- 1Department of Maritime Systems, Interdisciplinary Faculty, University of Rostock, Rostock, Germany
- 2Landscape Ecology, Faculty of Agricultural and Environmental Sciences, University of Rostock, Rostock, Germany
- 3Soil Physics, Faculty of Agricultural and Environmental Sciences, University of Rostock, Rostock, Germany
- 4Biological Oceanography, Leibniz Institute for Baltic Sea Research Warnemünde, Rostock, Germany
- 5Geochemistry and Isotope Biogeochemistry, Leibniz Institute for Baltic Sea Research Warnemünde, Rostock, Germany
- 6Fluid Mechanics, Faculty of Mechanical Engineering and Marine Technology, University of Rostock, Rostock, Germany
- 7Department of Physical Oceanography and Instrumentation, Leibniz Institute for Baltic Sea Research Warnemünde, Rostock, Germany
- 8Marine Biology, Faculty of Mathematics and Natural Sciences, University of Rostock, Rostock, Germany
- 9Phycology and Applied Ecology, Faculty of Mathematics and Natural Sciences, University of Rostock, Rostock, Germany
- 10Marine Chemistry, Leibniz Institute for Baltic Sea Research Warnemünde, Rostock, Germany
- 11Soil Science, Faculty of Agricultural and Environmental Sciences, University of Rostock, Rostock, Germany
- 12Hydrogeology, Carl von Ossietzky University of Oldenburg, Oldenburg, Germany
- 13Department of Applied Geology, University of Göttingen, Göttingen, Germany
- 14Ecohydrology Research Group, Water Institute and Department of Earth & Environmental Sciences, University of Waterloo, Waterloo, ON, Canada
- 15Aquatic Ecology, Faculty of Mathematics and Natural Sciences, University of Rostock, Rostock, Germany
Coastal zones connect terrestrial and marine ecosystems forming a unique environment that is under increasing anthropogenic pressure. Rising sea levels, sinking coasts, and changing precipitation patterns modify hydrodynamic gradients and may enhance sea–land exchange processes in both tidal and non-tidal systems. Furthermore, the removal of flood protection structures as restoration measure contributes locally to the changing coastlines. A detailed understanding of the ecosystem functioning of coastal zones and the interactions between connected terrestrial and marine ecosystems is still lacking. Here, we propose an interdisciplinary approach to the investigation of interactions between land and sea at shallow coasts, and discuss the advantages and the first results provided by this approach as applied by the research training group Baltic TRANSCOAST. A low-lying fen peat site including the offshore shallow sea area on the southern Baltic Sea coast has been chosen as a model system to quantify hydrophysical, biogeochemical, sedimentological, and biological processes across the land–sea interface. Recently introduced rewetting measures might have enhanced submarine groundwater discharge (SGD) as indicated by distinct patterns of salinity gradients in the near shore sediments, making the coastal waters in front of the study site a mixing zone of fresh- and brackish water. High nutrient loadings, dissolved inorganic carbon (DIC), and dissolved organic matter (DOM) originating from the degraded peat may affect micro- and macro-phytobenthos, with the impact propagating to higher trophic levels. The terrestrial part of the study site is subject to periodic brackish water intrusion caused by occasional flooding, which has altered the hydraulic and biogeochemical properties of the prevailing peat soils. The stable salinity distribution in the main part of the peatland reveals the legacy of flooding events. Generally, elevated sulfate concentrations are assumed to influence greenhouse gas (GHG) emissions, mainly by inhibiting methane production, yet our investigations indicate complex interactions between the different biogeochemical element cycles (e.g., carbon and sulfur) caused by connected hydrological pathways. In conclusion, sea–land interactions are far reaching, occurring on either side of the interface, and can only be understood when both long-term and event-based patterns and different spatial scales are taken into account in interdisciplinary research that involves marine and terrestrial expertise.
Sea–Land Interactions in Shallow Coastal Areas Under Anthropogenic Pressure
Coastal areas are the preferred habitats of humans, and it is estimated that between half a billion and more than a billion people live in low-lying coastal regions around the world (Bollmann et al., 2010; Neumann et al., 2015). Global climate change threatens this space, while the increasing population in coastal areas puts the coasts under pressure due to construction activities (including settlements, harbors, wind farms, and coastal protection structures such as dykes and levees), agriculture, and tourism (Nicholls et al., 2007; Doney, 2010; Kummu et al., 2016). These anthropogenic activities modify the water exchange processes between land and sea.
Shallow coasts at low-lying areas are characterized by a relatively wide ecocline from land to sea. Long-term changes in sea-level as well as periodic (tides) or episodic (storm surges) events can, thus, affect large areas on both sides of the shoreline. Our understanding of the sea–land connection is focused on extreme scenarios in which the ocean is flooding the land with often dramatic consequences to the people living on the coast. Studies of sea–land interactions are often focused on estuaries where large quantities of fresh water and solutes are mixing with ocean water (e.g., Newton et al., 2014). The impact of rivers on the coastal ocean is substantial and includes excess nutrient delivery (eutrophication) (Bollmann et al., 2010), transport of essential trace compounds (metals, silica) (Martin and Whitfield, 1983; Du Laing et al., 2009), and may lead to drastic environmental changes along the salinity gradient (Kemp et al., 2005). The coastline itself is rarely considered as an exchange interface for energy, water, and substances, yet this interface is very important as it operates continuously and may have far-reaching effects on (micro-)biological and hydro-biogeochemical processes on either side of the coast (e.g., Rullkötter, 2009; Gätje and Reise, 2012).
Sea level rise is a common process observable around the world, mainly caused by ongoing climate change (Church et al., 2013). Recent studies show a rapid increase of sea level worldwide (Nerem et al., 2018), that is amplified at the southern Baltic Sea by an ongoing isostatic subsidence of the coast (Johansson et al., 2014). Taking into consideration the possible scenario of a combination of sinking coasts (Hünicke and Zorita, 2016), the rising sea levels (Grinsted et al., 2015), and future increase of winter precipitation (BACC II Author Team, 2015), we can expect that sea–land connectivity will increase in the Southern Baltic Sea region in future as the area of the coastal system increases (Nicholls et al., 2007). Understanding of the prevailing physical, biogeochemical, and biological drivers as well as of potential tipping points of such coasts in transition is crucial for our ability to sustainably balance natural conservation and anthropogenic use of the coasts. Therefore, the overarching aim of the Baltic TRANSCOAST research training group is to enhance the fundamental understanding of the interconnected physico-chemical, biogeochemical, and ecological processes along the ecocline of the shallow coast using a southern Baltic coastal site, the Hütelmoor, as a model. The aim of the present paper is to outline the hypotheses and research questions of the interdisciplinary approach of Baltic TRANSCOAST and present the first results generated by this approach as a roadmap to gain deeper insight into processes across lowland coastal transects that can be applied to investigations of similar shallow coastal systems worldwide.
The Baltic Sea as a Model to Study Exchange Processes at Shallow Coasts with Low-Lying Land Areas
The Baltic Sea is a young shelf sea in northern Europe (Figure 1) connected to the North Sea since the time of the Litorina transgression about 8,000 years ago. The coastline of the Baltic Sea is roughly 2,000 km long (STALU-MM, 2010). The German coastal areas comprise 13,900 km2 currently mostly protected by dykes (Bollmann et al., 2010). Along the shoreline of the Baltic Sea, cliffs alternate with low-lying areas, with the latter often including peatlands. The extent of low-lying areas increases from West to East along the Baltic Sea coast in northeastern Germany (Figure 1).
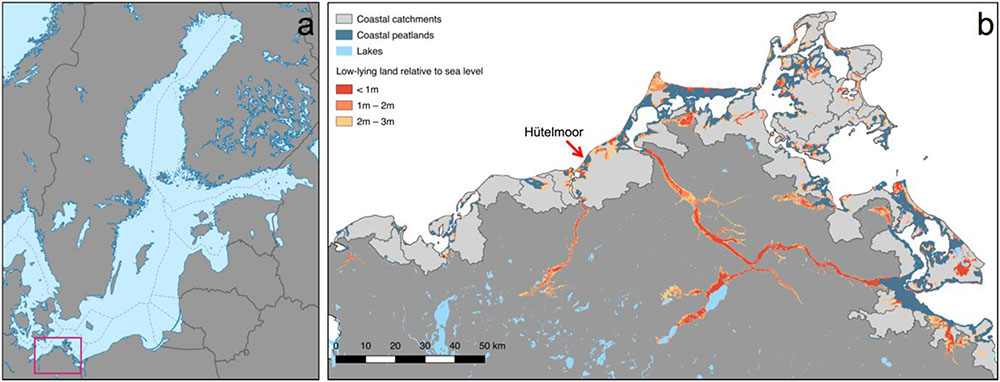
FIGURE 1. (a) Map of the Baltic Sea with the extent of the region to the right represented by a purple rectangle; (b) The Baltic Sea coast in the north-east of Germany with low-lying areas and coastal peatlands. Low-lying areas reach relatively far into the land and the wide river valleys are filled with peat. Coastal peatlands occur in the lowest-lying areas (<1 m). Note the coastal catchments (light gray) that are not connected to a river basin and discharge directly into the sea. Country borders were retrieved from www.gadm.org. Base data for the map in b and all other maps were provided by www.geoportal-mv.de.
At low-lying coastal areas, peatlands formed by the accumulation of organic material over millennia often constitute the interface between land and water bodies (Figure 1). They cover an area of approx. 40,000 ha in the northeastern German federal state of Mecklenburg-Western Pomerania and play a pivotal role in buffering the exchange of water and dissolved and particulate compounds. Fundamentally, two different processes contribute to formation of the two major types of coastal peatlands along the southern Baltic Sea coast, i.e., paludification (driven by very rare flooding when the water is hindered from leaving the flooded area) or episodic flooding (weekly to monthly) with flooded periods being generally shorter than non-flooded periods. Most coastal peatlands in Mecklenburg-Western Pomerania belong to the second, episodically flooded type. The sea–land transition zone is characterized by brackish water input into the terrestrial part of the coast through underground seawater intrusion or flooding. Submarine groundwater discharge (SGD) marks transport processes into the opposite direction delivering freshwater, dissolved organic matter (DOM), and nutrients to the sea, independent from riverine export and other point sources (e.g., Andersen et al., 2007; Knee and Paytan, 2011; Donis et al., 2017). SGD also encompasses recirculating seawater and mixtures of seawater with fresh waters (e.g., Böttcher et al., 2018). The direction of exchange across the coastline depends on the prevailing pressure gradients and their physically driven dynamics.
The natural dynamics at the coastline have been altered since centuries by flood control measures such as dykes and flood walls. The exchange of water across the sea–land interface is hampered by these structures, especially at low-lying coastal segments dominated by peatlands, which would be regularly flooded under natural conditions. The establishment of coastal protection measures allowed the artificial drainage of coastal peatlands for agricultural purposes. The resulting lowering of the groundwater tables modifies the prevailing physical and chemical gradients and hinders the possible land–sea connection. The necessity of coastal protection measures is controversial and much-debated. The need for protection of people and their possessions is indisputable. However, higher levels of protection and reduced exchange between land and sea increase the vulnerability of the coast and the potential for catastrophic events. Under sea-level rise, protection of low lying areas at shallow coasts may be an effective way for ensuring wealth and health of local residents, since they could be used as buffer zones for flooding events. Some of the coastal peatlands have been or will soon be rewetted by removing the dykes. The resulting exchange of water and compounds transported by water across the coastal interface has been perceived as a natural process worthy to be conserved or re-established. Likewise, the rewetting and restoration of wet- and peatlands have become a societal priority because of the enormous ecological services restored peatlands may provide like carbon sequestration, and water and nutrient buffering (Vasander et al., 2003).
Future weather and climate scenarios obtained from downscaling of global climate models draw a differentiated picture for the southern Baltic Sea region. While dryer summers with more frequent and occasional extreme storm events are to be expected, the winters may become warmer and wetter in terms of total precipitation (BACC II Author Team, 2015). The winter half-year (i.e., November–April) is the dominant period of discharge generation in the region since in summer evapotranspiration typically causes a negative water balance. With the expected addition of precipitation in winter, more pronounced discharge rates and steeper hydraulic gradients are expected. Considering the many catchments located on the coast that do not drain through rivers (Figure 1), it is likely that diffusive pathways from land to sea will become more important under future winter weather situations.
Elevated groundwater levels and associated steeper gradients toward the sea may provoke SGD (e.g., Church, 1996; Abarca et al., 2013). SGD is defined as ‘any and all flow of water on continental margins from the seabed to the coastal ocean’ (Burnett et al., 2003), originating, for instance, from fresh groundwater of modern terrestrial origin and/or recirculated seawater with the latter providing the main component of SGD in many places (e.g., Church, 1996; Burnett et al., 2003; Böttcher et al., 2018). Besides the hydro-geochemical measurements of dissolved substances, the patterns of Ra and other isotopes in surface waters are particularly helpful for identification and quantification of the benthic-pelagic coupling and source water contributions, respectively (e.g., Moore et al., 2011; Böttcher et al., 2014). Specifically, the pelagic presence of the short-lived 224Ra isotope, which is derived from thorium decay in the sediment, indicates a recent contribution of pore waters to the water column.
Submarine groundwater discharge as a pathway for the exchange of water and associated substances has been observed along the German coastline in tidal areas like the North Sea (e.g., Moore et al., 2011; Jeandel, 2016; Reckhardt et al., 2017) and non-tidal systems like the Baltic Sea (Piekarek-Jankowska, 1996; Massel, 2001; Peltonen, 2002; Kotwicki et al., 2014; Donis et al., 2017; Böttcher et al., 2018). Biological consequences of SGD have likewise been documented (e.g., Liu et al., 2017). Most significantly, the contribution of SGD to total input loads from the land into the sea has been considered as more important than that of riverine freshwater (Kwon et al., 2014). It remains challenging to prove the occurrence of SGD at low-lying coastal segments with small hydraulic gradients. In low-gradient systems, the geological substrate and the heterogeneity of hydraulic properties of individual sedimentary layers are crucial for the establishment of local groundwater fluxes.
The hydraulic properties of the marine sediments impact the near-ground circulation and thus solute dispersion in the shallow sea water. Furthermore, the sedimentary structures, such as ripple fields, have a direct influence on the turbulence dynamics and thereby on velocity and solute concentration profiles (Smyth et al., 2002). The hydraulic properties of marine sediments are considered highly changeable because of bioturbation. The connection between groundwater exfiltration, pore water transport, and near-ground circulation of solute spreading at the sediment–water interface remains as yet poorly described. Wave action and hydrodynamic processes at the water-sediment interface cause pore water fluxes in and out of the sediment (Massel, 2001), bringing oxygen as well as organic material into deeper sediment layers, thereby modifying redox gradients and, consequently, affecting biogeochemical processes like denitrification and/or iron, manganese and sulfate reduction (Huettel et al., 1998, 2003; de Beer et al., 2005; Gao et al., 2012).
Coastal marine sediments are often characterized by coarser grains (sand and gravel), and are commonly less sorted than sediments in deeper waters (Forster et al., 2003). Sediments are often resuspended and transported along the shore; consequently, they are permeable and the upper sediment layers in coastal zones are physically unstable and very mobile habitats. Benthic-pelagic coupling in shallow water is close, and coupling becomes stronger with increasingly shallow waters. The primary production rates are typically high and occur predominantly in the pelagic zone (Schiewer and Schubert, 2004). However, benthic primary production has rarely been considered in these systems despite the micro-phytobenthos and benthic macrophytes being responsible for most of the primary production in shallow lagoons. It has been shown that seagrass and macroalgae production can exceed 100 g C m-2 a-1 (Gocke et al., 2012). Additionally, benthic diatoms may contribute up to 60 g C m-2 a-1 to primary production in the temperate climate of the northern hemisphere (Cahoon, 1999). For the southern Baltic Sea, even higher values have been measured, ranging from 75 to >100 g C m-2 a-1 (Meyercordt and Meyer-Reil, 1999). In the case of the southern Baltic Sea coast with its abundant coastal peatlands, the fluxes across the sediment water interface might be modified hydrodynamically by the presence of outcropping peat layers in the water, which may also serve as a source of carbon and nutrients to the shallow sea. These outcrops are the result of the receding shoreline over the past several thousand years because of sea-level rise (Harff et al., 2017).
Baltic Transcoast Approach
The coastline appears to divide terrestrial from marine ecosystems, yet in reality it forms a unique sea–land transition zone due to the exchange processes between environmental compartments create a unique sea–land transition zone. Baltic TRANSCOAST aims to quantify the extent to which the sea influences terrestrial processes and assess how the shallow sea is affected by processes operating on land, thereby unraveling the system function of shallow coasts at low-lying land areas (Figure 2). We suggest a systematic and interdisciplinary research approach that considers the coast as a continuum of physical and biogeochemical processes that on the one hand influence marine and terrestrial biota, and on the other hand are impacted by this biota.
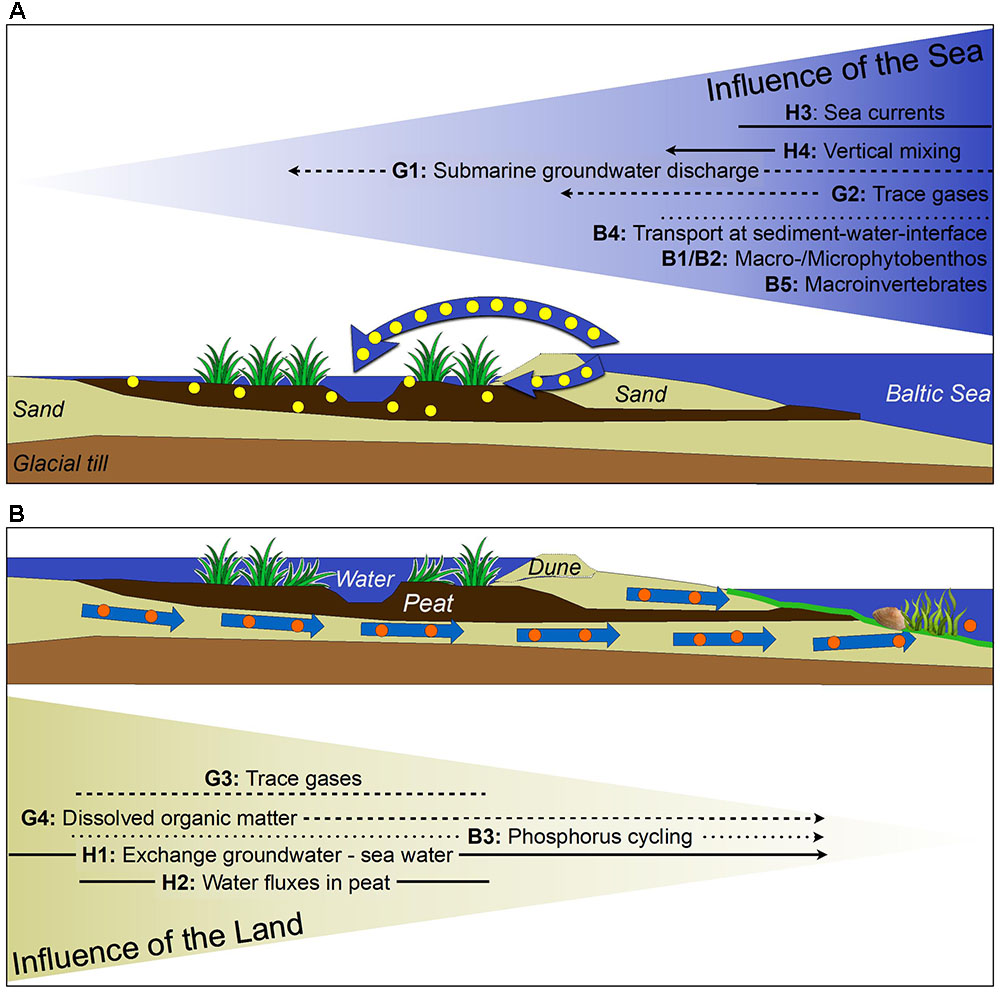
FIGURE 2. Baltic TRANSCOAST scheme showing the spatial coverage of the different research topics (— H — hydro-physical, --- G --- biogeochemical, ⋅⋅⋅⋅ B ⋅⋅⋅⋅ biological) and the prevailing water and substance exchange pathways across the coast line at two different possible states: (A) During storm surge events brackish seawater flows into the coastal peatland (above surface and through the sediment); (B) When the groundwater and surface water levels at the land side are high during winter, submarine groundwater discharge (SGD) may export nutrients and dissolved organic matter (DOM) to the shallow sea (not to scale). The spread of the lines indicate the spatial coverage of the respective topic across the coastal ecocline. Arrowheads indicate that those topics have a specific focus on exchange in the direction of the arrow. Non-arrowed topics consider two-way exchange processes instead.
The research in Baltic TRANSCOAST addresses the following main hypotheses:
(i) There is an exchange of water and matter between land and sea in both directions, on varying spatial and temporal scales at shallow coasts.
(ii) Both on land and in the sea, exchange processes influence the biogeochemical processes either directly or by impacting the biota.
(iii) The volume flux of water originating from the terrestrial side (SGD) in the shallow sea may be a low but possibly constant source of nutrients and complex organic molecules.
(iv) The impact of sea-borne water on the terrestrial side is expected to be episodic but with long lasting consequences for biogeochemical cycling.
The research designed to test these hypotheses is arranged around three core thematic areas, i.e., hydro-physics, biogeochemistry, and biology with topics anchored in one of the thematic areas but extending into other areas (see Figure 2).
Hydrophysics
In non-tidal systems, the water exchange between the sea and land is driven by vertical and horizontal exchange flows generated by wind, waves, and lateral density gradients (Figure 2). The hydraulic properties of the prevailing strata as well as exchange processes between porous media and the above lying water column are likewise influencing the linkage between the terrestrial and the marine compartment of the ecosystem. The hydro-physics thematic area in Baltic TRANSCOAST comprises oceanographic (sea side) and groundwater (land side) modeling as well as their coupling via water levels near the shoreline. The underlying hypothesis is that water fluxes between the land and the sea are substantial even at low lying coastal areas because of changing sea levels and variations in precipitation. These water fluxes are assumed to be enhanced locally, when the heterogeneity of the underground causes a concentration of flow pathways at specific locations.
Sea level dynamics are wind-driven in non-tidal systems and modeled in Baltic TRANSCOAST by interactively coupling a General Estuarine Transport Model (GETM) with a wind wave model (Moghimi et al., 2013). Near-shore hydrodynamics are determined by wind-driven transport and the plume of the adjacent Warnow River. The groundwater dynamics are represented using the MODFLOW code and measured groundwater levels along a land–sea transect. Compared to mineral substrates, organic soils forming the peatlands are often characterized by low bulk density, high porosity, and a non-rigid organic matrix. Hydraulic and solute transport properties are highly dependent on soil structure, and thus can change upon mechanical, hydraulic, and geochemical impacts (Rezanezhad et al., 2016). Hydraulic properties of the various substrates are either tested in situ or measured in the lab under varying salt concentrations. Hydrodynamic measurements and modeling are supported by geochemical stable (2H, 18O), non-stable isotope (Ra, 3H), and noble gas (He, Ne, Rn) analyses aimed at identifying flow pathways, benthic-pelagic coupling, SGD, and groundwater residence times.
Biogeochemistry
Peat-dominated wetlands are typically water saturated and, therefore, have low redox potentials. Consequently, they have unique nutrient and carbon cycling patterns that may also alter the processes of surrounding soils and sediments. In coastal areas, the biogeochemistry of peatlands is likely altered when seawater intrudes into the peat body. For example, carbon release and/or sequestration rates may shift and/or additional sulfate may become available for alternative metabolic processes (e.g., during storm surges or via saltwater intrusion). Under the impact of SGD, biogeochemical processes, including greenhouse gas (GHG) emissions in the shallow sea, may differ substantially from non-impacted sites because of differing supplies of electron donors and acceptors (e.g., Böttcher et al., 2018). The availability and transformation of dissolved sulfate is particularly important for carbon and nutrient (e.g., nitrogen and iron) cycling. In Baltic TRANSCOAST, we investigate the pathways of carbon, nitrogen, phosphorus, and sulfate across the land-ocean interface to foster our understanding of the complex interactions of salinity, substrate availability, water and element exchange, and geochemical signal formation in soils and sediments.
We hypothesize that the occasional flooding of the peat body with brackish sea water modifies the degradation of the peat substrate and the release of DOM from the peat, with possible consequences for the reactive transport of different forms of carbon, nitrogen and phosphorus, dissolved inorganic carbon (DIC), and metals to the shallow coastal waters. The exchange of compounds between the Baltic Sea and the peatland (and vice versa) will affect microbial processes and the offshore generation and emission of trace gasses since electron acceptors and donors are transferred between the compartments (e.g., dissolved sulfate enters the peatland). Pore waters and sedimentary substrates are therefore analyzed on transects along the land–sea interface to investigate vertical profiles of concentrations and stable isotope compositions of various dissolved, solid, and gaseous compounds, as well as to characterize the underlying biogeochemical processes and the impact of microbial activities. In addition, the composition of surface waters in the peatland as well as in the shallow Baltic Sea allows for an estimate of the exchange of water and substances between the sediments/soils and the overlying water column (i.e., ‘benthic-pelagic coupling’).
Biology
The hydro-physical and biogeochemical conditions in the shallow coastal sea create specific environments for the biota. Salinity variations caused by freshwater input into the shallow coastal waters may impact the physiological performance, viability, biodiversity, and distribution of microbes, micro- and macroalgae, as well as zoobenthos including bioturbators such as polychaetes and/or bivalves. The input of recirculated seawater and freshwater and the nutrients therein can generate nutrient-enriched conditions in the vicinity of SGD, creating hot spots of biological productivity and shaping the local benthic communities as observed in, e.g., estuaries (Encarnação et al., 2013; Leitão et al., 2015). Changes in the activity or abundance of zoobenthos may in turn affect the sediments via feedback mechanisms such as shifts in hydraulic properties of the seabed because of a modified bioturbation activity. These processes and reactions are only poorly understood up to date and are, thus, given priority in the investigation in Baltic TRANSCOAST in the biological thematic area.
Submarine groundwater discharge and unique seabed properties (e.g., peat out-cropping) may affect the micro- and macro-phytobenthos communities. Frequent resuspension events will likely modify the benthic communities, the oxygen consumption in sediments, and the (re)growth of flora and fauna. We hypothesize that these disturbances may change the community composition of micro- and macrobenthos and influence rates of primary production by nutrient inputs from SGD and/or terrestrial runoff. In addition, benthic diatoms typically exhibit a high heterotrophic potential, and hence might benefit from DOM derived from terrestrial sources. Furthermore, direct impacts of salinity fluctuations on biota are one of the most intriguing aspects of this study. Even small changes in salinity are probably crucial for species composition in the shallow waters at the study site, with opposite effects on benthic and pelagic communities (for review see Telesh et al., 2013). Furthermore, salinity fluctuations can affect secondary productivity and energy fluxes in macrozoobenthic communities through the effects on energetic processes, oxygen consumption, and growth and bioturbation activities of key macrozoobenthos species. We test the hypotheses by determining the effects of salinity fluctuations on bioenergetics of common bioturbators (i.e., sediment-dwelling bivalves). We assess the tolerance thresholds beyond which the physiological impacts of salinity translate into reduced activity, growth and/or survival, thereby affecting the key ecological functions of bioturbation and bioirrigation. The physiological data are upscaled to community-level effects by developing physiologically realistic models of bioturbators’ contributions to oxygen, nutrient, and water fluxes, as well as to the sediment reworking. The models consider the effects of environmental factors (such as temperature, salinity, or sediment characteristics) and biological traits (such as body size and/or bioenergetics status) of the bivalves.
Study Site and Instrumentation
Terrestrial Side: Low-Lying Land With Coastal Peatland
Baltic TRANSCOAST focuses on a common investigation site, the nature reserve ‘Heiligensee und Hütelmoor’ (in brief and in the following ‘Hütelmoor’). It is located on the southern Baltic Sea coast in northeastern Germany, near the city of Rostock and the estuary of the river Warnow. The reserve stretches over an area of 540 ha with 315 ha covered by peatland. A local depression shaped by a former glacial stream (Kolp, 1957) served as basis for the development of a fen peatland that formed when the rising sea level of the Baltic Sea caused rising groundwater levels on the landside. The climate is temperate in the transition zone between maritime and continental with an average annual temperature of 9.1°C and an average annual precipitation of 645 mm (data derived from grid product of the German Weather Service, reference climate period: 1981–2010). The evapotranspiration amounts to 620 mm in open water and 581 mm a-1 in reed areas (Miegel et al., 2016).
The peatland type in this site can be classified as a coastal paludification fen, and the largest portion of the peatland is covered by 1–3 m thick layers of sedge and reed peat. Due to its low elevation (-0.1 to + 0.7 m HN), the site was influenced by intermittent flooding events, resulting in the formation of thin sand layers within the peat in some areas. In the surroundings of the Heiligensee, organic or mineral lake sediments are found at the basis of the peat. Underneath the peat, 3–10 m thick basin sands form an aquifer underlain by glacial till. The peatland is surrounded by forest on mineral soils. At the eastern boundary, the peat stretches up to 100 m into the forest featuring Alder carr.
As most peatlands in northern Germany, the Hütelmoor has been artificially drained. The main ditch was installed in the 18th century and mainly served for transporting wood from the surrounding forests to the harbor (Kolp, 1957). The ditch was connected to the lower reach of the river Warnow. Originally, the area had discharged directly into the Baltic Sea via a small brook cutting through the dunes; this connection was later blocked. In accordance with intensifying agricultural production in central Europe (van Diggelen et al., 2006), large parts of the wetland complex were intensively drained in the 1970s by open ditches and then used as grassland for fodder production (i.e., mown twice a year). As a result, the mean annual water level dropped to 1.60 m below ground surface.
In the 1990s, the study site was moderately rewetted and grasses were cut once a year to keep the vegetation open. Extensive mowing supported the aims of nature conservation to preserve a resting site for migratory birds while allowing the restoration of the typical fen vegetation. The mean growing season water level during that time was close to the surface (Koebsch et al., 2013). However, water levels dropped down to 70 cm below the surface during summers, which raised concerns about ongoing aerobic peat decomposition. Therefore, a ground sill was installed in the outflow of the catchment in winter 2009/2010 which initiated a year-round shallow flooding for most areas of the study site (mean growing season water level 37 cm, values indicate flooding). The discharge from the peatland averages 44 L s-1, but stops during summer months when the water level drops below the ground sill (Miegel et al., 2016). Rewetting projects are common for much of the Baltic Sea coast where nature conservation has been given priority.
Currently, the vegetation is dominated by emergent macrophytes such as Common Reed [Phragmites australis (Cav.) Trin.]. Especially in the second part of the vegetation period submerged aquatic species like Soft Hornwort (Ceratophyllum submersum L.) dominate the relatively abundant areas of open shallow water. The typical brackish water emergent macrophyte Sea Clubrush (Bolboschoenus maritimus L.) was almost completely displaced, whereas another brackish water species, the Softstem Bulrush [Schoenoplectus tabernaemontani (C.C.Gmel.) Palla], was able to increase its cover considerably in the last decade (Koch et al., 2017). Dryer areas at the boundaries to the forest are mainly colonized by the Lesser Pond Sedge (Carex acutiformis Ehrh.) and the Common Rush (Juncus effusus L.).
Drainage and agricultural use led to aeration and mineralization of the peat resulting in a highly degraded top horizon. The soil type was identified as sapric histosol before rewetting. The remaining peat body is moderately to strongly decomposed with substantial heterogeneity of physical and chemical properties. Rewetting measures and permanently high water levels (above peat surface) have led to the sealing of the soil surface because of sedimentation of fine particles. The former soil surface now resembles a lake bottom surface, with the possible formation of a gyttja (a characteristic layer composed of either organic or mineral material that can be typically found beneath fen peats, which is considered as the initiation stage of fen peat formation). The ongoing sealing process at the soil surface limits the vertical infiltration, thus, possibly changing the hydraulic functioning of the entire wetland.
A dune dyke was installed at the coastline of the fen in 1903 for coastal protection of the nearby seaside resort Markgrafenheide and was reinforced in 1963 (Miegel et al., 2016). Already before 1903 there was a natural sand dune providing a barrier between the Baltic Sea and the Hütelmoor. Due to the artificial dune dyke the peatland has been almost completely cut off from the Baltic Sea. The last notable intrusion of brackish water occurred during a storm night in November 1995 (Bohne and Bohne, 2008). Afterwards, the dune dyke was rebuilt and strengthened. It is, however, not maintained anymore since the year 2000. It is anticipated that the natural impact of the sea including episodic flooding will re-establish in the near future likely resulting in repeated input of brackish waters (on decadal time scales) with possible consequences for hydro-physical and biogeochemical processes. A recent storm flood event in January 2017, which washed away considerable parts of the dune dyke at places where it was more than 20 m wide, failed to flood the study site.
Marine Side: Shallow Coast of the Baltic Sea
A mosaic pattern of eroding cliffs and sand deposition areas are dominant along the western part of the Baltic Sea coast in northeastern Germany. Due to coastal erosion and isostatic sea level rise the coastline has shifted landwards at the study site exposing former land-based geological strata to the sea. The peat that once developed on land now extends to the sea, forming a unique habitat for micro- and macro-phytobenthos. Most of the coastal area between 0 and 10 m depth is covered by coarse-grained sediments with gravel and cobbles dominating at some sites. In the northern area of the study site, gravel and coarse sands are abundant on the sea bottom surface whereas in the south fine and medium sands dominate (for details and a map, see Kreuzburg et al., 2018). This is typical for sea floor near the coast because coastal waves and prevailing south-westerly winds generate longshore currents, displacing the small particles and transporting them along the coast and toward greater depths. A few tens of meters away from the shoreline an underwater longshore bar has developed. This seems to be typical for the southern German Baltic coastline where no fine-grained sediments can be found (Tauber, 2012).
Roughly 10 km west from the study area, the Warnow estuary enters the Baltic Sea. The Warnow river basin is approximately 3,000 km2 in size with elevation differences of less than 100 m as is typical for lowland catchments mainly used for agriculture (Bahnwart et al., 1998; Deutsch et al., 2006). The river mouth is located 15 km landwards where a weir prevents sea water to travel further upstream. A gauging station is located at the weir and discharge is continuously recorded. The mean outflow rate is 16.5 m3 s-1 with a mean nitrate concentration of 1.78 mg N L-1 (or 127 μmol L-1)1. A small surface plume extends a few kilometers west – and northwards along the estuary while the salinity increases to the typical brackish values of 10–15 PSU. During the passage, inorganic nutrients are entirely consumed or mixed so that only recalcitrant substances like dissolved organic material of the plume are able to reach the study site off the Hütelmoor.
Instrumentation and Field Data Acquisition
The investigations in Baltic TRANSCOAST are carried out on a well-developed research site, the study site Hütelmoor. Some analyses within the project are based on data recorded before the project started in 2016. The investigations on the landside in the peatland have already started in 2008 with closed chamber measurements and the installation of dip wells. In 2009 an eddy covariance tower measuring CO2 exchange was set up, which was equipped with a methane sensor in 2011 and is running continuously ever since.
In the course of Baltic TRANSCOAST, the study site has been instrumented both on land and in the sea (Figure 3). The investigations started in 2016 and are ongoing. The now used locations for chamber measurements are arranged in clusters around the Eddy tower (Figure 3) with replicates in different vegetation types including, e.g., stands of Common Reed and Common Sedge as well as areas with open water and are equipped with boardwalks and dip wells. In each cluster a peeper is installed that allows for regular sampling of pore and surface water at defined depths. GHG chamber measurements and water sampling are carried out monthly. The chambers are custom developed and flexible which allows for measuring vegetation up to 2m height while still allowing for efficient application in the field (Günther et al., 2014). During the campaigns GHG exchange (CH4 and CO2) is measured with either a Los Gatos Ultraportable GHG Analyzer or a Picarro GasScouter.
Groundwater monitoring wells were installed in transects across the peatland in 2016 (Figure 3). Each measuring point consists of up to three wells filtered in different depths (upper sand, peat, lower sand). All wells are equipped with self-registering pressure-temperature-salinity probes and are regularly sampled. The geology of the study site was assessed with 17 drillings, mostly down to the glacial till, and numerous additional peat probings. Undisturbed peat cores (250–450 cmł) for analyses of hydraulic conductivity and dissolved organic carbon (DOC) leaching in the lab were taken both in the peatland and in the peat layer cropping out into the Baltic Sea.
On the marine side, two 4.5 m long stationary pore water lances (set-up modified after Beck et al., 2007) have been installed in the sediments in front of the Hütelmoor, as well as data loggers for salinity, irradiance, and temperature in the surface water close to the groins. Pore waters are retrieved from discrete depth intervals from the stationary pore water lances (Beck et al., 2007) as well as from further stations with mobile lances on a roughly monthly basis.
At greater distance to the shore line on the marine side, sampling took place via small boats. These were equipped with temperature and conductivity sensors, sampling devices for the collection of water and sediments, and further acoustic systems. Biweekly to monthly boat-based excursions have been performed to record temperature/salinity profiles, collect water from the surface and above the sea bottom, and to retrieve sediment samples from a grid of stations (Figure 3). Macrophytobenthos assessment was done by Scuba-diving along transects, as described in Schubert et al. (2011). For species determination of the macrophytobenthos the key used is described elsewhere (Schubert et al., 2011), and synonymies and nomenclature follow Schories et al. (2014).
Details on sample preparation, lab analytical procedures and lab experiments are given in the results section with the respective results.
First Results
Our overarching hypotheses are that there is exchange of water between land and sea across the shoreline along shallow coasts at low-lying areas, that the overground and underground pathways of these exchange processes may act on different time scales, and that biotic and biogeochemical processes on the respective receiving sides of the exchange are altered. In this section we briefly describe some results of our investigations and link them to the hypotheses.
Water Fluxes and Solute Transport
Frequency of Flood Events
Recent studies report a projected increase in storm surge levels in the Baltic Sea under different regional climate scenarios (e.g., Gräwe and Burchard, 2012; Weisse et al., 2014; Soomere and Pindsoo, 2016). According to a European wide modeling study (Vousdoukas et al., 2016), the Baltic Sea shows some of the highest increases in projected extreme storm surge levels in Europe, including increased storm surge activity during all the seasons of the year, but especially during spring and summer. We analyzed how sea levels above certain thresholds relative to mean sea level have changed over the past 60 years (Figure 4) and found that the number of hours per year exceeding thresholds of 0.5, 0.75, and 1.00 m above mean sea level have increased. So far, only the trend for the 0.5 m above sea level is significant. These results suggest, that the risk for salt water intrusion (both by flooding and via the subterranean pathway) into shallow coastal areas at the southern Baltic Sea is increasing because time periods with positive hydraulic gradients from sea to land are expanding, leading to more frequent and further inland reaching salt water intrusions by underground wedges, as well as to increased risk for flooding by storm surges. Thus, an increase of land–sea interactions is to be expected for the future which is of importance to all of our main hypotheses.
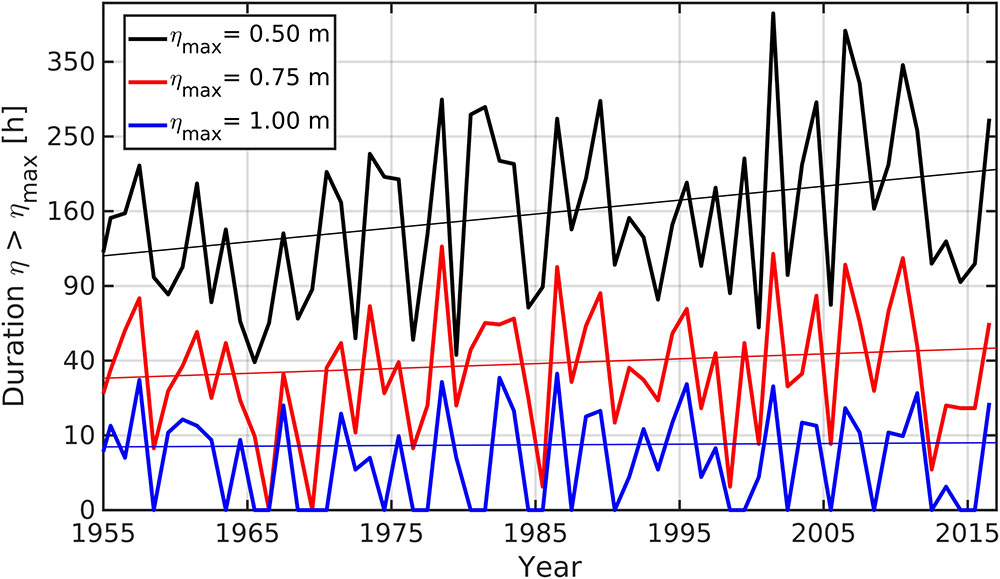
FIGURE 4. Number of hours per year exceeding a certain mean sea level. The trend for the 0.5 m threshold above m.s.l. is significant (Southern Baltic Sea Coast). The time series of sea surface elevations were taken from the gauge at Warnemünde and were provide by the Federal Maritime and Hydrographic Agency of Germany.
Warnow River Plume
The discharge of the Warnow-river follows an annual cycle with maximum values of between 50–60 m3 s-1 in February and lowest values of <5 m3 s-1 in mid-summer (Figure 5). To realistically simulate the hydrodynamic conditions in the Warnow Estuary and the adjacent coastal region including the interaction with the Baltic Sea, a nested model approach using the GETM (Burchard and Bolding, 2002) has been applied. This coastal ocean model is based on the three-dimensional hydrostatic momentum equations and transport equations for temperature, salinity, and passive marker tracers to track water masses. Due to the important role of turbulent mixing for the coastal zone, a two-equation turbulence closure model is used (Umlauf and Burchard, 2005). The model is driven by atmospheric forcing from the German Weather Service Model and lateral boundary conditions from larger-scale GETM model simulations of the entire Baltic Sea (Gräwe et al., 2015; Burchard et al., 2018), with lateral grid resolutions of about 2 km. Multiple one-way nesting is used to downscale these simulations to a lateral grid size of 20 m in the Warnow Estuary and the adjacent coast. Modeling results show that estuarine circulation causes a net inflow near the bed and an outflow near the surface of the Warnow estuary, amplifying the river discharge at the mouth by a factor of ten. According to the classification of Geyer and MacCready (2014), the almost non-tidal Warnow estuary is strongly stratified, due to its relatively low discharge and mixing. Similar to the Chesapeake Bay (Scully, 2010), which falls into the same category, its stratification is, however, strongly depending on wind forcing. Our analyses show that the estuarine discharge of the Warnow can form a river plume depending on the effects of mixing in the estuary and the strength of estuarine circulation. Plume formation is prevented when the estuarine circulation is inverted due to up-estuary wind or an inversion of the salinity gradient of Warnow river and coastal water. When leaving the estuary, the river water is deflected to the east due to earth rotation, forming a coastal current passing the Hütelmoor, as shown in Figure 5. This is amplified by the mainly westerly wind and may influence the measured water chemistry in front of the Hütelmoor. When assessing exchange processes between land and sea following the Baltic TRANSCOAST hypotheses, this is an important contribution that has to be acknowledged.
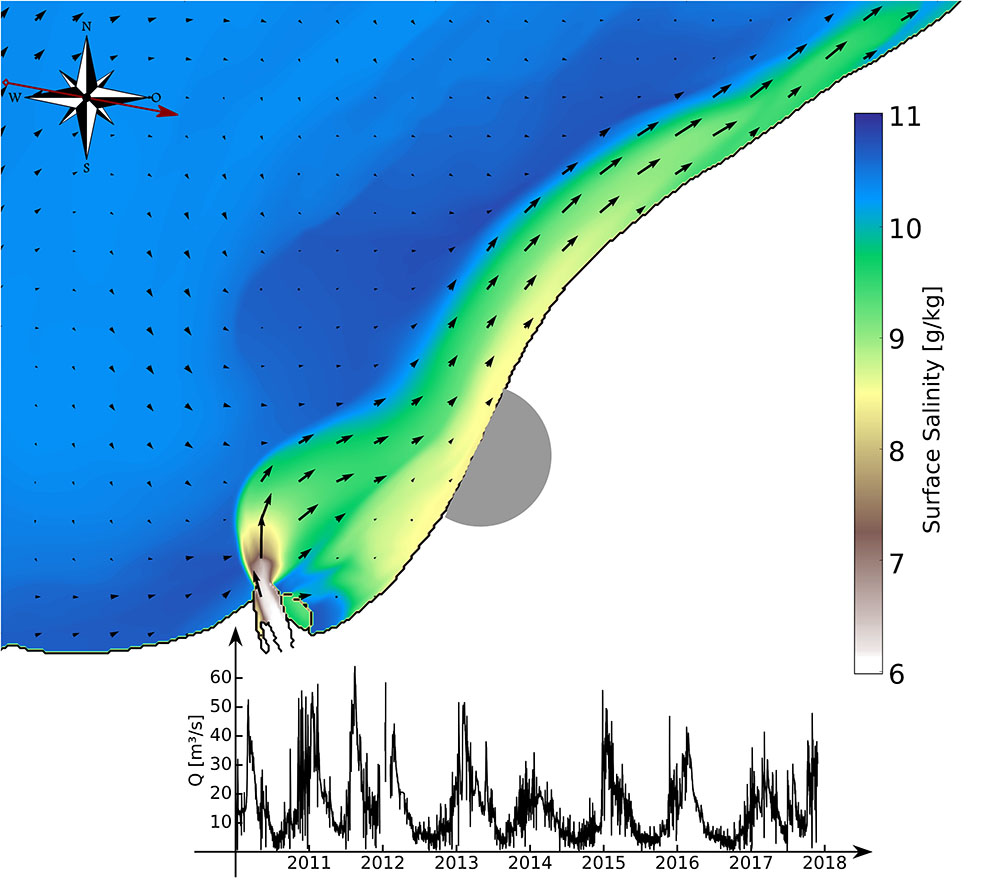
FIGURE 5. Snapshot of simulated surface salinity outside the mouth of the Warnow estuary under westerly wind forcing. Inset: Time series of observed freshwater runoff from the river Warnow during the years 2011–2017, data provided by the Office for Agriculture and Environment Mittleres Mecklenburg.
Hydraulic Gradients Between Land and Sea
Based on preliminary groundwater gauging data and average sea levels, a retrospective schematic of prevailing land-to-sea gradients has been developed (Figure 6). It is assumed that prior to human activities, water exchange processes were limited to events with either high groundwater or high sea water levels. The systematic drainage of the area resulted in negative gradients with a possible inland migration of a subsurface salt wedge. Rewetting of the peat to levels above ground resulted in average gradients in the positive range and, although gradients are still low, freshwater SGD can be traced, at least locally. Peat shrinkage results in a soil profile that is dominated by highly degraded upper horizons, while the peat is less decomposed (lower bulk density, higher porosity) at lower depths. In the Hütelmoor, we found significant peat degradation also up to 1 m depths and below, which is possibly attributed to natural processes operational during the development of the peat. Irrespective of the general situation of the gradient, significantly higher and lower gradients than expected may occur over short periods of time. These “hot moments” are more likely to occur in the future because of a shift of the precipitation patterns toward higher winter precipitation and storm events during summer.
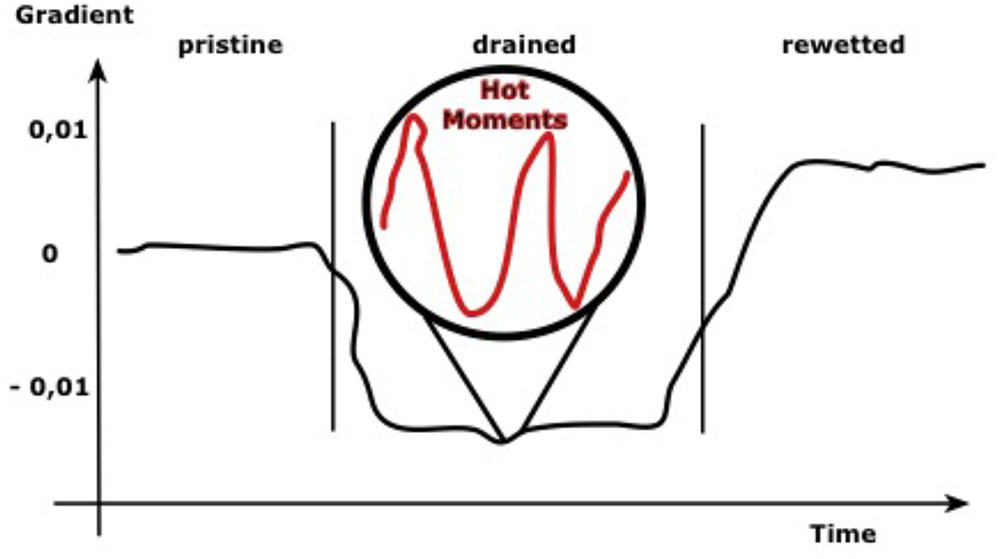
FIGURE 6. Schematic of average land-to-sea gradients in coastal peatlands under different water management scenarios. Irrespective of the general situation, extreme events may cause higher gradients than expected.
Seawater Intrusion
Input of seawater to the peatland can be traced by the salinity of the groundwater. While the fresh groundwater originating from the peatland’s forested catchment has a salinity of about 0.3, the salinity in the Baltic Sea in the study site ranges from 8 to 18 PSU. Recent measurements in groundwater wells across the peatland yielded salinities between 3 and 5 PSU both in the peat and the underlying sand aquifer (Figure 7A). During a previous sampling campaign, which focused on the central areas of the study site, salinities of up to 9 PSU were locally measured in the upper peat layers (Figure 7B; Koebsch et al., 2013). The values show only small variations with time and demonstrate a substantial impact of seawater on the Hütelmoor hydrology and biogeochemistry, as suggested in our first and fourth hypothesis (page 4). Less pronounced seasonal oscillations are presumably due to evaporation and dilution processes. Observations at groundwater wells behind the dune dyke show that storm events with high seawater levels cause subsurface saltwater intrusions into the sands behind the dune dyke, detectable as sudden increases in salinity with subsequent gradual decrease. However, these small seawater intrusions were only observed where the dune is most narrow and could not be detected at 50 m inland of areas with wider dunes. During the decades of drainage, the hydraulic gradients between peatland and Baltic Sea were reversed (Figure 6), suggesting a bigger salt wedge and regular intrusions of saline waters into the peatland by surface flooding via the drainage ditches (which are connected to the Baltic Sea via the Warnow estuary) at that time (supported by Bohne and Bohne, 2008, and an unpublished Master thesis from 1995). It is assumed that the high salinities found further inland in the peatland today are still a relic of former floodings. Peat pore water depth profiles along a transect from the coastline to the forest show that at all four coring locations, except the one closest to the forest, electrical conductivity was increasing with depth, and Selle et al. (2016) describe that salinity in the peat is decreasing slowly since the last flooding in 1995. Dating of the groundwater in the sand aquifer using the 3H/3He method showed that a large part of the water infiltrated before 1950. Only near to the southeastern boundary of the peatland close to the forest, groundwater originated from the year of rewetting (2009). These observations suggest a strong legacy effect of flooding with seawater in the peatland, supporting the hypothesis that the impact of sea water on the terrestrial side is expected to have long lasting consequences for biogeochemical cycling on the land side.
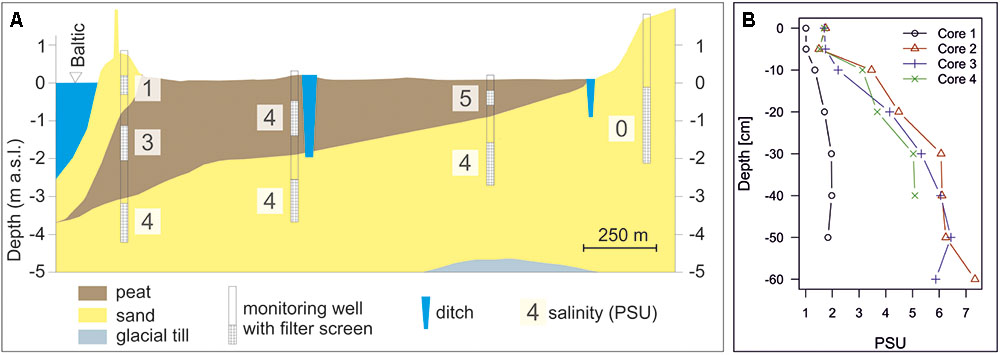
FIGURE 7. (A) Geological profile through the peatland with salinity distribution (PSU); (B) Depth distribution of salinity derived from pore water sampling along a transect from the forest (Core 1) to close behind the dune dyke (Core 4) (see Figure 3). All cores but the one near the forest edge show increasing salinities with depth within the peat.
Combined concentration and stable isotope analysis (C, S) indicates an intense exchange of water and or substances between the soil pore water and the drainage ditches, which can also be seen in the field by enhanced concentrations of metabolites and redox-sensitive metals in surface waters. DIC was enriched in the light carbon isotope compared to Baltic seawater and dissolved sulfate that was already isotopically modified by net microbial sulfate reduction – processes that only take place within the anoxic parts of the soils. Thus, when assessing the intensity of exchange between land and sea at shallow coasts, the influence of man-made structures like ditches has to be considered since the signal on the land side will likely be the result of both exchange processes across the shoreline, and through canals and ditches, which are widespread structures on shallow coasts.
Hydraulic Conductivity of Peat
Fen peatlands and wetlands in general are transitional habitats with associated unique processes occurring between land-based mineral soils and water bodies such as rivers and oceans. However, their buffer function for water and compound fluxes depends on forcing gradients and material properties. With regards to the latter, the saturated hydraulic conductivity (Ks) is of mayor importance, especially in rewetted systems where high water tables prevail. It has been suggested that the geochemistry of pore water may influence the hydraulic properties of non-rigid organic soils. We tested the sensitivity of Ks upon shifts in salinity on peat samples in different stages of degradation (Gosch et al., 2018). The hydraulic conductivity of the undisturbed peat samples was measured with the constant head method, using water with different electrical conductivities ranging from 1 to 55 mS cm-1. For details, see Gosch et al. (2018). The DOC content was measured with a DIMATOC® 2000 analyzer. Our results suggest that salinisation has only minor and non-directional impact on Ks, no matter how strongly we increased the salinity of the water. Our results showed a decrease of Ks with time, which did not depend on the water salinity but was differently shaped for different peat types (ibid.). Interestingly, these findings do not confirm earlier studies in which Ks was found to increase with increasing salinity (Ours et al., 1997). These studies were, however, conducted on bog peat samples whereas we have analyzed fen peat. Although systematic comparisons are missing, it is likely that Ks differs systematically between bog and fen peat since the pore structure of the peats differ strongly.
The hydraulic conductivity, as measured in percolation experiments, is to a certain extent an integrative signal of the soil pore structure (Rezanezhad et al., 2010, 2016). It can, thus, be concluded from the given results that varying salinity conditions do not necessarily modify the pore structure of peat soils as had been hypothesized. This research needs to be continued explicitly addressing pore structure employing imaging methods. A comparative computer tomography analysis on samples from the main peat body and from peat layers outcropping in the sea (continuous seawater impact) may be a promising way to study the effect of sea water on peat structure.
The Sediment–Water Interface
At the marine side of the Baltic TRANSCOAST site, approximately 80% of the area are covered by permeable sands (k ≥ 10-11 m s-1; falling head permeameter). In this sandy environment, hydrodynamic forces mostly related to wave action induce pore water flows when interacting with ripples and similar sedimentary structures. Overlying water is advected into and pore water driven out of the sediment in distinct areas on spatial scales of cm to dm based on ripple dimensions. Ripples change position in response to hydrodynamic forcing. At the coast off Rostock, these changes occur on time scales ranging from minutes to days. From in situ experiments using stirred benthic chambers (Huettel and Gust, 1992) we found fluxes of nutrients between pore water and overlying water to increase two- to fourfold when the sediment interface was exposed to pressure gradients mimicking typical hydrodynamic conditions in the overlying water. Adding the temporal dynamics of the interface topography this suggests a highly dynamic environment with respect to salinity, oxygen, sulfide, and pore water nutrient concentrations.
The discharge of groundwater into a benthic boundary layer agitated by wave motion was implemented in a lab experiment in a water tank of 5 m × 0.8 m × 1 m (l × w × h), which was equipped with a piston type wave generator. The main water volume was seeded with 5 μm polyamide particles to observe the motion of the fluid. The groundwater was marked with fluorescent dye and infused through a permeable sea bed made of porous foam over a surface of 0.7 m × 0.6 m (l × w). The flow measurement was performed by a laser optical PIV-LIF (particle image velocimetry – laser induced fluorescence) setup. Two Flowsense cameras (Dantec Dynamics) simultaneously observe an identical field of view of 132 mm × 241 mm parallel to the flow and perpendicular to the permeable ground. The field is illuminated by a YAG laser at a 7.5 Hz double pulse rate. The LIF camera captures the fluorescent light, which is then quantitatively evaluated indicating the concentration in the groundwater. The PIV camera captures the refracted light from the tracer particles in the flow in double frames. From the particle motion the flow velocities were calculated using an adaptive PIV algorithm with a final interrogation are of 32 pixel × 32 pixel. Both measurements were performed over 6,000 frames/double frames to cover 800 s of lab time. From the time resolved flow velocity and concentration fields the concentration boundary layer and turbulent Reynolds stresses and fluxes were calculated. Concerning the boundary conditions, three scenarios were investigated to cover different levels of agitation in the coastal zone. The “calm” scenario used a period of 1.99 s and an orbital velocity of max. 0.05 m s-1, the “average” scenario 2.52 s and 0.1 m s-1, and the “storm” scenario 3.3 s and 0.25 m s-1, respectively.
The transport coefficients derived from the fields of concentration and velocity for these scenarios can then be used in an improved modeling of the flow and transport in the benthic boundary layer under unsteady conditions. For the quantification of the transport, a 75% concentration of tracer dye was defined as the concentration boundary of the discharged groundwater (Figure 8). A 75% value was chosen to indicate the concentration boundary as it represents the highest gradient. This boundary was observed during a time period of 15 min. Three scenarios with increasing wave amplitude and orbital velocity were investigated. Our results from these measurements show that for a planar topography the vertical transport of the discharged groundwater is dominated by the diffusion velocity, whereas the horizontal transport is governed by the wave motion. Thus, with increasing wave motion the initially pure vertical transport is substituted by a horizontal efflux of the discharged groundwater. Further analysis will allow derivation of model equations of the distribution of discharged groundwater in the benthic boundary layer with respect to the wave motion and the surface topography. These experimentally validated model equations for the turbulent transport can then be used as improved boundary conditions for the numerical simulation of the distribution process on the entire coast bringing us closer to a better understanding of exchange processes on shallow coasts.
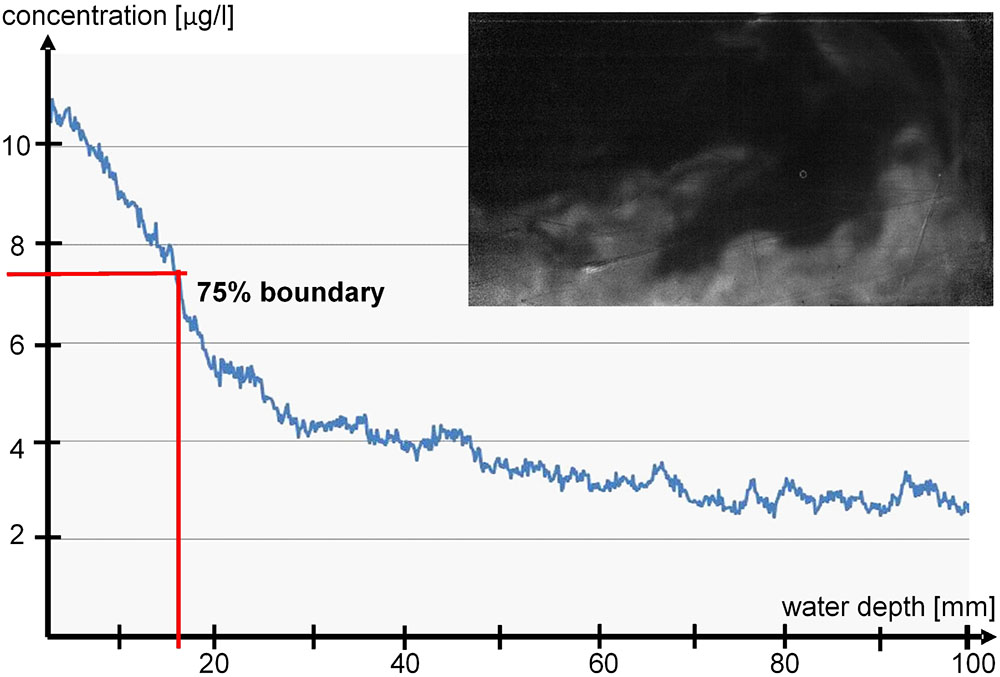
FIGURE 8. Thickness of the groundwater boundary layer in lab experiment derived from the concentration profile of the laser induced fluorescence (LIF) tracer in the discharged groundwater. The profile was derived from a horizontal averaging of the LIF-tracer concentration maps (example map displayed above).
Benthic-Pelagic Exchange of Water and Substances
Offshore monitoring campaigns revealed unique patterns of hydrographic parameters in front of the Hütelmoor due to salinity changes likely originating from the influence of freshwater in front of the Hütelmoor (Figure 9). To investigate the seasonal and event-driven effects on the transport and biogeochemical transformation processes, vertical pore water profiles were retrieved regularly from water lances. The oxygen isotope composition of the pore water was determined with a Picarro L2140-i Laser-cavity ring-down spectroscopy system (Böttcher et al., 2014), the concentration and stable carbon isotope composition of DIC was determined by IRMS using a Gasbench II coupled to a Thermo Finnigan MAT 253 gas mass spectrometer (Winde et al., 2014; Donis et al., 2017). Dissolved sulfide was measured spectrophotometrically (Specord 40 spectrophotometer) according to Cline (1969). Dissolved phosphate and silica were measured from acidified solutions by ICP-OES (Thermo iCAP 6300 Duo Thermo Fisher Scientific).
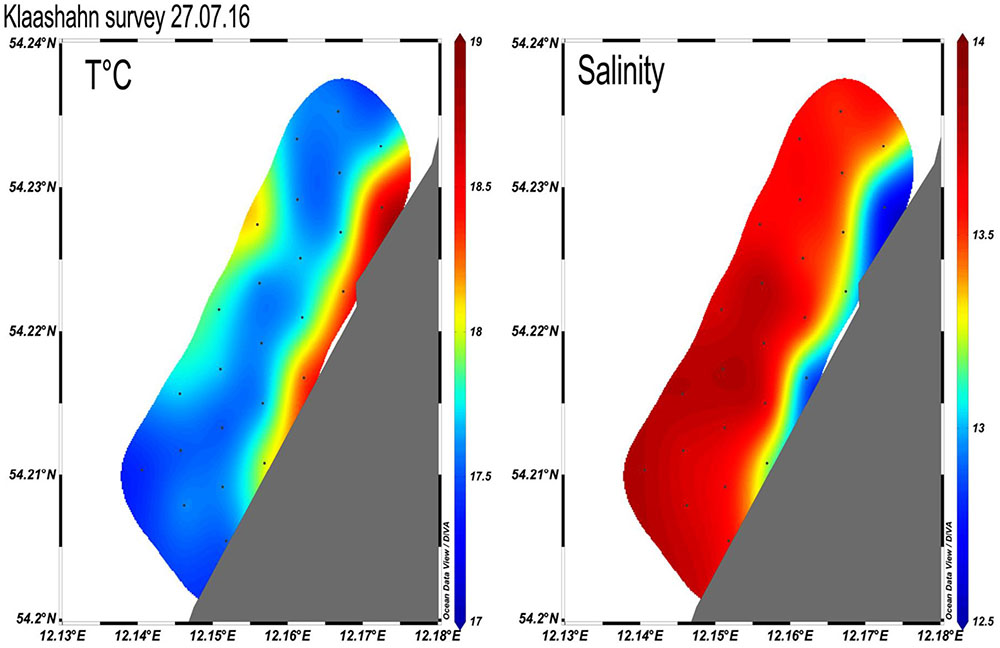
FIGURE 9. Temperature and salinity (PSU) distribution in front of the experimental coastal peatland (summer 2016).
A seasonal study of the Ra isotope distribution in surface waters showed high dynamics, and based on the detection of short-living Ra isotopes, a clear indication for fast and intense benthic-pelagic mixing of Ra-enriched pore waters into the water column. Along the shore line hydrochemical and isotopic composition of pore waters down to 5 m below sea floor were investigated regularly (Figure 10). The found freshening with depth indicates increasing contributions by less saline water that is also isotopically lighter than the Baltic Sea surface water. In parallel, the pore waters display the typical biogeochemical zonation with an increase in the concentrations of DIC, PO4, NH4, and H2S with depth (data not shown). The carbon isotope signature of DIC indicates the addition of biogenic CO2 derived from the mineralization of reduced carbon, probably superimposed by carbonate dissolution. Microbial sulfate reduction is the most important anaerobic process in these sediments leading to stable sulfur isotope signatures in dissolved sulfate and sulfide resembling a system in which the gross sulfate reduction is faster than the sulfide re-oxidation or diffusional/advective transport of sulfate from the bottom waters (e.g., Hartmann and Nielsen, 2012). Sulfide re-oxidation is likely limited to processes in the top part of the sediments and limited at depth due to a lack of reactive iron. The exchange of these modified pore waters with the Baltic Sea water column will lead to an SGD component acting as a source of DOM, PO4, H4SiO4, NO3 (after nitrification of NH4), protons, and an excess of isotopically light DIC. The investigations of the long pore water profiles indicate dynamics in the pore water system which support the hypothesis that the near shore exchange through the sediment is a constant source of nutrients and complex organic molecules to the coastal sea.
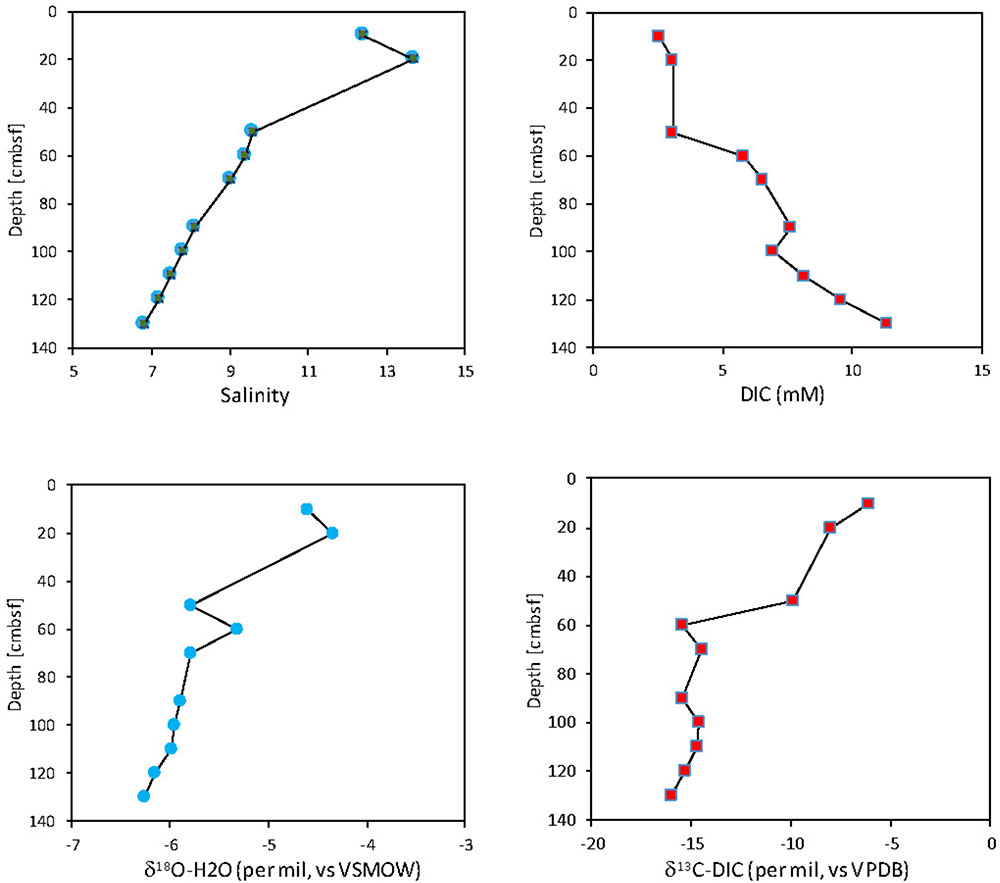
FIGURE 10. Examples for vertical profiles of the pore water composition at a submarine groundwater discharge (SGD) impacted site (taken with a mobile pore water lance in spring 2017). Whereas the water isotopes and salinity (PSU) indicate the contribution of fresh and isotopically lighter water at depth, the dissolved carbonate system shows a clear contribution of biogenic CO2 derived from the anaerobic mineralization of essentially marine organic matter. Dissolved sulfide, the product of microbial sulfate reduction, accumulated about 40 cm below sea floor and also phosphate and silica were substantially enriched in the pore waters when compared to surface water.
Dissolved Organic Matter (DOM)
The release of DOM from peat layers from both the terrestrial and the marine side varied in leaching experiments. DOM was released in higher concentrations from the terrestrial peat as compared to the outcropping peat layers in the sea. The composition of a typical pore water DOM from peat exposed to saltwater as revealed by pyrolysis-field ionization mass spectrometry (Py-FIMS) – a non-targeted soft ionization MS method (Leinweber et al., 2009) indicated the abundance of 1% carbohydrates, 19% of phenols/lignin monomers, 5% lignin dimers, 10% lipids, 21% alkylaromatics, 0.4% mostly cyclic N-containing compounds, 2% peptides, and 4% free fatty acids (percentages as proportion of total ion intensity in the Py-FI mass spectra). This composition differs from the mean composition of other fen peat DOM samples (Leinweber et al., 2009) in larger proportions of phenols/lignin monomers, and alkylaromatics at the expense of carbohydrates, heterocyclic N-containing compounds and peptides. These differences may reflect an influence of salinity on the DOM sampled because saline waters extracted more phenols and lignin monomers and alkylaromatics than freshwater. This finding has important consequences for a future with a predicted increase in flooding events—i.e., an increase of the sea–land influence – because the remaining peat on the land side may be subject to increased physico-chemical erosion reflecting just one process where episodic sea water influxes entail long-lasting consequences for biogeochemical cycling on the land side.
Greenhouse Gas Emissions
Methane Emissions in the Peatland
Due to its evolution and land management decisions in the past (first drainage, then rewetting), the studied coastal fens biogeochemistry is impacted by both fresh and saline waters. In the past, the fen must have received substantial sulfate loads from episodic flooding with brackish water and the legacies of those floodings can still be found in deeper layers of the peat. Despite the high sulfate loads, which are known to inhibit methanogenesis (Bartlett et al., 1987; Giani et al., 1996) or lead to anaerobic methane oxidation (Boetius et al., 2000), the fen became a methane source after rewetting by flooding with fresh water (Glatzel et al., 2011; Hahn et al., 2015; Koebsch et al., 2015). Our analysis of repeated pore water sampling shows that high sulfate concentrations and high methane concentrations from surface to pore waters are spatially separated (Figure 11) suggesting sulfate reduction and methanogenesis to happen in different depth zones and, thus, supporting the hypothesis that episodic flooding with seawater generates strong legacy effects in the biogeochemical cycling on land.
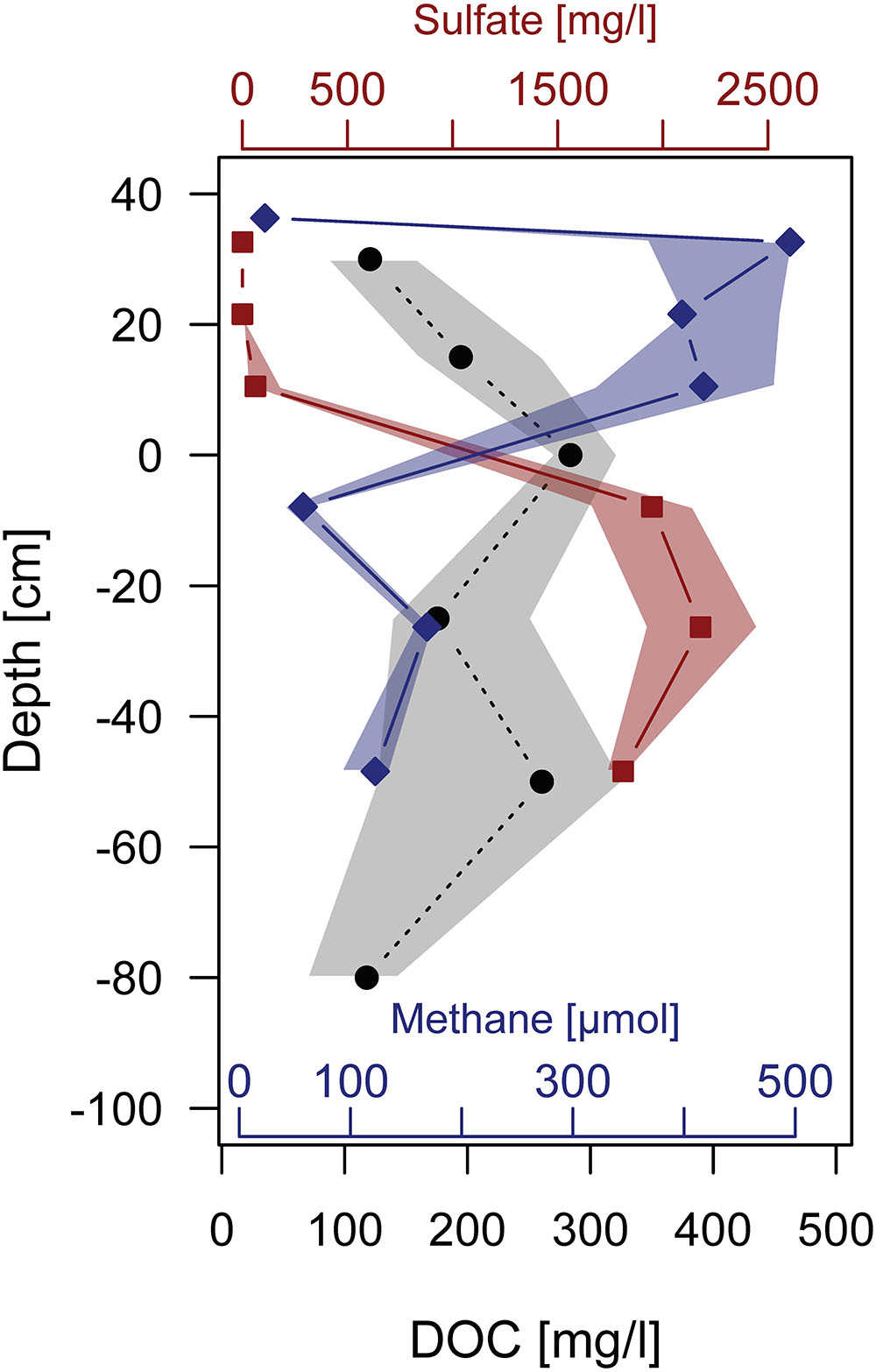
FIGURE 11. (Pore) water profiles of DOC (black, circle), CH4 (blue, diamond), and SO4 (red, square) derived from repeated sampling in the central part of the peatland. Symbols denote median concentrations over monthly samples taken from May to October 2015, shaded polygons stretch from the 1st to the 3rd quartile of the distribution at the specific depths. Lines as well as shading contours are linearly interpolated between depths.
An interdisciplinary analysis of the soil profiles along a transect perpendicular to and with different distances to the shoreline using a combination of stable isotope (H, C, O, S) partitioning, analysis of microbial community structure, and diagenetic pore water modeling enabled us to get a better idea of the prevailing biogeochemical processes (Fernández-Fernández et al., 2017). We found a strong increase in salinity with depth (Figure 7B) at all sampling locations likely caused by residual brackish solutions. The pore water composition indicates substantial net sulfate reduction under sulfate-limited conditions at depth which find their expression in the formation of isotopically heavy iron sulfides and incorporation of sulfur into the organic matter (Fernández-Fernández et al., 2017). This zone of intense sulfur cycling is positioned below a freshened soil zone were methanogenesis takes place, which is dominated by acetotrophic Methanosaeta. Anaerobic oxidation of methane is indicated for the transitional zone. A comparison of pore water and solid phase compositional profiles suggests temporal changes of the reaction zones in the past.
We believe that the development of a shallow methanogenesis zone above a zone of intense sulfur cycling may be a specific feature of episodically flooded coastal wetlands, especially when hydrological changes cause freshening of surface waters. Interestingly this layering of a methanogenesis zone above a sulfate reduction zone is reversal of the anaerobic methane oxidation zone, observed in typical marine sediments (e.g., Boetius et al., 2000; Jørgensen and Kasten, 2006). This highlights the need for a more differentiated perspective on coastal ecosystems as commonly neglected methane sources.
Methane Emissions in the Shallow Baltic Sea
The recorded distributions of temperature and salinity in coastal waters reveal a clear impact of distinctly different waters close to shore showing cross-slope gradients with slightly reduced salinities and elevated temperatures after a short period of stagnation (Figure 9). To analyze the trace gas distribution at the sediment surface, water was filled bubble-free into crimp vials that were immediately sealed. Bottles with 50 ml volume received 130 μl of a saturated HgCl solution and were stored cool and dark until analysis. Trace gas concentrations were analyzed on a gas chromatograph (Shimadzu GC-2014) with an FID (flame ionization detector) for methane and ECD (electron capture detector) for nitrous oxide determination.
Interestingly, the temperature and salinity anomalies are found near the coastal area with emerging peat deposits (Kreuzburg et al., 2018) and near areas with the highest bottom water methane concentrations (mean: 25.3 ± 9.3; range: 15–55 CH4 nmol L-1, Figure 12). Since peat may release DOC with advection of low saline pore water (Tiemeyer et al., 2016), these coastal sediments have an increased potential of organic matter release due to hydrological dynamics. The input of peat-derived DOC likely enhances CH4 production rates (Aravena and Wassenaar, 1993; Liu et al., 2011) in coastal surface waters. These findings match new data from the southern North Sea, where increasing concentrations and air-sea fluxes of methane were reported toward the shore in the Belgian coastal zone, in particular in areas of free gas occurrence (Borges et al., 2016). The free gas occurrence in the Belgian coastal zone has been suggested to be generated by the decomposition of a thin Pleistocene peat layer zone (Missiaen et al., 2002), which is a somewhat similar situation to ours. Thus, our results support the hypothesis that SGD is increasing the inflow of DOC and nutrients into the coastal zone and thereby instigating changes in biogeochemical cycling in the coastal sediments.
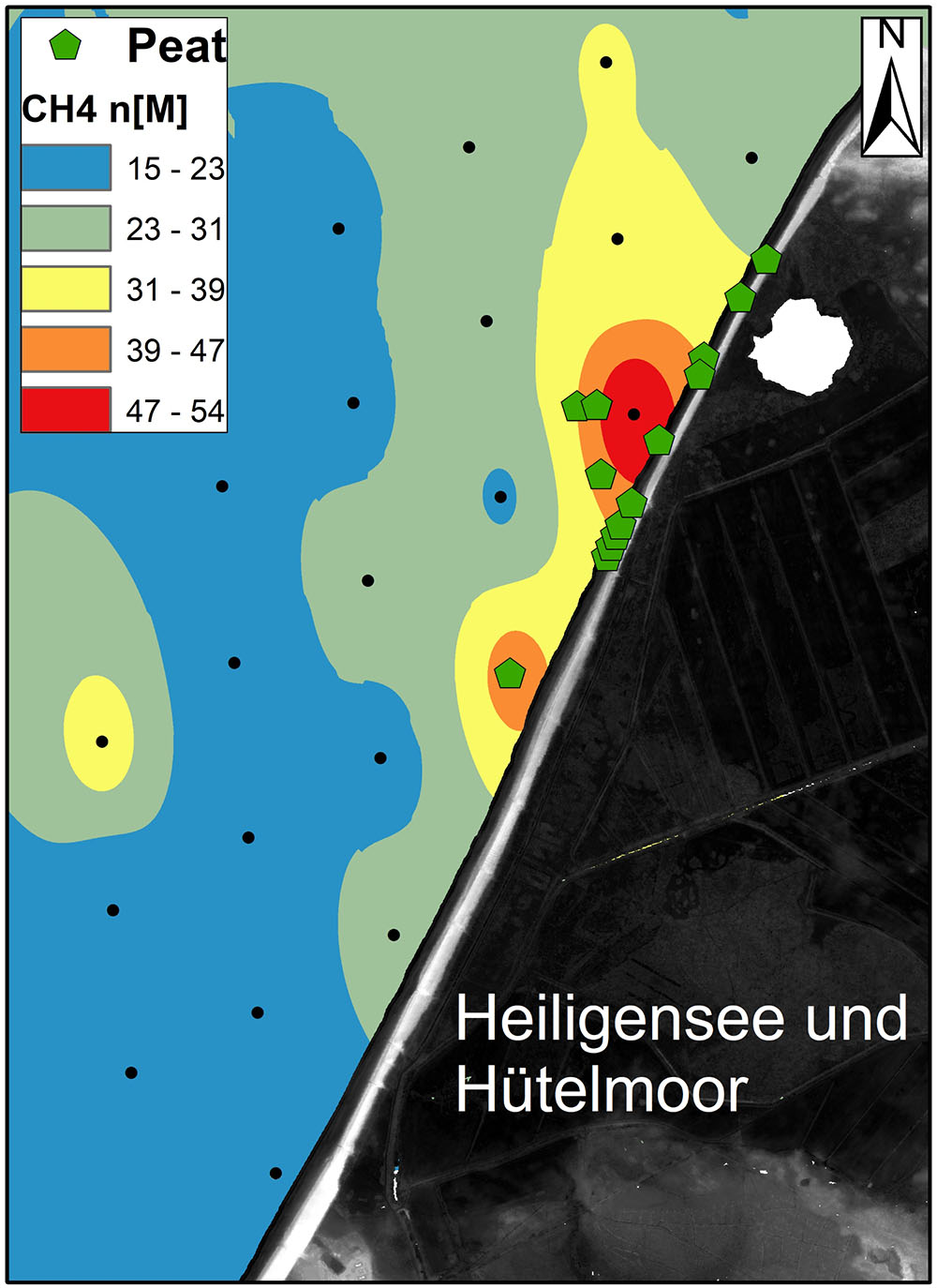
FIGURE 12. Dissolved methane distribution in the shallow Baltic Sea in front of the study site, composite of several measurements in June 2017.
Coastal-near dynamics apparently foster the transfer of methane from the sediment toward the water column, which is usually oxidized by effective anaerobic and aerobic oxidation (Knittel and Boetius, 2009). However, our data suggest that elevated methane concentrations near the shore are hardly oxidized in the water likely due to the shallow water depth suggesting possible methane emissions from the coastal waters to the atmosphere. In the future we plan to assess whether or not this is contributing substantially to atmospheric methane fluxes.
Ecological Effects of Freshwater Fluxes and Peat Outcropping in the Baltic Sea
Macro-Phytobenthos
Salinity plays a major role for macrophytobenthos in the eutrophic southern Baltic Sea (Volkmann et al., 2016). The number of macroalgae taxa has been shown to decrease with decreasing salinity in the Baltic Sea (Schubert et al., 2011; Telesh et al., 2013), yet so far the potential role of irregular freshwater input as an additional stressor has not been studied. Frequency as well as the amplitude of variability in salinity is the decisive factor for occurrence/absence of species at a given site (Telesh et al., 2013). In this context, SGD must be seen as an additional factor of variability, impacting macro- as well as microphytobenthic community composition. Our study site is located in a critical salinity range, where small local changes in salinity may cause drastic effects in community composition. Similarity between closely neighbored communities has been shown to drop sharply in this salinity range, indicating the relatively strong effect of small perturbations (Schubert et al., 2011). Transect sampling was thus performed in front of the Hütelmoor to estimate the impact of irregular freshwater pulses on the taxonomic composition of the macrophytobenthos community.
A striking result is the almost complete absence of Fucus spp., the only habitat-forming species able to grow in this region of the Baltic Sea (Wahl et al., 2011). Without this species, the macroalgae community in front of the Hütelmoor is severely species-depleted, consisting mainly of branched filamentous red algae, which contribute >80% to the total biomass both in spring and autumn. Total biomass along the depth gradient showed a sharp decline below 2 m water depth (Figure 13, left panel), which is consistent with the steep irradiance gradient, as can be seen from the attenuation spectra (Figure 13, right panel). The reason for the absence of non-filamentous, perennial species as well as dominance of red algae at the depths preferentially occupied by green algae needs to be investigated by means of physiological studies of tolerance against salinity fluctuations. If the species expected to be found at these depths and/or region (such as Fucus spp. or green algae) turn out to be less tolerant to salinity fluctuations than those found in abundance near the Hütelmoor, this would provide a strong support for our hypothesis that land–sea exchange processes impact the relevant biota in the coastal sea.
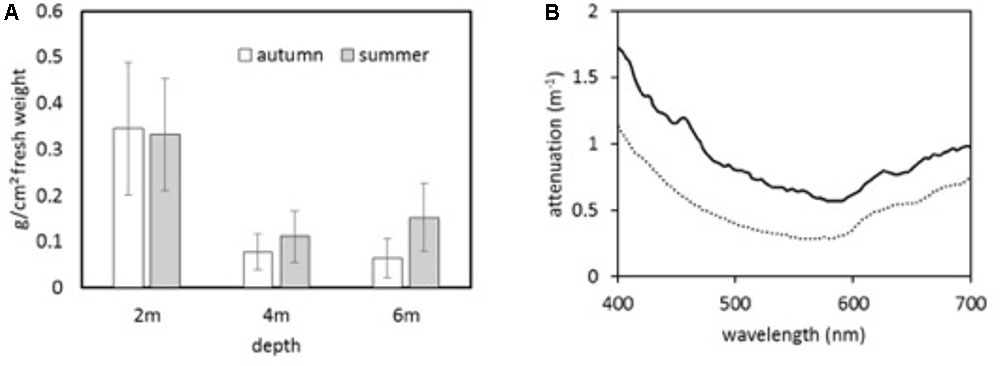
FIGURE 13. (A) Total fresh weight of macrophytobenthos along a transect in front of the Hütelmoor in autumn and summer 2016. For each depth, six replicates were sampled by means of Scuba-diving; (B) Attenuation spectra recorded in July (solid line) and October (dotted line) close to the transect position. Lower attenuation in autumn is overcompensated by lower solar elevation.
Micro-Phytobenthos
In contrast to macroalgae, benthic diatoms are much less affected by salinity fluctuations. In a previous study, three abundant benthic diatom taxa were isolated from sandy sediments sampled at a shallow-water area (15–30-cm water depth) (54°01.490′ N; 11°32.110′ E) at the southern Baltic Sea coast, the so-called ‘Boiensdorfer Werder’ opposite of the island Poel and the Mecklenburg Bight, and their salinity tolerance was evaluated (Woelfel et al., 2014). Benthic diatoms were isolated from the top 5 mm layer of the sediment cores, purified and established as unialgal cultures according the protocol of Stachura-Suchoples et al. (2016). These strains were used to measure growth and photosynthesis under various controlled abiotic conditions (Woelfel et al., 2014). Surprisingly, all species were characterized as euryhaline, growing across a wide range from almost freshwater to hypersaline conditions (1 up to 50 PSU). Consequently, these representative taxa can cope with very low in situ salinities, and hence SGD will most probably not have a negative osmotic effect on photosynthesis and growth.
In contrast, preliminary data on the influence of DOM in the Hütelmoor water body on benthic diatoms indicate a stimulating effect on growth of two species isolated in the shallow water in front of this fen peat site. To determine the effect of DOM in the Hütelmoor water body on heterotrophic growth in the dark, benthic diatom isolates were incubated for 10 days in darkness in 6 different chemically defined media. Afterwards the cell numbers were estimated, and the values were compared to a control. The Hütelmoor water organic compounds led to a statistically significant stimulation of growth in darkness, which suggests heterotrophic behavior. Other benthic diatoms have been experimentally characterized as mixotrophic microorganisms that photosynthesise in light and easily can switch to heterotrophy under dark conditions (Kitano et al., 1997). The heterotrophic lifestyle of benthic diatoms mainly includes the uptake of bioavailable sugars, amino acids, organic acids and other organic substrates from the environment, and some active transport mechanisms for such compounds are identified, which seem to be substrate and light regulated (Hellebust and Lewin, 1977; Armbrust et al., 2004). From an ecological standpoint, heterotrophy seems to be a very important mode of nutrition when cells are buried into the sediment because of unstable surface conditions in the shallow water habitat caused by wind, waves, and currents. Although benthic diatoms can actively move vertically in the sediment, this process requires energy and the uptake of organic substances from the peat might facilitate such movement to escape darkness. Our results suggest that SGD with increased DOM concentrations in front of the Hütelmoor may potentially increase the growth of benthic diatoms supporting the hypothesis that exchange processes alter the growth conditions on the seaside.
Zoobenthos
The multiple interconnected environmental shifts associated with SGD (such as changes in salinity, temperature, seawater chemistry, and nutrients) result in complex interactive effects on the benthic organisms inhabiting these areas that can be effectively understood by investigating the impacts on the energy fluxes through the organisms and the ecosystem (Figure 14). Physiological assessment of the burrowing capacity and endurance of marine bioturbators (the softshell clams Mya arenaria) as well as the energy costs of burrowing were conducted as described elsewhere (Haider et al., 2017). Briefly, clams were acclimated to the normal (15 PSU), low (5 PSU) and fluctuating (5–15 PSU) salinity for 3–4 weeks, and their burrowing speed and endurance was determined using video recordings during repeated forced burrowing. The respiratory activity of isolated mitochondria as well as the activity of the mitochondrial electron transfer chain in the tissue extracts were measured as proxies for the clams aerobic capacity under the resting conditions and after repeated burrowing. Energy costs of burrowing were assessed by measuring the amounts of tissue energy reserves (lipids, proteins, and carbohydrates) under resting conditions and after repeated burrowing, and the energy cost was calculated as the % of the total body reserves (expressed as energy equivalents) used to fuel a single burrowing cycle.
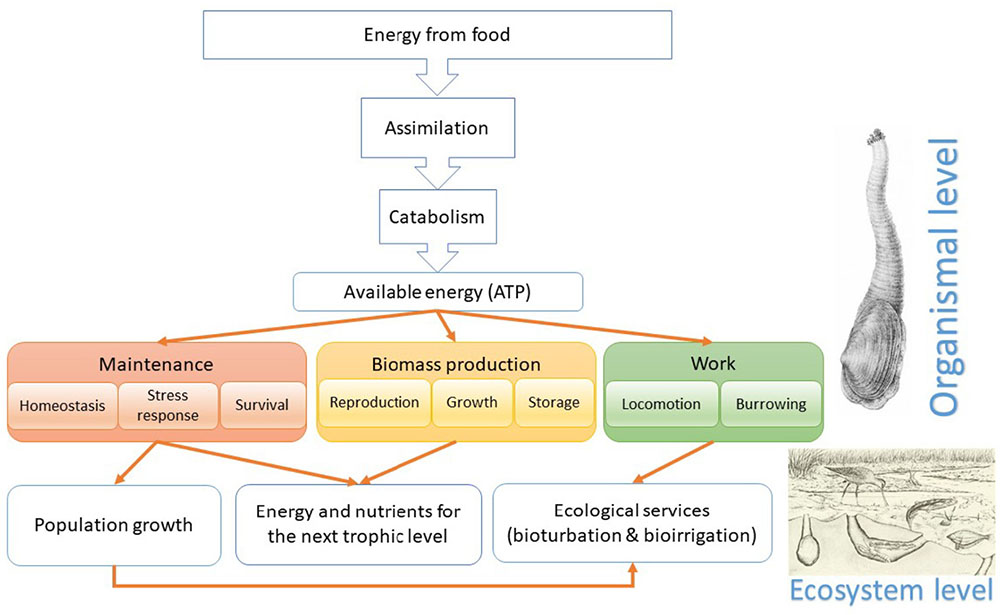
FIGURE 14. Bioenergetics-based model integrating the physiological effects and ecological consequences of multiple stress exposures in coastal habitats. Images are obtained from the Wikimedia Commons and are in the public domain.
Sediment reworking by bioturbators is considered one of the most energy expensive modes of activity, and our estimates show that a single digging cycle of a marine bioturbator M. arenaria uses up on average 7% of its energy reserves (Haider et al., 2017). Thus, it is not surprising that abiotic stressors (such as low and/or fluctuating salinity characteristic of SGD) negatively affect the burrowing capacity of sediment-dwelling zoobenthos including clams, polychaetes, and echinoderms (Turner and Meyer, 1980; Lardies et al., 2001; Przeslawski et al., 2009; Haider et al., 2017). These changes may have important implications for the ecological functions of bioturbators affecting the sediment reworking by the resident biota and the associated influx of oxygen and nutrients into the deeper sediment layers (Figure 14). Furthermore, fluctuating salinities led to changes in the biochemical composition of the clam tissues (Haider et al., 2017), potentially impacting the nutritional quality of the food for the top-level predators feeding on clams in this shallow coastal ecosystem.
Currently, we are developing bioenergetically based ecological models to assess the effects of the common environmental stressors (including salinity and temperature change) on survival, growth, and digging activity of the clams by assessing the effect of these factors on energy assimilation, maintenance costs, and energy available for the biomass production. Our model considers the effects of multiple stressors (regardless of their nature) on the distribution of energy fluxes through the organism and their implications for the higher-level ecosystem functions. For example, salinity shifts caused by SGD or freshwater run-off may cause physical disturbance by hydrodynamic forces, or warming may cause physiological stress, increasing energy demand for maintenance, altering the energy and nutrient content of the tissues, and negatively affecting the capacity to do the work. Negative impacts of abiotic stressors might be modulated and partially offset by increased food supply due to the stimulation of the algal production near the freshwater influx. These changes in energy fluxes and energy trade-offs between different fitness-related functions translate into changes in ecological performance of the organisms and ecosystem services they provide (such as bioturbation or trophic energy transfer) (van der Meer, 2006; Sokolova et al., 2012; Sokolova, 2013). Such models serve as mechanistically based tools to understand and predict the complex effects of the environmental shifts caused by SGD on the local communities and ecosystem services they provide and will help us to test the hypothesis that increased inflow of water from land to sea imposes substantial changes to the marine food web.
Conclusions/Outlook
A system approach, in which the coast is considered as a continuum that extents from land to sea, seems to be promising to indeed foster our understanding of the ecosystem functioning of shelf seas and low lying coastal (wet)lands. Here, we demonstrate that the interdisciplinary collaboration among both terrestrial and marine hydro-physicists, biogeochemists and biologists gives new insight into the operating processes.
Our results show that either side of the coastline is impacted by the respective other ecosystem compartment. It has to be emphasized that the mutual influence is operational despite low gradients and a non-tidal system. Although a dune functioning as a dyke between sea and land is still in place, we found evidence for earlier flooding events. Biogeochemical processes such as the production of GHGs are still affected by saltwater influx, despite the intrusion probably dating back decades. Water flux-based exchange processes within the main peat body operate at small rates, while deeper groundwater fluxes and at the border of the peatland might be more relevant. Considering the land area along the Baltic Sea coast that is discharging directly into the sea (catchments unconnected to main draining rivers), we conclude that biogeochemical and resulting biological processes in the shallow sea might be regulated to a great extent by nutrient and complex organic matter fluxes originating from (peat) land.
In the future, rate measurements of in situ transformations in the carbon-sulfur cycles and dating of the different water sources will allow for a better mechanistic understanding of the actual processes and the flow of water as an important driver for element transport. A model-based analysis linked to physical properties of the land and sea side will allow depicting larger scale (1–10 km) water fluxes as a basis for balance calculations of nutrients and other relevant compounds, while bioenergetically based ecological models assessing the effects of the common environmental stressors on marine biota will complete the system view onto the coastal ecocline.
Author Contributions
GJ, MJ, BL, MV, MEB, MB, HB, SF, UK, PL, GR, HS, HS-V, and IS developed the idea and elaborated the concept. LG, SG-P, FH, MI, NK, MK, XL, KR, HAS, RS, VU, and JW provided experimental or numerical data and organized and conducted the data analyses together with UG, GM, TP, FR, and GJ. BL, GJ, MJ, and MV led the manuscript writing, with contributions from all authors.
Funding
This study was conducted within the framework of the Research Training Group Baltic TRANSCOAST funded by the DFG (Deutsche Forschungsgemeinschaft) under grant number GRK 2000/1. This is Baltic TRANSCOAST publication no. GRK2000/00XX.
Conflict of Interest Statement
The authors declare that the research was conducted in the absence of any commercial or financial relationships that could be construed as a potential conflict of interest.
Acknowledgments
MEB and JW wish to thank I. Schmiedinger, R. I. Liskow, C. Burmeister, R. Bahlo, and A. Köhler (IOW) for their invaluable biogeochemical and stable isotope geochemical and SEM-EDX laboratory support. H. Nikolai (ICBM Wilhelmshaven) is thanked for the expert support with the manufacturing and in-situ establishment of the pore water lances. U. Mallast, W. S. (Billy) Moore, N. Moosdorf, J. Scholten, and J. Sltenfuß are thanked for their continuous active support with Rn, Ra, and dating investigations. Figure 14 uses images from the following sources: Mya arenaria by J. A. Herklots, ‘De Weekdieren van Nederland’, https://commons.wikimedia.org/w/index.php?curid=1748721; Internet Archive Book Images, https://commons.wikimedia.org/w/index.php?curid=43541209.
Footnote
References
Abarca, E., Karam, H., Hemond, H. F., and Harvey, C. F. (2013). Transient groundwater dynamics in a coastal aquifer: the effects of tides, the lunar cycle, and the beach profile. Water Resour. Res. 49, 2473–2488. doi: 10.1002/wrcr.20075
Andersen, M. S., Baron, L., Gudbjerg, J., Gregersen, J., Chapellier, D., Jakobsen, R., et al. (2007). Discharge of nitrate-containing groundwater into a coastal marine environment. J. Hydrol. 336, 98–114. doi: 10.1016/j.jhydrol.2006.12.023
Aravena, R., and Wassenaar, L. I. (1993). Dissolved organic carbon and methane in a regional confined aquifer, southern Ontario, Canada: carbon isotope evidence for associated subsurface sources. Appl. Geochem. 8, 483–493. doi: 10.1016/0883-2927(93)90077-T
Armbrust, E. V., Berges, J. A., Bowler, C., Green, B. R., Martinez, D., Putnam, N. H., et al. (2004). The genome of the diatom Thalassiosira pseudonana: ecology, evolution, and metabolism. Science 306, 79–86. doi: 10.1126/science.1101156
BACC II Author Team (2015). Second Assessment of Climate Change for the Baltic Sea Basin. Regional Climate Studies. Berlin: Springer.
Bahnwart, M., Hübener, T., and Schubert, H. (1998). Downstream changes in phytoplankton composition and biomass in a lowland river-lake system (Warnow River, Germany). Hydrobiologia 391, 99–111. doi: 10.1023/A:1003558209411
Bartlett, K. B., Bartlett, D. S., Harriss, R. C., and Sebacher, D. I. (1987). Methane emissions along a salt marsh salinity gradient. Biogeochemistry 4, 183–202. doi: 10.1007/BF02187365
Beck, M., Dellwig, O., Kolditz, K., Freund, H., Liebezeit, G., Schnetger, B., et al. (2007). In situ pore water sampling in deep intertidal flat sediments. Limnol. Oceanogr. Methods 5, 136–144. doi: 10.4319/lom.2007.5.136
Boetius, A., Ravenschlag, K., Schubert, C. J., Rickert, D., Widdel, F., Gleseke, A., et al. (2000). A marine microbial consortium apparently mediating anaerobic oxidation methane. Nature 407, 623–626. doi: 10.1038/35036572
Bohne, B., and Bohne, K. (2008). “Monitoring zum Wasserhaushalt einer auf litoralem Versumpfungsmoor gewachsenen Regenmoorkalotte - Beispiel Naturschutzgebiet “Hütelmoor” bei Rostock,” in Aspekte der Geoökologie, ed. O. Stüdemann (Berlin: Weißensee Verlag), 313–338.
Bollmann, M., Bosch, T., Colijn, F., Ebinghaus, R., Froese, R., Güssow, K., et al. (2010). World Ocean Review: Living with the Oceans. Hamburg: Maribus GmbH.
Borges, A. V., Champenois, W., Gypens, N., Delille, B., and Harlay, J. (2016). Massive marine methane emissions from near-shore shallow coastal areas. Sci. Rep. 6:27908. doi: 10.1038/srep27908
Böttcher, M. E., Lipka, M., Winde, V., Dellwig, O., Böttcher, E. O., Böttcher, T. M. C., et al. (2014). “Multi-isotope composition of freshwater sources for the southern North and Baltic Sea,” in Proceedings of the 23rd SWIM Conference, Husum, 46–49.
Böttcher, M. E., Mallast, U., Massmann, G., Moosdorf, N., Müller-Petke, M., and Waska, H. (2018). “Coastal-Groundwater interfaces (submarine groundwater discharge),” in Ecohydrological Interfaces, ed. S. Krause (New York, NY: Wiley).
Burchard, H., and Bolding, K. (2002). GETM, A General Estuarine Transport Model. Scientific Documentation. Brussels: European Commission.
Burchard, H., Bolding, K., Feistel, R., Gräwe, U., MacCready, P., Klingbeil, K., et al. (2018). The Knudsen theorem and the Total Exchange Flow analysis framework applied to the Baltic Sea. Prog. Oceanogr. 165, 268–286. doi: 10.1016/j.pocean.2018.04.004
Burnett, W. C., Bokuniewicz, H., Huettel, M., Moore, W. S., and Taniguchi, M. (2003). Groundwater and pore water inputs into the coastal zone. Biogeochemistry 66, 3–33. doi: 10.1023/B:BIOG.0000006066.21240.53
Cahoon, L. B. (1999). The role of benthic microalgae in neritic ecosystems. Oceanogr. Mar. Biol. Annu. Rev. 37, 47–86.
Church, J. A., White, N. J., Domingues, C. M., Monselesan, D. P., and Miles, E. R. (2013). Sea level and ocean heat-content change. Int. Geophys. 103, 697–725. doi: 10.1016/B978-0-12-391851-2.00027-1
Church, T. M. (1996). A groundwater route for the water cycle. Nature 380, 579–580. doi: 10.1038/380579a0
Cline, J. D. (1969). Spectrophotometric determination of hydrogen sulfide in natural waters. Limnol. Oceanogr. 14, 454–458. doi: 10.4319/lo.1969.14.3.0454
de Beer, D., Wenzhöfer, F., Ferdelman, T. G., Boehme, S. E., Hüttel, M., van Beusekom, J. E. E., et al. (2005). Transport and mineralization in North Sea sandy intertidal sediments, Sylt-Rømø Basin, Wadden Sea. Limnol. Oceanogr. 50, 113–127. doi: 10.4319/lo.2005.50.1.0113
Deutsch, B., Mewes, M., Liskow, I., and Voss, M. (2006). Quantification of diffuse nitrate inputs into a small river system using stable isotopes of oxygen and nitrogen in nitrate. Org. Geochem. 37, 1333–1342. doi: 10.1016/j.orggeochem.2006.04.012
Doney, S. C. (2010). The growing human footprint on coastal and open-ocean biogeochemistry. Science 328, 1512–1516. doi: 10.1126/science.1185198
Donis, D., Janssen, F., Liu, B., Wenzhöfer, F., Dellwig, O., Escher, P., et al. (2017). Biogeochemical impact of submarine ground water discharge on coastal surface sands of the southern Baltic Sea. Estuar. Coast. Shelf Sci. 189, 131–142. doi: 10.1016/j.ecss.2017.03.003
Du Laing, G., Rinklebe, J., Vandecasteele, B., Meers, E., and Tack, F. M. (2009). Trace metal behaviour in estuarine and riverine floodplain soils and sediments: a review. Sci. Total Environ. 407, 3972–3985. doi: 10.1016/j.scitotenv.2008.07.025
Encarnação, J., Leitão, F., Range, P., Piló, D., Chícharo, M. A., and Chícharo, L. (2013). The influence of submarine groundwater discharges on subtidal meiofauna assemblages in south Portugal (Algarve). Estuar. Coast. Shelf Sci. 130, 202–208. doi: 10.1016/j.ecss.2013.04.013
Fernández-Fernández, L., Westphal, J., Schmiedinger, I., Kreuzburg, M., Bahlo, R., Koebsch, F., et al. (2017). Sulfur isotope biogeochemistry of soils from an episodically flooded coastal wetland, southern Baltic Sea. Geophys. Res. Abstr. 19:EGU2017-14335.
Forster, S., Bobertz, B., and Bohling, B. (2003). Permeability of sands in the coastal areas of the southern Baltic Sea: mapping a grain-size related sediment property. Aquat. Geochem. 9, 171–190. doi: 10.1023/B:AQUA.0000022953.52275.8b
Gao, H., Matyka, M., Liu, B., Khalili, A., Kostka, J. E., Collins, G., et al. (2012). Intensive and extensive nitrogen loss from intertidal permeable sediments of the Wadden Sea. Limnol. Oceanogr. 57, 185–198. doi: 10.1371/journal.pone.0104517
Gätje, C., and Reise, K. (2012). Ökosystem Wattenmeer - Austausch-, Transport- und Stoffumwandlungsprozesse / The Wadden Sea Ecosystem - Exchange, Transport and Transformation Processes. Berlin: Springer.
Geyer, W. R., and MacCready, P. (2014). The estuarine circulation. Annu. Rev. Fluid Mech. 46, 175–197. doi: 10.1146/annurev-fluid-010313-141302
Giani, L., Dittrich, K., Martsfeld-Hartmann, A., and Peters, G. (1996). Methanogenesis in saltmarsh soils of the North Sea coast of Germany. Eur. J. Soil Sci. 47, 175–182. doi: 10.1111/j.1365-2389.1996.tb01388.x
Glatzel, S., Koebsch, F., Beetz, S., Hahn, J., Richter, P., and Jurasinski, G. (2011). Maßnahmen zur minderung der treibhausgasfreisetzung aus mooren im mittleren mecklenburg. Telma Beih. 4, 85–106.
Gocke, K., Lenz, J., Westphal, H., Koppe, R., Rheinheimer, G., and Hoppe, H.-G. (2012). Diel variations in microbial activity in hypertrophic coastal waters. Hydrol. Wasserbewirtschaftung 56, 48–58.
Gosch, L., Janssen, M., and Lennartz, B. (2018). Impact of the water salinity on the hydraulic conductivity of fen peat. Hydrol. Process. 32, 1214–1222. doi: 10.1002/hyp.11478
Gräwe, U., and Burchard, H. (2012). Storm surges in the Western Baltic Sea: the present and a possible future. Clim. Dyn. 39, 165–183. doi: 10.1007/s00382-011-1185-z
Gräwe, U., Holtermann, P., Klingbeil, K., and Burchard, H. (2015). Advantages of vertically adaptive coordinates in numerical models of stratified shelf seas. Ocean Model. 92, 56–68. doi: 10.1016/j.ocemod.2015.05.008
Grinsted, A., Jevrejeva, S., Riva, R. E., and Dahl-Jensen, D. (2015). Sea level rise projections for northern Europe under RCP8. 5. Clim. Res. 64, 15–23. doi: 10.3354/cr01309
Günther, A., Schenzle, E., and Jurasinski, G. (2014). Flexible Chambers for Greenhouse Gas Measurements in Tall Vegetation - A Construction Manual. Available at: http://bit.ly/2GLLy8O
Hahn, J., Glatzel, S., Köhler, S., and Jurasinski, G. (2015). Greenhouse gas exchange in a coastal fen in the first year after flooding - a systems shift. PLoS One 10:e0140657. doi: 10.1371/journal.pone.0140657
Haider, F., Sokolov, E. P., and Sokolova, I. M. (2017). Effects of mechanical disturbance and salinity stress on bioenergetics and burrowing behavior of the soft shell clam Mya arenaria. J. Exp. Biol. 221(Pt 4):jeb172643. doi: 10.1242/jeb.172643
Harff, J., Furmanczyk, K., and von Storch, H. (2017). Coastline Changes of the Baltic Sea from South to East: Past and Future Projections. Berlin: Springer. doi: 10.1007/978-3-319-49894-2
Hartmann, M., and Nielsen, H. (2012). δ34S values in recent sea sediments and their significance using several sediment profiles from the western Baltic Sea. Isotopes Environ. Health Stud. 48, 7–32. doi: 10.1080/10256016.2012.660528
Hellebust, J. A., and Lewin, J. (1977). “Heterotrophic nutrition,” in The Biology of Diatoms, ed. D. Werner (Berkeley, CA: University of California Press), 169–197.
Huettel, M., and Gust, G. (1992). Solute release mechanisms from confined sediment cores in stirred benthic chambers and flume flows. Mar. Ecol. Prog. Ser. 82, 187–197. doi: 10.3354/meps082187
Huettel, M., Røy, H., Precht, E., and Ehrenhauss, S. (2003). Hydrodynamical impact on biogeochemical processes in aquatic sediments. Hydrobiologia 494, 231–236. doi: 10.1023/A:1025426601773
Huettel, M., Ziebis, W., Forster, S., and Luther, G. W. (1998). Advective transport affecting metal and nutrient distributions and interfacial fluxes in permeable sediments. Geochim. Cosmochim. Acta 62, 613–631. doi: 10.1016/S0016-7037(97)00371-2
Hünicke, B., and Zorita, E. (2016). Statistical analysis of the acceleration of Baltic mean sea-level rise, 1900-2012. Front. Mar. Sci. 3:125. doi: 10.3389/fmars.2016.00125
Jeandel, C. (2016). Overview of the mechanisms that could explain the ’Boundary Exchange’ at the land-ocean contact. Philos. Trans. R. Soc. A Math. Phys. Eng. Sci. 374:20150287. doi: 10.1098/rsta.2015.0287
Johansson, M. M., Pellikka, H., Kahma, K. K., and Ruosteenoja, K. (2014). Global sea level rise scenarios adapted to the Finnish coast. J. Mar. Syst. 129, 35–46. doi: 10.1016/j.jmarsys.2012.08.007
Jørgensen, B. B., and Kasten, S. (2006). “Sulfur cycling and methane oxidation,” in Marine Geochemistry, eds H. D. Schulz and M. Zabel (Berlin: Springer), 271–309.
Kemp, W. M., Boynton, W. R., Adolf, J. E., Boesch, D. F., Boicourt, W. C., Brush, G., et al. (2005). Eutrophication of Chesapeake Bay: historical trends and ecological interactions. Mar. Ecol. Prog. Ser. 303, 1–29. doi: 10.3354/meps303001
Kitano, M., Matsukawa, R., and Karube, I. (1997). Changes in eicosapentaenoic acid content of Navicula saprophila, Rhodomonas salina and Nitzschia sp. under mixotrophic conditions. J. Appl. Phycol. 9, 559–563.
Knee, K. L., and Paytan, A. (2011). “Submarine groundwater discharge: a source of nutrients, metals, and pollutants to the coastal ocean,” in Treatise on Estuarine and Coastal Science 4, eds E. Wolanski and D. S. McLusky (London: Academic Press), 205–233.
Knittel, K., and Boetius, A. (2009). Anaerobic oxidation of methane: progress with an unknown process. Annu. Rev. Microbiol. 63, 311–334. doi: 10.1146/annurev.micro.61.080706.093130
Koch, M., Koebsch, F., Hahn, J., and Jurasinski, G. (2017). From meadow to shallow lake: monitoring secondary succession in a coastal fen after rewetting by flooding based on aerial imagery and plot data. Mires Peat 19:11. doi: 10.19189/MaP.2015.OMB.188
Koebsch, F., Glatzel, S., and Jurasinski, G. (2013). Vegetation controls methane emissions in a coastal brackish fen. Wetl. Ecol. Manag. 21, 323–337. doi: 10.1007/s11273-013-9304-8
Koebsch, F., Jurasinski, G., Koch, M., Hofmann, J., and Glatzel, S. (2015). Controls for multi-scale temporal variation in ecosystem methane exchange during the growing season of a permanently inundated fen. Agric. For. Meteorol. 204, 94–105. doi: 10.1016/j.agrformet.2015.02.002
Kotwicki, L., Grzelak, K., Czub, M., Dellwig, O., Gentz, T., Szymczycha, B., et al. (2014). Submarine groundwater discharge to the Baltic coastal zone: impacts on the meiofaunal community. J. Mar. Syst. 129, 118–126. doi: 10.1016/j.jmarsys.2013.06.009
Kreuzburg, M., Ibenthal, M., Janssen, M., Rehder, G., Voss, M., Naumann, M., et al. (2018). Submarine continuation of peat deposits from a coastal peatland in the southern Baltic Sea and its Holocene development. Front. Earth Sci. 6:103. doi: 10.3389/feart.2018.00103
Kummu, M., De Moel, H., Salvucci, G., Viviroli, D., Ward, P. J., and Varis, O. (2016). Over the hills and further away from coast: global geospatial patterns of human and environment over the 20th-21st centuries. Environ. Res. Lett. 11:034010. doi: 10.1088/1748-9326/11/3/034010
Kwon, E. Y., Kim, G., Primeau, F., Moore, W. S., Cho, H.-M., Devries, T., et al. (2014). Global estimate of submarine groundwater discharge based on an observationally constrained radium isotope model. Geophys. Res. Lett. 41, 8438–8444. doi: 10.1002/2014GL061574
Lardies, M. A., Clasing, E., Navarro, J. M., and Stead, R. A. (2001). Effects of environmental variables on burial depth of two infaunal bivalves inhabiting a tidal flat in southern Chile. J. Mar. Biol. Assoc. U.K. 81, 809–816. doi: 10.1017/S0025315401004635
Leinweber, P., Jandl, G., Eckhardt, K.-U., Schlichting, A., Hofmann, D., and Schulten, H.-R. (2009). “Analytical pyrolysis and soft-ionization mass spectrometry,” in Biophysico-Chemical Processes Involving Natural Nonliving Organic Matter in Environmental Systems, eds N. Senesi, B. Xing, and P. M. Huang (Hoboken, NJ: John Wiley & Sons), 539–588. doi: 10.1002/9780470494950.ch14
Leitão, F., Encarnação, J., Range, P., Schmelz, R. M., Teodósio, M. A., and Chícharo, L. (2015). Submarine groundwater discharges create unique benthic communities in a coastal sandy marine environment. Estuar. Coast. Shelf Sci. 163, 93–98. doi: 10.1016/j.ecss.2015.06.007
Liu, D. Y., Ding, W. X., Jia, Z. J., and Cai, Z. C. (2011). Relation between methanogenic archaea and methane production potential in selected natural wetland ecosystems across China. Biogeosciences 8, 329–338. doi: 10.5194/bg-8-329-2011
Liu, J., Su, N., Wang, X., and Du, J. (2017). Submarine groundwater discharge and associated nutrient fluxes into the Southern Yellow Sea: a case study for semi-enclosed and oligotrophic seas - implication for green tide bloom. J. Geophys. Res. Oceans 122, 139–152. doi: 10.1002/2016JC012282
Martin, J. M., and Whitfield, M. (1983). “The significance of the river input of chemical elements to the ocean,” in Trace Metals in Sea Water, ed. C. Wong (Boston, MA: Springer), 265–296.
Massel, S. R. (2001). Circulation of groundwater due to wave set-up on a permeable beach. Oceanologia 43, 279–290.
Meyercordt, J., and Meyer-Reil, L. A. (1999). Primary production of benthic microalgae in two shallow coastal lagoons of different trophic status in the southern Baltic Sea. Mar. Ecol. Prog. Ser. 178, 179–191. doi: 10.3354/meps178179
Miegel, M., Graeff, T., Selle, B., Salzmann, T., Franck, C., and Bronstert, A. (2016). Untersuchung eines renaturierten Niedermoores an der mecklenburgischen Ostseeküste - Teil I: systembeschreibung und hydrologische Grundcharakterisierung (Investigation of a renatured fen on the Baltic Sea coast of Mecklenburg - Part I: system description and basic hydrological characterisation). Hydrol. Wasserbewirtschaftung 60, 242–258.
Missiaen, T., Murphy, S., Loncke, L., and Hernriet, J.-P. (2002). Very high-resolution seismic mapping of shallow gas in the Belgian coastal zone. Cont. Shelf Res. 22, 2291–2301. doi: 10.1016/S0278-4343(02)00056-0
Moghimi, S., Klingbeil, K., Gräwe, U., and Burchard, H. (2013). A direct comparison of a depth-dependent radiation stress formulation and a vortex force formulation within a three-dimensional coastal ocean model. Ocean Model. 70, 132–144. doi: 10.1016/j.ocemod.2012.10.002
Moore, W. S., Beck, M., Riedel, T., Rutgers van der Loeff, M., Dellwig, O., Shaw, T. J., et al. (2011). Radium-based pore water fluxes of silica, alkalinity, manganese, DOC, and uranium: a decade of studies in the German Wadden Sea. Geochim. Cosmochim. Acta 75, 6535–6555. doi: 10.1016/j.gca.2011.08.037
Nerem, R. S., Beckley, B. D., Fasullo, J. T., Hamlington, B. D., Masters, D., and Mitchum, G. T. (2018). Climate-change-driven accelerated sea-level rise detected in the altimeter era. Proc. Natl. Acad. Sci. U.S.A. 115, 2022–2025. doi: 10.1073/pnas.1717312115
Neumann, B., Vafeidis, A. T., Zimmermann, J., and Nicholls, R. J. (2015). Future coastal population growth and exposure to sea-level rise and coastal flooding-a global assessment. PLoS One 10:e0118571. doi: 10.1371/journal.pone.0118571
Newton, A., Icely, J., Cristina, S., Brito, A., Cardoso, A. C., Colijn, F., et al. (2014). An overview of ecological status, vulnerability and future perspectives of European large shallow, semi-enclosed coastal systems, lagoons and transitional waters. Estuar. Coast. Shelf Sci. 140, 95–122. doi: 10.1016/j.ecss.2013.05.023
Nicholls, R. J., Wong, P. P., Burkett, V. R., Codignotto, J. O., Hay, J. E., McLean, R. F., et al. (2007). “Coastal systems and low-lying areas. Climate change 2007: impacts, adaptation and vulnerability,” in Contribution of Working Group II to the Fourth Assessment Report of the Intergovernmental Panel on Climate Change, eds M. L. Parry, O. F. Canziani, J. P. Palutikof, P. J. van der Linden, and C. E. Hanson (Cambridge: Cambridge University Press), 315–356.
Ours, D. P., Siegel, D. I., and Glaser, P. H. (1997). Chemical dilation and the dual porosity of humified bog peat. J. Hydrol. 196, 348–360. doi: 10.1016/S0022-1694(96)03247-7
Piekarek-Jankowska, H. (1996). Hydrochemical effects of submarine groundwater discharge to the Puck Bay. Geogr. Pol. 67, 103–120.
Przeslawski, R., Zhu, Q., and Aller, R. (2009). Effects of abiotic stressors on infaunal burrowing and associated sediment characteristics. Mar. Ecol. Prog. Ser. 392, 33–42. doi: 10.3354/meps08221
Reckhardt, A., Beck, M., Greskowiak, J., Schnetger, B., Böttcher, M. E., Gehre, M., et al. (2017). Cycling of redox-sensitive elements in a sandy subterranean estuary of the southern North Sea. Mar. Chem. 188, 6–17. doi: 10.1016/j.marchem.2016.11.003
Rezanezhad, F., Price, J. S., Quinton, W. L., Lennartz, B., Milojevic, T., and Van Cappellen, P. (2016). Structure of peat soils and implications for water storage, flow and solute transport: a review update for geochemists. Chem. Geol. 429, 75–84. doi: 10.1016/j.chemgeo.2016.03.010
Rezanezhad, F., Quinton, W. L., Price, J. S., Elliot, T. R., Elrick, D., and Shook, K. R. (2010). Influence of pore size and geometry on peat unsaturated hydraulic conductivity computed from 3D computed tomography image analysis. Hydrol. Process. 24, 2983–2994. doi: 10.1002/hyp.7709
Rullkötter, J. (2009). The back-barrier tidal flats in the southern North Sea—a multidisciplinary approach to reveal the main driving forces shaping the system. Ocean Dyn. 59, 157–165. doi: 10.1007/s10236-009-0197-2
Schiewer, U., and Schubert, H. (2004). Carbon budget and pelagic community compositions at two coastal areas that differ in their degree of eutrophication, in the Southern Baltic Sea. Estuar. Coast. Shelf Sci. 61, 89–100. doi: 10.1016/j.ecss.2004.04.006
Schories, D., Kuhlenkamp, R., Schubert, H., and Selig, U. (2014). Rote Liste und Gesamtartenliste der marinen Makroalgen (Chlorophyta, Phaeophyceae et Rhodophyta) Deutschlands. Nat. Biol. Vielfalt 70, 179–229.
Schubert, H., Feuerpfeil, P., Marquardt, R., Telesh, I. V., and Skarlato, S. O. (2011). Macroalgal diversity along the Baltic Sea salinity gradient challenges Remane’s species-minimum concept. Mar. Pollut. Bull. 62, 1948–1956. doi: 10.1016/j.marpolbul.2011.06.033
Scully, M. E. (2010). Wind modulation of dissolved oxygen in Chesapeake Bay. Estuaries Coast. 33, 1164–1175. doi: 10.1007/s12237-010-9319-9
Selle, B., Graeff, T., Salzmann, T., Oswald, S. E., Walther, M., and Miegel, K. (2016). Untersuchung eines renaturierten Niedermoores an der mecklenburgischen Ostseeküste - Teil II: salzdynamik und Wasserhaushalt (Investigation of a renatured fen catchment on the Baltic Sea coast of Mecklenburg - Part II: salt dynamics and water balance). Hydrol. Wasserbewirtschaftung 60, 259–268.
Smyth, C., Hay, A. E., and Zedel, L. (2002). Coherent Doppler profiler measurements of near-bed suspended sediment fluxes and the influence of bed forms. J. Geophys. Res. Oceans 107, 19-1–19-20. doi: 10.1029/2000JC000760
Sokolova, I. M. (2013). Energy-limited tolerance to stress as a conceptual framework to integrate the effects of multiple stressors. Integr. Compar. Biol. 53, 597–608. doi: 10.1093/icb/ict028
Sokolova, I. M., Frederich, M., Bagwe, R., Lannig, G., and Sukhotin, A. A. (2012). Energy homeostasis as an integrative tool for assessing limits of environmental stress tolerance in aquatic invertebrates. Mar. Environ. Res. 79, 1–15. doi: 10.1016/j.marenvres.2012.04.003
Soomere, T., and Pindsoo, K. (2016). Spatial variability in the trends in extreme storm surges and weekly-scale high water levels in the eastern Baltic Sea. Cont. Shelf Res. 115, 53–64. doi: 10.1016/j.csr.2015.12.016
Stachura-Suchoples, K., Enke, N., Schlie, C., Schaub, I., Karsten, U., and Jahn, R. (2016). Contribution towards a molecular taxonomic reference library of Arctic benthic marine diatoms from Kongsfjorden. Polar Biol. 39, 1933–1956. doi: 10.1007/s00300-015-1683-2
STALU-MM (2010). Regelwerk Küstenschutz Mecklenburg-Vorpommern, 2-1 Küstenlängen in Mecklenburg-Vorpommern. Staatliches Amt für Landwirtschaft und Umwelt Mittleres Mecklenburg url. Available at: http://service.mvnet.de/_php/download.php?datei_id=155707
Tauber, F. (2012). “Meeresbodensedimente in der deutschen Ostsee: Mecklenburger Bucht - Darß, Karte Nr. 2934,” in Maßstab 1 : 100 000, ed. Bundesamt für Seeschifffahrt und Hydrographie (Hamburg: Bundesamt für Seeschifffahrt und Hydrographie).
Telesh, I., Schubert, H., and Skarlato, S. (2013). Life in the salinity gradient: discovering mechanisms behind a new biodiversity pattern. Estuar. Coast. Shelf Sci. 135C, 317–327. doi: 10.1016/j.ecss.2013.10.013
Tiemeyer, B., Albiac Borraz, E., Augustin, J., Bechtold, M., Beetz, S., Beyer, C., et al. (2016). High emissions of greenhouse gases from grasslands on peat and other organic soils. Glob. Change Biol. 22, 4134–4149. doi: 10.1111/gcb.13303
Turner, R. L., and Meyer, C. E. (1980). Salinity tolerance of the brackish-water echinoderm Ophiophragmus filograneus (Ophiuroidea). Mar. Ecol. Prog. Ser. 2, 249–256. doi: 10.3354/meps002249
Umlauf, L., and Burchard, H. (2005). Second-order turbulence closure models for geophysical boundary layers. A review of recent work. Cont. Shelf Res. 25, 795–827. doi: 10.1016/j.csr.2004.08.004
van der Meer, J. (2006). Metabolic theories in ecology. Trends Ecol. Evol. 21, 136–140. doi: 10.1016/j.tree.2005.11.004
van Diggelen, R., Middleton, B., Bakker, J., Grootjans, A., and Wassen, M. (2006). Fens and floodplains of the temperate zone: present status, threats, conservation and restoration. Appl. Veg. Sci. 9, 157–162. doi: 10.1111/j.1654-109X.2006.tb00664.x
Vasander, H., Tuittila, E.-S., Lode, E., Lundin, L., Ilomets, M., Sallantaus, T., et al. (2003). Status and restoration of peatlands in northern Europe. Wetl. Ecol. Manag. 11, 51–63. doi: 10.1023/A:1022061622602
Volkmann, C., Halbedel, S., Voss, M., and Schubert, H. (2016). The role of dissolved organic and inorganic nitrogen for growth of macrophytes in coastal waters of the Baltic Sea. J. Exp. Mar. Biol. 477, 23–30. doi: 10.1016/j.jembe.2016.01.005
Vousdoukas, M. I., Voukouvalas, E., Annunziato, A., Giardino, A., and Feyen, L. (2016). Projections of extreme storm surge levels along Europe. Clim. Dyn. 47, 3171–3190. doi: 10.1007/s00382-016-3019-5
Wahl, M., Jormalainen, V., Eriksson, B. K., Coyer, J. A., Molis, M., Schubert, H., et al. (2011). Stress ecology in Fucus: abiotic, biotic and genetic interactions. Adv. Mar. Biol. 59, 37–105. doi: 10.1016/B978-0-12-385536-7.00002-9
Weisse, R., Bellafiore, D., Menéndez, M., Méndez, F., Nicholls, R. J., Umgiesser, G., et al. (2014). Changing extreme sea levels along European coasts. Coast. Eng. 87, 4–14. doi: 10.1016/j.coastaleng.2013.10.017
Winde, V., Böttcher, M. E., Escher, P., Böning, P., Beck, M., Liebezeit, G., et al. (2014). Tidal and spatial variations of DI13C and aquatic chemistry in a temperate tidal basin during winter time. J. Mar. Syst. 129, 396–404. doi: 10.1016/j.jmarsys.2013.08.005
Keywords: shallow coast, coastal peatland, land–sea coupling, greenhouse gas emissions, submarine groundwater discharge
Citation: Jurasinski G, Janssen M, Voss M, Böttcher ME, Brede M, Burchard H, Forster S, Gosch L, Gräwe U, Gründling-Pfaff S, Haider F, Ibenthal M, Karow N, Karsten U, Kreuzburg M, Lange X, Leinweber P, Massmann G, Ptak T, Rezanezhad F, Rehder G, Romoth K, Schade H, Schubert H, Schulz-Vogt H, Sokolova IM, Strehse R, Unger V, Westphal J and Lennartz B (2018) Understanding the Coastal Ecocline: Assessing Sea–Land Interactions at Non-tidal, Low-Lying Coasts Through Interdisciplinary Research. Front. Mar. Sci. 5:342. doi: 10.3389/fmars.2018.00342
Received: 30 April 2018; Accepted: 06 September 2018;
Published: 26 September 2018.
Edited by:
Anas Ghadouani, The University of Western Australia, AustraliaReviewed by:
Sabine Schmidt, Centre National de la Recherche Scientifique (CNRS), FranceHelena Granja, University of Minho, Portugal
Copyright © 2018 Jurasinski, Janssen, Voss, Böttcher, Brede, Burchard, Forster, Gosch, Gräwe, Gründling-Pfaff, Haider, Ibenthal, Karow, Karsten, Kreuzburg, Lange, Leinweber, Massmann, Ptak, Rezanezhad, Rehder, Romoth, Schade, Schubert, Schulz-Vogt, Sokolova, Strehse, Unger, Westphal and Lennartz. This is an open-access article distributed under the terms of the Creative Commons Attribution License (CC BY). The use, distribution or reproduction in other forums is permitted, provided the original author(s) and the copyright owner(s) are credited and that the original publication in this journal is cited, in accordance with accepted academic practice. No use, distribution or reproduction is permitted which does not comply with these terms.
*Correspondence: Bernd Lennartz, YmVybmQubGVubmFydHpAdW5pLXJvc3RvY2suZGU=
†These authors have contributed equally to this work