mM Br-, with later measurements confirming the exact concentrations.
We analyzed water samples for bromide at the start and the end of the experiment by iodometric titration according to Kremling (1983). At the end of the experiments the sediments were sectioned into slices of 0.5 cm intervals (0–3 cm) and 1 cm intervals (3 – max. 12 cm) and pore water extracted by centrifugation (Saager et al., 1990). Concentrations of bromide were analyzed using the same titration method. During the slicing procedure all macrofauna sufficiently large to be seen by the naked eye were noted and dominant bioirrigators identified. Porosity was determined as the difference between wet weight and dry weight after drying subsamples of known volume (1.0 cm3) at 60°C for 12 h (for details see Powilleit et al., 1994).
Bromide experiments on previously frozen defaunated cores were used as controls for the biologically enhanced transports.
Sediment Pigment Content
Sediment cores of the four stations 165, 039, 952, and 948 were sliced into ten 1 cm slices on board immediately after sampling and stored deep frozen until laboratory analyses. For chlorophyll a equivalent and phaeopigment determinations about 2 cm3 sediment subsamples of these slices (n = 1) were extracted in 8 cm3 of 90% acetone for at least 1 h with repeated vigorous shaking (Vortex) of the tubes that were kept dark and refrigerated. Pigment levels were measured by fluorescence before and after acidification on a Turner Designs Model 10 AU fluorometer according to Edler (1979) adapted to sediments. The extracted sediment was then dried at 60°C and weighed. Pigment concentrations are expressed as μg pigment cm-3 of sediment.
Water content, porosity and organic matter content were determined by weighing and as LOI in 1 cm sediment slices after combustion at 520°C.
Modeling
We used different standard transport models to compare and quantify biogenic transports, i.e., bioirrigation and particle reworking. These models interpret the depth distribution of tracer in the sediment by accounting for transport and, in the case of chlorophyll, reaction processes (details below). In case of bromide, molecular diffusion and non-local irrigation mimic the transport; no reaction of bromide occurs. For chloropigment profiles the model includes sedimentation, chloropigment degradation and diffusive mixing of sediments, a diffusion analogy. Model derived depth distributions of the respective tracers are compared to the measured tracer distributions from our experiments. A best fit finally yields the transport parameters describing bioirrigation and reworking of particles.
Chloropigment distributions with sediment depth, generated in situ, were simulated with a hierarchical family of steady state transport-reaction models published by Soetaert et al. (1996). Each member of the hierarchy, starting from a simple advection/decay model, includes all processes of the previous model. Model 1 simply includes sedimentation and decay of chloropigments. Model 2 adds diffusive particle mixing as biological transport (DB) and model 3 the direct injection of particles as a non-local transport into a specific deeper sediment layer, L (for details see Soetaert et al., 1996; Morys et al., 2016, 2017). We performed degradation experiments immediately after sampling and obtained site specific decay rates of chloropigments in the range of 0.1008 and 0.121 day-1. We used a sedimentation rate of 2.74 × 10-5cm day-1 determined by Leipe et al. (1995) for the nearby Arkona Basin and the Oder Haff in our modeling procedure. Best fits to our data were obtained mainly by the models 1 and 2. In one instance model 3 including the additional non-local injection flux to depth L gave the best fit.
Since chlorophyll profiles were generated in situ, reworking and non-local transports may be of other than biological origin as well.
We investigated solute exchange driven by macrofauna using Br- ions as tracer (Martin and Banta, 1992; Anderson et al., 2006) and calculated exchange coefficients from solute tracer profiles following (Forster et al., 2003b). Briefly, bioirrigation was formulated as a non-local irrigation function (α) of sediment depth (z) which was fit to the data. In the model vertical molecular diffusive transport of tracer into the pore water is enhanced by adding radial diffusion around burrow structures as envisioned by Aller (1980) and Boudreau (1984). This procedure takes into account site-specific porosity. Parameters in equation 1 are concentrations (C) in the overlying water (Colw) and at depth (Cz) in the sediment, molecular diffusivity (Ds) and constants α0 and α1.
While the constants α0 and α1 (given in Supplementary Table 1) obtained by the modeling procedure describe the decline of solute transport activity as a function of depth, a discrete value may be calculated for any depth by inserting the two constants into the original exponential function. Below we use these discrete values, irrigation constants averaged over 5 cm depth intervals (e.g., α0-5), for an easier comparison among stations.
Results
Sediments
Between April 1993 and October 1995 sediment temperatures at the study sites ranged between 4.3 and 17.1°C with only slight differences between sites at any given date due to water depth. Structural sediment parameters were basically homogeneously distributed all over the Bay (e.g., median grain size) with little spatial variations even in the channel region in the north-west. Porosity varied slightly between 0.36 (station 039) and 0.40 (station 952). Organic matter contents in surface sediments were generally very low at all stations investigated (<0.3 to 0.1% dw) with slightly higher values in the south-western coastal area and small seasonal variations.
Bioirrigation Experiments
Laboratory experiments revealed that bioirrigation activity by the benthic fauna increased the solute exchange across the sediment/water interface considerably compared to molecular diffusion. Bromide concentrations declined much slower in all inhabited cores compared to defaunated controls at all stations (Figure 2), indicating an increased solute transport depicted here by the non-local irrigation function α. Nevertheless, all control experiments, allowing only for molecular diffusion as a transport mechanism, still indicated α0-5 ranging from 32 to 42 year-1 but high attenuation with depth (Table 2 and Supplementary Table 1). If calculated for each cm interval, α declined to values <10 year-1 within the top 3 cm in these diffusive defaunated controls (data not shown).
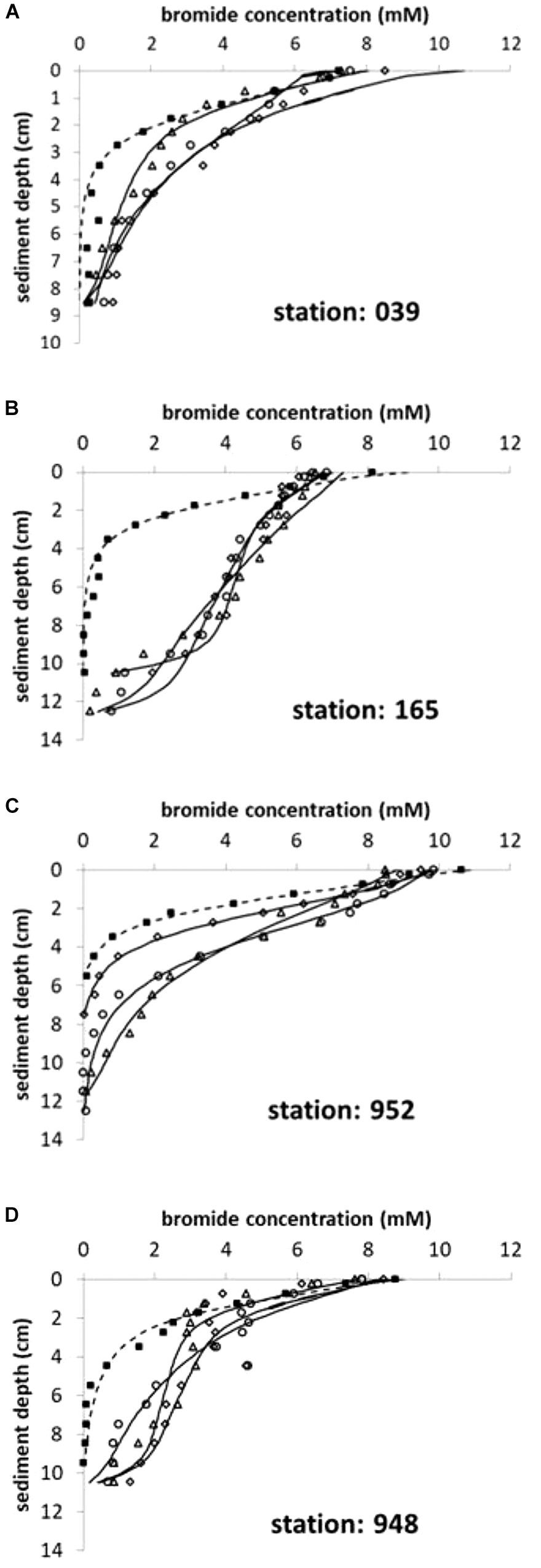
Figure 2. (A–D) Bromide concentration profiles in sediment cores after 3 days of incubation. Four stations in the Pomeranian Bay are shown with one control (squares; dashed line) and three replicate cores containing active fauna (core 1: triangles, core 2: circles, core 3: diamonds; solid lines). Lines depict the modeled tracer distribution with depth according to equation (2) and (3) using α0 and α1 values given in Supplementary Table 1.
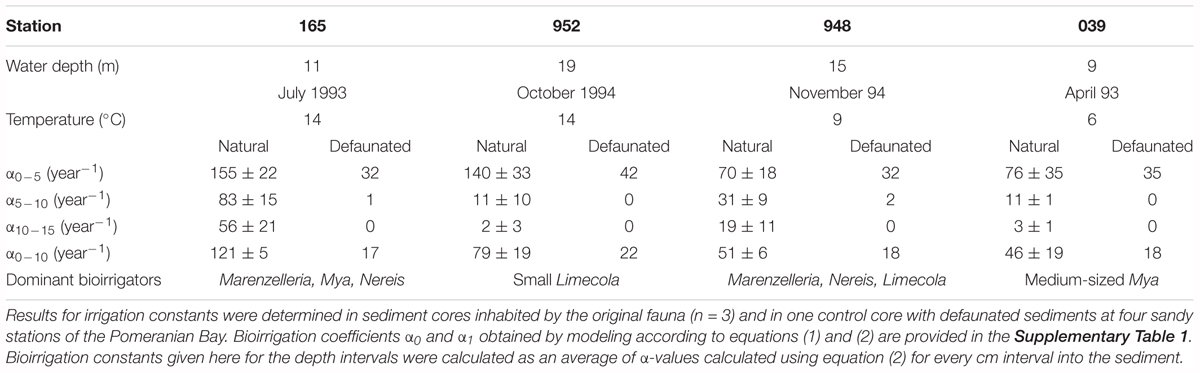
Table 2. Water depth, incubation temperature, and bioirrigation constants calculated for various depth intervals and dominant bioirrigating fauna in the bromide tracer experiments.
At all stations with the exception of 039 (shallow central Oder Bank) the solute tracer concentrations indicate a minimum depth of 10 or 11 cm to which sediments are influenced by the bioirrigation activity of the benthic community. Solute transport was highest at station 165 in the southwest (Table 2; α0-5 = 155 ± 22 year-1) with the dominant species Marenzelleria spp., Nereis diversicolor, and Mya arenaria in the sediment cores. The deepest location (952) in the northwestern part of the investigation area was next in bioirrigation intensity, with the dominant species L. balthica, Marenzelleria spp. and N. diversicolor. We calculated much smaller exchange coefficients at stations 039 on Oder Bank and 948 in the deeper northern region with medium sized M. arenaria and small sized L. baltica (Table 2). Here means in the upper 5 cm of the sediments were α0-5 ∼ 70–76 year-1 (Table 2).
In situ Pigment Profiles
When looking at seasonal changes in the sediment chloropigment content of all stations the highest concentrations were measured at the shallowest station 039 at the Oder Bank with values between 1.9 and 4.6 μg cm-3 (Figure 3). April and September 1994 show the highest concentrations. The northern station 948 reveals surface chlorophyll concentrations of about 2 μg cm-3. Both stations showed decreasing values with depths and accordingly model 2 (diffusion-like mixing) reveals the best fit results in most cases (Figure 3 includes the results of the simulations). For the only time in September 1994 at station 039 model 3, which includes a non-local tracer injection term, resulted in the best fit to the measured chloropigment concentrations.
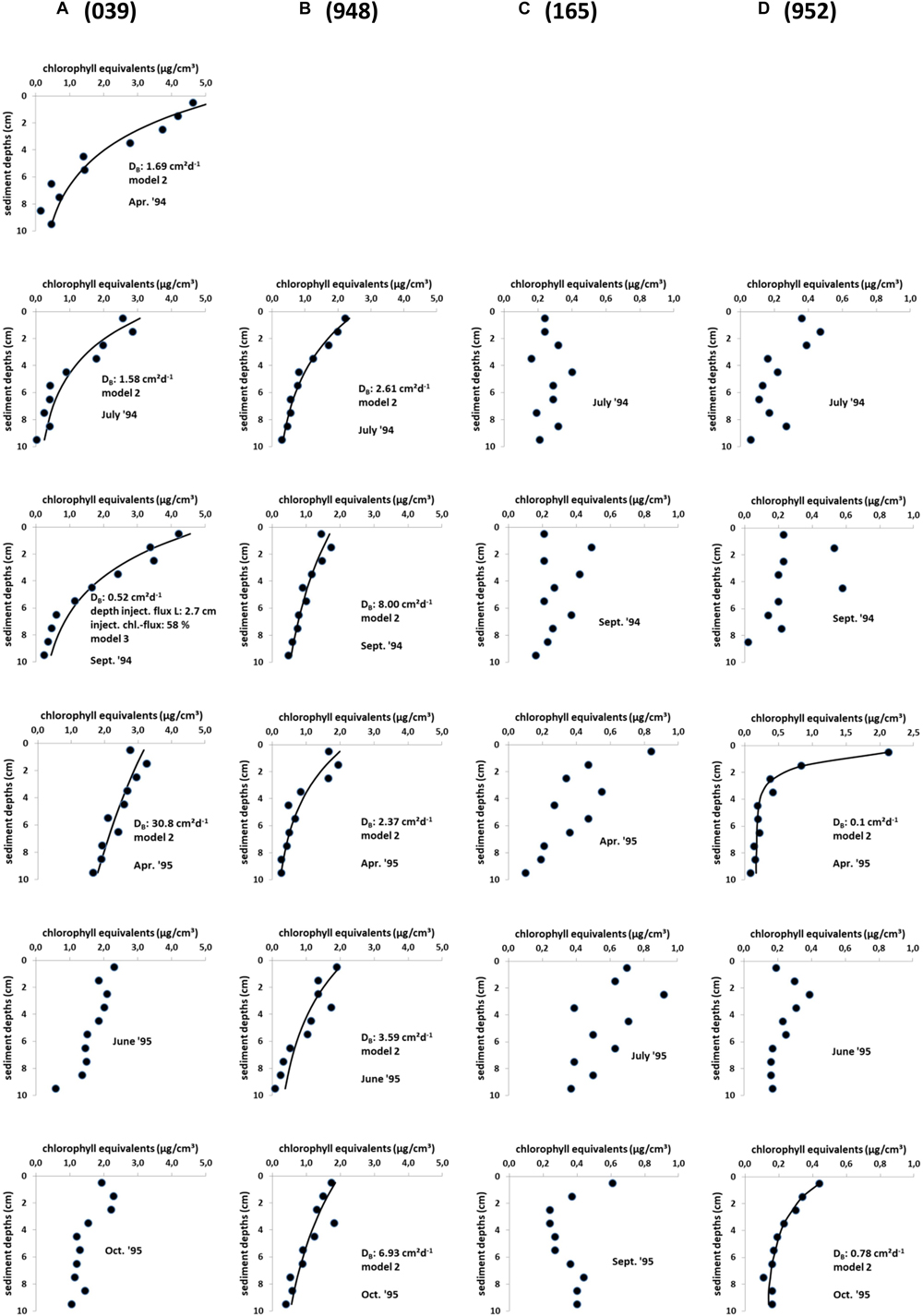
Figure 3. (A–D) In situ chlorophyll equivalent concentration profiles (dots) in sediment cores of stations 039 (A), 948 (B), 165 (C), and 952 (D) in the Pomeranian Bay with active fauna between April 1994 and October 1995. Solid lines indicate the simulations with the diffusion/non-local bioturbation models 2 and 3, which include bioturbative (diffusive) mixing only in model 2 or an additional non-local transport term of the pigment flux directly into a certain sediment depth L in model 3 (Soetaert et al., 1996).
Low chlorophyll concentrations characterized the sediment at the deepest station 952 (<0.5 μg cm-3) except for a spring peak in the surface sediment layer in 1995. Similarly the coastal station 165 was without pronounced seasonality (<0.9 μg cm-3). Here pigment profiles differ considerably from the other two stations due to several smaller subsurface peaks and no concentration gradient with depth. Accordingly in the modeling of chlorophyll profiles (at a significance level of 1%) model 1 gave the best fits at these two stations indicating that only sedimentation and no biological transport took place. Only in two cases at station 952 (April and October 1995) modeling resulted in model 2, which includes sedimentation and diffusive mixing (DB < 0.8 cm2 day-1). Highest diffusive bioturbation coefficients DB occurred at station 039 (up to 30.8 cm2 day-1) and station 948 (up to 8.0 cm2 day-1) (Figure 3).
Generally, the coastal station 165 is characterized by the highest mean pigment inventory of 32.7 μg 10 cm-3 (μg cm-2 10 cm-1) whereas station 948 shows the lowest mean of 23.6 μg 10 cm-3 (Figure 4). Station 165 and station 952 showed inventories of chlorophyll equivalents and phaeopigments generally typical for many sediments located at greater water depths, where degraded chlorophyll deposits at the sediment surface. Here phaeopigments clearly dominate the inventories (19–35 μg 10 cm-3) during all seasons with only low contents of chlorophyll equivalents (<6 μg 10 cm-3) indicating older organic material in the sediments. Considerably different pigment inventories, with similar medium contents of both components (9–24 μg 10 cm-3) and slightly higher chlorophyll equivalent values, characterized the sediment at the Oder Bank station 039. Slightly higher phaeopigment values, but also of similar magnitude in both components, prevail at the northern station 948 (Figure 4).
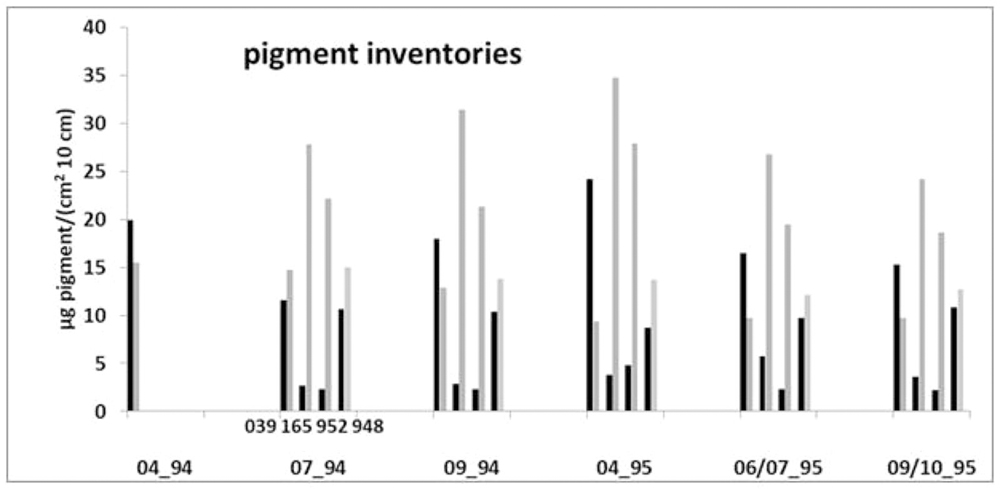
Figure 4. Chlorophyll equivalent and phaeopigment inventories of the upper 10 cm sediment (μg cm-2 10 cm-1) of the stations 039, 165, 952, and 948 in the Pomeranian Bay between April 1994 and October 1995.
Discussion
In this study we investigated solute and particle transport by benthic fauna into the sediments of the Pomeranian Bay. Bromide (bioirrigation) tracers are used ex situ under laboratory conditions which essentially makes the benthic fauna in the sediment the only possible agents causing solute transport. This may be different for the particle tracer employed, natural chlorophyll pigments occurring in situ. Here, in principle hydrodynamic action and bottom fishing gear may affect observed depth distributions in addition to fauna. Compared to such episodic physical events, however, bioturbation causes a more continuous transport. The ex situ tracer shows intense solute exchange by benthic animals to ∼10 cm sediment depth at all stations. Biological solute transport to this depth occurs within the experimental duration of 3 days. While physical transport mechanisms may add to natural chloropigment transport, this tracer, too, indicates mixing within the uppermost 10 cm of the sediment within at least ∼2–3 months (tracer life-time). We conclude that in Pomeranian Bay benthic fauna facilitates solute exchange on very short timescales (3 days) and particle reworking on short time scales of a few weeks. Especially in late summer, a period often characterized by low mixing and stagnant conditions in a stratified water column, the transports induced by fauna play a key role in biodegradation for instance due to the transport of oxygen into the sediment.
While relatively homogeneous in sediment structure, the existing differences in fauna composition among stations also show up in the biological transports investigated here. We identified one hot spot in bioirrigation activity (165, southern Bay) and one in particle reworking (039, Oder Bank). A qualitative judgment as to which location is characterized by the higher tracer transport and which by lower may be simply based on the shapes of tracer depth profiles and the tracer penetration depth. This approach uses the experimental results directly and is not affected by factors such as decay constants or model boundary conditions. Since we also modeled the depth distributions a quantitative comparison is also possible. Both qualitative and quantitative criteria were used to establish sequences of decreasing biological transport (Table 3). An important and reassuring result of this comparison is that, in principle, the sequences of biological transport intensities among the stations compared is the same. High particle reworking and relatively little bioirrigation characterize the central Oder Bank station 039. The southern location 165 near the Oder river mouth shows an opposite trend with very high solute transport and an unusual chloropigment tracer distribution, which we will discuss below.
Station 948, characteristic for the open northern area of the Pomeranian Bay sandy flats, and the Oder Bank station 039 have balanced ratio of chlorophyll a versus phaeopigments in common. In this, they differ from typical sediments where degradation of chloropigment leads to much higher phaeopigment than chlorophyll a concentrations (Josefson et al., 2012). At both these offshore locations light can penetrate to the seafloor (own observations at 039) such that we interpret generally higher pigment concentrations here (Figures 3, 4) as a sign of benthic primary production by microphytobenthos. Location 952 at the northwestern channel with considerable bioirrigation and reworking reflects conditions at 165, Oder river mouth, without pronounced benthic primary production and accordingly relatively high phaeopigment contents. It seems that different conditions with respect to the food supply, here indicated by benthic phototrophs, in addition to the slight differences in fauna community affect biological transports.
Magnitudes of Solute Transport
The bioirrigation coefficients for all four stations are high, in fact α0-10 of 121 ± 5 year-1 is one of the highest value reported for benthic communities in literature so far (Figure 5). The benthic community in the coastal part of Pomeranian Bay caused very high irrigation transport when compared to bromide tracer results and exceed those from Buzzards Bay, United States, fourfold (Martin and Banta, 1992), the southern North Sea results by Forster et al. (1995) threefold and those obtained from the western Baltic Sea by a factor 2.5 (Powilleit et al., 1994; Schlüter et al., 2000). All solute exchange profiles (Figure 2) indicate transport to depths far below 3 cm that are reached in control diffusion experiments, in which alpha declines to values <10 year-1 below 3 cm already. Bromide, and similar solutes like sulfate, oxygen, or DOM, can reach 10 cm depth within 3 days, while reduced substances, NH4+ or HS-, may be transported upward by the same principle (Aller, 1980; Boudreau, 1984). Since the shallow water sands of Pomeranian Bay are permeable (Forster et al., 2003a), water flows above the sediment will be able to induce advective pore water movement (Huettel et al., 1996), whenever the weather conditions permit. There are no measurements of the magnitude such advective flows available from this area. Advective downwelling typically reaches 3–5 cm deep within 24 h (Huettel and Gust, 1992). Given this velocity water from the sediment surface would potentially reach 10 cm depth during ∼2 days. We may thus conclude that biological solute transport will continuously mix dissolved substances to sediment depths which are comparable to those likely mixed intermittently by pore water advection.
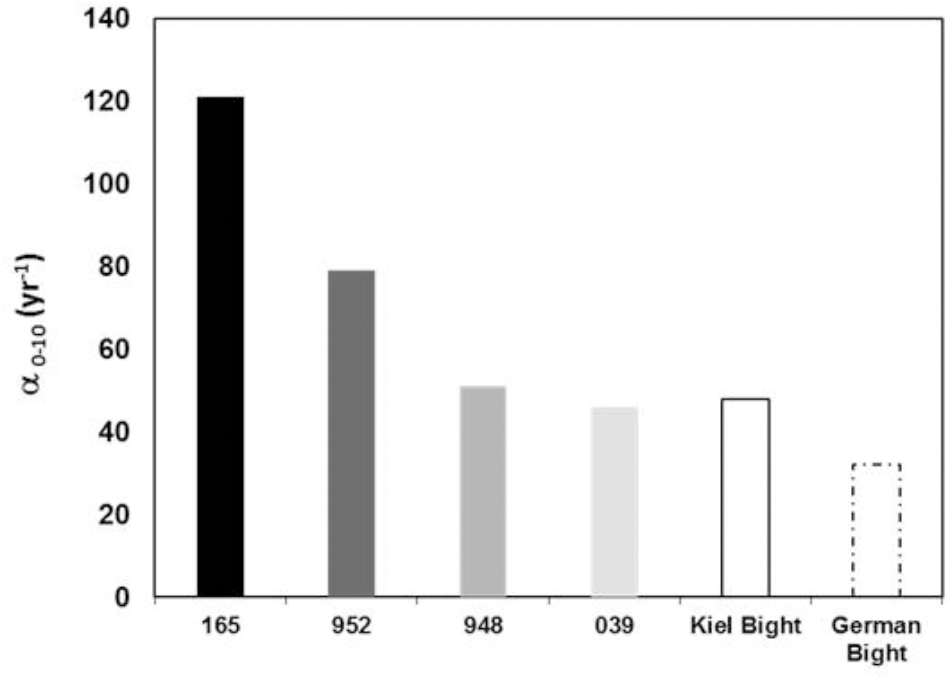
Figure 5. Irrigation rate constants integrated from 0 to 10 cm sediment depth using α0 and α1. Results from this study on Pomeranian Bay are compared to studies employing the same depth dependent analyses of the irrigation rate constant from Kiel Bight (Schlüter et al., 2000) and the German Bight (Forster et al., 2003b) and previous data for comparison.
Bioirrigation is caused mainly by feeding activities of polychaetes that generate bulky profiles with high bromide concentrations below 6 cm depth and reaching to more than 10 cm (165, 948). Higher bioirrigation coefficients were also determined in experiments exclusively containing Marenzelleria spp. or Heteromastus filiformis (Quintana et al., 2007; Renz and Forster, 2013). The later authors determined a rate of 130 year-1 on 1273 individuals per m2. At station 165, depicting high community bioirrigation rates, a high abundance of Marenzelleria spp. of 2400 individuals m-2 was found in 1993, while at the other locations it ranged from 50 to 130 individuals m-2. The species then called M. cf. viridis might today be identified as M. viridis or M. neglecta, the latter being the dominant Marenzelleria species in the Pomeranian Bay today. Since these two sibling species perform similarly in bioirrigation activity it is only important to state in this context that the smaller M. arctia does not inhabit the area. Direct observations revealed that Marenzelleria spp. waved its tentacles during feeding and moved vertically in its burrow especially during defecation, enabling a noticeable solute transport into deeper sediment layers by ventilation activity. Irrigation by the other dominant polychaete H. diversicolor may additionally explain the high exchange coefficients observed in the Pomeranian Bay (Davey et al., 1990; Riisgård, 1991; Clavero et al., 1994; Vedel et al., 1994; Davey and Watson, 1995). This species, too, was twice as abundant (360 individuals m-2) at 165 compared to the other locations. In addition siphon movements by adult, filter-feeding specimens of the bivalve Mya arenaria and crawling of Limecola balthica and medium-sized M. arenaria were observed and could be another cause of a considerable solute transport in the upper 6 cm of the sediment. Our results show that exceptionally high bioirrigation and high particle reworking rates can occur even in the absence of conspicuous and strong bioturbators like Arenicola marina (Meysman et al., 2006). In this context the role of M. arenaria awaits further exploration.
Comparing in situ temperatures and irrigation rates in Table 2 one might suspect temperature to cause high rates. In 1994 at 952 (14°C) and 948 (9°C) irrigation rates in the top 5 cm are twice as high at the elevated temperature, suggesting a relation similar to the temperature dependency of metabolism (Q10). In subsequent depth intervals 5–10 cm and 10–15 cm, however, the coefficients are higher at station 948 despite its lower temperature. This hints at the surficially active small Limecola found at 952. However, our data are not sufficient to extract, support or reject a Q10 value. Forster et al. (2003b) observed that bioirrigation was high and nearly the same in January (∼7°C) and in October (∼14°C) 1988/89 in a North Sea community. In that data set bioirrigation was relatively low and of the same intensity both in August (∼16°C) and February (∼5°C), when abundance of organisms was low at both dates and likely more important than temperature for the intensity of solute exchange. Similarly from the present data set we see that temperature may affect the irrigation activity of benthos, but not only and probably not predominantly so. Bioirrigation may also be influenced by factors such as food supply or hypoxia, but the structure (abundance, biomass, identity) of the benthic community seems the most relevant one.
Particle Transport Derived From Sedimentary Pigments
The general pattern found with the natural particle tracer chlorophyll is that particle reworking is highest on the central Oder Bank, followed by the northern area of the Bay and lower at the northwestern channel and southern Oder mouth area. Reworking rates determined ranged from 0.5 to 30 cm2 day-1 at 039 and from 2.4 to 8 cm2 day-1 at 948. At station 952 we could detect reworking rates only twice with small values <1 cm2 day-1, while no reworking was found at station 165 (see below). These reworking rates are among the highest reported so far (Boudreau, 1997).
There are several reasons that may explain why chlorophyll derived reworking rates are high compared to those obtained using other particle tracer. As food particles, cells or cell fragments containing chloropigments are clearly more attractive than indifferent tracers, such as luminophores, glass beads, or radioactive tracers adsorbed to the organic matrix on mineral grains. These trace average sediment particles and not the most attractive portion, which chlorophyll may represent.
In models that incorporate two or more transport mechanisms the allocation of tracer to local and non-local processes affects the magnitude of DB, too. In our data there is only one situation (September 1994, station 039) where the model software suggested non-local transport to a sediment depth L of 2.7 cm. According to the model, this flux constitutes more than half of the total chlorophyll transported into the sediment by bioturbation, with the remaining 42% left to diffusive mixing, DB. In fact, this coincides with the lowest DB determined at station Oder Bank. In the same area Morys et al. (2017) detected more incidences of non-local transport.
We cannot exclude additional transport of chlorophyll by physical mechanisms occurring only in the field, such as sediment resuspension and mixing by waves (Jenness and Duineveld, 1985) or advective particle transport (Grant, 1983; Huettel et al., 1996). Whatever the mechanism, chlorophyll tracer is found at 4–6 cm depth (extreme >10 cm) and reaches this depth during its life time of a few months. Thus, particles in sediments of the Pomeranian Bay are transported an order of magnitude slower than solutes.
At station 165, Oder river mouth, we found no bioturbation when using the interpretation software “mixing.” We suggest that in this situation the model approach is not suitable for an extraction of reworking rates. In temperate regions, like the Pomeranian Bay, primary production follows a relatively regular seasonal pattern with a maximum in spring and a smaller secondary fall maximum (e.g., Graf, 1992; Sun et al., 1994). The chloropigment inventories at the study sites in general follow this pattern with slightly higher spring values (Figure 4). Morys et al. (2017) suggested surface feeding and consequently reduced foraging (reworking) activity, when chlorophyll was higher concentrated at sediment surface. Murray et al. (2017) provide an example of highest reworking rates at medium, not at maximum levels of food concentration in the bottom water with the polychaete H. diversicolor.
Knowing that at 165 many organisms with high total biomass were present, we propose the following explanation for a lack of chlorophyll pigments in the sediment. All major fauna elements here, Marenzelleria spp., Nereis diversicolor, Limecola balthica, and Mya arenaria, are well capable of suspension feeding and prefer to feed on fresh labile organic particles from the water column and/or sediment surface (e.g., Riisgård, 1991). At the high biomass of up to 150 g AFDW m-2 and density of large organisms present, the community may have essentially taken all food from the interfacial water layer by suspension feeding and effectively prevented chloropigment containing material from settling onto the sediment. With particle tracer missing in the sediment (compare low concentrations at all times at 165), the model software could only interpret the depth distribution as no bioturbation (model 1). In fact, at the 5% confidence level, which we did not use in this study, mixing interprets those depth distributions as exceedingly high DB, which also shows that under these conditions the model framework is useless without additional information available. Possibly experiments reported by Webb (1993) with L. balthica in subtidal sediments without benthic primary production relate to the same mechanism, since they did not show any clear effect on sedimentary chlorophyll a concentrations. We consider 165 to be reworked by the abundant fauna, however, unable to extract quantitative information.
Comparing Then and Now
Our result stem from a study performed between 1993 and 1995. In recent studies on particle reworking (Morys et al., 2016, 2017; Morys, 2016) the authors sampled the location “Oder bank” at 16 m depth. It is closest to our station 948 (15 m depth) visited about 22 years before. A comparison of particle transport is possible since they also determined rates using chlorophyll as a tracer. Between summer 2014 and fall 2015 reworking rates determined at five occasions with 6–23 sediment cores analyzed each time, ranged from 0.4 and 3.9 cm2 day-1 (Morys, 2016). Only in winter 2014 DB were exceptionally low (0.005 cm2 day-1) (Morys et al., 2016). Our DB at station 948 range from 2.4 to 8.0 cm2 day-1 (n = 5) with no sign of non-local transport. Morys (2016) and Morys et al. (2016, 2017) did find substantially more non-local transport. In conclusion, particle transport seen today seems as intense as two decades ago when judged based on diffusive reworking of sediments. There may be a change in the nature of transport, i.e., more non-local rather than diffusive reworking, but due to the sample numbers in the 1990s and only partially comparable abundance/biomass data between both periods this aspect does not allow for a profound comparison.
Continuously high rates of particle reworking are consistent with the fauna inventory (community composition and dominant species), which has not changed profoundly since then, despite the obvious variations of abundance and biomass in time and space. Since reworking today is on the same order of magnitude as that two decades ago, the biological transport at the sea bed may not have changed so far in response of the reduction of nutrient inputs to the coastal zone (Schernewski et al., 2011; Wasmund et al., 2011). While chlorophyll concentrations in the waters of the Pomeranian Bay decline (Wasmund et al., 2011), the eutrophic state is probably still high. Benthic-pelagic coupling and nutrients cycling between seabed and water column buffers the system, so that a lag in a possible response may be expected. If bioturbation is predominantly dependent on the benthic community structure, as we suggest, continued research focusing on effects of species identity and abundance seem paramount.
Author Contributions
MP conducted all experiments, sample and data analysis as well as particle modeling. SF conducted bromide modeling, contributed to tables, and graphics. Both authors contributed equally to writing and editing.
Funding
This study was conducted at the Leibniz Institute for Baltic Sea Research Warnemünde (IOW) and at the University of Rostock and supported by the Federal Ministry of Research and Technology (BMBF) under grant number: 03F0105B.
Conflict of Interest Statement
The authors declare that the research was conducted in the absence of any commercial or financial relationships that could be construed as a potential conflict of interest.
Acknowledgments
We wish to thank U. Wolff, K. Fennel, and A. Khalili for their help during modeling as well as G. Graf and J. Renz for helpful comments on the draft version. G. Nausch and B. Koine kindly provided sediment data. Special thanks are due to C. Peters for her assistance during field and laboratory work. This manuscript also profited from two helpful reviews.
Supplementary Material
The Supplementary Material for this article can be found online at: https://www.frontiersin.org/articles/10.3389/fmars.2018.00472/full#supplementary-material
References
Aller, R. C. (1980). Quantifying solute distributions in the bioturbated zone of marine sediments by defining an average microenvironment. Geochim. Cosmochim. Acta 44, 1955–1965. doi: 10.1016/0016-7037(80)90195-7
Aller, R. C. (1982). “The effects of macrobenthos on chemical properties of marine sediment and overlying water,” in Animal Sediment Relations, eds P. L. McCall and M. J. S. Tevesz (New York, NY: Plenum Press), 53–102.
Aller, R. C., and Yingst, J. Y. (1985). Effects of the marine deposit-feeders Heteromastus filiformis (polychaeta), Macoma baltica (bivalvia) and Tellina texana (Bivalvia) on averaged sedimentary solute transport, reaction rates, and microbial distributions. J. Mar. Res. 43, 615–645. doi: 10.1357/002224085788440349
Anderson, M. J., Ellingsen, K. E., and Mcardle, B. H. (2006). Multivariate dispersion as a measure of beta diversity. Ecol. Lett. 9, 683–693. doi: 10.1111/j.1461-0248.2006.00926.x
Berner, R. A. (1980). Early Diagenesis, a Theoretical Approach. Princeton, NJ: Princeton Univ. Press.
Bobertz, B., and Harff, J. (2004). Sediment facies and hydrodynamic setting: a study in the south western Baltic Sea. Ocean Dyn. 54, 39–48. doi: 10.1007/s10236-003-0061-8
Boon, A. R., and Duineveld, G. C. A. (1998). Chlorophyll a as a marker for bioturbation and carbon flux in southern and central North Sea sediments. Mar. Ecol. Progr. Ser. 162, 33–43. doi: 10.3354/meps162033
Boudreau, B. P. (1984). On the equivalence of nonlocal and radial-diffusion models for porewater. J. Mar. Res. 42, 731–735. doi: 10.1357/002224084788505924
Boudreau, B. P. (1986a). Mathematics of tracer mixing in sediments: I. spacially-dependent, diffusive mixing. Am. J. Sci. 286, 161–198. doi: 10.2475/ajs.286.3.161
Boudreau, B. P. (1986b). Mathematics of tracer mixing in sediments: II. nonlogical mixing and biological conveyor belt phenomena. Am. J. Sci. 286, 199–238. doi: 10.2475/ajs.286.3.199
Boudreau, B. P. (1997). Diagenetic Models and Their Implementation: Modelling Transport and Reactions in Aquatic Sediments. Berlin: Springer.
Bradshaw, C., Kumblad, L., and Fagrell, A. (2006). The use of tracers to evaluate the importance of bioturbation in remobilising contaminants in Baltic sediments. Estuar. Coastal Shelf Sci. 66, 123–134. doi: 10.1016/j.ecss.2005.08.002
Brey, T. (1989). The impact of physical and biological factors on structure and dynamics of the sublitoral Macoma -community in Kiel Bay. Ber. Inst. Meereskd 186:248.
Clavero, V., Niell, F. X., and Fernandez, J. A. (1994). A laboratory study to quantify the influence of nereis diversicolor O.F.Müller in the exchange of phosphate between sediment and water. J. Exp. Mar. Biol. Ecol. 176, 257–267. doi: 10.1016/0022-0981(94)90188-0
Darr, A., Gogina, M., and Zettler, M. L. (2014a). Detecting hot-spots of bivalve biomass in the south-western Baltic Sea. J. Mar. Syst. 134, 69–80. doi: 10.1016/j.jmarsys.2014.03.003
Darr, A., Gogina, M., and Zettler, M. L. (2014b). Functional changes in benthic communities along a salinity gradient- a western Baltic case study. J. Sea Res. 85, 315–324. doi: 10.1016/j.seares.2013.06.003
Davey, J. T., and Watson, P. G. (1995). The activity of Nereis diversicolor (Polychaeta) and its impact on nutrient fluxes in estuarine waters. Ophelia 41, 57–70. doi: 10.1080/00785236.1995.10422037
Davey, J. T., Watson, P. G., Bruce, R. H., and Frickers, P. E. (1990). An instrument for the monitoring and collection of the vented burrow fluids of benthic infauna in sediment microcosms and its application to the polychaetes Hediste diversicolor and Arenicola marina. J. Exp. Mar. Biol. Ecol. 139, 135–149. doi: 10.1016/0022-0981(90)90043-C
Edler, L. (1979). Recommendations on methods for marine biological studies in the baltic sea Phytoplankton and Chlorophyll. Baltic Mar. Biol. 5, 1–38.
Emerson, S., Jahnke, R., and Heggie, D. (1984). Sediment-water exchange in shallow water estuarine sediments. J. Mar. Res. 42, 709–730. doi: 10.1016/j.marenvres.2018.09.013
Forster, S., Bobertz, B., and Bohling, B. (2003a). Permeability of sands in the coastal areas of the southern Baltic Sea: mapping a grain-size related sediment property. Aquat. Geochem. 9, 171–190. doi: 10.1023/B:AQUA.0000022953.52275.8b
Forster, S., Khalili, A., and Kitlar, J. (2003b). Variation of nonlocal irrigation in a subtidal benthic community. J. Mar. Res. 61, 335–357. doi: 10.1357/002224003322201223
Forster, S., Graf, G., Kitlar, J., and Powilleit, M. (1995). Effects of bioturbation in oxic and hypoxic conditions: a microcosm experiment with a North Sea sediment community. Mar. Ecol. Prog. Ser. 116, 153–161. doi: 10.3354/meps116153
Gerino, M., Aller, R. C., Lee, C., Cochran, J. K., Aller, J. Y., Green, M. A., et al. (1998). Comparison of different tracers and methods used to quantify bioturbation during a spring bloom: 234-Thorium, luminophores and chlorophyll a. Estuar. Coast. Shelf Sci. 46, 531–547. doi: 10.1006/ecss.1997.0298
Glockzin, M., and Zettler, M. L. (2008). Spatial macrozoobenthic distribution patterns in relation to major environmental factors- a case study from the Pomeranian Bay (southern Baltic Sea). J. Sea Res. 59, 144–161. doi: 10.1016/j.seares.2008.01.002
Graf, G. (1992). Benthic-pelagic coupling: a benthic view. Oceanogr. Mar. Biol. Annu. Rev. 30, 149–190. doi: 10.1007/s11356-015-4997-2
Grant, J. (1983). The relative magnitude of biological and physical sediment reworking in an intertidal community. J. Mar. Res. 41, 673–689. doi: 10.1357/002224083788520469
Griffiths, J. R., Kadin, M., Nascimento, F. J. A., Tamelander, T., Törnroos, A., Bonaglia, S., et al. (2017). The importance of benthic–pelagic coupling for marine ecosystem functioning in a changing world. Glob. Change Biol. 23, 2179–2196. doi: 10.1111/gcb.13642
Hedman, J. E., Gunnarsson, J. S., Samuelsson, G., and Gilbert, F. (2011). Particle reworking and solute transport by the sediment-living polychaetes Marenzelleria neglecta and Hediste diversicolor. J. Exp. Mar. Biol. Ecol. 407, 294–301. doi: 10.1016/j.jembe.2011.06.026
Huettel, M., and Gust, G. (1992). Solute release mechanisms from confined sediment cores in stirred benthic chambers and flume flows. Mar. Ecol. Prog. Ser. 82, 187–197. doi: 10.3354/meps082187
Huettel, M., Ziebis, W., and Forster, S. (1996). Flow-induced uptake of particulate matter in permeable sediments. Limnol. Oceanogr. 41, 309–322. doi: 10.4319/lo.1996.41.2.0309
Jenness, M. I., and Duineveld, G. C. A. (1985). Effects of tidal currents on chlorophyll a content of sandy sediments in the southern North Sea. Mar. Ecol. Prog. Ser. 21, 283–287. doi: 10.3354/meps021283
Josefson, A. B., Norrko, J., and Norrko, A. (2012). Burial and decomposition of plant pigments in surface sediments of the Baltic Sea: role of oxygen and benthic fauna. Mar. Ecol. Prog. Ser. 455, 33–49. doi: 10.3354/meps09661
Kauppi, L., Bernard, G., Bastrop, R., Norkko, A., and Norkko, J. (2018). Increasing densities of an invasive polychaete enhance bioturbation with variable effects on solute fluxes. Nat. Sci. Rep. 8:7619. doi: 10.1038/s41598-018-25989-2
Kersten, M. (1988). “Geobiological effects on the mobility of contaminants in marine sediments,” in Pollution of the North Sea, eds W. Salomons (Berlin: Springer), 36–58.
Kremling, K. (1983). “Determination of major constituents,” in Methods of Sea Water Analysis, ed. K. Grasshoff (Weinheim: Verlag Chemie), 247–268.
Kube, J. (1996). The ecology of macrozoobenthos and sea ducks in the Pomeranian Bay. Meereswiss Ber. Warnemünde 18, 1–128.
Kube, J., Peters, C., and Powilleit, M. (1996a). Spatial variation in growth of Macoma balthica and Mya arenaria (Mollusca, Bivalvia) in relation to environmental gradients in the Pomeranian Bay (southern Baltic Sea). Arch. Fish. Mar. Res. 44, 81–93.
Kube, J., Powilleit, M., and Warzocha, J. (1996b). The importance of hydrodynamic processes and food availability for the structure of macrofauna assemblages in the Pomeranian Bay (Southern Baltic Sea). Arch. Fuer Hydrobiol. 138, 213–228.
Kube, J., Zettler, M. L., Gosselck, F., Ossig, S., and Powilleit, M. (1996c). Distribution of Marenzelleria viridis (Polychaeta: Spionidae) in the Southwestern Baltic Sea in 1993/94 - ten years after introduction. Sarsia 81, 131–142.
Lampe, R. (1993). Environmental state and material flux in the western part of the Oder river estuary - results and consequences. Petermanns Geogr. Mitt. 137, 275–282.
Lee, H., and Swartz, R. C. (1980). Biological processes affecting the distribution of pollutants in marine sediments. part 2: biodeposition and bioturbation. contaminants and sediments. Anal. Chem. Biol. 2, 555–606.
Leipe, T., Neumann, T., and Emeis, K.-C. (1995). Schwermetallverteilung in holozänen Ostseesedimenten -untersuchungen im Einflußbereich der Oder. Geowissenschaften 13, 470–478.
Mahaut, M. L., and Graf, G. (1987). A luminophore tracer technique for bioturbation studies. Oceanol. Acta 10, 323–328.
Martin, W. R., and Banta, G. T. (1992). The measurement of sediment irrigation rates: a comparison of the Br – tracer and 222Rn/ 226Ra disequilibrium techniques. J. Mar. Res. 50, 125–154. doi: 10.1357/0022240927847P97737
Meysman, F. J. R., Galaktionov, O. S., Gribsholt, B., and Middelburg, J. J. (2006). Bioirrigation in permeable sediments: advective pore-water transport induced by burrow ventilation. Limnol. Oceanogr. 51, 142–156. doi: 10.4319/lo.2006.51.1.0142
Morys, C. (2016). Particle Dynamics in Sediments of the Western Baltic Sea. Ph.D. thesis, University Rostock, Rostock
Morys, C., Forster, S., and Graf, G. (2016). Variability of bioturbation in various sediment types and on different spatial scales in the southwestern Baltic Sea. Mar. Ecol. Progr. Ser. 557, 31–49. doi: 10.3354/meps11837
Morys, C., Powilleit, M., and Forster, S. (2017). Bioturbation in relation to the depth distribution of macrozoobenthos in the southwestern Baltic Sea. Mar. Ecol. Progr. Ser. 579, 19–36. doi: 10.3354/meps12236
Murray, F., Solan, M., and Douglas, A. (2017). Effects of algal enrichment and salinity on sediment particle reworking activity and associated nutrient generation mediated by the intertidal polychaete Hediste diversicolor. J. Exp. Mar. Biol. Ecol. 495, 75–82. doi: 10.1016/j.jembe.2017.06.002
Powilleit, M., Kitlar, J., and Graf, G. (1994). Particle and fluid bioturbation caused by the priapulid worm Halicryptus spinulosus (v.Seibold). Sarsia 79, 109–117. doi: 10.1080/00364827.1994.10413551
Powilleit, M., Kube, J., Maslowski, J., and Warzocha, J. (1995). Distribution of macrobenthic invertebrates in the Pomeranian Bay (Southern Baltic) in 1993/1994. Bull. Sea Fish. Inst. 3, 75–87.
Quintana, C. O., Tang, M., and Kristensen, E. (2007). Simultaneous study of particle reworking, irrigation transport and reaction rates in sediment bioturbated by the polychaetes Heteromastus and Marenzelleria. J. Exp. Mar. Biol. Ecol. 352, 392–406. doi: 10.1016/j.jembe.2007.08.015
Renz, J. R., and Forster, S. (2013). Are similar worms different? a comparative tracer study on bioturbation in the three sibling species Marenzelleria arctia, M. viridis, and M. neglecta from the Baltic Sea. Limnol. Oceanogr. 58, 2046–2058. doi: 10.4319/lo.2013.58.6.2046
Renz, J. R., and Forster, S. (2014). Effects of bioirrigation by the three sibling species of Marenzelleria spp. on solute fluxes and porewater nutrient profiles. Mar. Ecol. Progr. Ser. 505, 145–159. doi: 10.3354/meps10756
Riisgård, H. U. (1991). Suspension feeding in the polychaete Nereis diversicolor. Mar. Ecol. Prog. Ser. 70, 29–37. doi: 10.1016/j.marpolbul.2016.02.033
Saager, P. M., Sweerts, J.-P., and Ellermeijer, H. J. (1990). A simple pore-water sampler for coarse, sandy sediments of low porosity. Limnol. Oceanogr. 35, 747–751. doi: 10.4319/lo.1990.35.3.0747
Schaffner, L. C., Jonsson, P., Diaz, R. J., Rosenberg, R., and Gapcynski, P. (1992). Benthic communities and bioturbation history of estuarine and coastal systems: effects of hypoxia and anoxia. Sci. Total Environ. Suppl. 21–24, 1001–1016. doi: 10.1016/B978-0-444-89990-3.50087-0
Schernewski, G., Neumann, T., Opitz, D., and Venohr, M. (2011). Long-term eutrophication history and ecosystem changes in a large Baltic river basin – estuarine system. Transit. Waters Bull. 5, 21–49.
Schernewski, G., Neumann, T., Podsetchine, V., and Siegel, H. (2001). Spatial impact of the Oder river plume on water quality along the south-western Baltic coast. Int. J. Hyg. Environ. Health 204, 143–155. doi: 10.1078/1438-4639-00086
Schlüter, M., Sauter, E., Hansen, H. P., and Suess, E. (2000). Seasonal variations of bioirrigation in coastal sediments: modelling of field data. Geochim. Cosmochim. Acta 64, 821–834. doi: 10.1016/S0016-7037(99)00375-0
Siegel, H., Gerth, M., and Schmidt, T. (1996). Water exchange in the pomeranian bight investigated by satellite data and shipborne measurements. Cont. Shelf Res. 16, 1793–1817. doi: 10.1016/0278-4343(96)00012-X
Siegel, H., Seifert, T., Schernewski, G., Gerth, M., Ohde, T., Reißmann, J., et al. (2005). Discharge and transport processes along the German Baltic Sea Coast. Ocean Dyn. 55, 47–66. doi: 10.1007/s10236-005-0110-6
Soetaert, K., Herman, P. M. J., Middelburg, J. J., Heip, C., Destigter, H. S., Van Weering, T. C. E., et al. (1996). Modeling 210Pb-derived mixing activity in ocean margin sediments: diffusive versus nonlocal mixing. J. Mar. Res. 54, 1207–1227. doi: 10.1357/0022240963213808
Sun, M., Aller, R. C., and Lee, C. (1991). Early diagenesis of chlorophyll-a in Long Island Sound sediments: a measure of carbon flux and particle reworking. J. Mar. Res. 49, 379–401. doi: 10.1357/002224091784995927
Sun, M. Y., Aller, R. C., and Lee, C. (1994). Spatial and temporal distributions of sedimentary chloropigments as indicators of benthic processes in Long Island Sound. J. Mar. Res. 52, 149–176. doi: 10.1357/0022240943076768
Tauber, T., and Emeis, K.-C. (2005). Sediment mobility in the Pomeranian Bight (Baltic Sea): a case study based on sidescan-sonar images and hydrodynamic modelling. Geo. Mar. Lett. 25, 221–229. doi: 10.1007/s00367-004-0207-9
Vedel, A., Andersen, B. B., and Riisgard, H. U. (1994). Field investigations of pumping activity off the facultatively filter-feeding polychaete nereis diversicolor using an improved infrared phototransducer system. Mar. Ecol. Progr. Ser. 103, 91–102. doi: 10.3354/meps103091
Wasmund, N., Schöppe, C., Göbel, J., and von Weber, M. (2011). Chlorophyll-a in den Deutschen Ostseegewässern. Meeresumwelt aktuell Nord- und Ostsee 2011/2. Available at: http://www.blmp-online.de/Seiten/Berichte.html
Keywords: macrozoobenthos, bioturbation, bioirrigation, chloropigments, Baltic Sea
Citation: Powilleit M and Forster S (2018) Continuous and High Transport of Particles and Solutes by Benthos in Coastal Eutrophic Sediments of the Pomeranian Bay. Front. Mar. Sci. 5:472. doi: 10.3389/fmars.2018.00472
Received: 25 April 2018; Accepted: 23 November 2018;
Published: 07 December 2018.
Edited by:
Elinor Andrén, Södertörn University, SwedenReviewed by:
Robert Lafite, Université de Rouen, FranceCintia Organo Quintana, University of Southern Denmark, Denmark
Copyright © 2018 Powilleit and Forster. This is an open-access article distributed under the terms of the Creative Commons Attribution License (CC BY). The use, distribution or reproduction in other forums is permitted, provided the original author(s) and the copyright owner(s) are credited and that the original publication in this journal is cited, in accordance with accepted academic practice. No use, distribution or reproduction is permitted which does not comply with these terms.
*Correspondence: Martin Powilleit, bWFydGluLnBvd2lsbGVpdEB1bmktcm9zdG9jay5kZQ==