- 1Norwegian Institute for Water Research, Bergen, Norway
- 2The Sven Lovén Centre for Marine Sciences, University of Gothenburg, Gothenburg, Sweden
- 3SKLEC-NIVA Centre for Marine and Coastal Research, State Key Laboratory of Estuarine and Coastal Research, East China Normal University, Shanghai, China
Understanding links between the abiotic environment and organism fitness and function is a central challenge of biology, and an issue of growing relevance due to anthropogenic environmental changes. To date, our understanding of these links has largely been based on the findings of isolated experimental studies. This command may, however, be enhanced where currently disparate data are synthesized. By outlining a range of approaches appropriate in bringing together the findings of studies considering ocean acidification effects, we hope to provide insight as to how they may be used in the future. Specifically, approaches discussed in this narrative literature review include established literature review methods, as well as emerging schemes structured around biological theories (i.e., dynamic energy budget, DEB; oxygen- and capacity-limited thermal tolerance, OCLTT; multiple performance-multiple optima, MPMO), and strategies developed in other disciplines (i.e., adverse outcome pathways, AOP). In the future approaches to use such frameworks in creative combinations may be developed. Here we discuss some of these potential combinations, specifically the use of: AOPs to identify key steps that can be explored in more detail through literature review frameworks; OCLTT and DEB frameworks to consider effects on both energy supply and allocation; MPMO frameworks to identify the performance curves of organisms whose interactions are considered in an ecosystem model. Regardless of the approach taken, synthesizing scientific literature represents a potentially powerful method to enhance understanding of the influence of the abiotic environment on whole organism fitness.
Introduction
Patterns and processes observed in ecosystems are determined in large part by the functioning of component organisms whose fitness is, in turn, influenced by the surrounding environment. Understanding the links between organism function and environmental condition is of fundamental interest to resolving long-standing questions around the matter of what determines sensitivity or resistance to environmental change (Chapin et al., 1997). This relationship is of particular significance due to the increasing proliferation of human-induced effects (Pörtner and Farrell, 2008). A need exists, therefore, to improve the approaches used to develop understanding of links between fine-scale physiological processes that determine organism fitness and ecosystem structure, and broader-scale abiotic environmental conditions.
A key environmental change to which marine biota and ecosystems are expected to respond is ocean acidification. Modifications in seawater chemistry, including reduced pH, are resulting due to rising levels of anthropogenic carbon dioxide in the world’s atmosphere and oceans (Rhein et al., 2013). To date, evidence that ocean acidification can affect the physiological function and occurrence of marine taxa comes from various sources including the fossil record (e.g., Clarkson et al., 2015), contemporary field observations at vent and upwelling sites (e.g., Hall-Spencer et al., 2008; Fabricius et al., 2011; Connell et al., 2017), and experimental manipulations (e.g., Dupont et al., 2008; Russell et al., 2009; Falkenberg et al., 2013; Scanes et al., 2014).
While a multitude of studies demonstrating biological effects of ocean acidification has been relatively rapidly amassed, syntheses of the discrete primary research studies can enhance the insight they provide (Hutchison, 1993). Such syntheses are becoming increasingly possible through the development of online databases that host data (e.g., those associated with journals such as Figshare for Frontiers, or that are independent such as Pangaea). A variety of frameworks exist within which such information can then be brought together, including those associated with literature reviews, based on biological theory, and developed in other disciplines. These frameworks can unify ideas, explain observed patterns in internal processes, and facilitate prediction and generation of testable hypotheses (Green et al., 2006).
This qualitative literature review brings together descriptions of the key frameworks that we have observed applied most often, and believe appear most promising, in the context of synthesizing literature regarding biological effects of ocean acidification (Table 1). By taking this approach we have necessarily overlooked some frameworks, such as systems biology approaches (Loewe, 2016) and scale transition theory (Koussoroplis et al., 2017) which, while likely applicable in the context of ocean acidification, have yet to be explicitly considered in this context. In reviewing these disparate approaches together, we hope to provide insight as to how they may be used not only in isolation, but also prompt consideration of how they may be combined in the future. Where data is re-considered in this context, it will be more effectively synthesized and unifying principles identified.
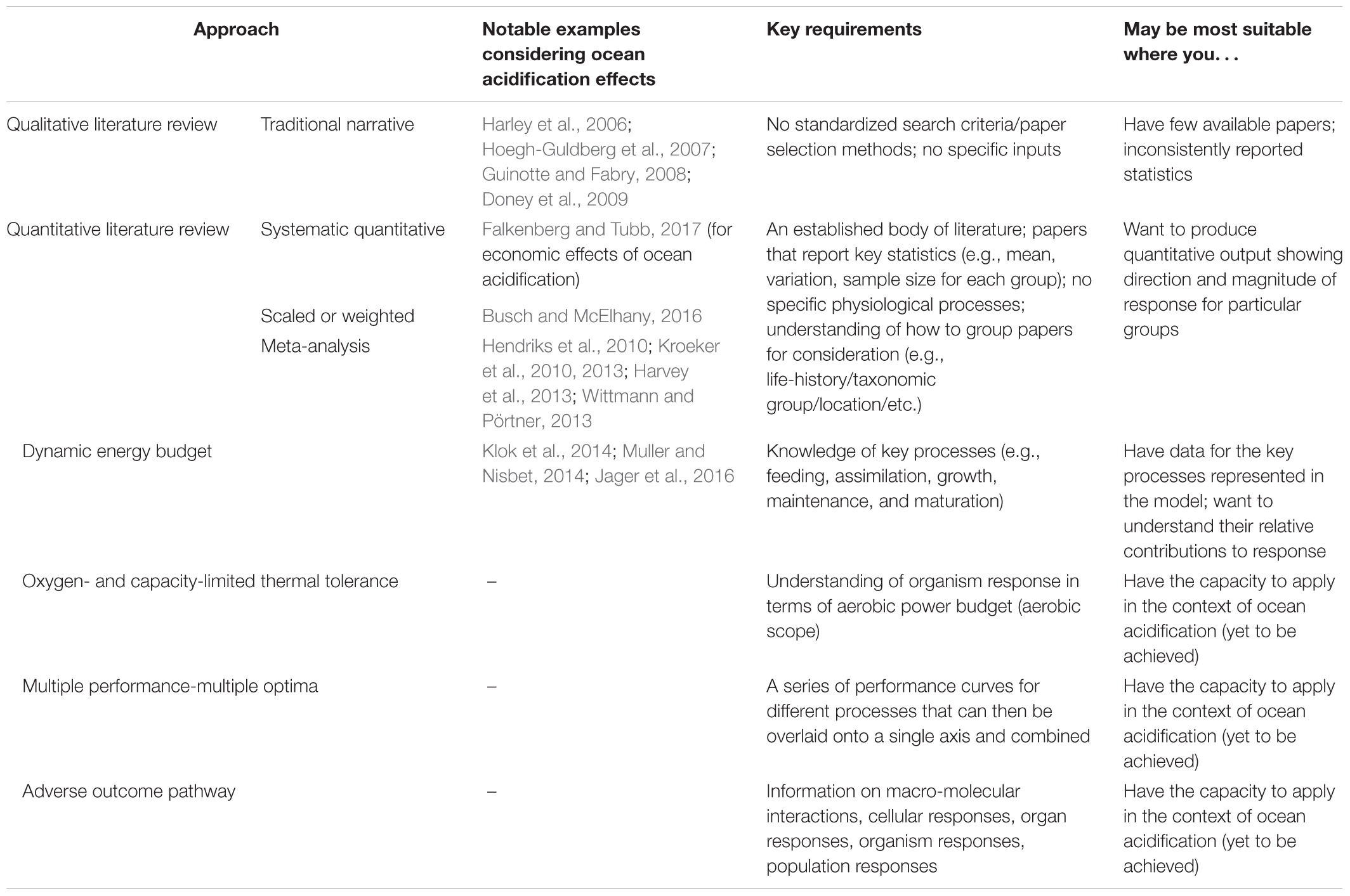
TABLE 1. Summary of key synthesis approaches, notable examples of their application, key requirements, and indication of the context in which they may be most appropriate for use.
Literature Reviews
The frameworks provided by literature review approaches have often been used when synthesizing existing primary research into comprehensive, contextualized overviews (Hutchison, 1993). These approaches range from the traditional qualitative narrative to the quantitative meta-analysis, with each approach having its own features, advantages, and disadvantages.
The first syntheses of ocean acidification effects used a qualitative narrative literature review framework (Figure 1). Under this approach the author(s) reads as much of the existing literature as possible, assesses its relevance, and then compiles what is perceived to be relevant into a compelling account (Green et al., 2006). Where done effectively, narrative literature reviews result in papers that are easy to consume as they are written in a digestible narrative style (Pickering and Byrne, 2014). The resulting qualitative syntheses are, however, also highly subjective, reliant on the author’s expertise and judgement, and not standardized, reproducible, or transparent (Pickering et al., 2014). There are, however, features that can reduce some of these issues. For example, if there is a large author team involved in the expert judgment and decision-making this can enhance the ability to identify all relevant papers and, therefore, confidence in the synthesis. Moreover, if certain aspects of the literature are initially overlooked, these can be identified and incorporated during the peer-review process.
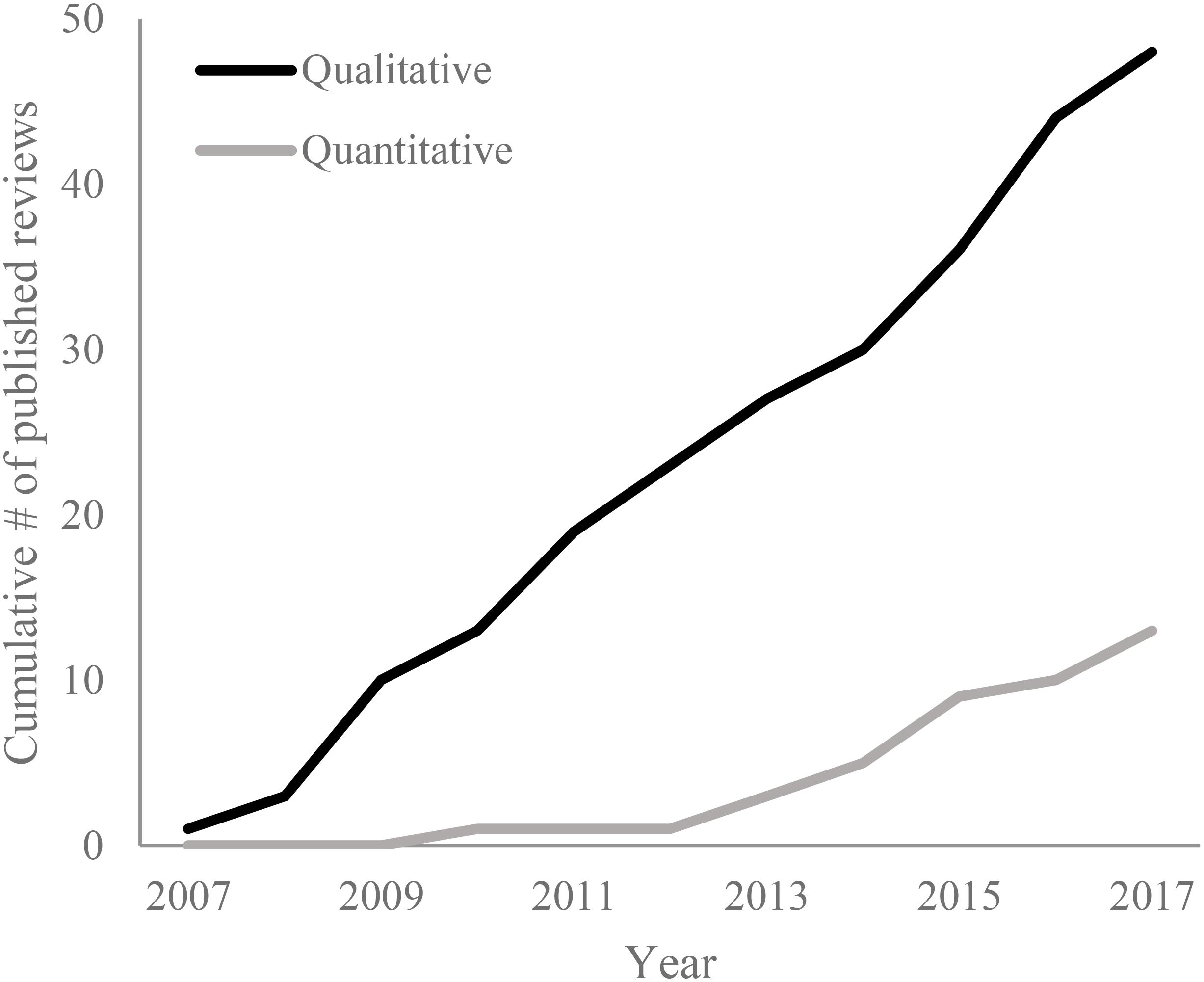
FIGURE 1. Publication pattern of qualitative (narrative) and quantitative (systematic, weighted, and meta-analyses) literature reviews of biological effects of ocean acidification over time (full description is included in-text). Papers were identified by searching Web of Science for documents with TITLE = “ocean acidification” or TOPIC = “ocean acidification” and DOCUMENT TYPE = “review” in October 2017. While this approach does not ensure all relevant literature is identified, it does provide an indication of publishing patterns over time.
Within the context of ocean acidification research, early qualitative reviews typically focused on considering the responses of broad groups of organisms and habitats (e.g., coral reefs in Hoegh-Guldberg et al., 2007, calcifying organisms in Hofmann et al., 2010). With time, these reviews have become more nuanced often considering, for example, particular processes (e.g., acid-base physiology, neurobiology, pharmacology and behavior in Tresguerres and Hamilton, 2017) or specific groups of organisms (e.g., Antarctic sea ice microbial communities in McMinn, 2017). The findings of these more focused reviews can then be used to inform studies or models that consider the changes to specific processes or organisms under environmental change.
In addition to the qualitative approach to literature synthesis, there are also a number of quantitative literature review frameworks gaining traction. Quantitative literature reviews can be separated into three types: systematic, weighted, and meta-analysis (Petticrew and Roberts, 2008; Pickering et al., 2014). In all quantitative review methods, knowledge creation involves searching the literature using clearly defined criteria to identify papers for inclusion to identify a potential direction and magnitude of effect. They do, however, differ in the way data within the papers reviewed are synthesized. That is, in systematic reviews studies are compared quantitatively, with gap analysis included, while in weighted reviews and meta-analyses the studies may be weighted via expert evaluation, with gap analysis either descriptive or not included. Moreover, in systematic approaches, the types of research included can be broader and include methods from the social as well as natural sciences. In all quantitative review approaches, the methods used to identify and select literature are explicit, reproducible, and without a priori assumptions regarding the relevance of identified literature (e.g., Falkenberg and Tubb, 2017). As such, these reviews have been suggested to be more comprehensive and less biased than their qualitative counterparts (Pickering et al., 2014). Bias can, however, still afflict quantitative approaches as a consequence of the need to define search terms and criteria (Hunt, 1997; Rosenthal and DiMatteo, 2001). Moreover, by including all papers the search criteria identify it is possible such frameworks may lead to conclusions of questionable quality (i.e., no “expert judgement” where researchers familiar with the field are involved in selecting, and possibly weighting, the included papers may result in “garbage in and garbage out” as the mixing together of good and bad studies in analyses negtively affects the conclusions drawn; Hunt, 1997; Rosenthal and DiMatteo, 2001). Perhaps the greatest concern, however, centers on the idea that these methods combine results from studies that can vary in important ways (i.e., analagous to combining “apples and oranges” when considering measures such as weights, sizes, flavors, and shelf lives; Hunt, 1997). In the case of biological ocean acidification research, where organism physiology is not incorporated into these approaches (to, for example, separate functional groups or life-history stages), the response of a particular functional group or life-history stage may be masked by combining it with others in analyses (Dupont et al., 2010). Moreover, details of experimental manipulations also need to be taken into account as they can modify predictions of responses; for example, a long exposure may result in a response of a small effect size that could have comparable negative outcomes as a shorter exposure driving a stronger response. Selecting sub-sets of the available data appropriately can, however, be a difficult task due to our lack of mechanistic understanding regarding why certain species respond in the way they do to modified conditions.
While there are still relatively few quantitative literature reviews of ocean acidification in comparison to qualitative reviews, their number is increasing (Figure 1). An early meta-analysis of particular significance is that conducted by Kroeker et al. (2010); within this review effects of ocean acidification on key processes (survival, calcification, growth, photosynthesis, and reproduction) are reported for different groups of organisms (calcifiers – algae, corals, coccolithophores, mollusks, echinoderms, and crustaceans vs. non-calcifiers – fish, fleshy algae, and seagrasses), and life stages (larvae vs. juvenile vs. adult). Another analysis is that by Wittmann and Pörtner (2013); this review evaluated the potential impact of ocean acidification on five animal taxa (corals, echinoderms, molluscs, crustaceans, and fishes). Importantly, they did so in the context of widely used representative concentration pathways (RCPs). Such an approach allowed them to find that corals, echinoderms, and molluscs are more sensitive to RCP 8.5 than are crustaceans. Subsequently, there have been a number of additional meta-analyses conducted with these reviews, similarly to the later qualitative reviews, increasingly focusing on more specific aspects of ocean acidification effects (e.g., bioerosion in Schönberg et al., 2017). Additionally, other quantitative approaches to syntheses are also being conducted and applied, such as that used by Busch and McElhany (2016) in considering the estimates of sensitivity to ocean acidification for functional groups in the California Current ecosystem. Importantly, this review highlighted that while the majority of functional groups responded negatively to ocean acidification, only a few had responses that were consistently negative when uncertainty in sensitivity was considered. The results of this review in particular highlight, therefore, the need to use frameworks which consider the physiological processes underlying species-specific responses to ocean acidification.
Use of Frameworks Drawn From Biological Theory
Biological theory can be used as the basis for synthesis frameworks that explicitly incorporate physiological mechanisms. Such theories in biology and ecology are constructs used to form conceptual, analytical, and computational models that allow us to understand patters in natural systems (Marquet et al., 2014; Gaylord et al., 2015). These constructs can be highly specialized or very general, covering everything from a specific hypothesis to conceptual frameworks for complete fields (Marquet et al., 2014). Despite these differences, all biological theories capture essential features of the system of interest, provide abstracted characterizations, and can be used to organize existing observational and experimental data under a comprehensive conceptual framework to develop explanations and make novel predictions (Marquet et al., 2014). While more commonly invoked when considering other aspects of climate change (e.g., metabolic theory of ecology considered in the context of warming Yvon-Durocher et al., 2010; Mertens et al., 2015; Sampaio et al., 2017; gill-oxygen limitation theory considered in the context of warming in Pauly and Cheung, 2017, described in Pauly, 1981), biological theories are increasingly also used when considering the effects of ocean acidification. Here we outline some key ways in which biological theory may generate insight to ocean acidification’s consequences enabling movement toward a more mechanistic- and hypothesis-driven approach.
Dynamic Energy Budget (DEB)
Frameworks used to consider ocean acidification effects include models developed based on dynamic energy budget (DEB) theory. This formal metabolic theory, and the associated mathematical frameworks, focus on the individual organism and the rates at which energy and essential matter are assimilated for maintenance, growth, reproduction, and development throughout the life cycle in a dynamic environment (Nisbet et al., 2000; Kooijman, 2010). These links are described using differential equations (Nisbet et al., 2000). DEB models can be adapted to describe the dynamics of organism growth and reproduction under different conditions (Pouvreau et al., 2006). This consideration is possible as rates of the key processes depend both on the state of the organism (e.g., age, size, and sex), as well as the state of the environment (e.g., food density, temperature, and carbon dioxide concentrations) (Nisbet et al., 2000). DEB theory does not a priori provide a causal or mechanistic explanation of the underlying cases, but rather provides explanations in hindsight based on empirical findings and patterns observed. Moreover, in addition to describing how individuals acquire and use energy, they can also link to different levels of biological organization such as molecular processes, population dynamics, and (more tenuously) ecosystem dynamics (Nisbet et al., 2000).
To date, notable applications of DEB theory to the issue of ocean acidification have resulted in the creation and parameterization of models considering larval maintenance costs in sea urchins (Jager et al., 2016), shell growth in cockles (Klok et al., 2014), and growth and calcification in coccolithophores (Muller and Nisbet, 2014). When applied to consider larval maintenance in sea urchins, a simplified DEB model (DEBkiss) provided a good explanation of developmental larval traits. The observed ocean acidification effects likely resulted as ocean acidification increased larval maintenance costs (reflected in slower development, increased respiration, but no effects on feeding). However, such effects were specific to age, with older larvae apparently able to compensate for these increased costs to some extent by increasing feeding and/or reducing maintenance rates. The stress factor for ocean acidification showed an apparent tipping point at pH 7.5 (Jager et al., 2016). In the context of cockle shell growth under ocean acidification, a DEB model was used to analyze indirect (metabolic) effects by describing changes in assimilation, maintenance, and growth. While cockle size data alone did not enable differentiation between the processes, by incorporating data for 11 bivalve species, assimilation and maintenance were found to be more relevant than growth (Klok et al., 2014). Finally, for growth and calcification of a coccolithophore, a DEB model predicted that increased ocean acidification will decrease growth and calcification; potential exists that, although these changes are rather modest, they could have implications for marine primary production and biogeochemical carbon cycling (Muller and Nisbet, 2014).
The application of DEB models in the context of ocean acidification can be difficult given that for most organisms data is required that is unavailable or has not been collected; DEB models require species-specific data on multiple physiological processes (such as feeding, absorption efficiency, maintenance, respiration, calcification, growth, and cell composition) (Klok et al., 2014; Muller and Nisbet, 2014; Jager et al., 2016). However, creative application of DEB theory can allow the development of testable hypotheses based on simpler data such as growth curves (as in Jager et al., 2016). Key to exploiting this theory within the context of ocean acidification will, therefore, be conducting targeted experimental manipulations to obtain key data that is currently missing and/or test the resulting hypotheses. Where effectively implemented, this approach is likely to give a reliable indication of the physiological response to modified conditions, moving us closer toward a mechanistic understanding than would be achieved by using literature review approaches. An additional benefit may be that once completed for acidification, the framework could be used to relatively easily incorporate effects of interactions among multiple stressors. Extending the findings from DEB models to other species will, however, be difficult given the species-specific nature of this framework (van der Meer, 2006).
Niche Theory
When developing simplified, readily generalized, biologically based frameworks the theory of the niche may be particularly relevant. An initial description of the niche did so in terms of species’ roles and requirements in communities (Grinnell, 1917; Elton, 1927). A later definition modified the niche to encompass the particular set of resources and environmental conditions exploited by an individual species, which include food, shelter, and climatic tolerances (Hutchinson, 1957). According to this so-called Hutchinsonian characterisation, if a habitat has conditions within a species’ niche, populations that are present should persist without immigration from external sources. In contrast, if conditions are outside the niche populations face extinction (Holt, 2009). While Hutchinson’s definition focusses on species and populations it is also applicable to individual organisms if it is assumed the physiological processes considered are fitness-relevant traits, and fitness would be affected if the processes were impaired or stopped (Leibold, 1995; Bach et al., 2015). Under this assumption, niche theory can be applied to enhance understanding of how patterns of organism function may be affected by environmental change.
Niche theory has recently begun to be used in bringing together existing literature and informing predictions of ocean acidification’s potential effects. For example, the niche concept has been applied in ocean acidification research to account for the experienced natural variability when re-analyzing published literature. That is, Vargas et al. (2017) considered the tested experimental scenario relative to the average or the extreme of the present variability to reconcile otherwise apparently contradictory experimental results. Heterogeneity in responses of different populations was linked to the variability in their native habitats, revealing the potential role of local adaptation and/or adaptive phenotypic plasticity in increasing resilience to environmental change. Niche theory has also been incorporated into a habitat suitability and mechanistic niche model (SS-DBEM) applied in the exploration of the effects of ocean acidification (and warming) on five commercially important fish and mollusk species in the United Kingdom and the resulting ecological, economic, and social impacts (Fernandes et al., 2017). In considering the combined effects of ocean acidification and warming, the bivalve and fish species were found to be affected to an extent that is likely to result in economic losses, although these effects will differ temporally (i.e., England and Scotland most negatively affected in absolute terms, Wales and North Ireland most affected in relative terms).
Oxygen- and Capacity-Limited Thermal Tolerance (OCLTT)
Where the effects of climate change on organism fitness are considered in the context of niche theory, a key derived framework is that of “oxygen- and capacity-limited thermal tolerance” (OCLTT) (Pörtner, 2002, 2010). This integrative concept can characterize the thermal limits to mechanisms that determine both performance and abundance. Specifically, this framework considers the thermal constraints on the capacity for oxygen supply relative to oxygen demand in the organism. Often changes in aerobic scope (the difference between the maximum metabolic rate and standard metabolic rate) have been used as a proxy of the aerobic power budget and indicated the thermal niche of organisms (Pörtner and Peck, 2010; Pörtner et al., 2017). That is, under OCLTT the ability (or inability) of organisms to sustain aerobic scope is a major distinguishing feature of moderate and extreme stress (Sokolova et al., 2012). Where the necessary data regarding the aerobic power balance are obtained and modeled it is anticipated to result in a performance curve whereby a species performs optimally at a particular temperature, above or below which there is a negative effect and performance falls (i.e., Thermal Performance Curve) (Pörtner, 2012). This negative effect on performance is typically marked by a negative energy balance indicated by the disturbance of cellular energy status (Sokolova et al., 2012). The OCLTT framework may enable consideration of the energy-based classification of a range of environmental stressors, as well as the integration of multiple conditions (Pörtner, 2010, 2012; Sokolova et al., 2012; Bozinovic and Pörtner, 2015; Pörtner et al., 2017). Moreover, this framework may be more generally applicable, as support has been found for the OCLTT principles in operation at ecosystem level (by testing niche limits based on minimal routine metabolic scope; Deutsch et al., 2015).
The OCLTT framework is in the relatively early stages of being incorporated into research on organism responses to ocean acidification. The framework has been used as a basis to re-consider published literature and inform a mathematical model of warming effects on Atlantic cod (Gadus morhua) (Holt and Jørgensen, 2015); this is a state-dependent energy allocation model that predicts optimal behavior and life-history strategies in response to environmental temperature. In the context of elevated CO2 literature, results of experiments examining the effects of ocean acidification and hypercapnia have been considered in the context of OCLTT for a range of species (for example, abalone Tripp-Valdez et al., 2017, 2019; scallops Schalkhausser et al., 2013; oysters Parker et al., 2017; crabs, Metzger et al., 2007; Walther et al., 2009; fish Araújo et al., 2018). In the future, care may need to be taken when applying the model of OCLTT to synthesize literature in the context of ocean acidification. For example, where a niche concept limited by temperature is adopted, this will have implications for the temperatures at which ocean acidification experiments are run – it will need to be considered if temperatures reflect a Q10 effect, or if they are becoming limiting due to an ocean acidification effect. Consideration of experimental treatment is also relevant to other synthesis methods, such as the meta-analysis approach outlined above. In addition, while, in certain scenarios, there has been limited support for some of the central assumptions and predictions, it has also been highlighted that the studies finding limited support are also based on subjective interpretations of data and response variables considered (e.g., CTmax) (Clark et al., 2013a,b; Holt and Jørgensen, 2015; Lefevre, 2016; Verberk et al., 2016) (but see also, Farrell, 2013; Pörtner and Giomi, 2013). This body of literature highlights that the interpretation of key elements of the OCLTT framework can, just as with any other framework, shift perception of its utility. Discussion regarding the utility of the concept remains ongoing (see Pörtner et al., 2017, 2018; Jutfelt et al., 2018).
Multiple Performance-Multiple Optima (MPMO)
A framework related to OCLTT is that of multiple performance-multiple optima (MPMO). Whereas OCLTT often uses aerobic scope as a proxy for the overall aerobic power budget, MPMO facilitates the incorporation of various additional physiological processes likely to be influenced by changing environmental conditions, including ocean acidification (Clark et al., 2013a). Under a MPMO framework, aerobic scope (the difference between minimum and maximum oxygen consumption rate; Clark et al., 2013a) is regarded as one of many physiological processes that contribute to organism fitness (with others perhaps including, for example, neural functioning, calcification, feeding, growth rates, and maturation). Each of these processes could have a unique performance curve and optimal condition (Clark et al., 2013a), a pattern which has been tested and discussed in several studies (e.g., Stevenson et al., 1985; Angilletta et al., 2002). Within this framework it would be possible, therefore, to explicitly consider effects of modified conditions on separate physiological functions with differing sensitivities by modeling multiple processes individually (Clark et al., 2013a; Holt and Jørgensen, 2015). Where a common dimension is identified on which the processes can all be represented, the overall curve produced should reflect the conditions preferred for whole organism fitness and response (Clark et al., 2013a). It is possible that this overarching performance curve will align with that of the OCLTT framework where all processes rely on (and compete for) an energy source derived through aerobic metabolism if in steady state. That is, the overall MPMO curve may represent that obtained by considering aerobic scope in isolation (Farrell, 2013).
While MPMO provides a potentially advantageous extension to OCLTT, applying the framework to forecasting biological effects of future changes, including ocean acidification, remains a challenge to be resolved with mathematical representations not yet published. Where such mathematical models are developed, they could enable data in the literature to be used to explore physiological mechanisms underlying responses, providing insight to the how and why of organism responses. Such understanding can, in turn, inform general forecasts (Clark et al., 2013b).
Use of Frameworks From Neighboring Disciplines
The questions currently faced by ocean acidification researchers are similar to those toxicologists have been considering more broadly for decades (reviewed in Hodgson, 2004; Stirling, 2006). Notably, as toxicologists recognize the impossibility of considering all toxicant and species combinations, they have been developing and applying more general frameworks (Hooper et al., 2013; Vinken, 2013). These general techniques could also be used in the field of climate change studies if we consider the changes, such as ocean acidification, to be “stressors” or “toxicants” (as in, for example, Hooper et al., 2013).
Adverse Outcome Pathways (AOPs)
A framework easily transferrable across species and contaminants is the adverse outcome pathway (AOP) (Ankley et al., 2010). In empirical AOPs, a consistent structure and terminology is used to organize data and information on stressor effects. More specifically, an AOP is a linear representation of knowledge about how changes in the environment prompt biological events that lead to one or more causally connected key events, which eventually have an adverse outcome at the level of individual or population (Ankley et al., 2010; Hooper et al., 2013; Segner et al., 2014; Chariton et al., 2016; Figure 2A). The structure of AOPs consists of mechanism-based molecular initiating events (interaction of environmental condition with biota) and a subsequent sequential cascade of responses (key events) across biological levels of organization (cellular, tissue, and organ) to culminate in the impact (or adverse outcome) (Ankley et al., 2010). The adverse outcomes are commonly observed at the individual level (e.g., changes to survival, growth, and reproduction), but can also be defined for populations (Ankley et al., 2010; Groh et al., 2015a). AOPs can then be used to develop mathematical models, which may allow the relationships considered to be more directly quantified (for an example in toxicology see Maxwell et al., 2014). A particular benefit of the AOP framework is that models can be developed even in scenarios where there are uncertainties in the pathway, or the effect of environmental change on a particular step is unclear (Ankley et al., 2010). However, this feature is also linked with one of the key criticisms; these simplified, empirical frameworks do not provide complete representations of complex physiological biological processes (Villeneuve et al., 2014). Similarly, while AOPs directly address questions regarding whether, and how, a particular initiating event can cause an adverse outcome, they do not address what dose of chemical (or other environmental change) will cause sufficient perturbation to drive the pathway to the adverse outcome (Ankley et al., 2010). This knowledge may be necessary when using literature syntheses to address some questions of interest.
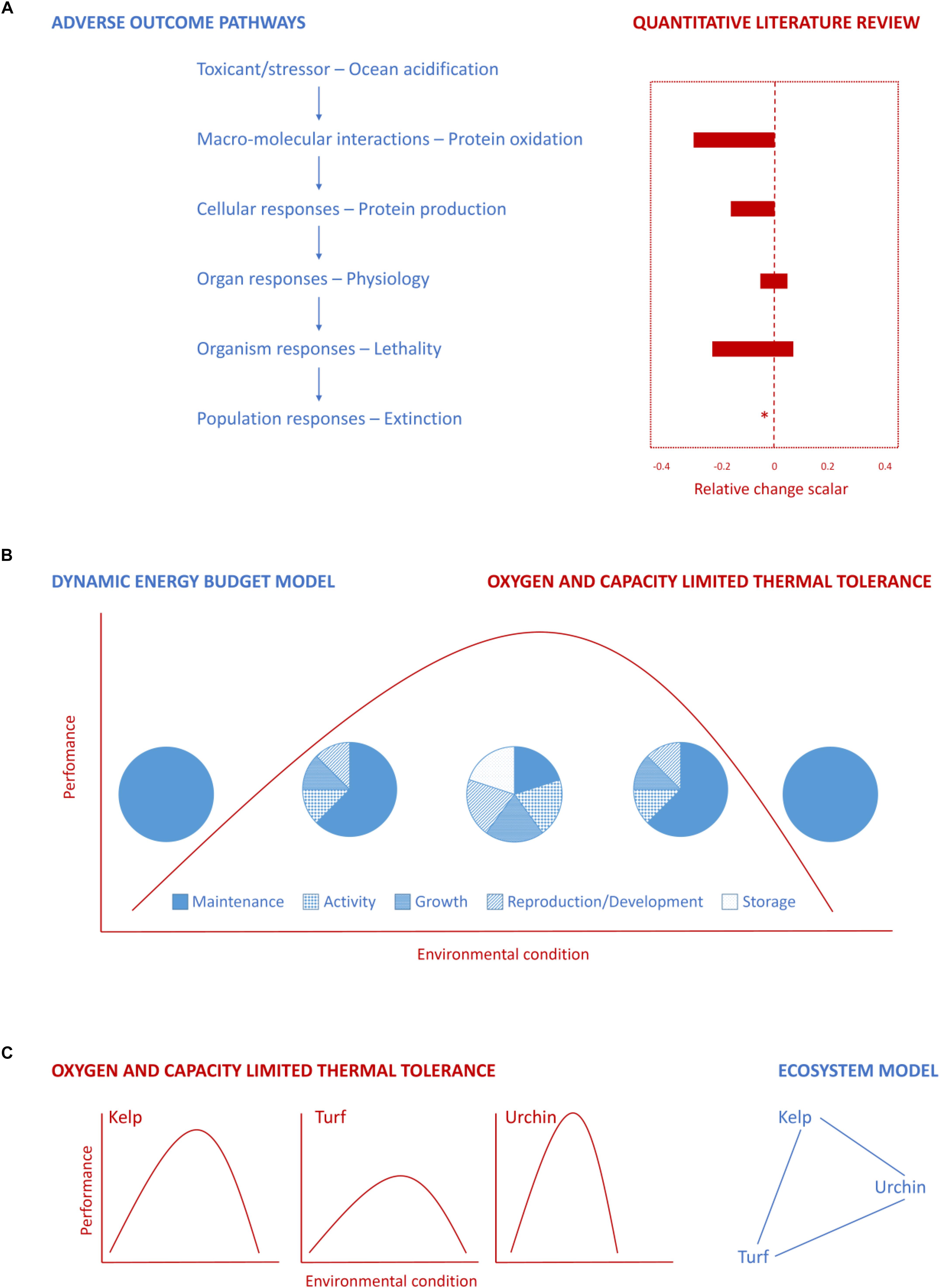
FIGURE 2. Conceptual diagrams illustrating three ways in which different synthesis frameworks could be combined to forecast the potential effects of ocean acidification on physiology, organisms, communities, and ecosystems. (A) Construct an AOP to identify key processes, use this to inform a quantitative literature review about potential effects of ocean acidification. Here we show an example in which these frameworks are used to link the effects of ocean acidification on protein oxidation and production with organ responses, organism lethality, and ultimately population extinction. Some adverse outcomes may not have data and so will not have a relative change indicated by the quantitative literature review, as denoted with an “∗” for “population responses – extinction.” (B) Link energetic supply as understood under the OCLTT framework with allocation and trade-offs in energy allocated to different processes identified via DEB models (based on Sokolova et al., 2012). In this example the frameworks are used to link changes in available energy with its allocation – specifically there is a shift from allocating a relatively large amount of energy to a range of processes under moderate conditions, toward allocating a smaller amount of energy to maintenance in extremes. (C) Use OCLTT to identify the optimum, pejus, pessimum, and lethal conditions and inform an ecosystem model (simplified example shown here) to identify a shift in the dominance/relative abundance of species. This approach could be applied to, as shown here, identify individual performance curves for kelp, turf, and urchins under a range of ocean acidification conditions, which are then used in an ecosystem model to identify how their interactions are modified.
There are examples of the AOP framework being used to consider the effect of other environmental change factors (e.g., warming, altered solar radiation, increased salinity, and hypoxia; Hooper et al., 2013), although it has yet to be used in the context of ocean acidification. For example, an AOP has been created for the study of basal cytotoxicity, or the ability of a chemical substance to damage living cells by compromising the functional and structural features that are related to cellular maintenance. This AOP consists of 3 steps: 1) the molecular initiating event which involves initial cell injury (necrosis, direct mitochondrial inhibition, decompartmentalisation) caused by the chemical and/or its metabolites, 2) the key event whereby a mitochondrial dysfunction, or mitochondrial permeability transition, occurs as a result of the primary insult and, 3) the adverse outcome of cell death (Vinken and Blaauboer, 2017). Where such frameworks are optimized, they could play a larger role in understanding the potential effects of new chemical entities (potentially with less, or no, animal experimentation).
Application of this framework to conditions other than those for which they were initially developed could be relatively simple as AOPs are not necessarily specific to an environmental condition or species. Consequently, if a previously considered environmental change has a similar initiating event, it is possible that the AOP framework could be used for ocean acidification (or a lightly modified AOP) (Hooper et al., 2013). Similarly, established AOPs can be transferred among organisms with similar physiologies (Hooper et al., 2013). This generality enables AOPs to integrate a broad range of obtained information, often in contexts removed from those where the initial observations were made (Hooper et al., 2013; Groh et al., 2015b). As additional detail specific to ocean acidification or the species of concern is obtained AOPs can be modified; these are “living” frameworks reflecting the current state of knowledge (Villeneuve et al., 2014). Additional changes may be made to alter AOPs from their current, stand-alone form to one that represents the interplay between multiple, crossing pathways likely driving the adverse outcome of interest (Vinken, 2013; Groh et al., 2015b).
Developing Links Between Approaches
While we have discussed the approaches largely separately to this point, it is likely that their future combined use will be what leads to development of the most effective understanding of biological responses to environmental change. Such links may be possible where there is understanding of the relevant physiological mechanisms. For example, the development of AOPs will require information from the literature, which can be arranged using the review frameworks (Figure 2A). Such a combination of approaches could be used to link changes in protein oxidation (e.g., altered advanced glycation end products and lipid peroxidation) and production under ocean acidification (as has been observed for the Norway lobster; Hernroth et al., 2012) with organ responses, organism lethality, and ultimately population extinction. Consequently, although review frameworks may have limited utility when considered in isolation, they could be used to inform more complex, mechanistically based approaches (e.g., Muller and Nisbet, 2014).
Another integrative approach may be to incorporate the physiological models of OCLTT with the understanding of energy allocation and trade-offs developed in DEB models; allowing the effects of ocean acidification which manifest in terms of available energy (i.e., aerobic scope) at the physiological level to be linked with trade-offs between processes (e.g., maintenance, reproduction, development, and growth) at the organism level, potentially informing understanding of longer-term, population-level consequences (Figure 2B; detailed in Sokolova et al., 2012 and described below). In the context of ocean acidification, this integration would be anticipated to identify that under unmodified conditions ATP supply via aerobic metabolism is sufficient to cover costs of key processes (e.g., maintenance, activity, growth, and reproduction/development), with any excess energy stored (Sokolova et al., 2012). Under moderate acidification, there may be negative effects of reduced extra- and intra-cellular pH on energy metabolism, as well as increasing the energy requirements for key processes (such as biomineralisation, or acid-base homeostasis). This could reduce the energy available overall, as well as drive energetic trade-offs (Sokolova et al., 2012). Under extreme ocean acidification, the impairment of aerobic metabolism or rise in energetic demand could mean there is insufficient energy to allow the organism to survive (Sokolova et al., 2012). Key to such an approach will be an understanding of energetic supply (e.g., changes in aerobic scope) (OCLTT) and its allocation (to processes such as maintenance, reproduction, development, and growth) (DEB), and how they are modified under ocean acidification scenarios. Where we are able to combine knowledge of these processes, we may move closer to determining the conditions under which a population can survive (even if with lowered reproduction/growth), and those where a population cannot survive.
The synthesis of knowledge regarding multi-stressor responses developed using different approaches, particularly those from contrasting disciplines, will also be beneficial and allow knowledge to be located within a broader context. Where studies on AOPs and life history theory are undertaken together, this could result in mutual progress (Ankley et al., 2010; Groh et al., 2015a). For example, consideration of AOPs and impact pathways in the context of species’ life history could provide a basis from which to improve understanding of phylogenetically driven differences in sensitivity (Ankley et al., 2010). Other frameworks, such as DEB models, could be used to inform individual-based models that incorporate organism effects and community interactions to consider the population and community response, such that they are both more complex and coherent (Grimm et al., 2017). Currently, in regional and earth system models, upscaling of organism response to multiple stressors requires an over-simplification of process representation, thus, the simplified mathematical representations of OCLTT and MPMO thresholds could be used to better parameterize regional and global ecosystem models (Figure 2C). For such an approach to be effectively used, it would be necessary to construct curves over a range of conditions for the relevant processes that are considered in ecosystem models, which could then be run at a series of specified scenarios. Where such links are established it enables forecasts of not only the internal responses of systems to external change, but also the feedbacks (either directional or quantitative) to the system that is providing the driver or stressor. The connections between frameworks will be enhanced where they are linked via mathematical models which can bring together data in the literature to explore physiological mechanisms and make forecasts. Consequently, there is a need for researchers to be aware of the range of frameworks which exist in different areas, and consider how they can be used together.
Conclusion
Reconsidering and synthesizing empirical measures within a structured framework represents a potentially powerful method to develop our capacity to understand and forecast the effects of modified environmental conditions on organism fitness. Here we have highlighted a selection of established and promising approaches; specifically those associated with literature reviews, derived from ecological theory, and borrowed from other disciplines. All the frameworks identified aim to provide a structure within which existing knowledge can be organized, uncertainties and research priorities identified, and predictions for species and ecosystems where empirical data is limited improved. Although we have focused here on the context of ocean acidification research, the approaches identified could be applied more broadly – to consider other fields of climate change research, and also address general issues in biology. Moreover, the inverse also holds. That is, ocean acidification research will likely benefit from incorporating frameworks developed elsewhere. By effectively using these frameworks to synthesize currently disparate results we will move closer to addressing one of the most fundamental questions in biology, specifically what makes species and ecosystems sensitive (or resistant) to changes in their surrounding environment.
Author Contributions
LF, SD, and RB contributed to the conception and structuring of this review. LF wrote the first draft of the manuscript and produced the figures. All authors contributed to manuscript revision, read, and approved the submitted version.
Funding
LF was funded through the Arctic Monitoring and Assessment Programme (AMAP). SD was financially supported by the Linnaeus Centre for Marine Evolutionary Biology at the University of Gothenburg (http://www.cemeb.science.gu.se) and a Linnaeus grant from the Swedish Research Councils VR and Formas. RB was funded through Arctic Monitoring and Assessment Programme (AMAP), Norwegian Research Council projects: “Combined effects of multiple organic stressors from jellyfish blooms and aquaculture operations on seafloor ecosystems” (JELLYFARM; 244572); “Processes and Players in Arctic Marine Pelagic Food Webs – Biogeochemistry, Environment and Climate Change” (MicroPolar; 225956); and “Adapting coastal zone management to ocean acidification” (ACIDCOAST; 519109) and ECNU Marginal seas socio-ecology of the East China Sea (MARSEAS).
Conflict of Interest Statement
The authors declare that the research was conducted in the absence of any commercial or financial relationships that could be construed as a potential conflict of interest.
References
Angilletta, M. J., Hill, T., and Robson, M. A. (2002). Is physiological performance optimized by thermoregulatory behaviour?: a case study of the eastern fence lizard, Sceloporus undulatus. J. Therm. Biol. 27, 199–204. doi: 10.1016/S0306-4565(01)00084-5
Ankley, G. T., Bennett, R. S., Erickson, R. J., Hoff, D. J., Hornung, M. W., Johnson, R. D., et al. (2010). Adverse outcome pathways: a conceptual framework to support ecotoxicology research and risk assessment. Environ. Toxicol. Chem. 29, 730–741. doi: 10.1002/etc.34
Araújo, J. E., Madeira, D., Vitorino, R., Repolho, T., Rosa, R., and Diniz, M. (2018). Negative synergistic impacts of ocean warming and acidification on the survival and proteome of the commercial sea bream, Sparus aurata. J. Sea Res. 139, 50–61. doi: 10.1016/j.seares.2018.06.011
Bach, L. T., Riebesell, U., Gutowska, M. A., Federwisch, L., and Schulz, K. G. (2015). A unifying concept of coccolithophore sensitivity to changing carbonate chemistry embedded in an ecological framework. Prog. Oceanogr. 135, 125–138. doi: 10.1016/j.pocean.2015.04.012
Bozinovic, F., and Pörtner, H.-O. (2015). Physiological ecology meets climate change. Ecol. Evol. 5, 1025–1030. doi: 10.1002/ece3.1403
Busch, D. S., and McElhany, P. (2016). Estimates of the direct effect of seawater pH on the survival rate of species groups in the California Current ecosystem. PLoS One 11:e0160669. doi: 10.1371/journal.pone.0160669
Chapin, F. S., Walker, B. H., Hobbs, R. J., Hooper, D. U., Lawton, J. H., Sala, O. E., et al. (1997). Biotic control over the functioning of ecosystems. Science 277, 500–504. doi: 10.1126/science.277.5325.500
Chariton, A., Sun, M., Gibson, J., Webb, J., Leung, K., Hickey, C., et al. (2016). Emergent technologies and analytical approaches for understanding the effects of multiple stressors in aquatic environments. Mar. Freshw. Res. 67, 414–428. doi: 10.1071/MF15190
Clark, T. D., Sandblom, E., and Jutfelt, F. (2013a). Aerobic scope measurements of fishes in an era of climate change: respirometry, relevance and recommendations. J. Exp. Biol. 216, 2771–2782. doi: 10.1242/jeb.084251
Clark, T. D., Sandblom, E., and Jutfelt, F. (2013b). Response to Farrell and to Pörtner and Giomi. J. Exp. Biol. 216, 4495–4497. doi: 10.1242/jeb.096313
Clarkson, M., Kasemann, S., Wood, R., Lenton, T., Daines, S., Richoz, S., et al. (2015). Ocean acidification and the Permo-Triassic mass extinction. Science 348, 229–232. doi: 10.1126/science.aaa0193
Connell, S. D., Doubleday, Z. A., Hamlyn, S. B., Foster, N. R., Harley, C. D., Helmuth, B., et al. (2017). How ocean acidification can benefit calcifiers. Curr. Biol. 27, R95–R96. doi: 10.1016/j.cub.2016.12.004
Deutsch, C., Ferrel, A., Seibel, B., Pörtner, H.-O., and Huey, R. B. (2015). Climate change tightens a metabolic constraint on marine habitats. Science 348, 1132–1135. doi: 10.1126/science.aaa1605
Doney, S. C., Fabry, V. J., Feely, R. A., and Kleypas, J. A. (2009). Ocean acidification: the other CO2 problem. Annu. Rev. Mar. Sci. 1, 169–192. doi: 10.1146/annurev.marine.010908.163834
Dupont, S., Dorey, N., and Thorndyke, M. (2010). What meta-analysis can tell us about vulnerability of marine biodiversity to ocean acidification? Estuar. Coast. Shelf Sci. 89, 182–185. doi: 10.1016/j.ecss.2010.06.013
Dupont, S., Havenhand, J., Thorndyke, W., Peck, L. S., and Thorndyke, M. (2008). Near-future level of CO2-driven ocean acidification radically affects larval survival and development in the brittlestar Ophiothrix fragilis. Mar. Ecol. Prog. Ser. 373, 285–294. doi: 10.3354/meps07800
Fabricius, K. E., Langdon, C., Uthicke, S., Humphrey, C., Noonan, S., De’ath, G., et al. (2011). Losers and winners in coral reefs acclimatized to elevated carbon dioxide concentrations. Nat. Clim. Chang. 1, 165–169. doi: 10.1038/nclimate1122
Falkenberg, L. J., Russell, B. D., and Connell, S. D. (2013). Future herbivory: the indirect effects of enriched CO2 may rival its direct effects. Mar. Ecol. Prog. Ser. 492, 85–95. doi: 10.3354/meps10491
Falkenberg, L. J., and Tubb, A. (2017). Economic effects of ocean acidification: publication patterns and directions for future research. Ambio 46, 543–553. doi: 10.1007/s13280-017-0895-9
Farrell, A. P. (2013). Aerobic scope and its optimum temperature: clarifying their usefulness and limitations – correspondence on J. Exp. Biol. 216, 2771-2782. J. Exp. Biol. 216, 4493–4494. doi: 10.1242/jeb.095471
Fernandes, J. A., Papathanasopoulou, E., Hattam, C., Queirós, A. M., Cheung, W. W., Yool, A., et al. (2017). Estimating the ecological, economic and social impacts of ocean acidification and warming on UK fisheries. Fish Fish. 18, 389–411. doi: 10.1111/faf.12183
Gaylord, B., Kroeker, K. J., Sunday, J. M., Anderson, K. M., Barry, J. P., Brown, N. E., et al. (2015). Ocean acidification through the lens of ecological theory. Ecology 96, 3–15. doi: 10.1890/14-0802.1
Green, B. N., Johnson, C. D., and Adams, A. (2006). Writing narrative literature reviews for peer-reviewed journals: secrets of the trade. J. Chiropr. Med. 5, 101–117. doi: 10.1016/S0899-3467(07)60142-6
Grimm, V., Ayllón, D., and Railsback, S. F. (2017). Next-generation individual-based models integrate biodiversity and ecosystems: yes we can, and yes we must. Ecosystems 20, 229–236. doi: 10.1007/s10021-016-0071-2
Grinnell, J. (1917). The niche-relationships of the California Thrasher. Auk 34, 427–433. doi: 10.2307/4072271
Groh, K. J., Carvalho, R. N., Chipman, J. K., Denslow, N. D., Halder, M., Murphy, C. A., et al. (2015a). Development and application of the adverse outcome pathway framework for understanding and predicting chronic toxicity: I. Challenges and research needs in ecotoxicology. Chemosphere 120, 764–777. doi: 10.1016/j.chemosphere.2014.09.068
Groh, K. J., Carvalho, R. N., Chipman, J. K., Denslow, N. D., Halder, M., Murphy, C. A., et al. (2015b). Development and application of the adverse outcome pathway framework for understanding and predicting chronic toxicity: II. A focus on growth impairment in fish. Chemosphere 120, 778–792. doi: 10.1016/j.chemosphere.2014.10.006
Guinotte, J. M., and Fabry, V. J. (2008). Ocean acidification and its potential effects on marine ecosystems. Ann. N. Y. Acad. Sci. 1134, 320–342. doi: 10.1196/annals.1439.013
Hall-Spencer, J. M., Rodolfo-Metalpa, R., Martin, S., Ransome, E., Fine, M., Turner, S. M., et al. (2008). Volcanic carbon dioxide vents show ecosystem effects of ocean acidification. Nature 454, 96–99. doi: 10.1038/nature07051
Harley, C. D. G., Randall Hughes, A., Hultgren, K. M., Miner, B. G., Sorte, C. J. B., Thornber, C. S., et al. (2006). The impacts of climate change in coastal marine systems. Ecol. Lett. 9, 228–241. doi: 10.1111/j.1461-0248.2005.00871.x
Harvey, B. P., Gwynn-Jones, D., and Moore, P. J. (2013). Meta-analysis reveals complex marine biological responses to the interactive effects of ocean acidification and warming. Ecol. Evol. 3, 1016–1030. doi: 10.1002/ece3.516
Hendriks, I. E., Duarte, C. M., and Álvarez, M. (2010). Vulnerability of marine biodiversity to ocean acidification: a meta-analysis. Estuar. Coast. Shelf Sci. 86, 157–164. doi: 10.1016/j.ecss.2009.11.022
Hernroth, B., Sköld, H. N., Wiklander, K., Jutfelt, F., and Baden, S. (2012). Simulated climate change causes immune suppression and protein damage in the crustacean Nephrops norvegicus. Fish Shellfish Immunol. 33, 1095–1101. doi: 10.1016/j.fsi.2012.08.011
Hodgson, E. (2004). A Textbook of Modern Toxicology. Hoboken, NJ: John Wiley & Sons. doi: 10.1002/0471646776
Hoegh-Guldberg, O., Mumby, P. J., Hooten, A. J., Steneck, R. S., Greenfield, P., Gomez, E., et al. (2007). Coral reefs under rapid climate change and ocean acidification. Science 318, 1737–1742. doi: 10.1126/science.1152509
Hofmann, G. E., Barry, J. P., Edmunds, P. J., Gates, R. D., Hutchins, D. A., Klinger, T., et al. (2010). The effect of ocean acidification on calcifying organisms in marine ecosystems: an organism-to-ecosystem perspective. Annu. Rev. Ecol. Evol. Syst. 41, 127–147. doi: 10.1146/annurev.ecolsys.110308.120227
Holt, R. D. (2009). Bringing the Hutchinsonian niche into the 21st century: ecological and evolutionary perspectives. Proc. Natl. Acad. Sci. U.S.A. 106, 19659–19665. doi: 10.1073/pnas.0905137106
Holt, R. E., and Jørgensen, C. (2015). Climate change in fish: effects of respiratory constraints on optimal life history and behaviour. Biol. Lett. 11:20141032. doi: 10.1098/rsbl.2014.1032
Hooper, M. J., Ankley, G. T., Cristol, D. A., Maryoung, L. A., Noyes, P. D., and Pinkerton, K. E. (2013). Interactions between chemical and climate stressors: a role for mechanistic toxicology in assessing climate change risks. Environ. Toxicol. Chem. 32, 32–48. doi: 10.1002/etc.2043
Hunt, M. (1997). How Science Takes Stock: The Story of Meta-Analysis. New York, NY: Russell Sage Foundation.
Hutchinson, G. E. (1957). Concluding remarks. Cold Spring Harb. Symp. Quant. Biol. 22, 415–427. doi: 10.1101/SQB.1957.022.01.039
Jager, T., Ravagnan, E., and Dupont, S. (2016). Near-future ocean acidification impacts maintenance costs in sea-urchin larvae: identification of stress factors and tipping points using a DEB modelling approach. J. Exp. Mar. Biol. Ecol. 474, 11–17. doi: 10.1016/j.jembe.2015.09.016
Jutfelt, F., Norin, T., Ern, R., Overgaard, J., Wang, T., McKenzie, D. J., et al. (2018). Oxygen- and capacity-limited thermal tolerance: blurring ecology and physiology. J. Exp. Biol. 221:jeb169615. doi: 10.1242/jeb.169615
Klok, C., Wijsman, J. W., Kaag, K., and Foekema, E. (2014). Effects of CO2 enrichment on cockle shell growth interpreted with a Dynamic Energy Budget model. J. Sea Res. 94, 111–116. doi: 10.1016/j.seares.2014.01.011
Kooijman, S. (2010). Dynamic Energy Budget Theory for Metabolic Organisation. Cambridge: Cambridge University Press.
Koussoroplis, A.-M., Pincebourde, S., and Wacker, A. (2017). Understanding and predicting physiological performances of organisms in fluctuating and multifactorial environments. Ecol. Monogr. 82, 178–197. doi: 10.1002/ecm.1247
Kroeker, K. J., Kordas, R. L., Crim, R. N., and Singh, G. G. (2010). Meta-analysis reveals negative yet variable effects of ocean acidification on marine organisms. Ecol. Lett. 13, 1419–1434. doi: 10.1111/j.1461-0248.2010.01518.x
Kroeker, K. J., Kordas, R. L., Crim, R., Hendriks, I. E., Ramajo, L., Singh, G.S., et al. (2013). Impacts of ocean acidification on marine organisms: quantifying sensitivities and interaction with warming. Glob. Chang. Biol. 19, 1884–1896. doi: 10.1111/gcb.12179
Lefevre, S. (2016). Are global warming and ocean acidification conspiring against marine ectotherms? A meta-analysis of the respiratory effects of elevated temperature, high CO2 and their interaction. Conserv. Physiol. 4:cow009. doi: 10.1093/conphys/cow009
Leibold, M. A. (1995). The niche concept revisited: mechanistic models and community context. Ecology 76, 1371–1382. doi: 10.2307/1938141
Loewe, L. (2016). Systems in evolutionary systems biology. Encycl. Evol. Biol. 4, 297–318. doi: 10.1016/B978-0-12-800049-6.00184-0
Marquet, O. A., Allen, A. P., Brown, J. H., Dunne, J. A., Enquist, B. J., Gillooly, J. F., et al. (2014). On theory in ecology. Bioscience 64, 701–710. doi: 10.1093/biosci/biu098
Maxwell, G., MacKay, C., Cubberley, R., Davies, M., Gellatly, N., Glavin, S., et al. (2014). Applying the skin sensitisation adverse outcome pathway (AOP) to quantitative risk assessment. Toxicol. Vitro 28, 8–12. doi: 10.1016/j.tiv.2013.10.013
McMinn, A. (2017). Reviews and syntheses: ice acidification, the effects of ocean acidification on sea ice microbial communities. Biogeosciences 14, 3927–3935. doi: 10.5194/bg-14-3927-2017
Mertens, N. L., Russell, B. D., and Connell, S. D. (2015). Escaping herbivory: ocean warming as a refuge for primary producers where consumer metabolism and consumption cannot pursue. Oecologia 179, 1223–1229. doi: 10.1007/s00442-015-3438-8
Metzger, R., Sartoris, F. J., Langenbuch, M., and Pörtner, H. O. (2007). Influence of elevated CO2 concentrations on thermal tolerance of the edible crab Cancer pagurus. J. Therm. Biol. 32, 144–151. doi: 10.1016/j.jtherbio.2007.01.010
Muller, E. B., and Nisbet, R. M. (2014). Dynamic energy budget modeling reveals the potential of future growth and calcification for the coccolithophore Emiliania huxleyi in an acidified ocean. Glob. Change Biol. 20, 2031–2038. doi: 10.1111/gcb.12547
Nisbet, R., Muller, E., Lika, K., and Kooijman, S. (2000). From molecules to ecosystems through dynamic energy budget models. J. Anim. Ecol. 69, 913–926. doi: 10.1046/j.1365-2656.2000.00448.x
Parker, L. M., Scanes, E., O’Connor, W. A., Coleman, R. A., Byrne, M., Pörtner, H.-O., et al. (2017). Ocean acidification narrows the acute thermal and salinity tolerance of the Sydney rock oyster Saccostrea glomerata. Mar. Pollut. Bull. 122, 263–271. doi: 10.1016/j.marpolbul.2017.06.052
Pauly, D. (1981). The relationships between gill surface area and growth performance in fish: a generalization of von Bertalanffy’s theory of growth. Meeresforschung 28, 251–282.
Pauly, D., and Cheung, W. W. L. (2017). Sound physiological knowledge and principles in modeling shrinking of fish under climate change. Glob. Change Biol. 24, e15–e26. doi: 10.1111/gcb.13831
Petticrew, M., and Roberts, H. (2008). Systematic Reviews in the Social Sciences: A Practical Guide. Hoboken, NJ: John Wiley & Sons.
Pickering, C., and Byrne, J. (2014). The benefits of publishing systematic quantitative literature reviews for PhD candidates and other early-career researchers. High. Educ. Res. Dev. 33, 534–548. doi: 10.1080/07294360.2013.841651
Pickering, C., Grignon, J., Steven, R., Guitart, D., and Byrne, J. (2014). Publishing not perishing: how research students transition from novice to knowledgeable using systematic quantitative literature reviews. Stud. High. Educ. 40, 1756–1769. doi: 10.1080/03075079.2014.914907
Pörtner, H.-O. (2002). Climate change and temperature dependent biogeography: systemic to molecular hierarchies of thermal tolerance in animals. Comp. Biochem. Physiol. 132, 739–761. doi: 10.1016/S1095-6433(02)00045-4
Pörtner, H.-O. (2010). Oxygen-and capacity-limitation of thermal tolerance: a matrix for integrating climate-related stressor effects in marine ecosystems. J. Exp. Biol. 213, 881–893. doi: 10.1242/jeb.037523
Pörtner, H.-O. (2012). Integrating climate-related stressor effects on marine organisms: unifying principles linking molecule to ecosystem-level changes. Mar. Ecol. Prog. Ser. 470, 273–290. doi: 10.3354/meps10123
Pörtner, H.-O., Bock, C., and Mark, F. C. (2018). Connecting to ecology: a challenge for comparative physiologists? Response to ‘Oxygen- and capacity-limited thermal tolerance: blurring ecology and physiology’. J. Exp. Biol. 221:jeb174185. doi: 10.1242/jeb.174185
Pörtner, H.-O., Bock, C., and Mark, F. C. (2017). Oxygen- and capacity-limited thermal tolerance: bridging ecology and physiology. J. Exp. Biol. 220, 2685–2696. doi: 10.1242/jeb.134585
Pörtner, H.-O., and Farrell, A. P. (2008). Physiology and climate change. Science 322, 690–692. doi: 10.1126/science.1163156
Pörtner, H.-O., and Giomi, F. (2013). Nothing in experimental biology makes sense except in the light of ecology and evolution–correspondence on J. Exp. Biol. 216, 2771-2782. J. Exp. Biol. 216, 4494–4495. doi: 10.1242/jeb.095232
Pörtner, H.-O., and Peck, M. (2010). Climate change effects on fishes and fisheries: towards a cause-and-effect understanding. J. Fish Biol. 77, 1745–1779. doi: 10.1111/j.1095-8649.2010.02783.x
Pouvreau, S., Bourles, Y., Lefebvre, S., Gangnery, A., and Alunno-Bruscia, M. (2006). Application of a dynamic energy budget model to the Pacific oyster, Crassostrea gigas, reared under various environmental conditions. J. Sea Res. 56, 156–167. doi: 10.1016/j.seares.2006.03.007
Rhein, M., Rintoul, S. R., Aoki, S., Campos, E., Chambers, D., Feely, R. A., et al. (2013). “Observations: ocean,” in Climate Change 2013: The Physical Science Basis. Contribution of Working Group I to the Fifth Assessment Report of the Intergovernmental Panel on Climate Change, eds T. F. Stocker, D. Qin, G.-K. Plattner, M. Tignor, S. K. Allen, J. Boschung, et al. (Cambridge: Cambridge University Press).
Rosenthal, R., and DiMatteo, M. R. (2001). Meta-analysis: recent developments in quantitative methods for literature reviews. Annu. Rev. Psychol. 52, 59–82. doi: 10.1146/annurev.psych.52.1.59
Russell, B. D., Thompson, J. A. I., Falkenberg, L. J., and Connell, S. D. (2009). Synergistic effects of climate change and local stressors: CO2 and nutrient-driven change in subtidal rocky habitats. Glob. Change Biol. 15, 2153–2162. doi: 10.1111/j.1365-2486.2009.01886.x
Sampaio, E., Rodil, I., Vaz-Pinto, F., Fernández, A., and Arenas, F. (2017). Interaction strength between different grazers and macroalgae mediated by ocean acidification over warming gradients. Mar. Environ. Res. 125, 25–33. doi: 10.1016/j.marenvres.2017.01.001
Scanes, E., Parker, L. M., O’Connor, W. A., and Ross, P. M. (2014). Mixed effects of elevated pCO2 on fertilisation, larval and juvenile development and adult responses in the mobile subtidal scallop Mimachlamys asperrima (Lamarck, 1819). PLoS One 9:e93649. doi: 10.1371/journal.pone.0093649
Schalkhausser, B., Bock, C., Stemmer, K., Brey, T., Pörtner, H.-O., and Lannig, G. (2013). Impact of ocean acidification on escape performance of the king scallop, Pecten maximus, from Norway. Mar. Biol. 160, 1995–2006. doi: 10.1007/s00227-012-2057-8
Schönberg, C. H., Fang, J. K., Carreiro-Silva, M., Tribollet, A., and Wisshak, M. (2017). Bioerosion: the other ocean acidification problem. ICES J. Mar. Sci. 74, 895–925. doi: 10.1098/rstb.2012.0442
Segner, H., Schmitt-Jansen, M., and Sabater, S. (2014). Assessing the impact of multiple stressors on aquatic biota: the receptor’s side matters. Environ. Sci. Technol. 48, 7690–7696. doi: 10.1021/es405082t
Sokolova, I. M., Frederich, M., Bagwe, R., Lannig, G., and Sukhotin, A. A. (2012). Energy homeostasis as an integrative tool for assessing limits of environmental stress tolerance in aquatic invertebrates. Mar. Environ. Res. 79, 1–15. doi: 10.1016/j.marenvres.2012.04.003
Stevenson, R. D., Peterson, C. R., and Tsuji, J. S. (1985). The thermal dependence of locomotion, tongue flicking, digestion, and oxygen consumption in the wandering garter snake. Physiol. Biochem. Zool. 58, 46–57. doi: 10.1086/physzool.58.1.30161219
Stirling, D. A. (2006). History of toxicology and allied sciences: a bibliographic review and guide to suggested readings. Int. J. Toxicol. 25, 261–268. doi: 10.1080/10915810600746064
Tresguerres, M., and Hamilton, T. J. (2017). Acid–base physiology, neurobiology and behaviour in relation to CO2-induced ocean acidification. J. Exp. Biol. 220, 2136–2148. doi: 10.1242/jeb.144113
Tripp-Valdez, M. A., Bock, C., Lannig, G., Koschnick, N., Pörtner, H. O., and Lucassen, M. (2019). Assessment of muscular energy metabolism and heat shock response of the green abalone Haliotis fulgens (Gastropoda: Philipi) at extreme temperatures combined with acute hypoxia and hypercapnia. Comp. Biochem. Physiol. B Biochem. Mol. Biol. doi: 10.1016/j.cbpb.2018.08.009 [Epub ahead of print].
Tripp-Valdez, M. A., Bock, C., Lucassen, M., Lluch-Cota, S. E., Sicard, M. T., Lannig, G., et al. (2017). Metabolic response and thermal tolerance of green abalone juveniles (Haliotis fulgens: Gastropoda) under acute hypoxia and hypercapnia. J. Exp. Mar. Biol. Ecol. 497, 11–18. doi: 10.1016/j.cbpb.2018.08.009
van der Meer, J. (2006). An introduction to Dynamic Energy Budget (DEB) models with special emphasis on parameter estimation. J. Sea Res. 56, 85–102. doi: 10.1016/j.seares.2006.03.001
Vargas, C. A., Lagos, N. A., Lardies, M. A., Duarte, C., Manríquez, P. H., Aguilera, V. M., et al. (2017). Species-specific responses to ocean acidification should account for local adaptation and adaptive plasticity. Nat. Ecol. Evol. 1:84. doi: 10.1038/s41559-017-0084
Verberk, W. C., Overgaard, J., Ern, R., Bayley, M., Wang, T., Boardman, L., et al. (2016). Does oxygen limit thermal tolerance in arthropods? A critical review of current evidence. Comp. Biochem. Physiol. A Mol. Integr. Physiol. 192, 64–78. doi: 10.1016/j.cbpa.2015.10.020
Villeneuve, D. L., Crump, D., Garcia-Reyero, N., Hecker, M., Hutchinson, T. H., LaLone, C. A., et al. (2014). Adverse outcome pathway (AOP) development I: strategies and principles. Toxicol. Sci. 142, 312–320. doi: 10.1093/toxsci/kfu199
Vinken, M. (2013). The adverse outcome pathway concept: a pragmatic tool in toxicology. Toxicology 312, 158–165. doi: 10.1016/j.tox.2013.08.011
Vinken, M., and Blaauboer, B. J. (2017). In vitro testing of basal cytotoxicity: establishment of an adverse outcome pathway from chemical insult to cell death. Toxicol. Vitro 39, 104–110. doi: 10.1016/j.tiv.2016.12.004
Walther, K., Sartoris, F. J., Bock, C., and Pörtner, H.-O. (2009). Impact of anthropogenic ocean acidification on thermal tolerance of the spider crab Hyas araneus. Biogeosciences 6, 2207–2215. doi: 10.5194/bg-6-2207-2009
Wittmann, A. C., and Pörtner, H.-O. (2013). Sensitivities of extant animal taxa to ocean acidification. Nat. Clim. Chang. 3, 995–1001. doi: 10.1038/nclimate1982
Keywords: adverse outcome pathway, dynamic energy budget, literature review, multiple performance-multiple optima, ocean acidification, oxygen and capacity limited thermal tolerance
Citation: Falkenberg LJ, Dupont S and Bellerby RGJ (2018) Approaches to Reconsider Literature on Physiological Effects of Environmental Change: Examples From Ocean Acidification Research. Front. Mar. Sci. 5:453. doi: 10.3389/fmars.2018.00453
Received: 07 May 2018; Accepted: 12 November 2018;
Published: 29 November 2018.
Edited by:
Christopher Edward Cornwall, Victoria University of Wellington, New ZealandReviewed by:
Nova Mieszkowska, Marine Biological Association of the United Kingdom, United KingdomAstrid C. Wittmann, Alfred Wegener Institut Helmholtz Zentrum für Polar und Meeresforschung, Germany
Hans O. Poertner, Alfred Wegener Institut Helmholtz Zentrum für Polar und Meeresforschung, Germany
Copyright © 2018 Falkenberg, Dupont and Bellerby. This is an open-access article distributed under the terms of the Creative Commons Attribution License (CC BY). The use, distribution or reproduction in other forums is permitted, provided the original author(s) and the copyright owner(s) are credited and that the original publication in this journal is cited, in accordance with accepted academic practice. No use, distribution or reproduction is permitted which does not comply with these terms.
*Correspondence: Laura J. Falkenberg, bGF1cmFmYWxrZW5iZXJnQGN1aGsuZWR1Lmhr
†Present address: Laura J. Falkenberg, Simon F. S. Li Marine Science Laboratory, School of Life Sciences, The Chinese University of Hong Kong, Shatin, China