- 1Instituto do MAR, Horta, Portugal
- 2Marine and Environmental Sciences Centre, Horta, Portugal
- 3Department of Ocean Systems, Royal Netherlands Institute for Sea Research and Utrecht University, Den Burg, Netherlands
- 4National Oceanography Centre, Southampton, United Kingdom
- 5Senckenberg am Meer, Department of German Centre for Marine Biodiversity Research, Wilhelmshaven, Germany
- 6Center of Natural History (CeNak), Zoological Museum, University of Hamburg, Hamburg, Germany
- 7Centre for Marine and Environmental Research, University of Algarve, Faro, Portugal
- 8Ifremer, REM/EEP, Laboratoire Environnement Profond, Plouzané, France
- 9Alfred Wegener Institute, Helmholtz Centre for Polar and Marine Research, Bremerhaven, Germany
- 10Fugro, Heriot-Watt University, Edinburgh, United Kingdom
- 11Marine Benthic Ecology, Biogeochemistry and In-situ Technology Research Group, The Lyell Centre for Earth and Marine Science and Technology, Heriot-Watt University, Edinburgh, United Kingdom
Mining impacts will affect local populations to different degrees. Impacts range from removal of habitats and possible energy sources to pollution and smaller-scale alterations in local habitats that, depending on the degree of disturbance, can lead to extinction of local communities. While there is a shortage or even lack of studies investigating impacts that resemble those caused by actual mining activity, the information available on the potential long-lasting impacts of seabed mining emphasise the need for effective environmental management plans. These plans should include efforts to mitigate deep-sea mining impact such as avoidance, minimisation and potentially restoration actions, to maintain or encourage reinstatement of a resilient ecosystem. A wide range of mitigation and restoration actions for deep-sea ecosystems at risk were addressed. From an ecological point of view, the designation of set-aside areas (refuges) is of utmost importance as it appears to be the most comprehensive and precautionary approach, both for well-known and lesser studied areas. Other actions range from the deployment of artificial substrates to enhance faunal colonisation and survival to habitat recreation, artificial eutrophication, but also spatial and temporal management of mining operations, as well as optimising mining machine construction to minimise plume size on the sea floor, toxicity of the return plume and sediment compression. No single action will suffice to allow an ecosystem to recover, instead combined mitigation/restoration actions need to be considered, which will depend on the specific characteristics of the different mining habitats and the resources hosted (polymetallic sulphides, polymetallic nodules and cobalt-rich ferromanganese crusts). However, there is a lack of practical experience regarding mitigation and restoration actions following mining impacts, which severely hamper their predictability and estimation of their possible effect and success. We propose an extensive list of actions that could be considered as recommendations for best environmental practice. The list is not restricted and, depending on the characteristics of the site, additional actions can be considered. For all actions presented here, further research is necessary to fully encompass their potential and contribution to possible mitigation or restoration of the ecosystem.
Introduction
Huge progress has been made in the last years toward technologically feasible and economically viable deep-sea mining. Various exploration contracts have been granted in international waters, outside national jurisdiction (by the International Seabed Authority, ISA, see Miller et al., 2018 for an overview) and in national waters (granted by local governments; Secretariat of the Pacific Community [SPC], 2013; Boschen et al., 2016). In 2017, the Ministry of Economy, Trade and Industry (METI) and the Japan Oil, Gas and Metals National Corporation (JOGMEC) succeeded in the world’s first pilot test of excavating polymetallic sulphides and lifting the ores to the surface (Ministry of Economy Trade and Industry [METI], 2017).
The interests in deep-sea exploitation are multiple, covering a wide array of resources and ecosystems, such as polymetallic sulphides at hydrothermal vents, cobalt-rich ferromanganese crusts at seamounts, and polymetallic nodules on abyssal plains. Depending on the resource to be mined, extraction activities will include excavation, cutting, collecting, comminution, slurrification and pumping. While mining techniques differ between resources and ecosystems, many of the potential impacts on the ecosystem are similar (Levin et al., 2016). Impacts are either a direct result of the extraction of the resource or occur following processing activities such as dewatering plumes. As biological resources are often attached to or associated with the mineral ones, any mining activity can be detrimental for the environment (e.g., sessile nodule encrusting fauna, Vanreusel et al., 2016; Fritz, 2016). Furthermore, owing to specific combinations of ecological characteristics (organism size, productivity, etc.), the deep sea may be particularly sensitive to human impacts (Smith et al., 2008; Sweetman et al., 2017).
There has been interest in deep-sea mining since the 1960s, which has resulted in a number of experiments designed to mimic possible mining disturbances to monitor recovery in abyssal nodule fields (see Jones et al., 2017 for an overview). No mining disturbance experiments have been conducted within deep-sea ecosystems other than those associated with nodules. Natural marine eruptions occurring at deep-sea hydrothermal vents and fishery impacts at seamounts may mimic some impacts associated with mining disturbance (reviewed by Gollner et al., 2017). Experiments typically show that it takes decades after the disturbance for signs of recovery to be observed in deep-sea ecosystems, and if faunal abundance does recover, the species that colonise can be different to those that were there previously (Mullineaux et al., 2012). While faunal densities recover more quickly than local diversity, the latter has been observed to remain significantly depauperate even after multiple decades (Gollner et al., 2017; Jones et al., 2017).
The available information on the potential long-lasting impacts of deep-sea mining emphasise the need for effective environmental management plans. These plans should include efforts to mitigate seabed mining impacts. Such mitigation actions can include spatial and temporal management of mining operations, but also engineering solutions (for example in mining collector design and operation) as well as potential restoration activities, measures possibly including deployment of artificial substrates, nutrient enhancement, propagation-and-transplants of fauna, to stimulate post-mining ecosystem recovery. Models of Environmental Impact Assessment (EIA) best practice often adopt a structured approach to mitigation, for example the commonly used mitigation hierarchy described by Ekstrom et al. (2015). The mitigation hierarchy is a framework for managing risks and potential impacts related to biodiversity and ecosystem services, where the first tier is avoidance followed by minimisation, restoration and offsets (Ekstrom et al., 2015; Van Dover et al., 2017). In the current study, as with the mitigation hierarchy, the term mitigation encompasses avoidance and minimisation actions, or actions that help prevent potential impacts, and the intrinsic, compliance or reputational risks that these would pose. Restoration is then used to accelerate the rates of recovery of biodiversity and function and is defined as the process of assisting the recovery of an ecosystem that has been degraded, damaged, or destroyed (Society for Ecological Restoration [SER], 2004). The latter lists ten “Attributes of Restored Ecosystems,” to provide a basis for determining when restoration has been accomplished, which have been adapted to the deep ocean by Van Dover et al. (2014). More recently, the Society for Ecological Restoration has updated the international standards for the practice of ecological restoration, including principles and six key concepts (McDonald et al., 2016). Finally, offsetting assumes that biodiversity loss can be compensated by protecting biodiversity within the same biogeographical region or elsewhere. It encompasses many unknowns, hence it remains ambiguous and appears meaningless or even impossible when applied to the deep sea (Van Dover et al., 2017; Niner et al., 2018).
This four-tier mitigation hierarchy appears to fall short when applied to the deep ocean as the goal of no net loss in biodiversity is not achievable through remediation and offset (Van Dover et al., 2017). Additionally, the high costs associated with restoration in the deep sea could significantly hinder its feasibility (Van Dover et al., 2014). Many knowledge gaps remain for the deep-sea ecosystems, in order to answer or put into practice all attributes to potentially consider an ecosystem as restored. There is a high uncertainty related to impacts, environment and efficacy. Therefore, more research is required to develop cost-effective approaches to deep-sea restoration. The objective of this paper is to provide guidance on possible future mitigation and restoration actions for deep-sea mining. A special focus is drawn on natural regeneration approaches that could potentially facilitate recolonisation of impacted substrates and accelerate the rates of recovery of biodiversity and functions. This paper resulted from a workshop held in 2015 within the framework of FP7 EU MIDAS project (Managing Impacts Of Deep Sea Resource Exploitation - FP7/2007-2013), in which tables with mitigation and restoration actions were drafted, backed-up by literature references whenever possible.
Ecosystems of Interest and Mining Effects
Hydrothermal Vents
Hydrothermal vents will be targeted for Seafloor Massive Sulphides (SMS) also referred to as polymetallic sulphides. While at the time of writing, the extreme risks of mining active vent systems mean that mining organisations are only focussing on exploiting inactive systems (i.e., systems that are no longer hydrothermally active), these technological challenges may be overcome in the future, which is why the authors also considered potential effects in active systems. Moreover, the distinction between active and inactive deposits is not always clear, and rapid switches in activity of deposits (on/off) complicates the definition of active/inactive (Gwyther, 2008). A single mining site is likely to contain numerous active and inactive deposits (Gwyther, 2008; Boschen et al., 2013), thus emphasising the need for mitigation strategies to minimise mining impacts at both active and inactive sites. Mining of SMS deposits edifices and mounds will consist of excavation. Large chimneys or edifices of precipitated metals will be toppled over to collect the deposits (Ishiguro et al., 2013) (Figure 1).
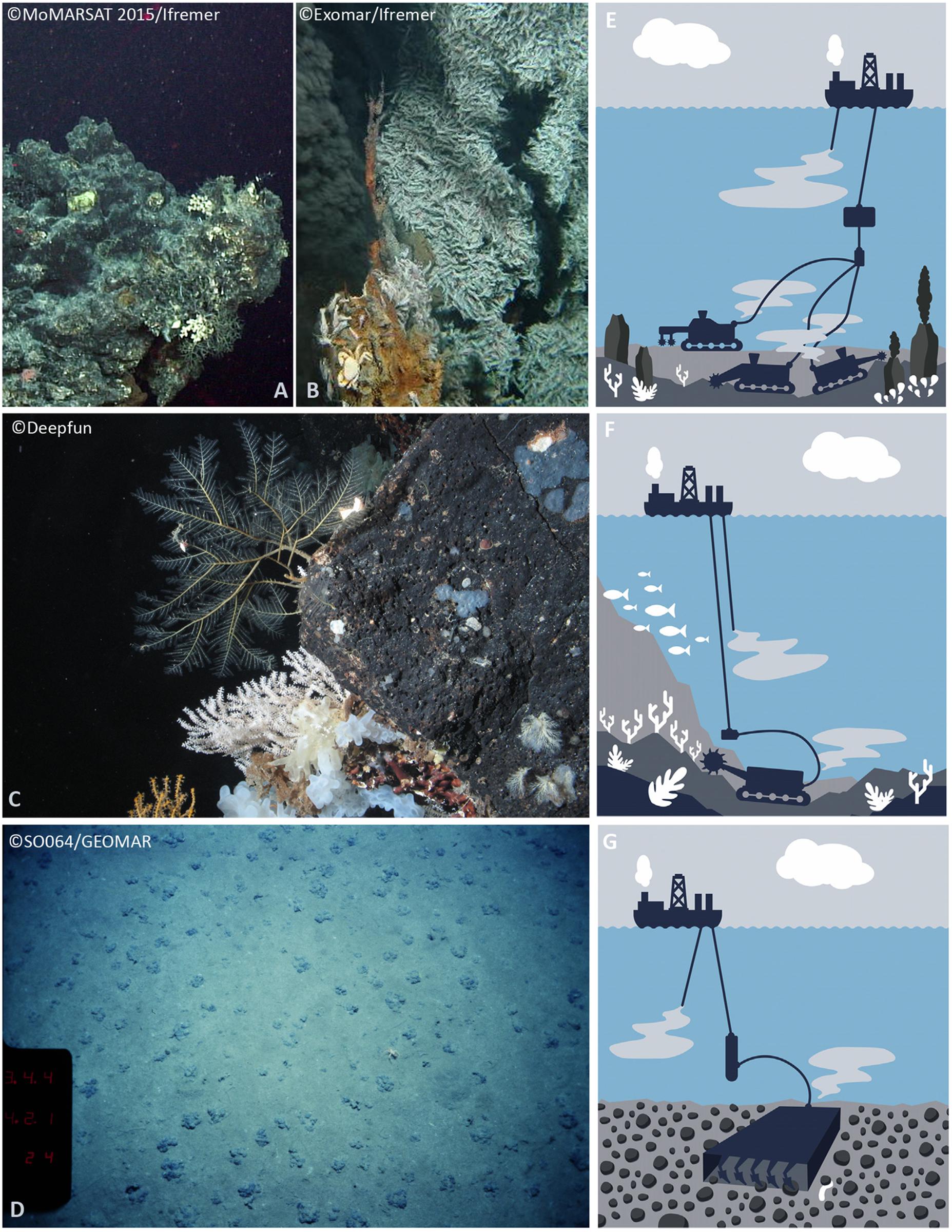
Figure 1. Overview of the ecosystem of interest to mining and examples of associated biological communities at inactive (A) and active hydrothermal vents (B), seamounts (C) and nodule fields (D). Next to each ecosystem, mining scenarios are portrayed for polymetallic sulphides at vents (E), cobalt-rich ferromanganese crusts at seamounts (F), and polymetallic nodule mining at abyssal plains (G) (E–G were taken from Gollner et al., 2017). Maps of licence areas and resource to be mined can be found at https://www.isa.org.jm/maps.
Active sulphide deposits have detectable fluid emissions, feature endemic fauna that rely mainly on chemoautotrophic primary production and are characterised by high biomass, low diversity, and rapid growth rates (Lutz et al., 1994; Van Dover, 2000). Hydrothermal edifices are characterised by mosaics of microhabitats featuring different faunal composition, and changing faunal zonation and composition with increasing distance to hydrothermal activity (e.g., Tunnicliffe et al., 1997; Shank et al., 1998; Waite et al., 2008; Cuvelier et al., 2009; Gollner et al., 2010; Marsh et al., 2012). Within an active vent field, smaller vent sites can have rapid turnover and active and inactive hydrothermal vent sites occur close to each other (Ondréas et al., 2012; Klose et al., 2015). The fauna of inactive sites is poorly studied and may comprise vent-endemic and non-endemic fauna (Levin et al., 2009; Gollner et al., 2013; Boschen et al., 2015).
Inactive deposits are relics of hydrothermal vents and have no detectable emissions. Though they are more abundant than active ones, they are less studied. They host suspension-feeding and grazing invertebrates that may also occur on seamounts (i.e., corals, sponges, echinoderms etc., Tunnicliffe et al., 1986; Galkin, 1997) and other rocky outcrops (e.g., basalt, Van Dover, 2011) (Figure 1). There is still a subsurface microbial population present (Erickson et al., 2009; Sylvan et al., 2012) which may influence colonisation and succession, and remnant vent fauna can be observed as well (Boschen et al., 2015). Inactive sulphide chimneys are long-lived habitats with ages reaching up to 100,000 years (Jamieson et al., 2014).
Seamounts
Seamounts host cobalt-rich ferromanganese crusts which are an important potential sources of metallic and rare earth elements (Hein et al., 2013). These crusts can precipitate on nearly all rock surfaces in the deep ocean that have been swept clean of sediment but are more commonly found on seamounts. Cobalt-rich crusts grow at extremely slow rates of 1–6 mm per million years by the precipitation of metals dissolved in seawater at depths in and bellow oxygen minimum zone between 800 and 2,500 metres (Hein, 2002; Hein and Petersen, 2013; Hein and Petersen, 2013). The thickness of the crust can vary from 1 millimetre to about 260 millimetres and form pavements of intergrown manganese and iron oxides (Koschinsky et al., 2010; Hein et al., 2013), covering an estimated area 1.7 million km2 (Petersen et al., 2016). However, the largest impediment for the exploitation of crusts is likely to be from an engineering point of view, with the challenge of a clean separation of the crust (cut and scraped off) from the rock substrate (Hein and Koschinsky, 2014).
Many seamount taxa are extremely long-lived and grow very slowly, especially those forming biogenic structures such as cold-water corals and sponges, known to be 100s to 1000s of years old (Clark et al., 2010, 2012; Carreiro-Silva et al., 2013). The high productivity at seamounts can support pelagic (Morato et al., 2010) and demersal fish populations and large benthic filter feeders comparable to adjacent deep continental margins at similar depths (Rowden et al., 2010) (Figure 1). Overall, depth and substrate type are key elements in determining the composition and distribution of benthic fauna on seamounts (e.g., Tittensor et al., 2009), while connectivity levels vary substantially between seamounts, resulting in the presence of organisms with very localised distributions as well as widely dispersed taxa (Clark et al., 2010). Verlaan (1992) showed a faunal preference for newly available ferromanganese crusts, but it is not yet clear if cobalt rich crusts may be a factor in structuring seamount communities since no strong differences in the community composition of benthic invertebrates between cobalt-rich and non-cobalt-rich crust sites have been found in the Pacific (Morgan et al., 2015). Contrastingly, benthic assemblages of invertebrates were shown to be structurally different between seamounts located inside and outside a region with cobalt-rich crusts (Schlacher et al., 2014). It is as of yet unknown if there is an effect of the metal components of the crust on microbial or other benthic colonisation.
Abyssal Plains
While the topographies of abyssal plains are predominantly flat, they are also characterised by raised structures (Durden et al., 2015; Leitner et al., 2017). Abyssal plains can host extensive fields of polymetallic nodules, which are of commercial interest for mining (Figure 1). Nodule fields are thought to occur in all oceans, with most commercially attractive deposits thus far situated at abyssal depths (3000–6000 m) in the Pacific and Indian Ocean.
The precipitation of manganese oxides and iron oxyhydroxides forming the nodules is driven by bacterial processes (Blöthe et al., 2015) and the precipitation layers are characterised by different chemical and mineralogical compositions determined by different growth processes (Halbach et al., 1988). Whether this micro-population or metal contents within the nodules influences associated fauna composition is unknown to date. Nodules grow slowly (1–10 mm per million years, Knoop et al., 1998) and offer stable hard surface habitats in the otherwise soft sediment abyssal plains.
Upon collecting nodules, either mechanically or separating them from sediments by using hydraulic water jets (Oebius et al., 2001), all hard substrata will be permanently removed from the abyssal seafloor (Figure 1). The nodule surface is often covered with sessile organisms such as sponges, cnidarians and xenophyophores (all > 1 cm), but also meiofauna, such as nematodes, harpacticoid copepods, tardigrades, and foraminiferan protists inhabit the crevices (Veillette et al., 2007a,b; Glover et al., 2015; Vanreusel et al., 2016; Lim et al., 2017). As nodules are removed during exploitation, all faunal size classes (including demersal scavengers) relying on this hard substrate will be particularly susceptible to mining activities (Vanreusel et al., 2016; Leitner et al., 2017). Amon et al. (2016) estimated that more than half (50%) of megafaunal species in the Clarion-Clipperton Fracture Zone (CCZ) depend on nodules as a hard substrate and that these thus play an important role in maintaining local and regional biodiversity. The density of the megafauna associated with the nodule fields depends on nodule size and density/coverage (Stoyanova, 2012; Vanreusel et al., 2016). In addition to hard substrata removal, the top ∼15 cm of the upper sediment layer containing much of the living biomass/organic material will be removed or resuspended. Nodule fauna may be different to fauna inhabiting the adjacent soft sediments (Mullineaux, 1987; Thiel et al., 1993; Veillette et al., 2007a) and may resemble abyssal areas elsewhere (e.g., Menzel et al., 2011 and references therein).
Mining Effects
Resource collection involves heavy machinery moving around on the sedimented deep-seafloor or excavation and cutting of hard substrata, destroying the local environment while creating primary sediment plumes, alongside other local disturbances (noise, light, sediment compaction). Collected resources are then transported up to the processing vessel and a secondary, post-processing discharge plume is returned to the benthic water column. The stages of the mining process which may cause impacts are grouped into three categories: (1) mining operations at seafloor, (2) resource removal and (3) (post-processing) plume discharge (Table 1). These impacts can lead to the following effects on the ecosystem: mortality of the fauna, habitat loss, habitat modification, habitat fragmentation and other direct impacts or a combination of these effects (Levin et al., 2016) (Table 1). Often these effects are intertwined; for example, habitat loss can lead to habitat fragmentation, and, depending on the scale, to local mortality and/or extinction of entire populations (Didham, 2010). In some cases, (partial) mortality of fauna can shift the faunal presence and composition to locally more adapted organisms, which were not specifically representative for the ecosystem (Gollner et al., 2017).
Mitigation and Restoration Actions
During the November 2015 workshop mitigation and restoration actions to facilitate and accelerate ecosystem recovery following mining activities were discussed. Mitigation actions may potentially minimise impacts and avoid exceeding ecosystem stability tipping points, while restoration actions could be attempted following mining impacts. The general objective of the presented actions serve to contribute to maintain the entirety of ecosystem functioning, as well as the goods and services they provide (Thurber et al., 2014). Actions presented here were not restricted to technical or ecological feasibility or costs. The likeliness and possible complications of the various approaches are addressed. While this list is not exhaustive or exclusive, these actions could be considered but still need further research to fully encompass their potential and contribution to possible mitigation or restoration of the ecosystem.
For All Ecosystems (Table 2)
Independent of the ecosystem concerned, several similar mitigation (avoidance and minimisation) and restoration actions were proposed, which often featured comparable advantages and disadvantages (Table 2). Figure 2 represents several of these actions for the nodule ecosystem. When more specific examples apply for a certain ecosystem, actions are elaborated according to the ecosystem considered (see Hydrothermal Vents (Table 3) and following). Based on the limited body of knowledge of ecosystem processes and interactions currently available, the authors consider predictability for the vast majority of the presented actions to be low. Therefore, the mitigation and restoration actions proposed in this paper will require further evidence to confirm their efficacy for management purposes.
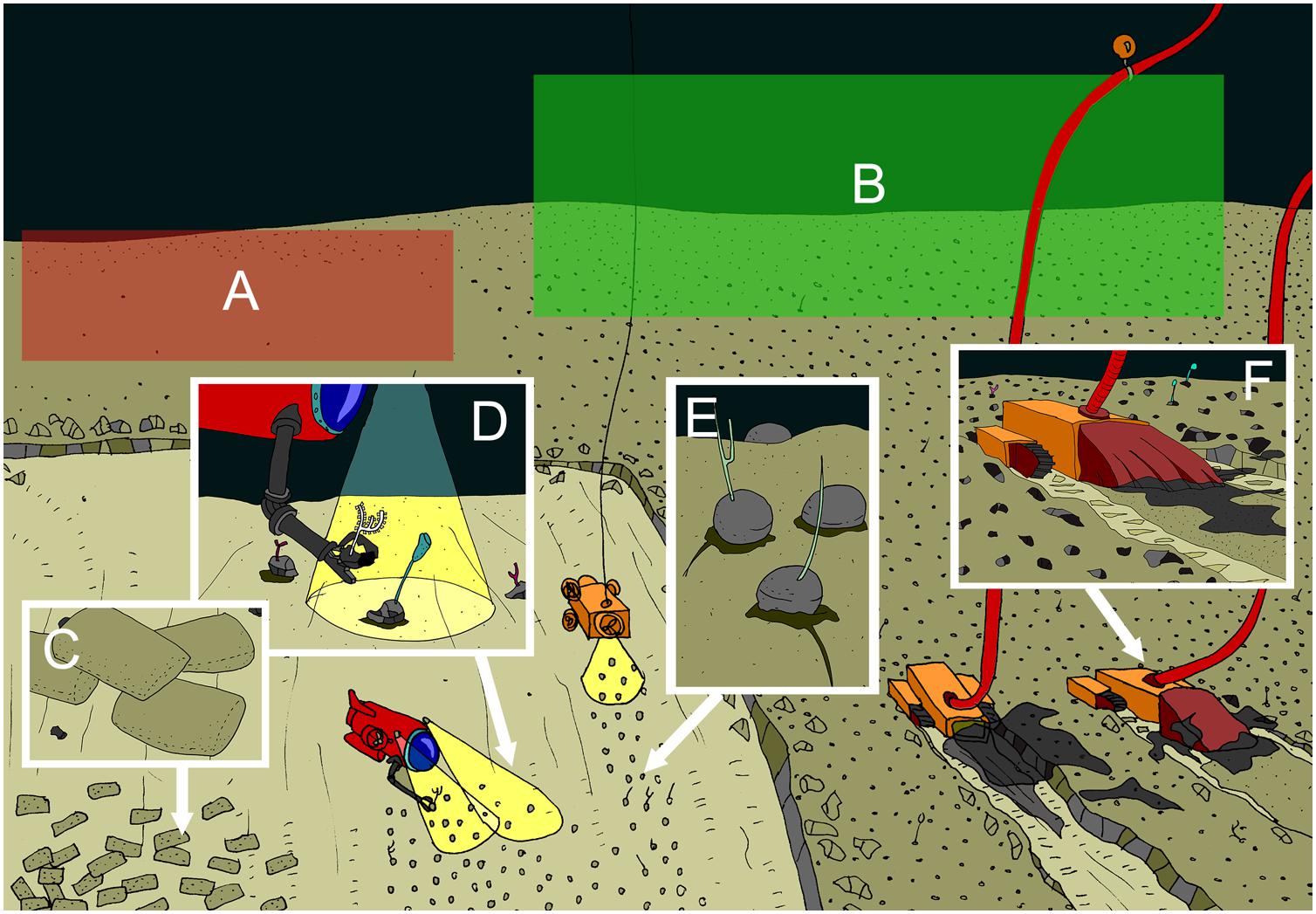
Figure 2. Overview figure (Courtesy of Autun Purser) showing examples of mitigation and restoration actions for the nodule fields ecosystem, with (A) a less suitable set-aside area featuring different nodule densities than the mined area, (B) a more suitable set-aside area featuring nodule densities similar to the mined area. To limit the plume extent, waste sediments (post-processing) can be returned to the deep-sea surface in e.g., as fast sinking bricks, or biodegradable bags and/or in the shape of nodules (C). (D) Faunal transplants and (E) artificial colonisation substrata (in this case artificial nodules (hard substrata) and artificial sponge stalks) are recurrent proposed restoration actions. Clever engineering or vehicle design can limit primary plumes, e.g,. light-weight vehicles equipped with shrouds (F).
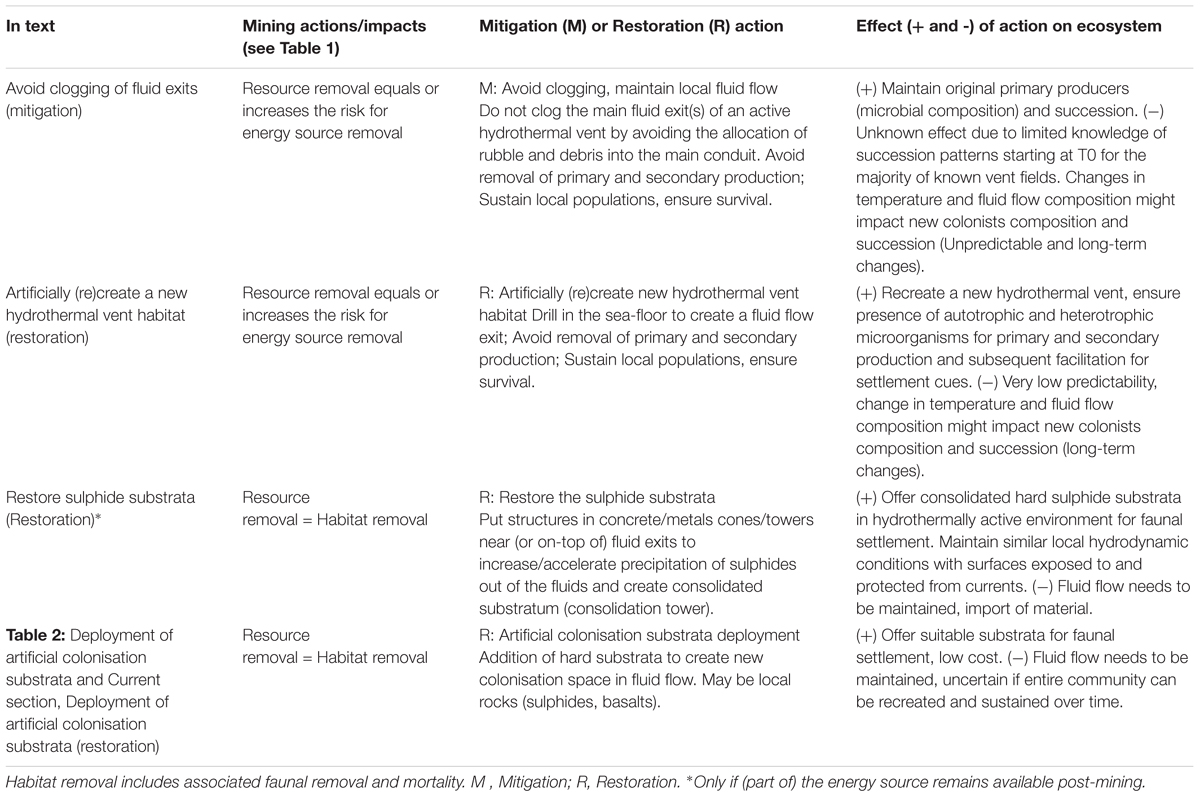
Table 3. Proposed mitigation and restoration actions specifically for seafloor massive sulphide mining at hydrothermal vents.
Mitigation (Avoidance and Minimisation)
Refuge and set-aside areas (avoidance)
Safeguarding areas from disturbances will play a key role in mitigating the impacts of deep-sea mining and will help maintain connectivity, population survival and regional biodiversity. Criteria for selecting appropriate areas include:
(i) Identifying areas with the closest degree of ecological similarity to areas to be mined. To select such suitable areas, a thorough knowledge of community composition and resemblance to the mined area is needed. In areas where local and wider-scale connectivity and meta-population biology are well-understood, areas with a high potential to support similar biological communities may also be considered.
(ii) In less studied, or less understood regions (or areas with high degrees of uncertainty) a precautionary assignment of (large) set-aside areas should be made. With increased size, the possibility for the inclusion of many biological parameters increases, such as a viable population, biodiversity and population sizes (Incorporated Society of British Advertisers [ISBA], 2007; Wedding et al., 2013; Lodge et al., 2014).
The main factors to take into account when selecting set-aside areas in order to increase their effectiveness are representativity, distance, connectivity and size (Gaines et al., 2010; Mora, 2011).
Representativity should be assessed in terms of faunal composition or other community parameters, and potential habitat suitability (Gladstone, 2007; Rice and Houston, 2011). It is critical to consider local characteristics during the selection and identification of representative areas to ensure the long term effectiveness of set-aside areas. Studies have shown that biodiversity of nodule areas appears proportional to the nodule resources present (Vanreusel et al., 2016, Figures 2A,B). Therefore, in order ensure representative areas and communities are protected, it is likely that set-aside areas must also include sufficient areas of high mineral resource density.
Where representative biological communities are associated with areas of mineral distribution of high economic value, trade-offs between mining projects’ short term economic sustainability will need to be considered against longer term ecological sustainability. Proposed mine plans must strike a balance between the two. While representativity is particularly hard to assess (Rice and Houston, 2011), it cannot be a reason to not implement set-aside areas, see the designation of Areas of Particular Environmental Interest (APEI) in the Clarion-Clipperton Zone where distance and size parameters along with other environmental parameters were taken into account (Incorporated Society of British Advertisers [ISBA], 2007; Tilot, 2010; Wedding et al., 2013).
Distance between impact site and refuge area is of prime importance. Refuge areas should be undisturbed and pristine and therefore need to remain outside the wider zone of influence. Nevertheless, a minimum distance between refuge and mined areas should be taken into account to ensure most similar (re-)colonisation as ecological variation tends to increase with distance (Palumbi, 2003; Legendre, 2014). Another approach might be necessary for seamounts, i.e., to protect multiple sites within a region, as adjacent seamounts can display high variations in benthic assemblages (Schlacher et al., 2014; Boschen et al., 2015). Since the plume imprint (primary and secondary) will have a larger footprint, extending far beyond the mining operation itself, the instalment of buffer zones between mined areas and refuge areas should be considered (Wedding et al., 2013). While a minimum distance should be taken into account, a large-enough distance from refuge to the mined site is paramount to make sure it is not impacted by any anthropogenic activity or any of it side effects. For instance, pioneer species were shown to invade the nearby undisturbed communities at hydrothermal vents on the East Pacific Rise, possibly after they became established in vents disturbed by an eruption (Mullineaux et al., 2012). This observation shows the persistence of new colonists but also shows that they could, be it temporary, alter the composition of a designated reference population.
The size of the set-aside area is likely to be at play as well in order to sustain a viable community and ecosystem over time (Botsford et al., 2001; Gaines et al., 2010; Mora, 2011; Wedding et al., 2013; Lodge et al., 2014). A minimum size for a set-aside area is currently unknown but it will depend on the species requirements (Groves et al., 2002). The minimum proportion for a region to be placed in a reserve was set at approximately one-third of the area considered in a number of empirical marine case studies (Airame et al., 2003; Gaines et al., 2010).
While the ecological advantages of (large) set-aside areas are clear, economically viable mining activities will require large and probably continuous areas of high-grade resources.
Plume extent (minimisation)
Exploitation of deep-sea mineral resources will result into two main types of sediment plumes. While primary plumes will originate from the mining activities and mining equipment operating at the deep-sea floor, a secondary plume, resulting from post-processing the ore will be discharged from the vessel into the water column (also known as discharge or de-watering plume) (Figure 1). Both plumes will contain sediments, traces of mined resources and potentially toxic chemicals and can, when settling down, smother neighbouring communities (Copley et al., 1999; Larsson et al., 2013; Nakajima et al., 2015).
The primary plume resulting from the excavation of the resource by the vehicles is not expected to spread over great distances, generating a more localised footprint (Figure 2F). In the case of nodule exploitation, such plumes will quickly lead to high levels of suspended sediments and accumulations of > 1 cm thick deposited particulates > 5 km away from the mining activity within 10 days, and 1 mm thick deposits > 50 km after 1 year (Aleynik et al., 2017), which are >1000 times background sedimentation rates. Designing mining tools to reduce the impacts of primary plumes, for example with shrouds to contain plumes, to increase the settlement rate of produced particulates, or to reduce the compaction effects on the seafloor could reduce the impacts of mining significantly (Figure 2F).
The imprint of the secondary plume is expected to be much larger and even in the case of SMS and nodule mining may extend 5–10 km and 50–100 km from the mining site (Ledwell et al., 2000; Rolinski et al., 2001; Gwyther, 2008). Moreover, the mining plume may stay suspended for years depending on the depth of discharge (Rolinski et al., 2001). This dewatering plume will most likely be discharged closer to the seafloor but it has, depending on its density and temperature, the potential to rise up into the water column, thus expanding its 3D footprint. Discharging the water along long horizontal or vertical pipes at different points in the water column could increase dilution and decrease overall flow to minimise disturbance of benthic fauna and minimise concentrations for pelagic fauna, although the extent of the imprint will be subject to changes (e.g., hydrodynamics) and the duration of the plume will be longer the higher up in the water column it is discharged (Rolinski et al., 2001).
All organisms, benthic, pelagic and of all sizes, will experience negative effects from plumes created by mining operations and thus will benefit from plume impact minimisations. Depending on the depth and content of the plume, fertilisation, eutrophication and acidification of sea waters can be expected (e.g., Gilmour, 1999; Phillips and Shima, 2006; Hauton et al., 2017). Changes in sediment composition, increase of organic input and increased aggregation lead to changes in the water column. Increased sinking rates through the eutrophic zone could cause planktonic organisms to starve. Related to changes in the water column are the role of isoclines and pycnoclines, which might “trap” plumes between water layers or work as a buffer not allowing further dilution/sinking across depths.
The extent of plumes and their toxicity can be minimised either by controlling the composition of the discharge plume or by influencing and limiting its dispersal. Following actions impact plume characteristics or limit its imprint (see Table 2).
(i) Define size of the discharge particles. The size of the discharge particles is currently set at 8 μm, retaining all larger particles on board the mining support vessel (Gwyther, 2008). Very fine discharge particles sink very slowly and while it may increase the settlement potential for colonists, it could possibly smother the feeding apparatus of philtre feeders (e.g., corals, Larson and Purser, 2011). Changes in the biogeochemistry and dilution of food concentrations in the water column can occur as well. It may also be possible to determine the optimal size distribution to (re)deposit the sediments over the seafloor or the mined tracks.
(ii) Adapt temperature/salinity of discharge plume. Since the temperature of the discharge plume will probably be higher than the surrounding water (Gwyther, 2008), this should be mitigated. Generally, deep-sea species have low tolerances to changes in temperature, salinity and O2 (Sweetman et al., 2017). If the temperature/salinity of the dewatering plume could approach ambient seawater temperatures the impacts would likely be smaller. In addition, such alterations could alter the dispersal of the plume, reducing the overall spatial footprint.
(iii) Inject discharged sediment into seafloor sediment. Even though this action would minimise the discharge plume, injecting sediment into seafloor sediment is likely to cause a plume as well by resuspending the top layers. Besides disturbing the infauna, negative effects on the ecosystem are unclear. Instead, the discharged sediment may be released close to the surface area or close to the sediment surface in order to avoid long-distance travel of sediment particles. Impact may be larger but on a smaller area, and may be combined with areas already impacted by mining.
(iv) Manipulate waste sediments after processing to increase precipitation of toxicants (see example of tailings in Ramirez-Llodra et al., 2015). Preference would be given to electrical processes over chemical reactions, because the latter would add additional substances to the ecosystem which could have the potential to be harmful. Waste sediment discharge could be done on land. If sediment discharge at sea is necessary, discharged sediments with low toxicity could be compacted into rapidly sinking bricks or lumps (Figure 2C). By separating the sediment form the discharge plume, the latter will be minimised. However, negative effects can be multiple and size-dependent, since large compacted sediment blocks may harm organisms through smothering or squashing. Also, larger blocks will cause hydrodynamic conditions near the seafloor to change and may thus change seafloor structure. In addition, the current lack of knowledge constrains the definition of a priori toxicity limits.
(v) Biodegradable sediment bags could be used to bring waste sediment down to the seafloor (Figure 2C). Whilst this action would definitely aid in minimising the discharge plume, it also will add relief (e.g., mounds) to the deep-sea floor, alongside smothering of local fauna wherever the bag lands and will add carbon to the soft-sediment seafloor.
(vi) Enhance surface net primary production [by adding in nutrients such as Fe (Bonnet et al., 2008)]. The organic material generated by this action will then be discharged with the sediments. The enhanced food input will reach the seafloor and could facilitate species recovery, since the upper sediment layers and biomass contained therein will be removed by the mining machine. The addition of organic material in excess at the bottom might raise problems through fertilisation, eutrophication, hypoxia and acidification.
(vii) Deploy (natural, non-toxic) flocculants to minimise plume extent. Whilst this action would minimise the dispersal of the secondary plume, it also encompasses a lot of risks such as changing sediment composition, increase of organic input, increase aggregation in the water column which in turn leads to increased cleaning of the water column. If sinking rate of all particles is altered, they can sink too fast through the eutrophic zone and cause starvation of plankton organisms.
Spatio-temporal restrictions (minimisation)
Considerate spatio-temporal planning of mining actions could mitigate the impact for certain ecosystem components. Seasons or periods, during which mining should be restricted or avoided, should be identified as well as a maximum duration for mining activities. Since intended mining activities will last several decades and over large areas, maximum duration and alternating “resting periods” in which no mining activities will take place will be important for management planning [see Refuge and set-aside areas (avoidance)]. Mitigation strategies for pelagic communities can encompass seasonal “closures” according to spatial distribution and migratory patterns of key species. Hence, essential habitats for reproduction, feeding, etc. can be maintained.
It is important to bear in mind that not all patches (or exploitation areas) are impacted in the same way at the same time. If time between mining actions is long enough, unmined patches may serve as source populations for (re)colonisation. However, breaks or “resting periods” in between mining activities are useless if they do not consider specific phenology and life cycles of species. Depending on previous activity and resistance of the local communities (Gollner et al., 2017), these resting periods could extend up to multiple years/decades, to be decided upon based on yearly monitoring programs. This action also includes preventing further anthropogenic activities at mined areas in order to avoid cumulative effects.
Lights and noise (minimisation)
Besides the destruction of the local habitats to collect the ores, other impacts of the mining action, independent of the ecosystem, consist of noise and light disturbance in an otherwise dark deep-sea ecosystem. Noise is likely to affect both pelagics and benthos, fish and invertebrates and can manifest itself in the organisms’ behaviour, anatomy, development and physiology (Aguilar de Soto et al., 2016; Weilgart, 2018). For instance, sediment vibrations were shown to adversely impact benthic invertebrates (crustacean and mollusc) by eliciting changes in behaviour and physiology and even cause physical damage (Roberts and Elliott, 2017). The type of noise and the frequency will play a role but to date few estimates have been published on noise emitted by mineral extraction (Kaikkonen et al., 2018). Lights may attract or scare certain organisms, e.g., fish or swimming crustaceans (Aguzzi et al., 2010; Cuvelier et al., 2014; Vanreusel et al., 2016). Wherever possible, mining vehicles should be designed to reduce these impacts as much as possible. Additionally, periods of active mining should be interspersed with pauses [see Spatio-temporal restrictions (minimisation)]. By reducing impacts of light and noise, benthic and pelagic communities will likely benefit either through increased survival, animal condition, reproductive success and recruitment. This type of measure will inevitably reduce the time allocated to mining.
Restoration
Deployment of artificial colonisation substrata
Artificial substrata can be used to provide additional suitable habitats for fauna to occupy when original habitat has been removed by mining activities (Figure 2E). It can also contribute to maintain connectivity between sites separated by large distances.
In chemosynthetic habitats, all experiments that deployed artificial substrata were done in the presence of active venting in order to answer diverse ecological questions unrelated to mining impacts (Van Dover et al., 1988; Mullineaux et al., 1998, 2003, 2009, 2010, 2012; Tunnicliffe, 1990; Govenar and Fisher, 2007; Kelly et al., 2007; Kelly and Metaxas, 2008; Pradillon et al., 2009; Ivanenko et al., 2011; Gaudron et al., 2012; Gollner et al., 2013, 2015; Cuvelier et al., 2014; Zeppilli et al., 2015; Plum et al., 2017; Baldrighi et al., 2018). To date, there has been no experimental deployment of colonisation of substrata in absence of a local source population (and activity).
The success of the use of artificial colonisation substrata at hydrothermal vents relies on the initial successful microbial colonisation and arrival of new colonists (Mullineaux et al., 1998, 2003). Increasing distance from a heat source allows increased colonisation and intrusions of background fauna which may alter species composition and interactions (Mullineaux et al., 2000, 2003; Micheli et al., 2002; Cuvelier et al., 2014). A couple of studies deployed substrata on basalt or young inactive vents (Van Dover et al., 1988; Mullineaux et al., 1998; Gollner et al., 2013, 2015; Plum et al., 2017; Baldrighi et al., 2018). However, basalt and inactive sulphides (possible target for mining) are two very different habitats for organisms to occupy and do not allow for generalisation among them.
Only one colonisation experiment was carried out at seamounts, comparing the colonisation rates on newly provided ferromanganese (Fe-Mn) crusts vs. basalts where fauna showed an 80% preference for crusts (Verlaan, 1992). Whether this preference was caused by the metal presence or associated (microbial) processes or rugosity remains to be investigated.
There have been no hard substrata deployments within or adjacent to nodule fields or abyssal plains [see further Abyssal plains – Nodules/Deployment of colonisation substrata (Restoration)]. Here as well, the possible role of metals, substratum rugosity or microbial colonists of the nodules in the success of new faunal colonisation is unknown. In addition, larval settlement on hard substrata in the deep sea (<1500 m) has been shown to be influenced by local currents and hydrodynamics (Mullineaux, 1988; Mullineaux and Butman, 1990).
However, even if deployment of artificial substrata could be used as a mechanism to assist restoration, the question becomes how to estimate the square (100s of) metres (or kilometres?) of surface to be provided or the number of substrata necessary to sustain a viable population. And, moreover, how to deploy the assumedly vast numbers required (e.g., by ROV) in a way that avoids further ecosystem disturbance and excessive cost. For the nodules, assuming an average nodule density of 10 nodules m-2, 1.5 × 1011 nodules would be required to replace nodules from a 15000 km2 mining area. For comparison, mean nodule abundances in United Kingdom licence area in CCZ ranged from 84 – 197 nodules/m2, depending on size (Amon et al., 2016) and ISA exploration contracts can cover areas (not necessarily contiguous) of 75000 km2 of which 11% (8500 km2) should, depending on the yield, support several years of nodule harvesting (Madureira et al., 2016).
Overall, knowledge is still too limited to fully appreciate the potential of introducing artificial substrata as a restoration action, although it represents an affordable and straightforward experiment with minimal effort that could be initiated or carried out very early on in the exploration phase. Such experiments will allow us to fill in the caveats in our knowledge regarding the provision of artificial substrates (e.g., hard substrata provision in soft sediment deep sea). Composition of substrata, which material, texture, rugosity etc. to be used to increase success rates is likely to be ecosystem dependent. Priority should be given to natural materials (e.g., rocks) instead of artificially-produced materials (e.g., plastics).
Transplants (include seeding/larva showering)
The transplant or translocation of fauna and/or sediments has been proposed for all ecosystems (Figure 2D), although the possible negative impacts this action may have on particular ecosystems may outweigh the benefits at this early stage of research.
Two scenarios are envisioned, where (i) species or assemblages are transplanted or translocated away to nearby undisturbed localities before the mining process starts and are transplanted back to the site of origin once the disturbance ceases, or (ii) undisturbed fauna and sediments are implanted on the post-mined site to kick-start (re-)colonisation. The establishment of already ecologically functional assemblages, including facilitators for subsequent succession, can promote faunal restoration. Also provision of secondary surfaces by larger sessile organisms (e.g., mussel beds, corals, sponges) could enhance faunal settlement, increase biodiversity and survival of associated fauna (Menge and Sutherland, 1976; Tunnicliffe et al., 1997; Sarrazin and Juniper, 1999; Van Dover and Trask, 2000; Sarrazin et al., 2002).
The main issues for transplantation are where the fauna and sediments will be imported from and where they will be transplanted to, respecting natural distribution patterns and local community composition. Depending on distance between the ‘harvesting’ site and the receptor site, deep-sea organisms will be brought up to the surface for transportation, which will only be possible for organisms with a robust constitution, able to withstand the hydrostatic pressure change (Company et al., 2007). Besides damage occurring during transport (see effects of coenosarc damage in corals, Larson and Purser, 2011), caution is needed to ensure new organisms or invasive species that could cause an unbalance in the ecosystem equilibrium are not transported to the intermediate receptor site or final receptor site. No transplants over large distances, across depth gradients, transform faults and other topographical barriers for dispersal are advisable, in order to respect existing gene flow pathways and patterns of genetic diversity (Won et al., 2003; McClain and Hardy, 2010). The presence of a microbial population, its composition and diversity of the transplants needs to be considered as well. Overall, there is a low predictability of this action and it might only work for certain species with a certain degree of physiological plasticity. There is no or very little knowledge on adaptation times from transplanted fauna (Bergquist et al., 2004).
Similarly, by showering a site with larvae, the re-colonisation of the benthic community may be enhanced by promoting the presence of early life stages. Such seeding strategy has been successfully developed to rescue shallow water coral reefs suffering from climate change effects (Nakamura et al., 2011; dela Cruz and Harrison, 2017). Larvae were obtained from laboratory culture using adults from healthy populations, and transplanted to impacted areas where they settled, grew and reached maturity. Knowledge on the life cycle of organisms and possibility to culture them in laboratory conditions are prerequisites that make such action rather challenging for deep-sea organisms. Although a number of them, mostly from vents and seeps have already been cultured (Miyake et al., 2010 among others), predictability rates are low, since settlement success will depend on local environmental conditions (such as local hydrography), geomorphology and presence of other organisms. Risks of alterations to the original community or reduction of genetic diversity remain main threats of this action.
Add in organic material
Loss of entire populations includes loss of primary and secondary producers that other fauna rely on. By adding organic material (e.g., key nutrients or baits) on the deep-sea floor, food availability can be guaranteed and can attract fauna from all size classes. Key nutrients can promote growth in early life stages. The instalment of a good functioning benthic community can lure in large predators and fish. Increased food availability favours growth and reproduction and accelerates succession. However, if the input of organic matter is too high, or the oxygen concentration is too low there are risks of hypoxia and/or acidification. While predictability of effects is low, all fauna could either benefit or suffer if this measure is implemented. At abyssal plains, increased flux of organic matter was shown to cause major changes in all size fractions of benthic communities, mostly resulting in increased abundances but reductions in body size for megafauna (Billett et al., 2001, 2010). There appears to be a positive relation between organism size and uptake of organic material (Stratmann et al., 2018), though certain taxa and size classes showed retarded responses to similar fluxes or food pulses (Witte et al., 2003; Sweetman et al., 2009). Reactions of different faunal size classes to food pulses may be very variable and some fauna may be outperformed by opportunistic feeders which will result in subsequent community changes.
There is quite some literature available on the use of baited lander deployments and food falls (Higgs et al., 2014; Smith et al., 2015), which can be used to lure in mega- and macro-fauna, be it temporary. Baits may be removed by large marine predators prior to serving its purpose. Efficiency of the current action would decrease if the timescales needed for initial recolonisation of primary and secondary producers are too long or if they would only be triggered after initial recolonisation starts.
Hydrothermal Vents (Table 3)
Mitigation and Restoration for Energy Source Removal
One of the most important structuring factors for the composition, distribution and dynamics of deep-sea hydrothermal vent assemblages is the high spatial variability of biotic and abiotic factors related to hydrothermal vent activity and more specifically, the chemical composition and flow intensity of the vent fluids (Hessler et al., 1988; Tunnicliffe, 1991; Sarrazin et al., 1997; Shank et al., 1998; Desbruyères et al., 2000, 2001; Luther et al., 2001; Govenar et al., 2005). Consequently, alteration of fluid composition, intensity of flow or cessation in fluid flow causes small-scale disturbances on short time-scales and can initiate significant faunal changes (Hessler et al., 1985, 1988; Fustec et al., 1987; Tunnicliffe, 1991; Sarrazin et al., 1997, 2002; Shank et al., 1998; Desbruyères et al., 2000). Hence, interruption, cessation or alteration of fluid flow will severely impact faunal communities as it equals the removal of the (local) energy source and of primary and secondary producers.
Avoid clogging of fluid exits (mitigation)
In order to maintain the local fluid flow during mining actions, the clogging of the main conduits should be avoided. Allocation of rubble or debris into main conduits should be prevented by provident mining actions. This mitigation action allows survival of the original microbial population associated with the vent fluids and pioneer colonisers, which represent the ‘corner stones’ of the communities. Original microbial composition living in the subsurface flow could allow for the most similar colonisation and succession patterns, be it microbial, meio-, macro- or megafaunal. If local fluid exits get clogged, fluids will get expelled elsewhere, but their biogeochemical composition will be different, as will subsequent colonising megafauna (Nakajima et al., 2015; O’Brien et al., 2015). The impossibility to estimate response time for new organisms to arrive, the lack of knowledge on the time needed for full or partial recovery and the (dis)similarity to the original population are disadvantages. Time necessary for recovery will be subject to, among others, local variations, spreading rate and distance to other inhabited active vent sites.
Artificially (re)create a new hydrothermal vent habitat (restoration)
Improvident mining activities could easily compromise or shut down fluid flows by clogging the main conduits. New vent habitats could be recreated by drilling holes to ensure fluid flow and allow for vent fauna to recolonise the newly created sites. There is some information available on the effect of Ocean Drilling Program (IODP) drilling exercises at hydrothermal vents, though results are not conclusive. Copley et al. (1999) showed redistribution of nearby shrimp populations to the newly created fluid exits (at TAG 3680 m depth, MAR). Contrastingly, the faunal composition changed for the long-term following drilling actions in Iheya North field (Japan, 1000 m depth, Nakajima et al., 2015). The general outcome of a drilling action is unknown, hard to predict and will depend on many local environmental factors and variations. For instance, reaction time or differences in response of fauna might be due to motility and trophic position and is hard to predict (especially for more sessile organisms; differences between shrimp and anemones were described by Copley et al., 1999). Also, the reactions of nearby fauna to recolonise newly active fluid exits might be temporary (Copley et al., 1999). When combined with the transient nature of drilled holes (past IODP drilling holes that are no longer active), they may be unable to sustain long-term survival of the fauna. There is a lack of data and gaps in knowledge on natural succession patterns.
Mitigation and Restoration for Habitat Loss
Mining of SMS implies local habitat removal and for the hydrothermal edifices, removal of the 3D structure inhabited by the faunal assemblages (relief removal). Removal of, often colonised, substrata means removal of the available habitat for animals to settle on. In the case of active hydrothermal vents, the substrata that are colonised have a specific composition and porosity (Sarrazin et al., 2002).
Along with the local habitat removal, the loss of entire populations/communities/assemblages due to mining actions is unavoidable and will increase distance between neighbouring vent sites and fields, disrupting faunal survival, connectivity and gene flow. If local biodiversity is to be maintained chances of survival need to be increased to restore sustainable populations.
Refuge areas were discussed in Section “Refuge and set-aside areas (avoidance).”
Restore sulphide substrata (restoration)
If fluids are still expelled following a mining action, minerals will start to precipitate straight away due to the steep temperature gradients between hot fluids (∼300°C) and cold ambient sea water (2–4°C). However, these newly formed chimneys are friable, porous and unsuitable for immediate (re-)colonisation (Tunnicliffe and Juniper, 1990). Sessile fauna is unable to attach to such friable substrata. The hot vent fluid seeping through the porous freshly precipitated substrates, makes them even more unsuitable habitats. Time needed for consolidation and subsequent colonisation of a hydrothermal site is unknown (exact time-scales depend, among others, on local environmental conditions, depth, geographical locality and spreading rate).
This restoration action would consist of a cone or tower structure positioned on-top of an active fluid exit (where fluids are being expelled), enhancing sulphide precipitation and consolidating the freshly precipitated anhydrites to make them more stable, less porous and suitable for colonisation (faunal settlement and attachment). The material for the consolidation structure to be used should be able to resist continuous high temperatures and the chemistry of the vent fluid but without influencing the local communities or new colonists. A suitable porous medium was proposed by Nozaki et al. (2016) or high temperature resistant titanium was shown to be readily colonised by microorganisms and metazoans (Alain et al., 2004; Pradillon et al., 2005, 2009). By assisting in the reconstruction of 3D edifices and local reliefs, substratum provision for faunal/larvae settlement and exposure of the new surfaces to nutrient flow might facilitate subsequent colonisation. However, the faunal response remains hard to predict as well as the time-scale needed for possible (re-)colonisation and recovery [years to decades for faster spreading ridges and no current knowledge for slower spreading ridges but likely to be (much) longer, see Gollner et al., 2017]. Despite some modelling attempts of microbial reaction rates in submarine hydrothermal chimneys (LaRowe et al., 2014), there is still a lack of knowledge of the mineralogy of the new structures as well as the microbial turnover over time, especially if exposed to fluid flow. The interaction of mineral and microbial processes is of recognised importance (Breier et al., 2010). There are no previous studies available of this kind.
Deployment of colonisation substrata (restoration)
The use of artificial colonisation substrata as “stepping stones” could aid in population survival and sustainability at hydrothermal vents, and contribute to larval survival or dispersion (Metaxas and Kelly, 2010; Génio et al., 2013; Cuvelier et al., 2014).
When deployed adjacent to a source population and hydrothermal activity, all substrata tend to get colonised, independent of the material used. However, the composition of substrata needs to be taken into account based on objective and if specific taxa or communities are targeted for restoration. The use of slates, plastics and organic substrata should be avoided for a multitude of reasons, going from unable to recruit certain taxa (slate and siboglinid polychaetes, Van Dover et al., 1988; Tunnicliffe, 1990) to less sustainable materials (plastic) and transient nature of the substrata (decomposition of organic materials) (Cunha et al., 2013). Possible eligible hard substrata can be local rocks (e.g., sulphides, basalts) (Van Dover et al., 1988; Mullineaux et al., 1998, 2003; Shank et al., 1998; Kelly et al., 2007; Kelly and Metaxas, 2008; Gaudron et al., 2010).
There is no knowledge on the deployment of colonisation substrata in absence of a known nearby source population (within several m’s or occasionally few hundred metres). Consequently, the possible success rates of substrata provision in absence of a local hydrothermal vent population (and activity) are unknown.
Seamounts – Fe-Mg Crusts (Table 4)

Table 4. Proposed mitigation and restoration actions specifically for Fe-Mg crust mining at seamounts.
Mitigation and Restoration for Habitat Loss
When crusts are removed through mining actions, associated animals are removed as well and there will be a substantial loss in habitat. Whether the cobalt-rich ferromanganese crusts play a role in faunal composition is not yet clear, though benthic assemblages of invertebrates were shown to be structurally different between seamounts located inside and outside a region with cobalt-rich crusts (Schlacher et al., 2014). Verlaan (1992) showed a faunal preference for newly available Fe-Mn crusts. Seamounts also provide essential habitats for benthic and pelagic animals, such as corals, fish, seabirds and turtles (Clark et al., 2010). Since there is a more pronounced link between seamount and pelagic components (Morato et al., 2010), the refuge areas [see For all ecosystems/Refuge and set-aside areas (avoidance)] should be extended into the water column with possibilities of seasonal closure, in order to take into account migratory patterns and nursery function or reproduction areas for fish and other large marine predators [see For All ecosystems/Spatio-temporal restrictions (minimisation)]. There is a high variation in benthic assemblages found between seamounts located within the same area (Schlacher et al., 2014). Protecting one seamount to enable mining at an adjacent seamount may not be a suitable strategy, rather it may be necessary to protect multiple seamounts or a network of sites to conserve the suite of assemblages present (Boschen et al., 2015).
Seamounts are characterised by long-lived species, hence transplant actions might be of particular interest in this specific ecosystem [see For all ecosystems/Transplants (include seeding/larva showering) and Table 2].
Increase rugosity of mined substrata (restoration)
Hard substrata, their rugosity and associated habitat complexity have been shown to play an important role in the success of larval settlement (Rogers, 1999). The post-mining process could include a mechanistic increase of substrate roughness/complexity to promote larval settlement and recruitment. Advantages of this action would be to promote early colonisation stages of benthic communities, although the outcomes are uncertain.
Abyssal Plains – Nodules (Table 5)
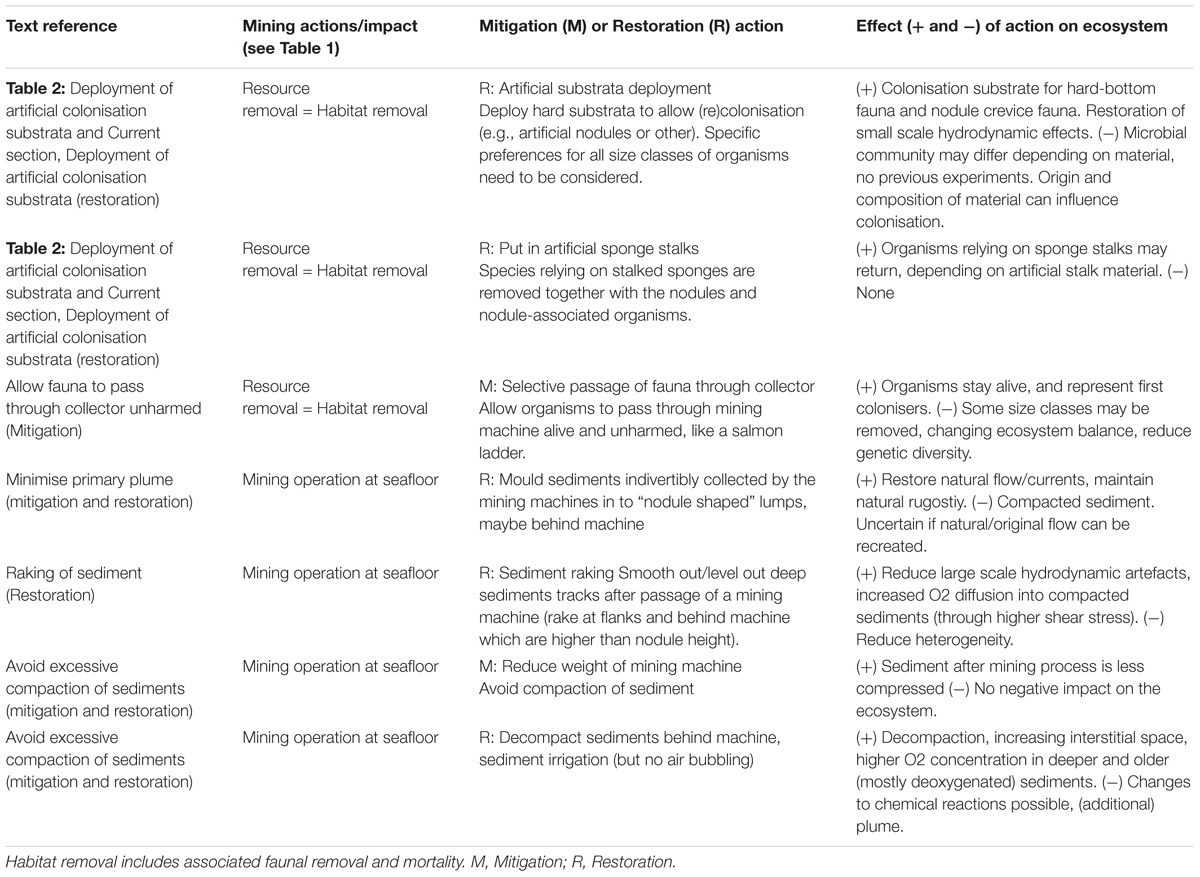
Table 5. Proposed mitigation and restoration actions specifically for Mn nodule mining at abyssal plains.
Mitigation and Restoration for Habitat Loss
Nodule fields are characterised by different nodule densities and size of nodules with correlated faunal composition (Vanreusel et al., 2016). These differences need to be taken into account when identifying possible refuge areas [see For all ecosystems/Refuge and set-aside areas (avoidance).]. Nodules are also found in the sediment, so restoration would need to restore within sediment heterogeneity as well.
Deployment of colonisation substrata (restoration)
Artificial nodules (Figure 2E) – Since a mining machine will remove all hard substrata (nodules) on an otherwise soft-sediment abyssal plain, the deployment of artificial hard substrata could provide compensatory hard substrata for the fauna to colonise as well as recreating favourable small-scale local hydrodynamics. Main difficulty, putting its feasibility in cause, is the vast numbers of nodules that supposedly need to be deployed to reseed an area (see Section “For All Ecosystems/Deployment of artificial colonisation substrata” for estimated numbers) and their deployment. These artificial nodules cannot be dropped from the ship as they would sink into the sediment when hitting the seafloor, but one by one deployment by an ROV implies many years spent on the bottom to put them in place. Other disadvantages are that the material to shape the artificial nodules has to be imported from elsewhere and may introduce additional disturbance to the system. The artificial material should also meet several requirements to ensure that its characteristics will match those of real nodules (e.g., porosity). All size classes of nodule associated organisms and their specific preferences for substrata selection should be considered. Although it is not yet clear whether the local metal content influences the type of organisms (crevice fauna and macrofauna) present on a nodule, the colonising microbial community might be different according to the material used, influencing faunal colonisation. To date, however, only two hard substratum deployments have been carried out on soft sediment deep-sea floor (<1300 m depth) (Mullineaux, 1988; Girard et al., 2016) but not in the vicinity of a nodule field. No information is available on the possible success of such substrata provision in the vicinity of the nodule fields or on deep abyssal plains as a whole.
Artificial stalks (Figure 2E) – Next to the nodule associated fauna, the animals colonising organism stalks (e.g., sponges, cnidarians, crinoids) will also be removed by mining. There is some information available on the importance of sponge stalks for the fauna associated with these stalks, or their spicules, being important for increasing local biodiversity (Bett and Rice, 1992; Beaulieu, 2001). Sponge stalks take a long time to grow (Leys and Lauzon, 1998) and sponge densities were still severely diminished and nearly absent 26-years post-disturbance at the DISCOL site (Purser and Simon-Lledo, unpublished data). One single experiment has been carried out deploying artificial sponge stalks, constructed of basal root tuft spicules from sampled Hyalonema sponges tied together with plastic strips, which successfully recruited several taxa present in stalk communities (Beaulieu, 2001). Part of the stalk-colonising organisms could thus be restored, although alternative materials for stalks, not relying on sponge spicules (e.g., fibreglass on cement flagstone to avoid use of plastics), needs to be tested to see if they have similar successful outcomes.
Allow fauna to pass through collector unharmed (mitigation)
Upon removal of upper sediment layers, all associated soft sediment organisms are removed as well. Being entrained by a collector will likely cause serious harm to most organisms across many size classes. Dredging has been shown to cause damage on soft bottom macrobenthic species and the severity of the injuries was shown to be related to the organism’s morphology and fragility (Gaspar et al., 2002). However, the most resistant taxa could survive. Samples taken from dredger hoppers show that varying proportions of fishes, crabs and other invertebrates can survive entrainment (Stevens, 1981; Armstrong et al., 1982; Larson and Moehl, 1990; Lees et al., 1992). By allowing organisms of certain size class (e.g., macrofauna) to pass through the mining machinery (like a salmon ladder), survival rates for some soft sediment organisms could be increased. Through this action, organisms could stay alive and provide “new colonisers” in the post-disturbance sediments. However, it is impossible to accurately assess the survivorship of the organisms as well as their condition after being passed through a dredge or, in this case, mining vehicle (Newell et al., 1998, 2004). Little is known about the longer term survivorship of entrained individuals, experiments on dredged Dungeness crabs have demonstrated that some individuals will survive more than 60 h after dredging (Stevens, 1981). Survivorship will be critical to all successful restoration efforts. Additionally, the ecosystem will be altered after mining (see the following section on Mitigation and Restoration of Impacts Caused by Mining Equipment and Processing), which creates a very low degree of predictability of success and a possible dominance of one or two resistant members of the original community (Newell et al., 1998). Through the selective passing of animal size classes, some of these size classes or less resistant taxa would not be retained, and therefore would be removed from the surviving group with the potential to restore the ecosystem.
Technological challenges associated with this proposed mitigation action are possibly high. However, pumping systems for other applications are designed to reduce faunal injury and mortality e.g., for fish: wellboat fish transport systems in the fish farming industry (Lyngstad et al., 2015) and pumping station (for water supply and management) (Vis and Kemper, 2012). Experience from these and other pumping applications could inform the development of collector designs to maximise the return of unharmed benthic fauna to the seabed.
Mitigation and Restoration of Impacts Caused by Mining Equipment and Processing
Considerate spatio-temporal planning of mining actions and designation of refuge areas is addressed above [see For all ecosystems/Spatio-temporal restrictions (minimisation)].
Minimise primary plume (mitigation and restoration)
The primary plume caused by the mining vehicle and the excavation activity could be partially controlled through vehicle developments, with the smaller machines creating only a small plume or even retaining the resuspended sediment [see section For all ecosystems/Plume extent (minimisation)], e.g., by the use of shrouds (Van Dover et al., 2017) (Figure 2F). Another option would be to mould the sediments into “nodule shaped” lumps which can be released behind the machine. Advantages are that natural small-scale flow and currents are readily restored and the natural rugosity is maintained. Filter feeders, microbes, meio-and macrofauna could benefit from this. Disadvantages encompass the compaction of the sediment to mould them into nodules which would impact microbes, meiofauna and epibenthic fauna.
Raking of sediment (restoration)
Mining machines leave deep tracks and high flanks at the created track (valleys and ripples). Up to 36 years post disturbance these tracks are still very pronounced and visible (Khripounoff et al., 2006; Martínez Arbizu and Haeckel, 2015). The deep tracks could be smoothed out/levelled out by raking the sediment at the flanks and behind the machine. Advantages of this action are that it reduces hydrodynamic artefacts. Main disadvantages are that it will reduce the heterogeneity of the sediments, additional disturbance and associated fauna will suffer.
Avoid excessive compaction of sediments (mitigation and restoration)
When a mining machine collects the nodules it will also remove the upper sediment layers and leaves compacted sediment behind. Compaction of the sediment could be mitigated by developing “light-weight” mining machines or to reduce the overall weight of the mining machine (Figure 2F). This is likely to pose a technological challenge and might increase the production costs.
As a restoration action, de-compacting the sediments behind the machine by e.g., mechanical sediment irrigation could be envisioned. Decompaction increases the interstitial space and allows for a higher oxygen concentration to penetrate to the older and deeper sediments as well. While all organisms will suffer from the compaction occurring after the passage of a mining machine and decompaction will be beneficial for all size classes, they also may suffer from the decompaction process through the increased shear stress of the sediment particles.
Conclusion and Implications
There is a shortage or even lack of studies investigating impacts that resemble those caused by actual mining activity. The few existing anthropogenic and natural disturbance studies (Gollner et al., 2017; Jones et al., 2017) do not adequately mimic all of the impacts associated with deep-sea mining, not in type of impact or extent (scale). In addition, we only have fragmentary information on natural processes of (re-)colonisation, succession and recovery (Gollner et al., 2017). Hence, possible success of mitigation or restoration actions remain hard to predict. At present, none of the restoration strategies outlined here are derived from practical experience. There is thus no evidence that they somehow accelerate recovery in the strictest sense, i.e., by turning back an impacted ecosystem to its original (pre-impact) status. Some knowledge gaps could be filled in by providing guidelines to the industry. Several experiments that may possibly contribute to restoration of the ecosystem, but for which knowledge is lacking, could be carried out before or early on in the exploration phase of possible mining sites. Such experiments include disturbance studies resembling actual mining actions. In situ experiments to be considered are e.g., (i) deployment of artificial substrates to estimate recolonisation potential and to assess the composition and settlement potential of the regional species pool on these surfaces and (ii) transplant experiments (see Carreiro-Silva et al., 2016). Additional laboratory experiments could be conceived to culture larvae, to estimate larvae survival and settlement on substrata with different rugosity and composition. Possible effects of adding in different quantities of organic matter to monitor the impact of enrichment in selected species could be tested as well.
Another factor limiting the stage of knowledge is the distinctiveness of the different deep-sea ecosystems, combined with the high spatial and often temporal variability between and within. This contributes to high levels of uncertainty in generalising or extrapolating observed patterns, which reduces the usefulness and effectiveness of generalised recommendations for mitigation and restoration measures. Each individual potential mining site should be considered unique and will feature local characteristics that should be assessed accordingly.
Based on the research available, some concrete recommendations can be made. From an ecological point of view, the designation of set-aside areas (refuges) is of utmost importance as it appears to be the most comprehensive and precautionary approach, especially when an area is less well-known or studied. While it cannot replace the mined area, it can help to safeguard similar populations and ecosystems. Set-aside areas, where no mining takes place and no derived disturbance can be felt, should be implemented in each ecosystem planned to be subject to mining.
For the mined sites, not one (single) mitigation or restoration action will suffice; instead a set of combined actions should be envisioned. The types of actions will be highly dependent upon the local environment and ecosystem characteristics. Restoration can be plausible/possible in a limited number of scenarios but will always be associated with high levels of uncertainty. However, at this stage (current knowledge and technology) there exist quite a number of cases for which restoration is impossible, mostly when resource removal equals energy source removal. For those cases, a set of mitigation measures should be taken in order to avoid significant adverse impacts. The list of mitigation and restoration actions presented here is not restricted but can be added upon. We chose to discuss those actions, recurrent across the ecosystems of interest, deserving further research in order to fully appraise their potential.
The opportunity to inform the industry on how to “sustainably” exploit deep-sea resources is unique and timely for the scientific community. A broader dialogue with regulators and industry is encouraged as well as discussions on multidisciplinary fora, working toward a goal of best possible mining practices. However, the considerable lack of knowledge in the deep-sea ecosystems, when compared to more accessible ecosystems, needs to be emphasised. For the majority of deep-sea species, life history, dispersal, settlement, population dynamics are still unknown. A precautionary approach has to be applied, in order to prevent accelerated biodiversity decline on our planet and a responsibility to preserve deep-sea ecosystems for future generations. Recommendations as presented here represent a concrete contribution and can feed directly into guidelines for regulators and industry.
Author Contributions
All authorslisted have made a substantial, direct and intellectual contribution to the work, and approved it for publication.
Funding
DC is supportedby a post-doctoral scholarship (SFRH/BPD/110278/2015) from FCT. AC is supported by Programme Investigador (IF/00029/2014/CP1230/CT0002) from FCT. This work was funded by EU MIDAS project – Managing Impacts Of Deep Sea Resource Exploitation (from the European Union Seventh Framework Programme FP7/2007-2013), grant agreement 603418). DJ, AS, and TM were supported for this work by the EU Horizon 2020 project Marine Ecosystem Restoration in Changing European Seas (MERCES) grant agreement 689518. NM acknowledges the support from FCT through the grant UID/MAR/00350/2013 attributed to CIMA, University of Algarve. This study had also the support of PO AÇORES 2020 project Acores-01-0145-Feder-000054_RECO and of Fundação para a Ciência e Tecnologia (FCT), through the strategic projects UID/MAR/04292/2013 granted to MARE. The views and recommendations expressed in this paper are solely those of the authors.
Conflict of Interest Statement
The authors declare that the research was conducted in the absence of any commercial or financial relationships that could be construed as a potential conflict of interest.
Acknowledgments
This paper reflects discussions of a joint workshop on “colonisation of artificial substrates by deep-sea fauna” and “rates and modalities of ecosystem recovery” within the EU MIDAS project – Managing Impacts Of Deep Sea Resource Exploitation (from the European Union Seventh Framework Programme (FP7/2007-2013), grant agreement 603418) convening both experts in science and industry. Thanks to Mafalda Masceranhas and Henrique Cabral (MARE-FCUL) for providing the possibility to host the workshop at FCUL.
References
Aguilar de Soto, N., Gkikopoulou, K., Hooker, S., Isojunno, S., Johnson, M., Miller, P., et al. (2016). “From physiology to policy: a review of physiological noise effects on marine fauna with implications for mitigation,” in Proceedings of Meetings on Acoustics 4ENAL, Vol. 27 (Washington, DC: ASA), 040008. doi: 10.1121/2.0000299
Aguzzi, J., Costa, C., Furushima, Y., Chiesa, J., Company, J. B., Menesatti, P., et al. (2010). Behavioral rhythms of hydrocarbon seep fauna in relation to internal tides. Mar. Ecol. Prog. Ser. 418, 47–56. doi: 10.3354/meps08835
Airame, S., Dugan, J. E., Lafferty, K. D., Leslie, H., McArdle, D. A., and Warner, R. R. (2003). Applying ecological criteria to marine reserve design: a case study from the California Channel Islands. Ecol. Appl. 13, S170–S184. doi: 10.1890/1051-0761(2003)013[0170:AECTMR]2.0.CO;2
Alain, K., Zbinden, M., Le Bris, N., Lesongeur, F., Quérellou, J., Gaill, F., et al. (2004). Early steps in microbial colonization processes at deep-sea hydrothermal vents. Environ. Microbiol. 6, 227–241. doi: 10.1111/j.1462-2920.2003.00557.x
Aleynik, D., Inall, M. E., Dale, A., and Vink, A. (2017). Impact of remotely generated eddies on plume dispersion at abyssal mining sites in the Pacific. Sci. Rep. 7:16959. doi: 10.1038/s41598-017-16912-2
Amon, D. J., Ziegler, A. F., Dahlgren, T. G., Glover, A. G., Goineau, A., Gooday, A. J., et al. (2016). Insights into the abundance and diversity of abyssal megafauna in a polymetallic-nodule region in the eastern Clarion-Clipperton Zone. Sci. Rep. 6:30492. doi: 10.1038/srep30492
Armstrong, D. A., Stevens, B. G., and Hoeman, J. C. (1982). Distribution and Abundance of Dungeness Crab and Crangon Shrimp, and Dredging-Related Mortality of Invertebrates and Fish in Grays Harbor, Washington. Technical Report for Washington Department of Fisheries and U.S. Army Corps of Engineers. Seattle, WA: Seattle District by School of Fisheries, University of Washington, Seattle, 349.
Baldrighi, E., Zeppilli, D., Crespin, R., Chauvaud, P., Pradillon, F., and Sarrazin, J. (2018). Colonization of synthetic sponges at the deep-sea lucky Strike hydrothermal vent field (Mid-Atlantic Ridge): a first insight. Mar. Biodivers. 45, 89–103. doi: 10.1007/s12526-017-0811-3
Beaulieu, S. E. (2001). Colonisation of habitat islands in the deep sea: recruitment to glass sponge stalks. Deep Sea Res. I 48, 1121–1137. doi: 10.1016/S0967-0637(00)00055-8
Bergquist, D. C., Fleckenstein, C., Szalai, E. B., Knisel, J., and Fisher, C. R. (2004). Environment drives physiological variability in the cold seep mussel Bathymodiolus childressi. Limnol. Oceanogr. 49, 706–715. doi: 10.4319/lo.2004.49.3.0706
Bett, B. J., and Rice, A. L. (1992). The influence of hexactinellid sponge (Pheronema carpenteri) spicules on the patchy distribution of macrobenthos in the porcupine seabight (bathyal NE Atlantic). Ophelia 36, 217–226. doi: 10.1080/00785326.1992.10430372
Billett, D. S. M., Bett, B. J., Reid, W. D. K., Boorman, B., and Priede, M. (2010). Long-term change in the abyssal NE Atlantic: the ‘Amperima event’ revisited. Deep-Sea Res. Part II 57, 1406–1417. doi: 10.1016/j.dsr2.2009.02.001
Billett, D. S. M., Bett, B. J., Rice, A. L., Thurston, M. H., Galéron, J., Sibuet, M., et al. (2001). Long-term change in the megabenthos of the porcupine abyssal plain (NE Atlantic). Prog. Oceanogr. 50, 325–348. doi: 10.1016/S0079-6611(01)00060-X
Blöthe, M., Wegorzewski, A., Müller, C., Simon, F., Kuhn, T., and Schippers, A. (2015). Manganese-cycling microbial communities inside deep-sea manganese nodules. Environ. Sci. Technol. 49, 7692–7700. doi: 10.1021/es504930v
Bonnet, S., Guieu, C., Bruyant, F., Prášil, O., Van Wambeke, F., Raimbault, P., et al. (2008). Nutrient limitation of primary productivity in the Southeast Pacific (BIOSOPE cruise). Biogeosciences 5, 215–225. doi: 10.5194/bg-5-215-2008
Boschen, R. E., Rowden, A. A., Clark, M. R., Barton, S. J., Pallentin, A., and Gardner, J. P. A. (2015). Megabenthic assemblage structure on three New Zealand seamounts: implications for seafloor massive sulfide mining. Mar. Ecol. Prog. Ser. 523, 1–14. doi: 10.3354/meps11239
Boschen, R. E., Rowden, A. A., Clark, M. R., and Gardner, J. P. A. (2013). Mining of deep-sea seafloor massive sulfides: a review of the deposits, their benthic communities, impacts from mining, regulatory frameworks and management strategies. Ocean Coast. Manage. 84, 54–67. doi: 10.1016/j.ocecoaman.2013.07.005
Boschen, R. E., Rowden, A. A., Clark, M. R., Pallentin, A., and Gardner, J. P. A. (2016). Seafloor massive sulfide deposits support unique megafaunal assemblages: implications for seabed mining and conservation. Mar. Environ. Res. 115, 78–88. doi: 10.1016/j.marenvres.2016.02.005
Botsford, L. W., Hastings, A., and Gaines, S. D. (2001). Dependence of sustainability on the configuration of marine reserves and larval dispersal distance. Ecol. Lett. 4, 144–150. doi: 10.1046/j.1461-0248.2001.00208.x
Breier, J. A., White, S. N., and German, C. R. (2010). Mineral–microbe interactions in deep-sea hydrothermal systems: a challenge for Raman spectroscopy. Philos. Trans. R. Soc. A 368, 3067–3086. doi: 10.1098/rsta.2010.0024
Carreiro-Silva, M., Andrews, A. H., Braga-Henriques, A., Matos, V., Porteiro, F. M., and Santos R. S. (2013). Variability in growth rates of long-lived black coral Leiopathes sp. from the Azores (Northeast Atlantic). Mar. Ecol. Prog. Ser. 473, 189–199. doi: 10.3354/meps10052
Carreiro-Silva, M., Martins, I., Godinho, A., Carvalho, A., Rodrigues, F., Rakka, M., et al. (2016). “Restoration tools for cold-water communities impacted by mining activities,” in Proceedings of the MIDAS final Meeting, 3-7 October 2016, Abstract Book, Ghent.
Clark, M. R., Rowden, A. A., Schlacher, T., Williams, A., Consalvey, M., Stocks, K. I., et al. (2010). The ecology of seamounts: structure, function, and human impacts. Annu. Rev. Mar. Sci. 2, 253–278. doi: 10.1146/annurev-marine-120308-081109
Clark, M. R., Schlacher, T. A., Rowden, A. A., Stocks, K. I., and Consalvey, M. (2012). Science priorities for seamounts: research links to conservation and management. PLoS One 7:e29232. doi: 10.1371/journal.pone.0029232
Company, R., Serafim, A., Cosson, R., Fiala-Médioni, A., Dixon, D. R., and Bebianno, M. J. (2007). Adaptation of the antioxidant defence system in hydrothermal-vent mussels (Bathymodiolus azoricus) transplanted between two mid-atlantic ridge sites. Mar. Ecol. 28, 93–99. doi: 10.1111/j.1439-0485.2006.00125.x
Copley, J. T. P., Tyler, P. A., Van Dover, C. L., Schultz, A., Dickson, P., and Singh, S. (1999). Subannual temporal variation in faunal distributions at the TAG hydrothermal mound (26°N, Mid-Atlantic Ridge). Mar. Ecol. 20, 291–306. doi: 10.1046/j.1439-0485.1999.2034076.x
Cunha, M. R., Matos, F. L., Génio, L., Hilário, A., Moura, C. J., Ravara, A., et al. (2013). Are organic falls bridging reduced environments in the deep sea?—Results from colonisation experiments in the Gulf of Cádiz. PLoS One 8:e76688. doi: 10.1371/journal.pone.0076688
Cuvelier, D., Beesau, J., Ivanenko, V., Zeppilli, D., Sarradin, P. M., and Sarrazin, J. (2014). First insights into macro-and meiofaunal colonisation patterns on paired wood/slate substrata at Atlantic deep-sea hydrothermal vents. Deep-Sea Res. Part I 87, 70–81. doi: 10.1016/j.dsr.2014.02.008
Cuvelier, D., Sarrazin, J., Colaço, A., Copley, J., Desbruyères, D., Glover, A. G., et al. (2009). Distribution and spatial variation of Atlantic hydrothermal faunal assemblages revealed by high-resolution video image analysis. Deep Sea Res. I Oceanogr. Res. Pap. 56, 2026–2040. doi: 10.1016/j.dsr.2009.06.006
dela Cruz, D. W., and Harrison, P. L. (2017). Enhanced larval supply and recruitment can replenish reef corals on degraded reefs. Sci. Rep. 7:13985. doi: 10.1038/s41598-017-14546-y
Desbruyères, D., Almeida, A., Biscoito, M., Comtet, T., Khripounoff, A., Le Bris, N., et al. (2000). A review of the distribution of hydrothermal vent communities along the northern Mid-Atlantic Ridge: dispersal vs. environmental controls. Hydrobiologia 440, 201–216. doi: 10.1023/A:1004175211848
Desbruyères, D., Biscoito, M., Caprais, J. C., Colaco, A., Comtet, T., Crassous, P., et al. (2001). Variations in deep-sea hydrothermal vent communities on the Mid-Atlantic Ridge near the Azores plateau. Deep Sea Res. Part I – Oceanogr. Res. Pap. 48, 1325–1346. doi: 10.1016/S0967-0637(00)00083-2
Didham, R. K. (2010). Ecological Consequences of Habitat Fragmentation: Encyclopedia of Life Sciences (ELS). Chichester: John Wiley & Sons Ltd., 1–11. doi: 10.1002/9780470015902.a0021904
Durden, J. M., Bett, B. J., Jones, D. O. B., Huvenne, V. A. I., and Ruhl, H. A. (2015). Abyssal hills – Hidden source of increased habitat heterogeneity, benthic megafaunal biomass and diversity in the deep sea. Prog. Oceanogr. 137, 209–218. doi: 10.1016/j.pocean.2015.06.006
Ekstrom, J., Bennun, L., and Mitchell, R. (2015). A Cross-Sector Guide for Implementing the Mitigation Hierarchy. Cambridge: Cross Sector Biodiversity Initiative.
Erickson, K. L., Macko, S. A., and Van Dover, C. L. (2009). Evidence for a chemoautotrophically based food web at inactive hydrothermal vents (Manus Basin). Deep Sea Res. II 56, 1577–1585. doi: 10.1016/j.dsr2.2009.05.002
Fritz, J. S. (2016). Commentary: threatened by mining, polymetallic nodules are required to preserve abyssal epifauna. Front. Mar. Sci. 3:190. doi: 10.3389/fmars.2016.00190
Fustec, A., Desbruyères, D., and Juniper, K. S. (1987). Deep-sea hydrothermal vent communities at 13°N on the East Pacific Rise: microdistribution and temporal variations. Biol. Oceanogr. 4, 121–164.
Gaines, S. D., White, C., Carr, M. H., and Palumbi, S. R. (2010). Designing marine reserve networks for both conservation and fisheries management. Proc. Natl. Acad. Sci. U.S.A. 107, 18286–18293. doi: 10.1073/pnas.0906473107
Galkin, S. V. (1997). Megafauna associated with hydrothermal vents in the Manus back-arc Basin (Bismarck Sea). Mar. Geol. 142, 197–206. doi: 10.1016/S0025-3227(97)00051-0
Gaspar, M. B., Leitão, F., Santos, M. N., Sobral, M., Chícharo, L., Chícharo, A., et al. (2002). Influence of mesh size and tooth spacing on the proportion of damaged organisms in the catches of the Portuguese clam dredge fishery. ICES J. Mar. Sci. 59, 1228–1236. doi: 10.1006/jmsc.2002.1310
Gaudron, S. M., Lefebvre, S., Nunes-Jorge, A., Gaill, F., and Pradillon, F. (2012). Spatial and temporal variations in food web structure from newly-opened habitat at hydrothermal vents. Mar. Environ. Res. 77, 129–140. doi: 10.1016/j.marenvres.2012.03.005
Gaudron, S. M., Pailleret, M., Duperron, S., Pradillon, F., and Gaill, F. (2010). Colonisation of symbiotic species and associated fauna into organic substrates deployed in deep-sea reducing habitats. Mar. Environ. Res. 70, 1–12. doi: 10.1016/j.marenvres.2010.02.002
Génio, L., Warén, A., Matos, F. L., and Cunha, M. R. (2013). The snails’ tale in deep-sea habitats in the Gulf of Cadiz (NE Atlantic). Biogeosciences 10, 5159–5170. doi: 10.5194/bg-10-5159-2013
Gilmour, J. (1999). Experimental investigation into the effects of suspended sediment on fertilisation, larval survival and settlement in a scleractinian coral. Mar. Biol. 135, 451–462. doi: 10.1007/s002270050645
Girard, F., Lacharité, M., and Metaxas, A. (2016). Colonization of benthic invertebrates in a submarine canyon in the NW Atlantic. Mar. Ecol. Prog. Ser. 544, 53–64. doi: 10.3354/meps11555
Gladstone, W. (2007). Requirements for marine protected areas to conserve the biodiversity of rocky reef fishes. Aquat. Conserv. Mar. Freshw. Ecosyst. 17, 71–87. doi: 10.1002/aqc.759
Glover, A. G., Dahlgren, T. G., Wiklund, H., Mohrbeck, I., and Smith, C. R. (2015). An end-to-end DNA taxonomy methodology for benthic biodiversity survey in the clarion-clipperton zone, Central Pacific Abyss. J. Mar. Sci. Eng. 4:2. doi: 10.3390/jmse4010002
Gollner, S., Govenar, B., Martinez Arbizu, P., Mills, S., Le Bris, N., Weinbauer, M., et al. (2015). Differences in recovery between deep-sea hydrothermal vent and vent-proximate communities after a volcanic eruption. Deep-Sea Res. I 106, 167–182. doi: 10.1016/j.dsr.2015.10.008
Gollner, S., Kaiser, S., Menzel, L., Jones, D. O. B., Brown, A., Mestre, N. C., et al. (2017). Resilience of benthic deep-sea fauna to mining activities. Mar. Environ. Res. 12976–101. doi: 10.1016/j.marenvres.2017.04.010
Gollner, S., Miljutina, M., and Bright, M. (2013). Nematode succession at deep-sea hydrothermal vents after a recent volcanic eruption with the desciption of two dominant species. Org. Divers. Evol. 13, 349–371. doi: 10.1007/s13127-012-0122-2
Gollner, S., Riemer, B., Martinez Arbizu, P., Le Bris, N., and Bright, M. (2010). Diversity of meiofauna from the 9u50′N East Pacific Rise across a gradient of hydrothermal fluid emissions. PLoS One 5:e12321. doi: 10.1371/journal.pone.0012321
Govenar, B. W., and Fisher, C. R. (2007). Experimental evidence of habitat provision by aggregations of Riftia pachyptila at hydrothermal vents on the East Pacific Rise. Mar. Ecol. 28, 3–14. doi: 10.1111/j.1439-0485.2006.00148.x
Govenar, B. W., Le Bris, N., Gollner, S., Glanville, J., Aperghis, A., Hourdez, S., et al. (2005). Epifaunal community structure associated with Riftia pachyptila aggregations in chemically different hydrothermal vent habitats. Mar. Ecol. Progr. Ser. 2005, 67–77. doi: 10.3354/meps305067
Groves, C. R., Jensen, D. B., Valutis, L. L., Redford, K. H., Shaffer, M. L., Scott, J. M., et al. (2002). Planning for biodiversity conservation: putting conservation science into practice. A seven–step framework for developing regional plans to conserve biological diversity, based upon principles of conservation biology and ecology, is being used extensively by the nature conservancy to identify priority areas for conservation. BioScience 52, 499–512.
Gwyther, D. (2008). Environmental Impact Statement: Nautilus Minerals Niugini Limited, Solwara 1 Project, Vol. A. Brisbane, QL: Coffey Natural Systems, 226.
Halbach, P., Friedrich, G., and von Stackelberg, U. (1988). The Manganese Nodule Belt of the Pacific Ocean, ed. E. Verlag. Stuttgart: F. Enke.
Hauton, C., Brown, A. E., Thatje, S., Mestre, N. C., Bebianno, M. J., Martins, I., et al. (2017). Identifying toxic impacts of metals potentially released during deep-sea mining – A synthesis of the challenges to quantifying risk. Front. Mar. Sci. 4:368. doi: 10.3389/fmars.2017.00368
Hein, J. R. (2002). Cobalt-rich ferromanganese crusts: global distribution, composition, origin and research activities. Int. Seab. Authority Tech. Study 2, 36–89.
Hein, J. R., Mizell, K., Koschinsky, A., and Conrad, T. A. (2013). Deep-ocean mineral deposits as a source of critical metals for high-and green-technology applications: comparison with land-based resources. Ore Geol. Rev. 51, 1–14. doi: 10.1016/j.oregeorev.2012.12.001
Hein, J. R., and Petersen, S. (2013). “The geology of cobalt-rich ferromanganese crusts,” in Deep Sea Minerals: Cobalt-rich Ferromanganese Crusts; A Physical, Biological, Environmental, and Technical Review, eds E. Baker and Y. Beaudoin (Nouméa: Secretariat of the Pacific Communit), 7–14.
Hein, J. R., and Koschinsky, A. (2014). “Deep-ocean ferromanganese crusts and nodules,” in Treatise on Geochemistry, Chap. 11, Vol. 13, 2nd Edn, eds H. D. Holland and K. K. Turekian (Amsterdam: Elsevier), 273–291. doi: 10.1016/B978-0-08-095975-7.01111-6
Hessler, R. R., Smithey, W. M., Boudrias, M. A., Keller, C. H., Lutz, R. A., and Childress, J. J. (1988). Temporal change in megafauna at the rose garden hydrothermal vent (Galapagos Rift – Eastern tropical Pacific). Deep-Sea Res. Part A-Oceanogr. Res. Pap. 35, 1681–1709.
Hessler, R. R., Smithey, W. M., and Keller, C. H. (1985). Spatial and temporal variation of giant clams, tubeworms and mussels at deep-sea hydrothermal vents. Bull. Biol. Soc. Wash. 6, 411–428.
Higgs, N. D., Gates, A. R., and Jones, D. O. B. (2014). Fish food in the deep sea: revisiting the role of large food-falls. PLoS One 9:e96016. doi: 10.1371/journal.pone.0096016
Incorporated Society of British Advertisers [ISBA] (2007). Rationale and Recommendations for the Establishment of Preservation Reference Areas for Nodule Mining in the Clarion-Clipperton Zone. [Summary Outcomes of a Workshop to Design Marine Protected Areas for Seamounts and the Abyssal Nodule Province in Pacific High Seas, 23-26 October 2007. Manoa: University of Hawaii.
Ishiguro, S., Yamauchi, Y., Odaka, H., and Akiyama, S. (2013). Development of mining element engineering test machine for operating in seafloor hydrothermal deposits. Mitsub. Heavy Ind. Tech. Rev. 50, 21–26.
Ivanenko, V. N., Ferrari, F. D., Defaye, D., Sarradin, P.-M., and Sarrazin, J. (2011). Description, distribution and microhabitats of a new species of (Copepoda: Harpacticoida: Tisbidae) from a deep-sea hydrothermal vent field at the Mid-Atlantic Ridge (37N, Lucky Strike). Cah. Biol. Mar. 52, 89–106.
Jamieson, J. W., Clague, D. A., and Hannington, M. D. (2014). Hydrothermal sulfide accumulation along the Endeavour Segment, Juan de Fuca Ridge. Earth Planet. Sci. Lett. 395, 136–148. doi: 10.1016/j.epsl.2014.03.035
Jones, D. O. B., Kaiser, S., Sweetman, A. K., Smith, C. R., Menot, L., Vink, A., et al. (2017). Biological responses to disturbance from simulated deep-sea polymetallic nodule mining. PLoS One 12:e0171750. doi: 10.1371/journal.pone.0171750
Kaikkonen, L., Venesjärvi, R., Nygård, H., and Kuikka, S. (2018). Assessing the impacts of seabed mineral extraction in the deep sea and coastal marine environments: current methods and recommendations for environmental risk assessment. Mar. Pollut. Bull. 135, 1183–1197. doi: 10.1016/j.marpolbul.2018.08.055
Kelly, N. E., and Metaxas, A. (2008). Diversity of invertebrate colonists on simple and complex substrates at hydrothermal vents on the Juan de Fuca Ridge. Aquat. Biol. 3, 271–281. doi: 10.3354/ab00085
Kelly, N. E., Metaxas, A., and Butterfield, D. A. (2007). Spatial and temporal patterns of colonisation by deep-sea hydrothermal vent invertebrates on the Juan de Fuca Ridge, NE Pacific. Aquat. Biol. 1, 1–16. doi: 10.3354/ab00001
Khripounoff, A., Caprais, J. C., Crassous, P., and Etoubleau, J. (2006). Geochemical and biological recovery of the disturbed seafloor in polymetallic nodule fields of the Clipperton-Clarion Fracture Zone (CCFZ) at 5000-m depth. Limnol. Oceanogr. 51, 2033–2041. doi: 10.4319/lo.2006.51.5.2033
Klose, J., Polz, M. F., Wagner, M., Schimak, M. P., Gollner, S., and Bright, M. (2015). Endosymbionts escape dead hydrothermal vent tubeworms to enrich the free-living population. Proc. Natl. Acad. Sci. U.S.A. 112, 11300–11305. doi: 10.1073/pnas.1501160112
Knoop, P. A., Owen, R. M., and Morgan, C. L. (1998). Regional variability in ferromanganese nodule composition: northeastern tropical Pacific Ocean. Mar. Geol. 147, 1–12. doi: 10.1016/S0025-3227(97)00077-7
Koschinsky, A., Hein, J., Schmidt, K., Alexander, B., and Bau, M. (2010). Rare and Valuable Metals for High-Tech Applications Found in Marine Ferromanganese Nodules and Crusts: Relationships to Genetic Endmembers. Underwater Mining Institute. Gelendzhik, Russia.
LaRowe, D. E., Dale, A. W., Aguilera, D. R., L’Heureux, I., Amend, J. P., and Regnier, P. (2014). Modeling microbial reaction rates in a submarine hydrothermal vent chimney wall. Geochim. Cosmochim. Acta 124, 72–97. doi: 10.1016/j.gca.2013.09.005
Larson, A. I., and Purser, A. (2011). Sedimentation on the cold-water coral Lophelia pertusa: cleaning efficiency from natural sediments and drill cuttings. Mar. Pollut. Bull. 62, 1159–1168. doi: 10.1016/j.marpolbul.2011.03.041
Larson, K. W., and Moehl, C. E., (1990). “Entrainment of anadromous fish by hopper dredge at the mouth of the columbia river,” in Effects of Dredging on Anadromous Pacific Coast Fishes. Workshop Proceedings, Seattle, September 8-9, 1988. Washington Sea Grant Program, ed. C. A. Simenstad (Seattle, WA: University of Washington).
Larsson, A. I., van Oevelen, D., Purser, A., and Thomsen, L. (2013). Tolerance to long-term exposure of suspended benthic sediments and drill cuttings in the cold-water coral Lophelia pertusa. Mar. Pollut. Bull. 70, 176–188. doi: 10.1016/j.marpolbul.2013.02.033
Ledwell, J. R., Montgomery, E. T., Polzin, K. L., St. Laurent, L. C., Schmitt, R. W., and Toole, J. M. (2000). Evidence for enhanced mixing over rough topography in the abyssal ocean. Nature 403, 179–182. doi: 10.1038/35003164
Lees, P., Kenny, A., and Pearson, R. (1992). The Condition of Benthic Fauna in Suction Dredger Outwash: Initial Findings. Annex II. In: Report of the Working Group on the Effects of Extraction of Marine Sediments on Fisheries. International Council for the Exploration of the Sea (ICES Copenhagen), 22–28.
Legendre, P. (2014). Interpreting the replacement and richness difference components of beta diversity. Glob. Ecol. Biogeogr. 23, 1324–1334. doi: 10.1111/geb.12207
Leitner, A. B., Neuheimer, A. B., Donlon, E., Smith, C. R., and Drazen, J. C. (2017). Environmental and bathymetric influences on abyssal bait-attending communities of the Clarion Clipperton Zone. Deep. Res. Part I Oceanogr. Res. Pap. 125, 65–80. doi: 10.1016/j.dsr.2017.04.017
Levin, L. A., Mendoza, G. F., Konotchick, T., and Lee, R. (2009). Macrobenthos community structure and trophic realtionships within active and inactive Pacific hydrothermal sediments. Deep-Sea Res. II 56, 1632–1648. doi: 10.1016/j.dsr2.2009.05.010
Levin, L. A., Mengerink, K., Gjerde, K. M., Rowden, A. A., Van Dover, C. L., Clark, M. R., et al. (2016). Defining “serious harm” to the marine environment in the context of deep seabed mining. Mar. Policy 74, 245–259. doi: 10.1016/j.marpol.2016.09.032
Leys, S. P., and Lauzon, N. R. J. (1998). Hexactinellid sponge ecology: growth rates and seasonality in deep water sponges. J. Exp. Mar. Biol. Ecol. 230, 111–129. doi: 10.1016/S0022-0981(98)00088-4
Lim, S.-C., Wiklund, H., Glover, A. G., Dahlgren, T. G., and Tan, K.-S. (2017). A new genus and species of abyssal sponge commonly encrusting polymetallic nodules in the Clarion-Clipperton Zone. East Pacific Ocean. Syst. Biodivers. 15, 507–519. doi: 10.1080/14772000.2017.1358218
Lodge, M., Johnson, D., Le Gurun, G., Wengler, M., Weaver, P., and Gunn, V. (2014). Seabed mining: international seabed authority environmental management plan for the clarion-clipperton zone, a partnership approach. Mar. Policy 49, 66–72. doi: 10.1016/j.marpol.2014.04.006
Luther, G. W., Rozan, T. F., Taillefert, M., Nuzzio, D. B., Di Meo, C., Shank, T. M., et al. (2001). Chemical speciation drives hydrothermal vent ecology. Nature 410, 813–816. doi: 10.1038/35071069
Lutz, R., Shank, T., Fornari, D., Haymon, R., Lilley, M., Von Damm, K., et al. (1994). Rapid growth at the deep-sea vents. Nature 371, 663–664. doi: 10.1038/371663a0
Lyngstad, T. M., Høgåsen, H. R., Jansen, M. D., and Nilsen, A. (2015). Risk of Disease Transfer with Wellboats in Norway – Technical Report. Veterinærinstituttets Oslo Rapportserie 15–2015. Oslo: Norwegian Veterinary Institute.
Madureira, P., Brekke, H., Cherkashov, G., and Rovere, M. (2016). Exploration of polymetallic nodules in the area: reporting practices, data management and transparency. Mar. Policy 70, 101–107. doi: 10.1016/j.marpol.2016.04.051
Marsh, L., Copley, J. T., Huvenne, V. A. I., Linse, K., Reid, W. D. K., and Rogers, A. D. (2012). Microdistribution of faunal assemblages at deep-sea hydrothermal vents in the southern ocean. PLoS One 7:e48348. doi: 10.1371/journal.pone.0048348
Martínez Arbizu, P., and Haeckel, M. (2015). Ecoresponse assessing the ecology, connectivity and resilience of polymetallic nodule field systems. GEOMAR Rep. Nr. 25, 76–88. doi: 10.3289/GEOMAR_REP_NS_25_2015
McClain, C. R., and Hardy, S. M. (2010). The dynamics of biogeographic ranges in the deep sea. Proc. R. Soc. B Biol. Sci. 277, 3533–3546. doi: 10.1098/rspb.2010.1057
McDonald, T., Jonson, J., and Dixon, K. W. (2016). National standards for the practice of ecological restoration in Australia. Restor. Ecol. 24(S1), S4–S32. doi: 10.1111/rec.12359
Menge, B. A., and Sutherland, J. P. (1976). Species diversity gradients: synthesis of the roles of predation, competition, and temporal heterogeneity. Am. Nat. 110:351. doi: 10.1086/283073
Menzel, L., George, K. H., and Martínez Arbizu, P. (2011). Submarine ridges do not prevent large-scale dispersal of abyssal fauna: a case study of Mesocletodes (Crustacea, Copepoda, Harpacticoida). Deep-Sea Res. I 58, 839–864. doi: 10.1016/j.dsr.2011.05.008
Metaxas, A., and Kelly, N. E. (2010). Do larval supply and recruitment vary among chemosynthetic environments of the deep sea? PLoS One 5:e11646. doi: 10.1371/journal.pone.0011646
Micheli, F., Peterson, C. H., Mullineaux, L. S., Fisher, C., Mills, S. W., Sancho, G., et al. (2002). Predation structures communities at deep-sea hydrothermal vents. Ecol. Monogr. 72, 365–382. doi: 10.1890/0012-9615(2002)072[0365:PSCADS]2.0.CO;2
Miller, K. A., Thompson, K. F., Johnston, P., and Santillo, D. (2018). An overview of seabed mining including the current state of development, environmental impacts, and knowledge gaps. Front. Mar. Sci. 4:418. doi: 10.3389/fmars.2017.00418
Ministry of Economy Trade and Industry [METI] (2017). World’s First Success in Continuous Ore Lifting test for Seafloor Polymetallic Sulphides Pilot test of Excavating and ore Lifting Conducted for Seafloor Polymetallic Sulphides Under the Sea Area Near Okinawa Prefecture. Available at: http://www.meti.go.jp/english/press/2017/0926_004.html [accessed Jan 11, 2018].
Miyake, H., Kitada, M., Itoh, T., Nemoto, S., Okuyama, Y., Watanabe, H., et al. (2010). Larvae of deep-sea chemosynthetic ecosystem animals in captivity. Cah. Biol. Mar. 51, 441–450.
Mora, C. (2011). 13 r REPRESENTATIVENESS-Effectiveness of the global network ofmarine protected areas. Mar. Prot. Areas Multidiscipl. Approach, 334–346.
Morato, T., Hoyle, S. D., Allain, V., and Nicol, S. J. (2010). Seamounts are hotspots of pelagic biodiversity in the open ocean. Proc. Natl. Acad. Sci. U.S.A. 107, 9707–9711. doi: 10.1073/pnas.0910290107
Morgan, N. B., Cairns, S., Resiwig, H., and Baco, A. R. (2015). Benthic megafaunal community structure of cobalt-rich manganese crusts on Necker Ridge. Deep-Sea Res. I 104, 92–105. doi: 10.1016/j.dsr.2015.07.003
Mullineaux, L. S. (1987). Organisms living on manganese nodules and crusts: distribution and abundance at three North Pacific sites. Deep Sea Res. Part A. Oceanogr. Res. Pap. 34, 165–184. doi: 10.1016/0198-0149(87)90080-X
Mullineaux, L. S. (1988). The role of settlement in structuring a hard-substratum community in the deep sea. J. Exp. Mar. Biol. Ecol. 120, 241–261. doi: 10.1016/0022-0981(88)90005-6
Mullineaux, L. S., Adams, D. K., Mills, S. W., and Beaulieu, S. E. (2010). Larvae from afar colonize deep-sea hydrothermal vents after a catastrophic eruption. Proc. Natl. Acad. Sci. U.S.A. 107, 7829–7834. doi: 10.1073/pnas.0913187107
Mullineaux, L. S., and Butman, C. A. (1990). Recruitment of encrusting benthic invertebrates in boundary-layer flows: a deep-water experiment on Cross Seamount. Limnol. Oceanogr. 35, 409–423. doi: 10.4319/lo.1990.35.2.0409
Mullineaux, L. S., Fisher, C. R., Peterson, C. H., and Schaeffer, S. W. (2000). Tubeworm succession at hydrothermal vents: use of biogenic cues to reduce habitat selection error? Oecologia 123, 275–284. doi: 10.1007/s004420051014
Mullineaux, L. S., Le Bris, N., Mills, S. W., Henri, P., Bayer, S. R., Secrist, R. G., et al. (2012). Detecting the influence of initial pioneers on succession at deep-sea vents. PLoS One 7:e50015. doi: 10.1371/journal.pone.0050015
Mullineaux, L. S., Micheli, F., Peterson, C. H., Lenihan, H. S., and Markus, N. (2009). Imprint of past environmental regimes on structure and succession of a deep-sea hydrothermal vent community. Oecologia 161, 387–400. doi: 10.1007/s00442-009-1390-1
Mullineaux, L. S., Mills, S. W., and Goldman, E. (1998). Recruitment variation during a pilot colonisation study of hydrothermal vents (9(50′N, East Pacific Rise). Deep-Sea Res. II 45, 441–464. doi: 10.1016/S0967-0645(97)00045-3
Mullineaux, L. S., Peterson, C. H., Micheli, F., and Mills, S. W. (2003). Successional mechanism varies along a gradient in hydrothermal fluid flux at deep-sea vents. Ecol. Monogr. 73, 523–542. doi: 10.1890/02-0674
Nakajima, R., Yamamoto, H., Kawagucci, S., Takaya, Y., Nozaki, T., and Chen, C. (2015). Post-drilling changes in seabed landscape and megabenthos in a deep-sea hydrothermal system, the iheya north field, Okinawa Trough. PLoS One 10:e0123095. doi: 10.1371/journal.pone.0123095.g007
Nakamura, R., Ando, W., Yamamoto, H., Kitano, M., Sato, A., Kakamura, M., et al. (2011). Corals mass-cultured from eggs and transplanted as juveniles to their native, remote coral reef”. MEPS 436, 161–168. doi: 10.3354/meps09257
Newell, R. C., Seiderer, L. J., and Hitchcock, D. R. (1998). The impact of dredging works in coastal waters: a review of the sensitivity to disturbance and subsequent recovery of biological resources on the sea bed. Oceanogr. Mar. Biol. 36, 127–178.
Newell, R. C., Seiderer, L. J., Simpson, N. M., and Robinson, J. E. (2004). Impacts of marine aggregate dredging on benthic macrofauna. J. Coast. Res. 20, 115–125. doi: 10.2112/1551-5036(2004)20[115:IOMADO]2.0.CO;2
Niner, H. J., Ardron, J. A., Escobar, E. G., Gianni, M., Jaeckel, A., Jones, D. O. B., et al. (2018). Deep-sea mining with no net loss of biodiversity—An Impossible Aim. Front. Mar. Sci. 5:53. doi: 10.3389/fmars.2018.00053
Nozaki, T., Ishibashi, J.-I., Shimada, K., Nagase, T., Takaya, Y., Kato, Y., et al. (2016). Rapid growth of mineral deposits at artificial seafloor hydrothermal vents. Sci. Rep. 6:22163. doi: 10.1038/srep22163
O’Brien, C. E., Giovannelli, D., Govenar, B., Luther, G. W., Lutz, R. A., Shank, T. M., et al. (2015). Microbial biofilms associated with fluid chemistry and megafaunal colonization at post-eruptive deep-sea hydrothermal vents. Deep. Res. Part II Top. Stud. Oceanogr. 121, 31–40. doi: 10.1016/j.dsr2.2015.07.020
Oebius, H. U., Becker, H. J., Rolinski, S., and Jankowski, J. A. (2001). Parametrization and evaluation of marine environmental impacts produced by deep-sea manganese nodule mining. Deep Sea Res. Part II Top. Stud. Oceanogr. 48, 3453–3467. doi: 10.1016/S0967-0645(01)00052-2
Ondréas, H., Cannat, M., Fouquet, Y., and Normand, A. (2012), Geological context and vents morphology of the ultramafic-hosted Ashadze hydrothermal areas (Mid-Atlantic Ridge 13°N). Geochem. Geophys. Geosyst. 13:Q0AG14. doi: 10.1029/2012GC004433
Palumbi, S. R. (2003). Population genetics, demographic connectivity, and the design of marine reserves. Ecol. Appl. 13, 146–158. doi: 10.1890/1051-0761(2003)013[0146:PGDCAT]2.0.CO;2
Petersen, S., Krätschell, A., Augustin, N., Jamieson, J., Hein, J. R., and Hannington, M. D. (2016). News from the seabed–geological characteristics and resource potential of deep-sea mineral resources. Mar. Policy 70, 175–187. doi: 10.1016/j.marpol.2016.03.012
Phillips, N. E., and Shima, J. S. (2006). Differential effects of suspended sediments on larval survival and settlement of New Zealand urchins Evechinus chloroticus and abalone Haliotis iris. Mar. Ecol. Prog. Ser. 314, 149–158. doi: 10.3354/meps314149
Plum, C., Pradillon, F., Fujiwara, Y., and Sarrazin, J. (2017). Copepod colonization of organic and inorganic substrata at a deep-sea hydrothermal vent site on the Mid-Atlantic Ridge. Deep. Res. Part II Top. Stud. Oceanogr. 137, 335–348. doi: 10.1016/j.dsr2.2016.06.008
Pradillon, F., Zbinden, M., Le Bris, N., Hourdez, S., Barnay, A.-S., and Gaill, F. (2009). Developpement of assemblages associated with alvinellid colonies on the walls of high-temperature vents at the East Pacific Rise. Deep-Sea Res. II 56, 1622–1631. doi: 10.1016/j.dsr2.2009.05.009
Pradillon, F., Zbinden, M., Mullineaux, L. S., and Gaill, F. (2005). Colonisation of newly-opened habitat by a pioneer species, alvinella pompejana (Polychaeta: Alvinellidae), at East Pacific Rise vent sites. Mar. Ecol. Prog. Ser. 302, 147–157. doi: 10.3354/meps302147
Ramirez-Llodra, E., Trannum, H. C., Evenset, A., Levin, L. A., Andersson, M., Finne, T. E., et al. (2015). Submarine and deep-sea mine tailing placements: a review of current practices, environmental issues, natural analogs and knowledge gaps in Norway and internationally. Mar. Pollut. Bull. 97, 13–35. doi: 10.1016/j.marpolbul.2015.05.062
Rice, J., and Houston, K. (2011). Representativity and networks of marine protected areas. Aquat. Conserv. Mar. Freshw. Ecosyst. 21, 649–657. doi: 10.1002/aqc.1232
Roberts, L., and Elliott, M. (2017). Good or bad vibrations? Impacts of anthropogenic vibration on the marine epibenthos. Sci. Total Environ. 595, 255–268. doi: 10.1016/j.scitotenv.2017.03.117
Rogers, A. D. (1999). The biology of Lophelia pertusa (LINNAEUS 1758) and other deep-water reef-forming corals and impacts from human activities. Int. Rev. Hydrobiol. 84, 315–406. doi: 10.1002/iroh.199900032
Rolinski, S., Segschneider, J., and Sündermann, J. (2001). Long-term propagation of tailings from deep-sea mining under variable conditions by means of numerical simulations. Deep. Res. Part II Top. Stud. Oceanogr. 48, 3469–3485. doi: 10.1016/S0967-0645(01)00053-4
Rowden, A. A., Dower, J. F., Schlacher, T. A., Consalvey, M., and Clark, M. R. (2010). Paradigms in seamount ecology: fact, fiction and future. Mar. Ecol. 31, 226–241. doi: 10.1111/j.1439-0485.2010.00400.x
Sarrazin, J., and Juniper, S. K. (1999). Biological characteristics of a hydrothermal edifice mosaic community. Mar. Ecol. Prog. Ser. 185, 1–19. doi: 10.3354/meps185001
Sarrazin, J., Levesque, C., Juniper, S. K., and Tivey, M. K. (2002). Mosaic community dynamics on Juan de Fuca Ridge sulfide edifices: substratum, temperature and implications for trophic structure. Cah. Biol. Mar. 43, 275–279.
Sarrazin, J., Robigou, V., Juniper, S. K., and Delaney, J. R. (1997). Biological and geological dynamics over four years on a high-temperature sulfide structure at the Juan de Fuca Ridge hydrothermal observatory. Mar. Ecol. Prog. Ser. 153, 5–24. doi: 10.3354/meps153005
Schlacher, T. A., Baco, A. R., Rowden, A. A., O’Hara, T. D., Clark, M. R., Kellet, C., et al. (2014). Seamount benthos in a cobalt -rich crust region of the central Pacific: conservation challenges for future seabed mining. Divers. Distribut. 20, 491–502. doi: 10.1111/ddi.12142
Secretariat of the Pacific Community [SPC] (2013). Deep Sea Minerals: Sea-floor Massive Sulphides, a Physical, Biological, Environmental, and Technical Review, 1A, eds E. Baker and Y. Beaudoin. Nouméa: Secretariat of the Pacific Community.
Shank, T. M., Fornari, D. J., Von Damm, K. L., Lilley, M. D., and Haymon, R. M. (1998). Temporal and spatial patterns of biological community development at nascent deep-sea hydrothermal vents (9°50′N, East Pacific Rise). Deep Sea Res. Part 2 Top. Stud. Oceanogr. 45, 465–515. doi: 10.1016/S0967-0645(97)00089-1
Smith, C. R., Glover, A. G., Treude, T., Higgs, N. D., and Amon, D. J. (2015). Whale-fall ecosystems: recent insights into ecology, paleoecology, and evolution. Annu. Rev. Mar. Sci. 7, 571–596. doi: 10.1146/annurev-marine-010213-135144
Smith, C. R., Levin, L. A., Koslow, A., Tyler, P. A., and Glover, A. G. (2008). “The near future of the deep seafloor ecosystems,” in Aquatic Ecosystems: Trends and Global Prospects, ed. N. V. C. Polunin (Cambridge: Cambridge University Press), 334–353. doi: 10.1017/CBO9780511751790.030
Society for Ecological Restoration [SER] (2004). The SER Primer on Ecological Restoration. Washington, DC: Society for Ecological Restoration International, Science and Policy Working Group.
Stevens, B. (1981). Dredging-Related Mortality of Dungeness Crabs Associated with Four Dredges Operating in Grays Harbor, Washington. Seattle, WA: Washington Department of Fisheries Report to U.S. Army Engineers District. doi: 10.21236/ADA100139
Stoyanova, V. (2012). Megafaunal diversity associated with deep-sea nodule-bearing habitats in the eastern part of the clarion-clipperton zone, NE pacific. Int. Multidiscipl. Sci. 1:645. doi: 10.5593/sgem2012/s03.v1032
Stratmann, T., Mevenkamp, L., Sweetman, A. K., Vanreusel, A., and van Oevelen, D. (2018). Has phytodetritus processing by an abyssal soft-sediment community recovered 26 years after an experimental disturbance? Front. Mar. Sci. 5:59. doi: 10.3389/fmars.2018.00059
Sweetman, A. K., Sommer, S., Pfannkuche, O., and Witte, U. (2009). Retarded response by macrofauna-size foraminifera to phytodetritus in a deep Norwegian fjord. J. Foraminiferal Res. 39, 15–22. doi: 10.2113/gsjfr.39.1.15
Sweetman, A. K., Thurber, A. R., Smith, C. R., Levin, L. A., Mora, C., Wei, C.-L., et al. (2017). Major impacts of climate change on deep-sea benthic ecosystems. Elem. Sci. Anth 5:4. doi: 10.1525/elementa.203
Sylvan, J. B., Toner, B. M., and Edwards, K. J. (2012). Life and death of deep-sea vents: bacterial diversity and ecosystem succession on inactive hydrothermal sulfides. mBio 3:e00279-11. doi: 10.1128/mBio.00279-11
Thiel, H., Schriever, G., Bussau, C., and Borowski, C. (1993). Managanese nodule crevice fauna. Deep Sea Res. 40, 419–423. doi: 10.1016/0967-0637(93)90012-R
Thurber, A. R., Sweetman, A. K., Narayanaswamy, B. E., Jones, D. O. B., Ingels, J., and Hansman, R. L. (2014). Ecosystem function and services provided by the deep sea. Biogeosciences 11, 3941–3963. doi: 10.5194/bg-11-3941-2014
Tilot, V. (2010). Biodiversity and Distribution of Faunal Assemblages Options for the Management and Conservation of the Nodule Ecosystem in the Clarion Clipperton Fracture Zone (NE Pacific Ocean): Scientific, Legal and Institutional Aspects: UNESCO/IOC, 2010 (IOC Technical Series, 69). Paris: Intergovernmental Oceanographic Commission.
Tittensor, D. P., Baco-Taylor, A. R., Brewin, P., Clark, M. R., Consalvey, M., et al. (2009). Predicting global habitat suitability for stony corals on seamounts. J. Biogeogr. 36, 1111–1128. doi: 10.1111/j.1365-2699.2008.02062.x
Tunnicliffe, V. (1990). Observations on the effects of sampling on hydrothermal vent habitat and fauna of Axial Seamount, Juan-de-Fuca Ridge. J. Geophys. Res. Solid Earth Planets 95, 12961–12966. doi: 10.1029/JB095iB08p12961
Tunnicliffe, V. (1991). The biology of hydrothermal vents – Ecology and evolution. Oceanogr. Mar. Biol. 29, 319–407.
Tunnicliffe, V., Botros, M., De Burgh, M. E., Dinet, A., Johnson, H. P., Juniper, S. K., et al. (1986). Hydrothermal vents of explorer ridge, northeast Pacific. Deep Sea Res. Part A 33, 401–412. doi: 10.1016/0198-0149(86)90100-7
Tunnicliffe, V., Embley, R. W., Holden, J. F., Butterfield, D. A., Massoth, G. J., and Juniper, S. K. (1997). Biological colonisation of new hydrothermal vents following an eruption on Juan De Fuca Ridge. Deep Sea Res. Part 44, 1627–1644. doi: 10.1016/S0967-0637(97)00041-1
Tunnicliffe, V., and Juniper, S. K. (1990). Dynamic character of the hydrothermal vent habitat and the nature of sulphide chimney fauna. Prog. Oceanogr. 24, 1–13. doi: 10.1016/0079-6611(90)90015-T
Van Dover, C. L. (2000). The Ecology of Deep-Sea Hydrothermal Vents. Princeton: Princeton University Press, 412.
Van Dover, C. L. (2011). Mining seafloor massive sulphides and biodiversity: what is at risk? ICES J. Mar. Sci. 68, 341–348. doi: 10.1093/icesjms/fsq086
Van Dover, C. L., Ardron, J. A., Escobar, E., Gianni, M., Gjerde, K. M., Jaeckel, A., et al. (2017). Biodiversity loss from deep-sea mining. Nat. Geosci. 10, 464–465. doi: 10.1038/ngeo2983
Van Dover, C. L., Aronson, J., Pendleton, L., Smith, S., Arnaud-Haond, S., Moreno-Mateos, D., et al. (2014). Ecological restoration in the deep sea. supplementary file: principles of ecological restoration. Mar. Policy 44, 1–10. doi: 10.1038/nmeth.1674
Van Dover, C. L., Berg, C. J. Jr., and Turner, R. D. (1988). Recruitment of marine invertebrates to hard substrates at deep-sea hydrothermal vents on the East Pacific Rise and Galapagos spreading center. Deep-Sea Res. 35, 1833–1849. doi: 10.1016/0198-0149(88)90052-0
Van Dover, C. L., and Trask, J. L. (2000). Diversity at deep-sea hydrothermal vent and intertidal mussel beds. Mar. Ecol. Prog. Ser. 195, 169–178. doi: 10.3354/meps195169
Vanreusel, A., Hilario, A., Ribeiro, P. A., Menot, L., and Mrtinez Arbizu, P. (2016). Threatened by mining, polymetallic nodules are required to preserve abyssal epifauna. Sci. Rep. 6:26808. doi: 10.1038/srep26808
Veillette, J., Juniper, S. K., Gooday, A. J., and Sarrazin, J. (2007a). Influence of surface texture and microhabitat heterogeneity in structuring nodule faunal communities. Deep Sea Res. Part I Oceanogr. Res. Pap. 54, 1936–1943. doi: 10.1016/j.dsr.2007.06.012
Veillette, J., Sarrazin, J., Gooday, A. J., Galéron, J., Caprais, J. C., Vangriesheim, A., et al. (2007b). Ferromanganese nodule fauna in the Tropical North Pacific Ocean: species richness, faunal cover and spatial distribution. Deep Sea Res. Part I: Oceanogr. Res. Pap. 54, 1912–1935. doi: 10.1016/j.dsr.2007.06.011
Verlaan, P. A. (1992). Benthic recruitment and manganese crust formation on seamounts. Mar. Biol. 113, 171–174. doi: 10.1007/BF00367651
Vis, H., and Kemper, J. H. (2012). Test Fish Survivability Bedford Pumps Saf.90.05.12 PumP at 330 rpm (1.3 m3/s). Report: VA2011_28. Nieuwegein: VisAdvies BV.
Waite, T. J., Moore, T. S., Childress, J. J., Hsu-Kim, H., Mullaugh, K. M., Nuzzio, D. B., et al. (2008). Variation in sulphur speciation with shellfish presence at a Lau Basin diffuse flow vent site. J. Shellfish Res. 27, 163–168. doi: 10.2983/0730-8000(2008)27[163:VISSWS]2.0.CO;2
Wedding, L. M., Friedlander, A. M., Kittinger, J. N., Watling, L., Gaines, S. D., Bennett, M., et al. (2013). From principles to practice: a spatial approach to systematic conservation planning in the deep sea. Proc. R. Soc. B 280:20131684. doi: 10.1098/rspb.2013.1684
Weilgart, L. (2018). The Impact of Ocean Noise Pollution on Fish and Invertebrates. Report for OceanCare, Switzerland. Ph.D., Oceancare & Dalhousie University, Dalhousie, 34.
Witte, U., Wenzhöfer, F., Sommer, S., Boetius, A., Heinz, P., Aberle, N., et al. (2003). In situ experimental evidence of the fate of a phytodetritus pulse at the abyssal sea floor. Nature 424:763. doi: 10.1038/nature01799
Won, Y., Young, C. R., Lutz, R. A., and Vrijenhoek, R. C. (2003). Dispersal barriers and isolation among deep-sea mussel populations (Mytilidae: Bathymodiolus) from eastern Pacific hydrothermal vents. Mol. Ecol. 12, 169–184. doi: 10.1046/j.1365-294X.2003.01726.x
Keywords: deep sea, mining, restoration, mitigation, impacts, assisted recovery, (re-)colonisation
Citation: Cuvelier D, Gollner S, Jones DOB, Kaiser S, Arbizu PM, Menzel L, Mestre NC, Morato T, Pham C, Pradillon F, Purser A, Raschka U, Sarrazin J, Simon-Lledó E, Stewart IM, Stuckas H, Sweetman AK and Colaço A (2018) Potential Mitigation and Restoration Actions in Ecosystems Impacted by Seabed Mining. Front. Mar. Sci. 5:467. doi: 10.3389/fmars.2018.00467
Received: 15 May 2018; Accepted: 22 November 2018;
Published: 10 December 2018.
Edited by:
Angel Borja, Centro Tecnológico Experto en Innovación Marina y Alimentaria (AZTI), SpainReviewed by:
Jan-Stefan Fritz, German Marine Research Consortium, GermanyGeorgios Kazanidis, The University of Edinburgh, United Kingdom
Copyright © 2018 Cuvelier, Gollner, Jones, Kaiser, Arbizu, Menzel, Mestre, Morato, Pham, Pradillon, Purser, Raschka, Sarrazin, Simon-Lledó, Stewart, Stuckas, Sweetman and Colaço. This is an open-access article distributed under the terms of the Creative Commons Attribution License (CC BY). The use, distribution or reproduction in other forums is permitted, provided the original author(s) and the copyright owner(s) are credited and that the original publication in this journal is cited, in accordance with accepted academic practice. No use, distribution or reproduction is permitted which does not comply with these terms.
*Correspondence: Daphne Cuvelier, ZGFwaG5lLmN1dmVsaWVyQGdtYWlsLmNvbQ==