- 1Posgrado en Ciencias del Mar y Limnología, Universidad Nacional Autónoma de México, Mexico City, Mexico
- 2Unidad Académica de Sistemas Arrecifales Puerto Morelos, Instituto de Ciencias del Mar y Limnología, Universidad Nacional Autónoma de México, Puerto Morelos, Mexico
Coral reefs are affected by the deterioration of the oceans due to global warming and other anthropogenic perturbations, increasing the frequency, and severity of bleaching and disease. To overcome some of these conditions, reef corals and other cnidarians rely on a mucus layer housing a diverse community of beneficial microorganisms and mechanisms of innate immune response. The antimicrobial defense has been associated with the bacterial community in these organisms, but the potential antimicrobial activity of the mucus layer itself has not been explored fully. We hypothesized that the bacteria-free mucus layer of different cnidarians would show differential and specific antimicrobial and immunological responses when challenged with two potentially pathogenic bacteria. We evaluated this capability through antimicrobial properties, immune response and biochemical composition of the mucus. Results clearly showed that the mucus of healthy cnidarians has the capability of inhibiting the growth of Serratia marcescens and Aurantimonas sp. in a species-specific way that includes differences in the potency of the response. The anemone Exaiptasia pallida was particularly potent against Aurantimonas sp. while the coral Pseudodiploria strigosa and the medusa Cassiopea xamachana had similar capabilities against both bacterial strains. In coral affected by black band disease, this antibacterial capability diminished in the mucus layer, but the associated bacteria remained potent. Results showed that hydroxyproline and phenoloxidase increased in the transition zone of diseased corals, although melanin was not detected in any of the animals tested. Bleaching of anemones and medusas also diminished the antibacterial capability of the surface mucus layer, but in anemones, the associated bacteria did not show a significant reduction in their ability to inhibit the growth of the bacterial strains. The mucus of bleached medusas showed an increased inhibitory activity against Aurantimonas sp. that may be associated with a specific bacterial strain we isolated. Mucus collected from bleached anemones and medusas did not show a significant immune response. In this work, we show that the surface mucus layer itself has antibacterial properties not associated with the bacteria this layer houses; such properties diminished due to disease or bleaching, while immunological responses increased in the mucus of diseased animals.
Introduction
As many aquatic invertebrates, reef cnidarians possess a complex surface layer (SML) of mucopolysaccharides that functions as the interface between the animal tissue and the external environment, constituting a physicochemical and physiological barrier (Brown and Bythell, 2005; Wahl et al., 2012). This layer houses a microbial community mostly composed of bacteria, that varies depending on the animal species, geographic location, physiological status, nutrition, and health (Rohwer et al., 2002; Ainsworth et al., 2006; Koren and Rosenberg, 2006; Longford et al., 2007; Wahl et al., 2012; Thompson et al., 2015). Nevertheless, a recent study on the bacterial community of the model organism Exaiptasia pallida strongly suggests that this bacterial community may be more related to environmental conditions than to geographic location, animal species or symbiont type (Brown et al., 2017). Studies that have sequenced this community show that it differs from bacterial communities in the adjacent water column and the sediments, suggesting some degree of specificity (Rohwer et al., 2002; Brown and Bythell, 2005). Further, it has been demonstrated that the bacterial community that colonizes newly settled coral polyps has a composition apparently related to the host identity (Sharp et al., 2010). In addition, microbes in the SML alter the immune system by stimulating specific responses, and the immune system influences the microbial composition in return (Wahl et al., 2012; Shöder and Bosch, 2016).
The surface mucus layer represents a biofilm that provides various substrates for a complex microbial community. This layer also contains bioactive molecules with antimicrobial activity (some produced by resident bacteria). Then, the SML represents the first defense against potential pathogens, contributing to the function and composition of the microbial community it supports. As an important observation, microscopic examination of the coral epidermis beneath the mucus layer reveals a nearly sterile environment (Johnston and Rohwer, 2007). Further, the presence of the SML reduces fouling or settlement of unwanted microbes on cnidarian surfaces. The mucosal surface in other invertebrates has a role in chemical defense as part of the innate immune system (Abbas et al., 2007), defending the host organism with diverse substances that lyse potentially pathogenic bacterial cells, sequester microbial nutrients or act as decoy to bind and trap microorganisms (Fleming, 1922; Cole et al., 1999).
Mucosal surfaces appeared for the first time in animal evolution in Cnidaria (Bosch and McFall-Ngai, 2011). In Hydra, several studies have determined that different species house different microbial communities, strongly suggesting a specific interaction of the SML with potential microbial commensals and the environment (Fraune and Bosch, 2007; Franzenburg et al., 2013). Actually, the study of immunology in cnidarians initiated with studies of Hydra and later of Nematostella, that identified a lack of specific immune cells, thus implicating other mechanisms for their defense (reviewed in Augustin and Bosch, 2010; Ocampo and Cadavid, 2015). Additionally, cnidarians generate antimicrobial peptides (AMPs) like aurelin in the medusa Aurelia aurita (Ovchinnikova et al., 2006), hydramycin in Hydra (Jung, 2009) or damicornin in the coral Pocillopora damicornis (Vidal-Dupiol et al., 2011). They also have bacterial recognition receptors, in particular, Nod-like receptors that activate cell death, so that apoptosis seems to be important in the innate immune response (Augustin and Bosch, 2010). Other responses include melanin encapsulation of microbial cells catalyzed by phenoloxidase (Petes et al., 2003; Mydlarz et al., 2008), coagulation, and immune cell activation after disease and injuries, all of which have been defined as key components of this defense (Mydlarz et al., 2010; Palmer and Taylor-Knowles, 2012; Ocampo and Cadavid, 2015). All these immunological responses are located within live tissues, but there have been no assessments of immunological activity of the mucus layer in Cnidarians. However, a recent study of untreated mucus collected from the reef zoanthid Palythoa caribaeorum indicated the presence of bioactive compounds like lectins and proteolytic enzymes (Camargo Guarnieri et al., 2018). Further, scleractinian corals release some antibacterial compounds upon mechanical stress (Greffen and Rosenberg, 2005).
Environmental and anthropogenic perturbations have important consequences on coral health, because they modify the associated microbial community (e.g., increase under nutrient enrichment and decrease after bleaching). Although these responses suggest a dynamic community, such variations may allow for the infection by pathogens, compromising the health of the animals due to the loss of the protective qualities of the SML (Ritchie, 2006; Sekar et al., 2006; Bourne et al., 2007; Vega Thurber et al., 2009; Augustin and Bosch, 2010; Mydlarz et al., 2010; Thompson et al., 2015). Interestingly, no diseases have been reported for the model organisms Cassiopea xamachana or E. pallida. It is possible that these symbiotic cnidarians do get diseased, but soon die or are eaten by other animals.
The susceptibility of corals to disease and bleaching has been inversely correlated with the investment in protection when assessed by variables commonly associated with invertebrate immunity, such as the size of melanin-containing granular cells, phenoloxidase activity, and the concentration of fluorescent proteins (Palmer et al., 2010). The investment of energy in immune mechanisms can translate to the species being more resistant to perturbations such as high temperature. In addition, thickness of the tissue and the mucus layer have been found to relate to the temperature tolerance of corals and to disease susceptibility (Glynn and D’Croz, 1990; Fitt et al., 2000, 2009; Green et al., 2008). Further, reproductive patterns and colony morphology in closely related coral species can vary the levels invested in immunity (Pinzón et al., 2014b); and in terms of evolutionary history, older coral lineages have a lower number of diseases and disease prevalence than more recently diverged lineages (Pinzón et al., 2014a). Within the Cnidaria, different taxonomic groups may, therefore, show important differences in the defensive capabilities of their SML, in addition to species-specific differences in resources allocated to immune defense, as well as variations in the composition of their microbial communities. Our hypothesis is that there is a differential, species-specific capability of the SML to help fight pathogens, not related to the bacterial community it houses. With this in mind, we included distant relatives in our experimental approach.
Although the antimicrobial function of the SML has been usually assigned to the microbial community housed by this layer (Gil-Turnes and Fenical, 1992; Castillo et al., 2001; Rypien et al., 2010), the potential antimicrobial activity of this layer per se, has not been explored fully (Ritchie, 2006). Therefore, the purpose of this study was to experimentally evaluate possible differences in the capabilities of the SML associated with different taxonomic groups within the Cnidaria when challenged by two potentially pathogenic bacterial strains. We hypothesize that the three cnidarians we selected, a coral (P. strigosa), an anemone (E. pallida) and a medusa (C. xamachana), will demonstrate different and specific protective capabilities of the SML. The bacterial pathogens we tested were identified as causative of diseases in certain coral species (Richardson et al., 1998; Patterson et al., 2002; Denner et al., 2003), but not associated with black band disease or bleaching. Our experimental approach included assays employing the surface mucus layer complex (MC), mucus layer free of bacteria (SML), and isolated bacteria from the CM (BAM), against two tester bacterial strains. We also looked at some of the innate immune activity by measuring hydroxyproline (Hyp), phenoloxidase activity (PO), and melanin. We collected mucus from three symbiotic cnidarians, healthy and affected by disease or bleaching, to contrast the responses.
Materials and Methods
Animals and Sampling Conditions
Medium size (25 cm minimum diameter, 25 cm minimum high) coral colonies of P. strigosa, healthy or visibly affected with black band disease (BBD), were sampled from the back reef at Petempich location in the Puerto Morelos Reef National Park, México. Colonies from 3 to 5 m deep were sampled between October and November 2017. Mucus was collected from 6 colonies each, in triplicate samples, with sterile syringes after softly disturbing the surface layer with a tool (similar to a microbiological spreader) and stored in an ice chest to be processed in the lab. For diseased colonies, two areas were sampled: the transition zone (2–4 cm from the lesion band) and the apparently healthy-looking tissue (15 cm away from the band). E. pallida anemones were collected from the water pipes of the aquaria system at our department facilities. Bleaching of anemones was produced after cold shocks (Muscatine et al., 1991; Estes et al., 2003). Bleached anemones were kept in the dark for 2–3 weeks before being sampled. The Xcaret Park donated healthy and bleached C. xamachana medusas. Mucus from these animals was collected after rinsing the animals in sterile filtered seawater; after 10–20 min the liberated mucus was collected with a syringe.
Mucus Treatments
All mucus samples were centrifuged twice (20 min in a clinical centrifuge, 5 min in a microcentrifuge) at maximum speed to remove excess seawater, with a short vortex agitation in between. Mucus collected from the different animals was experimentally processed to compare the antibacterial activity of the mucus layer itself and that of the associated bacteria. Each sample was distributed in three microtubes for the following treatments: (1) Sterilization by irradiating for 20 min under UV-light (λ = 254 nm; Krediet et al., 2009), named SML. (2) Bacteria that were cultured after inoculating 1 mL of marine broth with 10 μL of mucus, grown overnight at 27°C and used in further tests, named MAB. (3) Mucus without treatment named MC. Sterilization of the mucus (treatment SML) was corroborated by incubating an aliquot on marine-agar at 27°C, overnight, and checking for the absence of bacterial growth.
Antibacterial Activity
We employed a swarming assay to evaluate the antimicrobial activity of the three mucus treatments. We first used a marine-agar layer (15 g L-1 of agar) which was left 12 h to settle. Glass fiber discs embedded in 10 μL of mucus or 7 μL of cultured bacteria were placed over this layer. Next, a second cooler (48°C), softer marine-agar layer (7 g L-1) with an inoculum (100 μL of overnight culture) of each tester bacteria, was poured over and left to settle for 30 min. Plates were incubated at 27°C in a controlled culture chamber. Growth measurements were taken after the growth of the tester bacteria had formed a confluent lawn on the medium which was about 16 h for Serratia marcescens and 48h for Aurantimonas sp. We scored the presence/absence of an inhibition zone formed around each filter. As a negative control we used embedded filters in filtered seawater and for positive control, embedded filters in one of two wide-spectrum antibiotics, ciprofloxacin or ceftriaxone (at 100 mg mL-1). Assays were done in triplicate.
Immune Response Assessment
The immune response was assessed by measuring the content of hydroxyproline and melanin, and the activity of phenoloxidase. The presence of collagen was evaluated by quantification of the unique amino acid Hyp following the protocol described in Hofman et al., 2011. We processed 10 μL of each mucus sample, with three technical replicates, to be read in a Biospectrometer (Eppendorf, United States) at 540 nm. Calculations were performed against the standard Trans-4-hydroxy-L-proline. For melanin quantification, we followed a protocol described in Mydlarz and Palmer, 2011 using 10 μL of mucus for each sample, with three technical replicates. Readings in the spectrophotometer were at 410 and 490 nm to distinguish between two forms of melanin, employing pure melanin (SIGMA) as a standard. Phenoloxidase (PO) activity was measured according to Mydlarz and Palmer, 2011, using 10 μL of mucus from each sample, with three technical replicates; data are presented as change in absorbance A490 mg protein-1min-1.
Biochemical Determinations
A basic biochemical determination of the mucosal surface included the total content of protein, carbohydrates, and lipids. Total protein in mucus from each sample (10 μL) was estimated by the Bradford method against a standard curve with BSA, according to the manufacturer (SIGMA). For the estimation of total lipids, we followed the colorimetric method of sulfo-phospho-vanillin as described (Mishra et al., 2014), using 10 μL of sample and readings at 530 nm after processing. Total carbohydrates were estimated by a colorimetric sulfuric-phenol assay (Dubois et al., 1951) with 10 μL of mucus sample. Readings were done at 490 nm and normalized to a standard curve prepared with glucose. All assays had three technical replicates.
Statistical Analyses
Antibacterial activity of mucus samples against tester bacteria and among the condition of animals (diseased or bleached) was analyzed by the exact Fisher test, due to the qualitative nature of the results (nominal variables) and the sample size used (n = 6). To compare the different treatments of the mucus layer, we employed the Cochran Q analysis, using Q0 > χ2 = 5.99 of significance. For the comparison of the immunological responses -hydroxyproline content and phenoloxidase activity- as well as the biochemical composition of the mucus samples, we employed a Wilcoxon test. This type of analysis was applied since the data were not normally distributed as evaluated by a graphical test with the software R Project. In all tests, statistical significance was established at p < 0.05.
Results
Assessment of Protective Capabilities of the Mucus Layer in the Coral P. strigosa
To evaluate the antibacterial capability of the mucus layer in corals, anemones, and medusas, we conducted swarming assay experiments employing three differentially treated mucus samples: (1) mucus layer complex without treatment, or CM; (2) UV-sterilized mucus layer without bacteria, or SML; and (3) bacteria isolated and cultured from the mucus layer, or MAB. We tested this capability against the growth of two potentially pathogenic bacterial strains. In coral colonies, we sampled the mucus layer as follows: (1) on healthy colonies, and on diseased colonies in two positions, (2) close to the lesion (transition zone), and (3) away from the lesion (healthy-looking). Results showed that in healthy P. strigosa colonies, only coral mucus free of bacteria (SML) inhibited significantly the growth of S. marcescens, while the same assay against Aurantimonas sp. showed an inhibitory effect that was similar for all the differentially treated mucus (Table 1). The inhibitory capability in the mucus collected from the transition zone in diseased colonies was lost in the SML, but the MAB and CM treatments showed a positive inhibition of S. marcescens. However, only MAB treated mucus showed some inhibition against Aurantimonas sp. (Table 1). Mucus collected from healthy-looking tissues in diseased corals had a similar inhibitory effect on the growth of S. marcescens for all mucus treatments, but against Aurantimonas sp. the mucus only fraction (SML) apparently lost its inhibitory effect, although this was not statistically significant (Table 1). As controls, filtered seawater had no effect against the growth of both tester bacteria while the antibiotics ciprofloxacin and ceftriaxone inhibited completely the growth of both tester bacteria (data not shown).
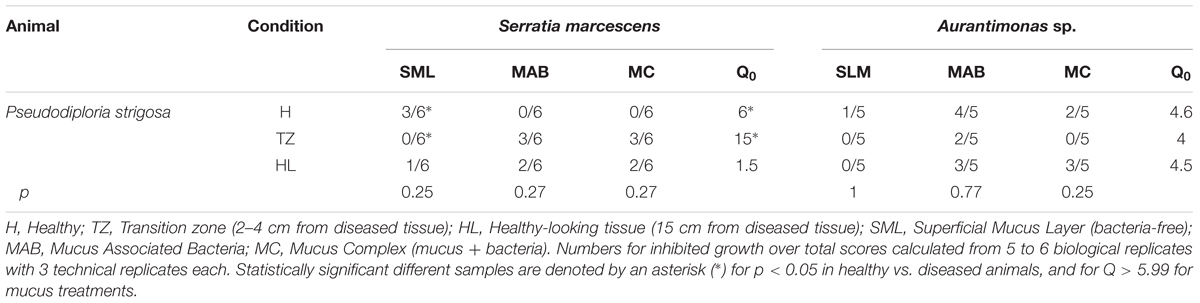
TABLE 1. Frequency for the presence of inhibition growth zone of treated mucus collected from healthy or diseased Pseudodiploria strigosa (coral). Treated mucus was offered to plated tester strains Serratia marcescens and Aurantimonas sp., their growth inhibition scored after 16 and 48 h, respectively.
To assess the immune response of the mucus surface layer in all three animals, we measured the relative concentration of Hyp and the activity of PO in this layer. We also measured melanin content, but values were below the limit of detection of the assay (results not shown). Mucus collected from healthy coral colonies had a lower concentration of Hyp than mucus collected from the transition zone of diseased colonies, while mucus collected away from the affected area in diseased colonies had intermediate levels of Hyp (Figure 1A). No statistically significant differences were detected among the three sampled tissues, probably due to the dispersion of the data, in particular in mucus sampled from the transition zone. The results for the activity of phenoloxidase in mucus from healthy and diseased coral colonies were similar to these results (Figure 1B).
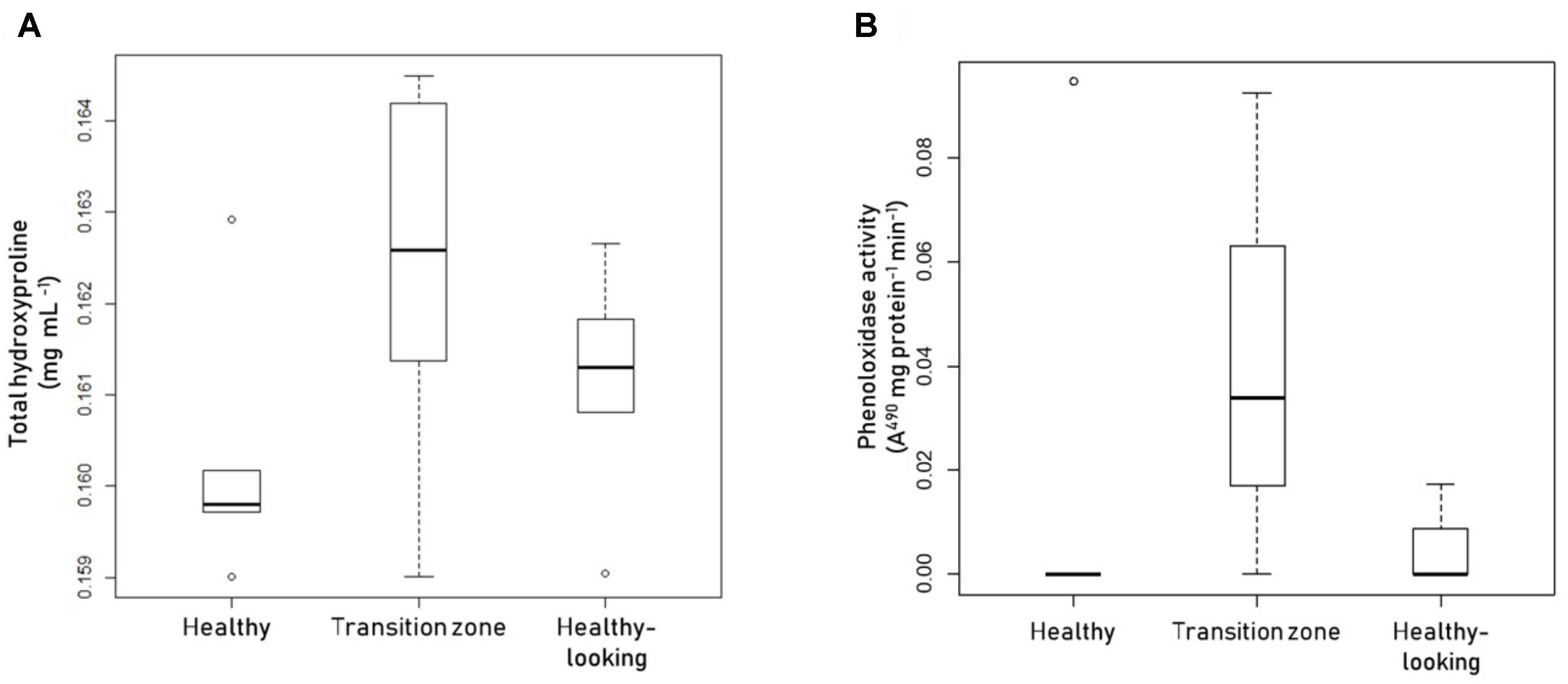
FIGURE 1. Assessment of the immunological response of the mucus complex in coral colonies. Boxplots showing (A) total hydroxyproline or (B) phenoloxidase activity levels in mucus samples collected from healthy Pseudodiploria strigosa colonies (left), and colonies affected by black band disease, collected above the transition zone (center), or above healthy-looking tissues (right). Dots represent atypical data. Data from 5 independent determinations, with three technical replicates each.
We evaluated if the antibacterial and immunological responses of the different animals considered could be associated with the composition of the mucus layer. With this in mind, we compared the biochemical composition of the mucus surface layer from healthy to diseased coral colonies. Results showed that the total content of proteins, lipids, and carbohydrates was not significantly different in corals under three different conditions: healthy, close (transition zone) and away (healthy-looking) from the lesion (Table 2).
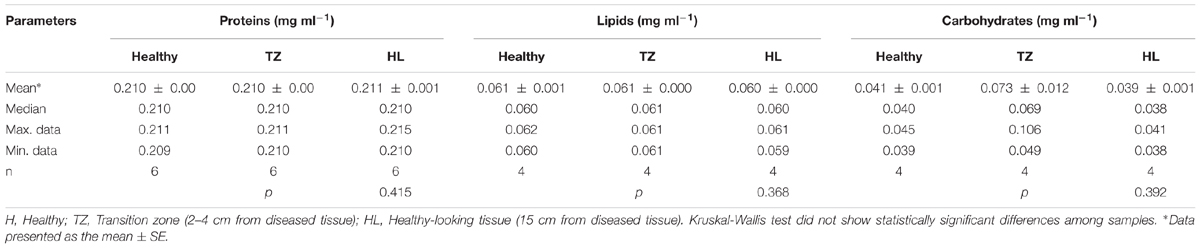
TABLE 2. Total protein, lipid and carbohydrates in mucus samples collected from P. strigosa, healthy and affected by black band disease, on the transition zone and on healthy looking tissues.
Assessment of Protective Capabilities of the Mucus Layer in the Medusa C. xamachana and the Anemone E. pallida
We also evaluated the antibacterial capability of the mucus layer from medusas and anemones, healthy and bleached. The mucus free of bacteria (SML) of healthy medusa showed some inhibitory effect against the growth of both tester strains (Table 3). In bleached medusas, the only positive, significant inhibitory activity was observed for the untreated mucus fraction (MC) against Aurantimonas sp. The mucus collected from healthy anemone E. pallida (Table 3) showed the highest inhibitory effect against both tester bacteria, except for mucus free of bacteria (SML) against S. marcescens, which showed no inhibitory effect on its growth. In mucus collected from bleached anemone, results were similar to healthy animals, except for a significantly higher inhibitory effect in the mucus complex (MC) against Aurantimonas sp. The results of controls for medusa and anemone assays were identical to the controls used in coral assays (data not shown).
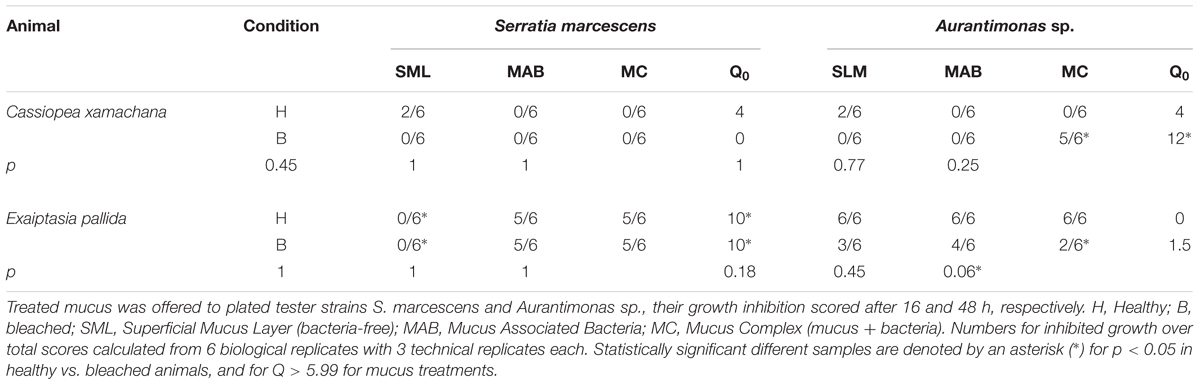
TABLE 3. Frequency for the presence of inhibition growth zone of treated mucus collected from healthy or bleached Exaiptasia pallida (anemone) and Cassiopea xamachana (medusa).
The immunological response of the mucus layer in medusas and anemones, healthy and bleached, was also evaluated. In mucus collected from medusas, the concentration of Hyp was not significantly different between healthy and bleached animals (Table 4). The activity of PO in healthy medusa was higher and the data more dispersed than in bleached medusa, although not statistically different (Table 4). In anemones, we did not measure significantly different levels of Hyp or PO between healthy and bleached animals, although average values for Hyp in bleached animals were higher (Table 4), as in bleached medusa.
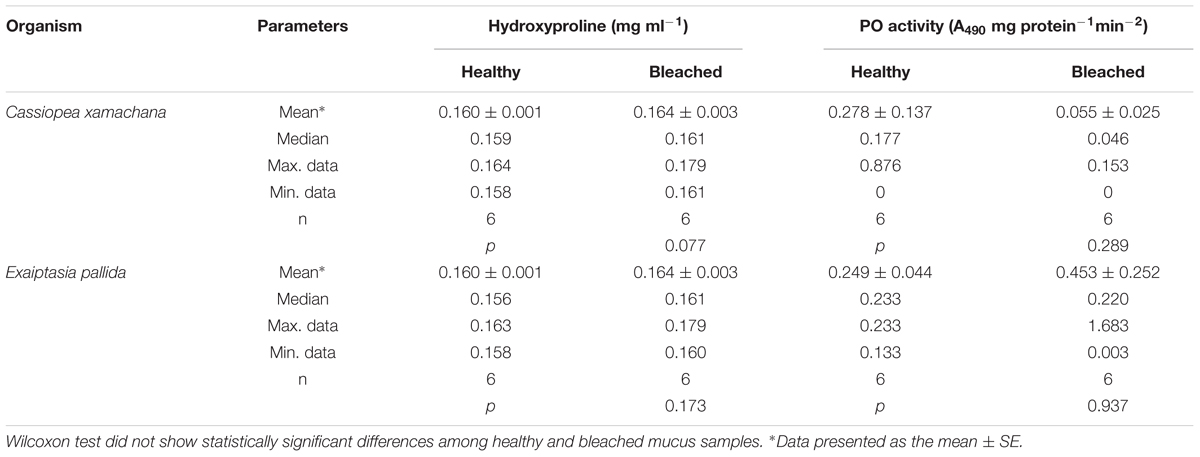
TABLE 4. Total hydroxyproline and phenoloxidase (PO) activity levels of the mucus complex samples collected from healthy and bleached C. xamachana and E. pallida.
The composition of the mucus layer from medusas and anemones indicated that in mucus collected from medusa, total protein and lipid content were significantly lower in bleached animals (Table 5). By contrast, in anemones, we measured significantly lower levels of protein and carbohydrates but similar lipid levels in bleached compared to healthy animals (Table 5).
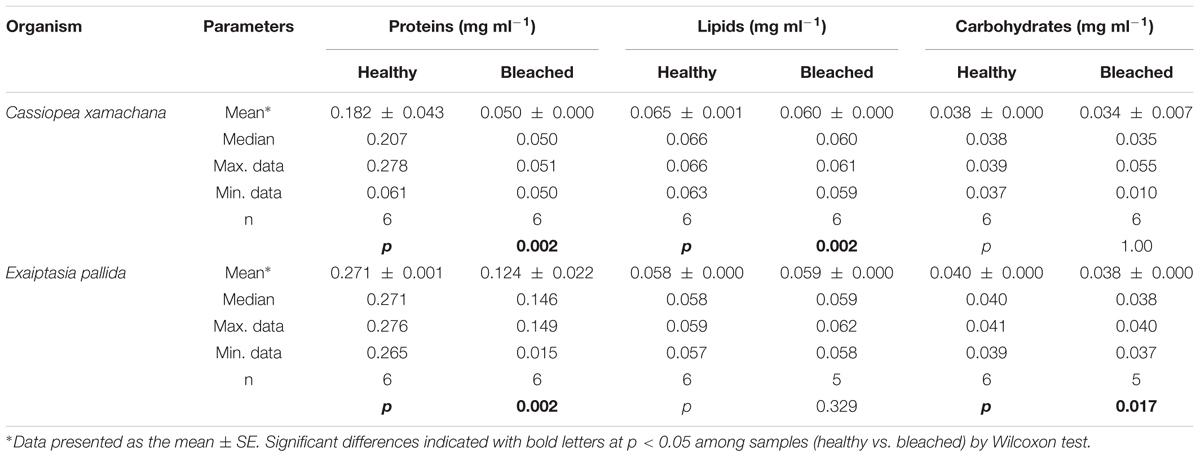
TABLE 5. Total protein, lipid and carbohydrates in mucus samples collected from healthy and bleached C. xamachana and E. pallida.
Discussion
The central role of the surface mucus layer in corals and reef cnidarians is the protection of live tissue, not only as a physical barrier but also as a chemical one (Brown and Bythell, 2005; Ritchie, 2006; Wahl et al., 2012). In addition, the SML has antimicrobial compounds and other molecules that can fight potential pathogenic microorganisms (Abbas et al., 2007; Mydlarz et al., 2010). We evaluated the antimicrobial capability of the SML per se, by irradiating collected mucus with UV light. We found that this layer has antimicrobial activity against tester strains S. marcescens and Aurantimonas sp. However, as we hypothesized, results differed among the mucus collected from the different animals we considered. In healthy animals, only anemones did not show an inhibitory effect against S. marcescens, associated exclusively with the surface mucus layer. On the other hand, the mucus layer of healthy corals and medusas was not as effective to inhibit the growth of Aurantimonas sp. as that of anemones. Our results agree with the concept of the SML as a chemical barrier with antimicrobial capabilities, containing molecules that contend potential pathogens, related to the phylogenetic position of these animals and perhaps to their particular life history.
A single publication on the antibacterial properties and the innate immune response of corals has analyzed these properties in the surface mucus layer (Ritchie, 2006). In this work, the author evaluated UV irradiated mucus collected from the reef coral Acropora palmata and found that it has antimicrobial activity, suggesting its role as a biochemical defense. In addition, some of the bacteria isolated and cultured from this same layer showed antimicrobial activity (Ritchie, 2006). In that same work, UV irradiated mucus collected from colonies that had experienced increased temperature also was evaluated and found that it had lost its antimicrobial capability. We found that, although the SML showed diminished inhibitory activity in diseased colonies, the antimicrobial capability of mucus-associated bacteria rather improved against S. marcescens. The antimicrobial capabilities of the mucus layer that we assessed in healthy P. strigosa colonies over two tester strains, demonstrated a differential response: the inhibitory activity against S. marcescens was associated with the bacteria-free mucus, while the inhibitory activity against Aurantimonas sp. was mostly associated with the bacteria cultured from the SML. These results suggest that the antimicrobial activity of the SML has some kind of species-specific effect, also indicating that S. marcescens may be a common potential pathogen.
Previous work has indicated that corals affected by a disease have reduced defensive capabilities (Palmer et al., 2010; Pinzón et al., 2014a). Consistent with these reports, diseased coral colonies of P. strigosa showed a diminished inhibitory effect against Aurantimonas sp. in mucus over the transition zone, for all mucus treatments. However, this capacity was not different in healthy colonies when compared to the mucus over healthy-looking tissue of diseased colonies. It is interesting that against S. marcescens, the SML antibacterial activity in diseased colonies was reduced, but the bacteria associated with this layer showed positive, inhibitory activity in mucus from diseased tissue, suggesting that SML associated bacteria may contribute significantly to protect diseased corals. Black band disease is characterized as a dark band, formed by a microbial consortium dominated by cyanobacteria, which migrates across living tissues degrading and killing entire colonies over a period of several months (Garret and Ducklow, 1975; Rützler and Santavy, 1983). Various studies have reported that the microbial community in different species of corals affected by BBD, increases in diversity, including some specific genera like Desulfobacteraceae and toxin-producing cyanobacteria (Frias-Lopez et al., 2004; Sekar et al., 2006). In P. strigosa colonies affected by BBD, the mucus layer showed the presence of hydroxyproline, suggesting that the immune response in these colonies is active and producing collagen to encapsulate potential pathogens (Mydlarz et al., 2010; Palmer and Taylor-Knowles, 2012; Ocampo and Cadavid, 2015). Although no melanin was detected in mucus from any of the animals studied, phenoloxidase activity behaved similarly to hydroxyproline in coral diseased colonies. These results are in agreement with studies on Acropora millepora affected with white syndrome that detected more phenoloxidase activity near the lesion (Palmer et al., 2011). In addition, the healthy-looking tissue of diseased colonies of Orbicella faveolata affected by yellow band disease was shown to have an active immune response (Weil et al., 2009; Morgan et al., 2015). Concurring with previous reports (Fleming, 1922; Ritchie, 2006; Abbas et al., 2007), we were able to detect an innate immune activity in the mucus layer, suggesting that corals expel molecules with such activity from their tissues.
It seems relevant to comment that there is a lack of reports on diseases in Exaiptasia anemones or Cassiopea medusas in the field. Our results suggest that main antibacterial capacity of these organisms seems to be located in different components of the superficial mucus layer, being specific to the tested bacteria: against S. marcescens the mucus of anemones seemed to depend on the associated bacteria, while inhibitory effects in medusas associated with the bacteria-free mucus layer. Against Aurantimonas sp., the anemones had the same inhibitory effect independent of the component of the mucus complex, which diminished in bleached animals. But in medusas, only the bacteria-free mucus layer demonstrated inhibition of the growth of Aurantimonas sp., which was also lost in bleached animals. Apparently, E. pallida does not have a characteristic core bacterial community (Brown et al., 2017; Herrera et al., 2017). This community varies according to the environmental conditions and does so rapidly (Herrera et al., 2017). This characteristic could explain the high resilience of this anemone to stress that we corroborated in bleached animals, as they did not show a diminished capacity to inhibit the growth of S. marcescens and, although lower, still showed an appreciable inhibitory effect against Aurantimonas sp. S. marcescens is a ubiquitous, opportunistic terrestrial pathogen, often infecting humans. In the ocean, it could be linked to municipal discharges. This bacterium has been found to cause white pox disease in the coral A. palmata (Patterson et al., 2002). Interestingly, the response in E. pallida could implicate a previous contact with this pathogen, contrasting with P. strigosa and C. xamachana, which were less successful in inhibiting its growth. As for Aurantimonas sp., it may be a more common inhabitant of the sea, which would explain why the mucus complex of all three cnidarians showed inhibitory activity against it.
Increased temperatures commonly cause bleaching in symbiotic reef cnidarians (Brown, 1997; Hoegh-Guldberg, 1999). After bleaching, there is a diminished translocation of carbon (Muscatine et al., 1991; McGill and Pomeroy, 2008) and an essential loss of energy for their health (Iglesias-Prieto et al., 1992; Grottoli et al., 2006; reviewed in Mydlarz et al., 2010). As a consequence, bleached corals have been found to be more susceptible to disease (Miller et al., 2006; Bruno et al., 2007). Our results showed that the mucus extracted from bleached anemones did not have a reduced effect against S. marcescens but the inhibitory effect against Aurantimonas sp. was reduced. Although bleaching of the medusa reduced the inhibitory effect of the SML, interestingly the mucus complex exhibited an increased inhibition over the growth of Aurantimonas sp.
On the other hand, bleaching did not change the levels of hydroxyproline or phenoloxidase in the SML of anemones or medusas, suggesting that the loss of symbionts is not enough to stimulate immunological activities that may reach the SML. However, the loss of symbionts leads to a loss of translocated carbon (Muscatine et al., 1991; McGill and Pomeroy, 2008). In anemones, this reflected directly in the levels of carbohydrates of the surface mucus layer. But in medusas, the loss of potentially translocated carbon was detected in lipid levels. This difference may relate to the time these animals had been without symbionts. E. pallida were bleached by cold shocks and maintained for 2–3 weeks before the experimental measures were taken, while C. xamachana were bleached at low light over a 2 months period with daily feeding, but their lipid reserves may have been significantly reduced. In any case, the loss of symbionts probably changes the composition of the surface mucus layer; but this change did not affect the antibacterial properties of this layer in anemones but did so in medusas. Although we did not find antibacterial and immunological responses to be associated with the composition of the mucus layer in any of the animals considered, it is interesting that the values for total proteins, carbohydrates, and lipids among healthy animals were similar, implying a balanced C-budget.
Disease and bleaching in tropical symbiotic cnidarians have increased severely in the past years due to the deterioration of the reef environment. However, the defensive responses these organisms possess may help them in a species-specific way. In coral colonies of P. strigosa affected by BBD, our results indicate that even though the superficial mucus layer and the associated bacterial community have antibacterial capabilities, and some innate immune response was measured, their response may not be strong enough to fight this disease successfully. Bleached animals did not show significant changes in their immune response. The techniques that were used to bleach anemones and medusas in this work did not involve high temperature, which may have resulted in less substantial changes in the associated bacterial community. Diminished carbohydrates or lipids in the mucus of bleached animals and the presence of immune activity, may have reduced the antimicrobial capability of the mucus layer, except for the response against Aurantimonas sp. in medusas, which was improved. The activity may have been due to a specific bacterium with antimicrobial capabilities that was isolated from bleached medusas. We are currently working on its identification.
Author Contributions
JR-O and PT designed the experiments. JR-O conducted the experiments, undertook the statistical analyses, and prepared the figures and tables. PT wrote the manuscript. All authors contributed to the final version of the manuscript.
Funding
This work was supported by DGAPA-PAPIIT-UNAM Grant IN204318 to PT.
Conflict of Interest Statement
The authors declare that the research was conducted in the absence of any commercial or financial relationships that could be construed as a potential conflict of interest.
Acknowledgments
JR-O wishes to recognize a scholarship for her master studies by CONACyT México and UNAM-DGAPA-PAPIIT through grant IN204318. Medusas were made kindly available by Xcaret Park, México. We thank Yislem Beltrán Díaz for fieldwork. We are grateful to two reviewers who helped to improve this manuscript. We also want to thank the Academic Writing Workshop CEP-UNAM for suggestions to the manuscript. Coral mucus was collected with a permit from INAPESCA number PPF/DGOPA-110/16.
References
Abbas, A. K., Lichtman, A. H., and Pillai, S. (2007). Cellular and Molecular Immunology, 6th Edn. Hoboken, NJ: Elsevier.
Ainsworth, T. D., Fine, M., and Blackall, L. L. (2006). Fluorescence in situ hybridization and spectral imaging of coral-associated bacterial communities. Appl. Environ. Microbiol. 72, 30126–33020. doi: 10.1128/AEM.72.4.3016-3020.2006
Augustin, R., and Bosch, T. C. (2010). “Cnidarian immunity: a tale of two barriers,” in Invertebrate Immunity. Advances in Experimental Medicine and Biology, Vol. 708, ed. K. Söderhäll (Boston, MA: Springer), 1–16.
Bosch, T. C., and McFall-Ngai, M. J. (2011). Metaorganisms as the new frontier. Zoology 114, 185–190. doi: 10.1016/j.zool.2011.04.001
Bourne, D., Iida, Y., Uthicke, S., and Smith-Keune, C. (2007). Changes in coral-associated microbial communities during a bleaching event. ISME J. 2, 350–363. doi: 10.1038/ismej.2007.112
Brown, B. (1997). Coral bleaching: causes and consequences. Coral Reefs 16(Suppl. 1), S129–S138. doi: 10.1007/s003380050249
Brown, B. E., and Bythell, J. C. (2005). Perspectives on mucus secretion in reef corals. Mar. Ecol. Prog. Ser. 296, 291–309. doi: 10.3354/meps296291
Brown, T., Otero, C., Grajales, A., Rodríguez, E., and Rodríguez-Lanetty, M. (2017). Worldwide exploration of the microbiome harbored by the cnidarian model, Exaiptasia pallida (Agassiz in Verrill, 1864) indicates a lack of bacterial association specificity at a lower taxonomic rank. PeerJ 16:e3235. doi: 10.7717/peerj.3235
Bruno, J. F., Selig, E. R., Casey, K. S., Page, C. A., Willis, B. L., Harvell, C. D., et al. (2007). Thermal stress and coral cover as drivers of coral disease outbreaks. PLoS Biol. 5:e124. doi: 10.1371/journal.pbio.0050124
Camargo Guarnieri, M., Albuquerque Modesto, J. C., Pérez, C. D., Fontes Ottaiano, T., daSilva Ferreira, R., Pereira Batista, F., et al. (2018). Zoanthid mucus as new source of useful biologically active proteins. Toxicon 143, 96–107. doi: 10.1016/j.toxicon.2018.01.012
Castillo, I., Lodeiros, C., Nunez, M., and Campos, I. (2001). In vitro evaluation of antibacterial substances produced by bacteria isolated from different marine organisms. Rev. Biol. Trop. 49, 1213–1222.
Cole, M. A., Dewan, P., and Ganz, T. (1999). Innate antimicrobial activity of nasal secretions. Infect. Immun. 67, 3267–3275.
Denner, E. B. M., Smith, G. W., Busse, H.-J., Schumann, P., Narzt, T., Polson, S. W., et al. (2003). Aurantimonas corallicida gen. nov., sp. nov. the causative agent of white plague type II on Caribbean scleractinian corals. Int. J. Syst. Evol. Microbiol. 53, 1115–1122. doi: 10.1099/ijs.0.02359-0
Dubois, M., Gilles, K., Hamilton, J. K., Rebers, P. A., and Smith, F. (1951). A colorimetric method for the determination of sugars. Nature 4265:167. doi: 10.1038/168167a0
Estes, A. M., Kempf, S. C., and Henry, R. P. (2003). Localization and quantification of carbonic anhydrase activity in the symbiotic Scyphozoan Cassiopea xamachana. Biol. Bull. 204, 278–289. doi: 10.2307/1543599
Fitt, W. K., Gates, R. D., Hoegh-Guldberg, O., Bythel, J. C., Jatkar, A., Grottoli, A. G., et al. (2009). Response of two species of Indo-Pacific corals, Porites cylindrica and Stylophora pistillata, to short-term thermal stress: the host does matter in determining the tolerance of corals to bleaching. J. Exp. Mar. Biol. Ecol. 373, 102–110. doi: 10.1016/j.jembe.2009.03.011
Fitt, W. K., McFarland, F. K., Warner, M. E., and Chilcoat, G. C. (2000). Seasonal patterns of tissue biomass and densities of symbiotic dinoflagellates in reef corals and relation to coral bleaching. Limnol. Oceanogr. 45, 677–685. doi: 10.4319/lo.2000.45.3.0677
Fleming, A. (1922). On a remarkable bacteriolytic element found in tissues and secretions. Proc. R. Soc. Lond. 93, 306–318. doi: 10.1098/rspb.1922.0023
Franzenburg, S., Walter, J., Künzel, S., Wang, J., Baines, J. F., Bosch, T. C., et al. (2013). Distinct antimicrobial peptide expression determines host species-specific bacterial associations. Proc. Natl. Acad. Sci. U.S.A. 110, E3730–E3738. doi: 10.1073/pnas.1304960110
Fraune, S., and Bosch, T. C. (2007). Long-term maintenance of species-specific bacterial microbiota in the basal metazoan Hydra. Proc. Natl. Acad. Sci. U.S.A. 104, 13146–13151. doi: 10.1073/pnas.0703375104
Frias-Lopez, J., Klaus, J. S., Bonheyo, G. T., and Fouke, B. W. (2004). Bacterial community associated with black band disease in corals. Appl. Environ. Microbiol. 70, 5955–5962. doi: 10.1128/AEM.70.10.5955-5962.2004
Garret, P., and Ducklow, H. W. (1975). Coral disease in Bermuda. Nature 253, 349–350. doi: 10.1038/253349a0
Gil-Turnes, M. S., and Fenical, W. (1992). Embryos of Homarus americanus are protected by epibiotic bacteria. Biol. Bull. 182, 105–108. doi: 10.2307/1542184
Glynn, P. W., and D’Croz, L. (1990). Experimental evidence for high temperature stress as the cause of El Nino-coincident coral mortality. Coral Reefs 8, 181–191. doi: 10.1007/BF00265009
Green, D. H., Edmunds, P. J., and Carpenter, R. C. (2008). Increasing relative abundance of Porites astreoides on Caribbean reefs mediated by an overall decline in coral cover. Mar. Ecol. Prog. Ser. 359, 1–10. doi: 10.3354/meps07454
Greffen, Y., and Rosenberg, E. (2005). Stress-induced rapid reléase of antibacterials by scleractinian corals. Mar. Biol. 146, 931–935. doi: 10.1007/s00227-004-1505-5
Grottoli, A. G., Rodriguez, L. J., and Palardy, J. E. (2006). Heterotrophic plasticity and resilience in bleached corals. Nature 440, 1186–1189. doi: 10.1038/nature04565
Herrera, M., Ziegler, M., Voolstra, C. R., and Aranda, M. (2017). Laboratory cultured strains of the sea anemone Exaiptasia reveal distinct bacterial communities. Front. Mar. Sci. 4:115. doi: 10.3389/fmars.2017.00115
Hoegh-Guldberg, O. (1999). Climate change, coral bleaching and the future of the world’s coral reefs. Mar. Freshw. Res. 50, 839–866. doi: 10.1071/MF99078
Hofman, K., Hall, B., Cleaver, H., and Marshall, S. (2011). High-throughput quantification of hydroxyproline for determination of collagen. Anal. Biochem. 417, 289–291. doi: 10.1016/j.ab.2011.06.019
Iglesias-Prieto, R., Matta, J. L., Robins, W. A., and Trench, R. K. (1992). The photosynthetic response to elevated temperature in the symbiotic dinoflagellate Symbiodinium microadriaticum in culture. Proc. Natl. Acad. Sci. U.S.A. 89, 10302–10305. doi: 10.1073/pnas.89.21.10302
Johnston, I. S., and Rohwer, F. (2007). Microbial landscapes on the outer tissue surfaces of the reef-building coral Porites compressa. Coral Reefs 26, 375–383. doi: 10.1007/s00338-007-0208-z
Jung, S. (2009). Hydramacin-1: structure and antibacterial activity of a protein from the basal metazoan Hydra. J. Biol. Chem. 284, 1896–1905. doi: 10.1074/jbc.M804713200
Koren, O., and Rosenberg, E. (2006). Bacteria associated with mucus and tissues of the coral Oculina patagonica in summer and winter. Appl. Environ. Microbiol. 72, 5254–5259. doi: 10.1128/AEM.00554-06
Krediet, C. J., Ritchie, K. B., Cohen, M., Lipp, E. K., Sutherland, K. P., and Teplitski, M. (2009). Utilization of mucus from the coral Acropora palmata by the pathogen Serratia marcescens and by environmental and coral commensal bacteria. Appl. Environ. Microbiol. 75, 3851–3858. doi: 10.1128/AEM.00457-09
Longford, S. R., Tujula, N. A., Crocetti, G. R., Holmes, A. J., Holmström, C., Kjelleberg, S., et al. (2007). Comparisons of diversity of bacterial communities associated with three sessile marine eukaryotes. Aquat. Microb. Ecol. 48, 217–229. doi: 10.3354/ame048217
McGill, C. J., and Pomeroy, C. M. (2008). Effects of bleaching and nutrient supplementation on wet weight in the jellyfish Cassiopea xamachana (Bigelow) (Cnidaria: Scyphozoa). Mar. Freshw. Behav. Physiol. 41, 179–189. doi: 10.1080/10236240802369899
Miller, J., Waara, M., Muller, E., and Rogers, C. (2006). Coral bleaching and disease combine to cause extensive mortality on reefs in US Virgin Islands. Coral Reefs 25:418. doi: 10.1007/s00338-006-0125-6
Mishra, S. K., Suh, W., Farooq, Q., Moon, M., Shrivastav, A., Park, M. S., et al. (2014). Rapid quantification of microalgal lipids in aqueous medium by a simple colorimetric method. Bioresour. Technol. 155, 330–333. doi: 10.1016/j.biortech.2013.12.077
Morgan, M., Goodner, K., Ross, J., Poole, A. Z., Stepp, E., Stuart, C. H., et al. (2015). Development and application of molecular biomarkers for characterizing Caribbean Yellow Band Disease in Orbicella faveolata. PeerJ 3:E1371. doi: 10.7717/peerj.1371
Muscatine, L., Grossman, D., and Doino, J. (1991). Release of symbiotic algae by tropical sea anemones and corals after cold shock. Mar. Biol. Prog. Ser. 77, 233–243. doi: 10.3354/meps077233
Mydlarz, L. D., Holthouse, S. F., Peters, E. C., and Harvell, C. D. (2008). Cellular responses in sea fan corals: granular amoebocytes react to pathogen and climate stressors. PLoS One 3:e1811. doi: 10.1371/journal.pone.0001811
Mydlarz, L. D., McGinty, E. S., and Harvell, C. D. (2010). What are the physiological and immunological responses of coral to climate warming and disease? J. Exp. Biol. 213, 934–945. doi: 10.1242/jeb.037580
Mydlarz, L. D., and Palmer, C. V. (2011). The presence of multiple phenoloxidases in Caribbean reef-building corals. Comp. Biochem. Physiol. A 159, 372–378. doi: 10.1016/j.cbpa.2011.03.029
Ocampo, I. V., and Cadavid, L. F. (2015). Mechanisms of immune responses in cnidarians. Acta Biol. Colomb. 20, 5–11. doi: 10.154446/abc.v20n2.46728
Ovchinnikova, T. V., Balandin, S. V., Aleshina, G. M., Tagaev, A. A., Leonova, Y. F., Krasnodembsky, E. D., et al. (2006). Aurelin, a novel antimicrobial peptide from jellyfish Aurelia aurita with structural features of defensins and channel-blocking toxins. Biochem. Biophys. Res. Commun. 348, 514–523. doi: 10.1016/j.bbrc.2006.07.078
Palmer, C. V., Bythel, J. C., and Willis, B. L. (2010). Levels of immunity parameters underpin bleaching and disease susceptibility of reef corals. FASEB J 24, 1935–1946. doi: 10.1096/fj.09-152447
Palmer, C. V., and Taylor-Knowles, N. (2012). Towards an integrated network of coral immune mechanisms. Proc. R. Soc. B 279, 4106–4114. doi: 10.1098/rspb.2012.1477
Palmer, C. V., Traylor-Knowles, N. G., Willis, B. L., and Bythell, J. C. (2011). Coral use similar immune cells and wound-healing processes as those of higher organisms. PLoS One 6:e23992. doi: 10.1371/journal.pone.0023992
Patterson, K. L., Porter, J. W., Ritchie, K. B., Polson, S. W., Mueller, E., Peters, E. C., et al. (2002). The etiology of white pox, a lethal disease of the Caribbean elkhorn coral, Acropora palmata. Proc. Natl. Acad. Sci. U.S.A. 99, 8725–8730. doi: 10.1073/pnas.092260099
Petes, L. E., Harvell, C. D., Peters, E. C., Webb, M. A. H., and Mullen, K. M. (2003). Pathogens compromise reproduction and induce melanization in Caribbean sea fans. Mar. Ecol. Prog. Ser. 264, 167–171. doi: 10.3354/meps264167
Pinzón, C., Beach-Letendre, L., Weil, E., and Mydlarz, L. D. (2014a). Relationship between phylogeny and immunity suggest older Caribbean coral lineages are more resistant to disease. PLoS One 9:e104787. doi: 10.1371/journal.pone.0104787
Pinzón, C., Dornberger, J. H., Beach-Letendre, L., Weil, E., and Mydlarz, L. D. (2014b). The link between immunity and life history traits in scleractinian corals. PeerJ 2:e628. doi: 10.7717/peerj.628
Richardson, L., Goldberg, W. M., Kuta, K. G., Aronson, R. B., Smith, G. W., and Ritchie, K. B. (1998). Florida’s mystery coral-killer identified. Nature 392, 557–558. doi: 10.1038/33302
Ritchie, K. B. (2006). Regulation of microbial populations by coral surface mucus and mucus-associated bacteria. Mar. Ecol. Prog. Ser. 322, 1–14. doi: 10.3354/meps322001
Rohwer, F., Seguritan, V., Azam, F., and Knowlton, N. (2002). Diversity and distribution of coral-associated bacteria. Mar. Ecol. Prog. Ser. 243, 1–10. doi: 10.3354/meps243001
Rützler, K., and Santavy, D. (1983). The black band disease of Atlantic reef corals. I. Description of a cyanophyte pathogen. Mar. Ecol. 4, 301–319. doi: 10.1111/j.1439-0485.1983.tb00116.x
Rypien, K. L., Ward, J. R., and Azam, F. (2010). Antagonistic interactions among coral-associated bacteria. Environ. Microbiol. 12, 28–39. doi: 10.1111/j.1462-2920.2009.02027.x
Sekar, R., Mills, D. K., Remily, E. R., Voss, J. D., and Richardson, L. L. (2006). Microbial communities in the surface mucopolysaccharide layer, and the black band microbial mat of Black Band-Diseased Siderastrea siderea. Appl. Environ. Microbiol. 72, 5963–5973. doi: 10.1128/AEM.00843-06
Sharp, K. H., Ritchie, K. B., Schupp, P. J., Ritson-Williams, R., and Paul, V. J. (2010). Bacterial acquisition in juveniles of several broadcast spawning coral species. PLoS One 5:e10898. doi: 10.1371/journal.pone.0010898
Shöder, K., and Bosch, T. C. (2016). The origin of mucosal immunity: lessons from the holobiont Hydra. mBio 7:e01184-16. doi: 10.1128/mBio.01184-16
Thompson, J. R., Rivera, H. E., Closek, C. L., and Medina, M. (2015). Microbes in the coral holobiont: partners through evolution, development and ecological interactions. Front. Cell. Infect. Microbiol. 4:176. doi: 10.3389/fcimb.2014.00176
Vega Thurber, R. V., Willner-Hall, A., Rodríguez-Mueller, B., Desnues, C., Edwards, R. A., Angly, F., et al. (2009). Metagenomic analysis of stressed coral holobionts. Environ. Microbiol. 11, 2148–2163. doi: 10.1111/j.1462-2920.2009.01935.x
Vidal-Dupiol, J., Ladrière, O., Destoumieux-Garzón, D., Sautiere, P. E., Meistertzheim, A. L., Tambutté, E., et al. (2011). Innate immune responses of a scleractinian coral to viriosis. J. Biol. Chem. 286, 22688–22698. doi: 10.1074/jbc.M110.216358
Wahl, M., Goecke, F., Labes, A., Dobrestov, S., and Weinberger, F. (2012). The second skin: ecological role of epibiotic biofilms on marine organisms. Front. Microbiol. 3:292. doi: 10.3389/fmicb.2012.00292
Keywords: antimicrobial capability, coral mucus, immunological defense, innate immunity, bacteria-free mucus, coral immunity, Serratia marcescens, Aurantimonas
Citation: Rivera-Ortega J and Thomé PE (2018) Contrasting Antibacterial Capabilities of the Surface Mucus Layer From Three Symbiotic Cnidarians. Front. Mar. Sci. 5:392. doi: 10.3389/fmars.2018.00392
Received: 01 June 2018; Accepted: 09 October 2018;
Published: 30 October 2018.
Edited by:
Michael Sweet, University of Derby, United KingdomReviewed by:
Aldo Cróquer, Simón Bolívar University, VenezuelaCharles Alan Jacoby, St. Johns River Water Management District, United States
Copyright © 2018 Rivera-Ortega and Thomé. This is an open-access article distributed under the terms of the Creative Commons Attribution License (CC BY). The use, distribution or reproduction in other forums is permitted, provided the original author(s) and the copyright owner(s) are credited and that the original publication in this journal is cited, in accordance with accepted academic practice. No use, distribution or reproduction is permitted which does not comply with these terms.
*Correspondence: Patricia E. Thomé, dGhvbWVAY21hcmwudW5hbS5teA==