- 1Marine Biological Association, Plymouth, United Kingdom
- 2School of Ocean and Earth Science, University of Southampton, Southampton, United Kingdom
- 3Biosciences, College of Life and Environmental Sciences, University of Exeter, Exeter, United Kingdom
- 4Department of Biology and Marine Biology, University of North Carolina Wilmington, Wilmington, NC, United States
Coccolithophores are globally abundant marine microalgae characterized by their ability to form calcite platelets (coccoliths). The coccoliths are produced internally in a Golgi-derived vesicle. Mature coccoliths are extruded from the cell to form a protective covering on the cell surface, known as the coccosphere. Current evidence indicates that calcite precipitation in the coccolith vesicle (CV) is modulated by coccolith-associated polysaccharides (CAPs). Whilst previous research into CAPs has focussed on their roles in calcite precipitation within the CV, little is known of their extracellular roles. Using fluorescent lectins, we visualize the extracellular polysaccharide-rich organic layer associated with external coccoliths and demonstrate that it differs between species in structure and composition. Biochemical analysis of polysaccharide extracted from coccoliths indicated substantial differences between species in monosaccharide composition and uronic acid content. In Coccolithus braarudii our studies indicate that polysaccharide-rich material is extruded with the coccoliths, where it plays a role in the adhesion of the coccoliths to the cell surface and contributes to the overall organization of the coccosphere. Together, these results highlight the important extracellular roles of CAPs and their contribution to the dynamic nature of the coccosphere.
Introduction
Coccolithophores are photosynthetic unicellular marine algae that are characterized by their ability to form intricate calcite platelets known as coccoliths (Taylor et al., 2017). Coccoliths are produce internally in a Golgi-derived coccolith vesicle (CV). Coccolithophores transport Ca2+ and from the environment into the CV where the precipitation of calcite occurs (Brownlee and Taylor, 2004). Mature coccoliths are extruded and organized into an extracellular layer (the coccosphere) covering the cell surface (Young and Henriksen, 2003; Brownlee and Taylor, 2004). Coccolithophores are globally abundant, with some species forming vast blooms that can be visible from space (Westbroek et al., 1993), making them important global producers and significant contributors to the ocean carbon cycle (Rost and Riebesell, 2004). The important role coccolithophores play in biogeochemical cycling has driven much research investigating the underlying cellular mechanisms of calcification and the roles of the extracellular covering of coccoliths.
Coccolith-associated polysaccharides (CAPs) are thought to play an important role in the nucleation and shaping of the calcite crystals (Borman et al., 1982; Marsh, 2003). There is considerable variability in the morphology of coccoliths and the nature of the coccosphere between species. For example, the heavily-calcified placolith-bearing species such as Coccolithus braarudii or Calcidiscus leptoporus, which contribute significantly to calcite production in the Atlantic Ocean, exhibit a single layer of interlocking coccoliths (Young et al., 2003; Daniels et al., 2014). The cosmopolitan bloom forming species Emiliania huxleyi also produces placoliths, but these can be arranged into either single or multiple layers within its coccosphere (Paasche, 2001). Scyphosphaera apsteinii is broadly distributed in tropical and sub-tropical waters and produces a dimorphic coccosphere, comprising of flat muroliths and barrel-shaped lopadoliths (Drescher et al., 2012). These form a single layer on the cell surface, but are not interlocking (Young et al., 2003). The proportion of muroliths and lopadoliths in the coccosphere of S. apsteinii can vary due to environmental conditions, with a greater proportion of lopadoliths observed in cells grown at low light intensities (Drescher et al., 2012). The coccosphere of all species likely forms a protective covering around the cell (Monteiro et al., 2016), which must be both flexible (to enable cell growth and division) but also tightly organized to ensure full covering of the cell surface and to prevent excess shedding of coccoliths.
The mechanisms supporting the arrangement of coccoliths on the cell surface and their tethering to the plasma membrane have not been closely investigated. There are several reports describing an organic layer surrounding the cell, which is integrated with the coccoliths and comprised of several distinct components (van der Wal et al., 1983a,b; Marsh, 1994; Taylor et al., 2007). In the majority of coccolithophores, a layer of organic scales composed of cellulose or similar polysaccharide can be observed underneath the coccosphere (Drescher et al., 2012; Taylor et al., 2017), that are secured to the plasma membrane via distinct fibrillar material. A few coccolithophore species, most notably E. huxleyi, lack this layer of organic scales (van der Wal et al., 1983b). The organic scales are produced intracellularly and are secreted to the surface (Outka and Williams, 1971). Whilst these scales cover the majority of the cell surface, they are not found directly beneath the central region of the coccolith baseplate. Instead, the fibrillar material can be observed in direct contact with the mineralized coccolith in these regions, which is thought to play a role in tethering the coccolith to the plasma membrane (Drescher et al., 2012; Taylor et al., 2017). The organic scales may be bound by amorphous polysaccharide (Pienaar, 1994). TEM imaging in combination with ruthenium red staining to reveal polysaccharides identified a layer of amorphous polysaccharide coating the coccoliths of S. apsteinii, as well as heavy staining of organic scales and fibrillar material (Drescher et al., 2012). However, the nature of these polysaccharides and their roles in different coccolithophores with distinct coccolith morphologies are not known.
While there has been considerable interest in the role of polysaccharides in modulating calcite precipitation (Westbroek et al., 1973; De Jong et al., 1976), little is known about the extracellular CAPs in coccolithophores. CAPs are predominantly classified as water-soluble acidic polysaccharides primarily composed of neutral monosaccharides, acidic sulfate esters and uronic acid residues (De Jong et al., 1976). The uronic acid residues are pivotal in modulating calcification because their negatively charged carboxyl groups are known to bind to Ca2+ cations and are thought to impede calcite precipitation at key points in coccolith production. Studies both in vivo and in vitro have demonstrated that CAPs regulate the precipitation of calcite in various species of coccolithophore (Borman et al., 1982, 1987; Henriksen and Stipp, 2009; Kayano et al., 2011; Gal et al., 2016).
Localization studies have shown polysaccharide situated internally in the CV in E. huxleyi (van der Wal et al., 1983b) and Chrysotila carterae (formerly Pleurochrysis carterae) (van der Wal et al., 1983a; Marsh, 1994). It is also clear that extracellular coccoliths are coated with an organic layer that may have an important influence on the dissolution of coccoliths (Young et al., 1999; Engel et al., 2004; Hirokawa et al., 2005). It is likely that polysaccharides contribute significantly to this organic layer (Hirokawa et al., 2005), but their origin and potential roles in relation to the extruded coccolith have not been extensively investigated.
The CAPs that have been isolated so far from coccolithophores show diversity between species in both their biochemical composition and in the number of isoforms present. For example, a single CAP was isolated from E. huxleyi (Borman et al., 1987) whereas three distinct forms were identified in C. carterae (Marsh, 2003). It has been proposed that the additional polysaccharides in C. carterae may function as a component of a Ca2+ delivery system, possibly replacing the function of the reticular body, a membrane-rich organelle that is not found in Chrysotila species (Marsh and Dickinson, 1997), but is a predominant feature in other species such as E. huxleyi and C. braarudii (Taylor et al., 2007, 2017). Moreover, the chemical composition of CAPs differs between species and strains (Borman et al., 1987; Lee et al., 2016). Significant differences in the uronic acid content of CAPs have been proposed to influence the shaping of the calcite crystals (Marsh and Dickinson, 1997) or to reflect adaptations to differing calcite saturation states of the CV (Lee et al., 2016).
A degree of ambiguity surrounds the nature and roles of CAPs due to the aforementioned diversity between species and because many investigations use different polysaccharide extraction procedures (Supplementary Table 1). Although CAPs are often referred to collectively, the varied extraction techniques used will not always differentiate between polysaccharides from the CV and those found extracellularly. Note that intracoccolith extractions have previously been referred to as intracrystalline (Westbroek et al., 1973; Lee et al., 2016), but as the localization of polysaccharides internally in calcite crystals is subject to debate we shall refer to these fractions as intracoccolith in this discussion. Previous investigations into CAPs have predominately focussed on the water-soluble components, although stable water-insoluble polysaccharides are also associated with the coccoliths (van der Wal et al., 1983b). The nature of the polysaccharides in the organic layer surrounding the cell has not been explored in detail. This layer appears to play an important role in the organization of the coccosphere, but whether these polysaccharides are related to those characterized in previous analyses of CAPs is not clear.
We have recently demonstrated that polysaccharides can be visualized around the coccolithophore C. braarudii using fluorescent dyes conjugated to lectins (Walker et al., 2018). In the present study, we use fluorescence microscopy to examine the extracellular polysaccharides associated with coccoliths in five coccolithophore species. We show that there are significant differences in the nature of the insoluble polysaccharides associated with coccoliths between species. Biochemical analyses suggest that these polysaccharides also differ in monosaccharide and uronic acid content. We show that the extracellular polysaccharides of C. braarudii are likely produced internally with the coccolith and play a subsequent role in the adhesion and organization of the coccosphere.
Materials and Methods
General Culture Conditions
Coccolithus braarudii (PLY182g) (formerly Coccolithus pelagicus ssp. braarudii), C. leptoporus (RCC1130), C. carterae (PLY406), and E. huxleyi (CCMP1516) were grown in filtered seawater (FSW) with added f/2 nutrients (Guillard and Ryther, 1962). S. apsteinii (RCC 1456) was grown in FSW with added f/2 nutrients and 10% K medium. Cells were grown in batch cultures, incubated at 15°C and illuminated with 65–75 μmol photons m-2 s-1 in a 16:8 light:dark cycle.
Decalcification of Coccolithophores
Coccolithophores were allowed to settle and excess f/2 media removed. To decalcify, the cells were washed in Harrison’s broad spectrum artificial seawater (ASW) (Harrison et al., 1980) without CaCl2 and pH adjusted to 7.0 with HCl. Cells were washed twice to remove any residual Ca2+ and adjust the pH, with time allowed for cells to settle between washes. Cells were incubated in the ASW for approximately 30 min to decalcify, allowed to settle and finally re-suspended in FSW f/2 media prior to staining.
Staining and Confocal Microscopy
Extracellular polysaccharides were stained using the fluorescent lectins Concanavalin A (ConA) (100 μg/ml) and wheat germ agglutinin (WGA) (100 μg/ml) conjugated to either fluorescein isothiocyanate (FITC) or Texas Red (all lectins: Invitrogen, United Kingdom). Cells were imaged using a Zeiss LSM 510 laser scanning confocal microscope, with excitation at 488 nm and emission at 500–530 nm (FITC) and 650–715 nm (chlorophyll). Where stated, certain samples were also visualized using a Nikon Ti epifluorescent microscope with a Photometrics Evolve EM-CCD camera (excitation 475–495 nm, emission 505–535 nm).
Electron Microscopy
Samples for SEM were filtered onto a 13 mm 0.4 μm Isopore filter (Millipore EMD) and rinsed with 5 ml of 1 mM HEPES buffered (pH 8.2) MilliQ water to remove any salt. Filters were air dried, mounted onto aluminum stubs and sputter coated with 10 nm Pt/Pd (Cressington, United States). Samples were examined using a Phillips XL30S FEG SEM (FEI-Phillips, United States) and imaged in high-resolution secondary electron mode with beam acceleration of 5 kV.
Coccolith Preparation
Late exponential phase cultures were harvested by centrifugation at 4800 g, 4°C for 5 min. Cells were resuspended in 10 ml of f/2 FSW and subjected to probe sonication (Soncs Vibra Cell VCX750) at 30% amplification for two 10 s pulses. Cells were mixed by inverting the tube between pulses. The cell debris and coccoliths were pelleted by centrifugation (4800 g, 4°C for 5 min) and the supernatant removed. The pellet was resuspended in 50% Percoll® (Sigma-Aldrich, United Kingdom) to separate the cell debris and coccoliths by density centrifugation. The mixture was centrifuged at 4800 g, at 4°C for 20 min. The density centrifugation step was repeated twice to ensure a clean coccolith preparation. The Percoll® was then removed from the coccoliths by washing three times in NH4HCO3 0.5 M. The coccolith preparation was then subjected to polysaccharide extraction.
A sample of the coccolith preparation was cleaned by incubating in 10% NaClO at room temperature overnight to remove all organic material. The coccoliths were pelleted and the supernatant removed. The cleaned calcite was then stained using FITC-ConA to test if the lectin bound non-specifically to the coccoliths.
Polysaccharide Extraction and GC–MS Analysis
The composition of polysaccharides extracted from coccolith preparations for C. braarudii, S. apsteinii, C. carterae, and E. huxleyi was determined using GC–MS. Calcite and other soluble material was removed by resuspending the coccolith pellets in 0.1 M EDTA (pH 8.0). The mixture was then centrifuged at 16000 g for 5 min and the supernatant removed. This step was repeated twice. Insoluble polysaccharides in this pellet were further cleaned by washing in 80% ethanol three times (centrifugation at 16000 g for 5 min).
Polysaccharides were hydrolyzed by heating to 105°C for 2 h in the presence of 2 M trifluoroacetic acid (200 μl). 50 μl of hydrolyzed sample was centrifuged at 16000 g for 10 min to remove any solid material. 40 μl of supernatant was transferred to glass MS vials and 10 μl of internal standard added (myo-inositol, final concentration 2 μM). Samples were air dried and 20 μl of 20 mg ml-1 methoxyamine hydrochloride dissolved in pyridine was added to each sample before incubation at 37°C for 2 h. 35 μl of N-methyl-N-trimethylsilyltrifluoroacetamide (MSTFA) was then added and the samples returned to 37°C for a further 30 min. Derivatising agents were purchased from Sigma-Aldrich, United Kingdom.
Blanks (TFA) and sugar standards were treated in the same way, along with a derivatization agent only blank. A standard mix was prepared containing D-glucose, D-galactose, D-mannose, D-xylose, D-arabinose, L-fucose, L-rhamnose, D-galacturonic acid, D-glucuronic acid, and myo-inositol.
Derivatised samples were analyzed using an Agilent 7200 series accurate mass Q-TOF GC–MS together with a 7890A GC system (Agilent Technologies, Santa Clara, CA, United States), equipped with an electron ionization ion source. 5 μl of each sample was injected into a non-deactivated, baffled glass liner with a 12:1 split ratio (14.448 ml min-1 split flow) and the inlet temperature was maintained at 250°C. A 3 ml min-1 septum purge flow was applied. A Zebron semi-volatiles (Phenomenex, Torrance, CA, United States) column (30 m × 250 μm × 0.25 μm) coupled with a 10 m guard column, was maintained at a constant helium flow of 1.2 ml min-1. The temperature gradient of the GC was ramped up at a rate of 15°C min-1, from 70 to 310°C over 16 min, and then held at 310°C for a further 6 min. The total run time of 22 min, was followed by a 7 min backflush at 310°C to clean the column at the end of every run. The MS emission current and emission voltage were held at 35 μA and 70 eV respectively, and the MS was automatically calibrated after every run. The mass range was set from 50 to 600 amu, with an acquisition rate of 5 spectra s-1, and a solvent delay of 3.5 min. Extraction solvent blanks were run at the beginning and end of the run.
Data were analyzed using Agilent technologies MassHunter qualitative and quantitative software. Peak areas were normalized for the internal standard and then peak areas for each monosaccharide residue was then individually normalized to the internal its standard for each sample. Normalized peak areas were then divided by that of the corresponding compound standard (to negate differences in ionization). Data are expressed as the percentage for each compound of the total peak areas of positively identified monosaccharide residues for each species.
Results
Localization of Extracellular Polysaccharides Using the Fluorescent Lectin FITC-ConA
We examined the nature of the extracellular polysaccharides found in five diverse coccolithophore species. C. braarudii, S. apsteinii, C. leptoporus, C. carterae, and E. huxleyi were chosen as they represent a range of cell sizes, coccolith morphologies (Figure 1), biogeographical distributions and distinct phylogenic clades (Liu et al., 2010). To examine the distribution of extracellular polysaccharides, we stained both calcified and decalcified cells with fluorescent conjugates of the lectin Concanavalin A (ConA), which binds primarily to D-mannose and D-glucose residues, and imaged them using confocal microscopy.
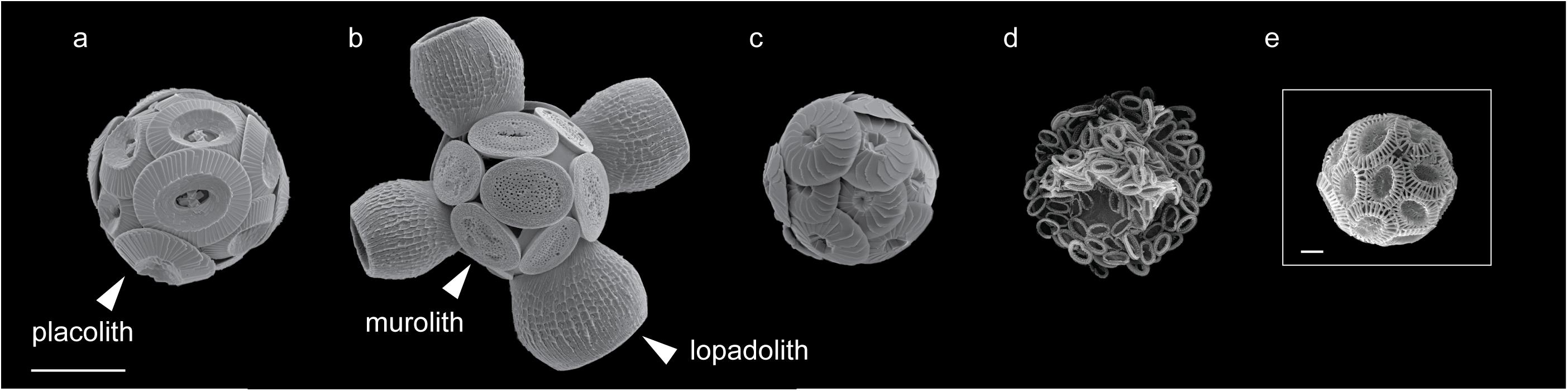
FIGURE 1. Scanning electron microscopy images of coccolithophore species included in this study. (a) Coccolithus braarudii, (b) Scyphosphaera apsteinii, (c) Calcidiscus leptoporus, (d) Chrysotila carterae, and (e) Emiliania huxleyi. All species included are placolith bearing with the exception of S. apsteinii (b) which bears disk-shaped muroliths and barrel-shaped lopadoliths, representative examples of each coccolith type are labeled (arrowhead). Scale bars represent 10 μm (a–d) and 1 μm (e).
Decalcified cells of all five species displayed FITC-ConA staining in a layer surrounding the cells (Figure 2). This indicates that cells from each species are coated in a polysaccharide-rich organic layer containing D-glucose and/or D-mannose residues. Some structural diversity was observed in the polysaccharide layers between species. C. braarudii exhibited a granular polysaccharide layer with distinct regularly spaced areas in which staining was absent (Figure 2A), as observed previously (Walker et al., 2018). These regular ellipsoidal regions likely correspond to the position of the coccoliths. Interestingly, this distinctive structural organization was not observed in the closely related species C. leptoporus. Although some irregularities were observed in the staining of the polysaccharide layer in C. leptoporus, these clearly differ from the regularly spaced oval-shaped areas found in C. braarudii (Figure 2B). In the other three species, the FITC-ConA staining revealed a relatively smooth consistently-stained polysaccharide layer on the cell surface (Figures 2C–E).
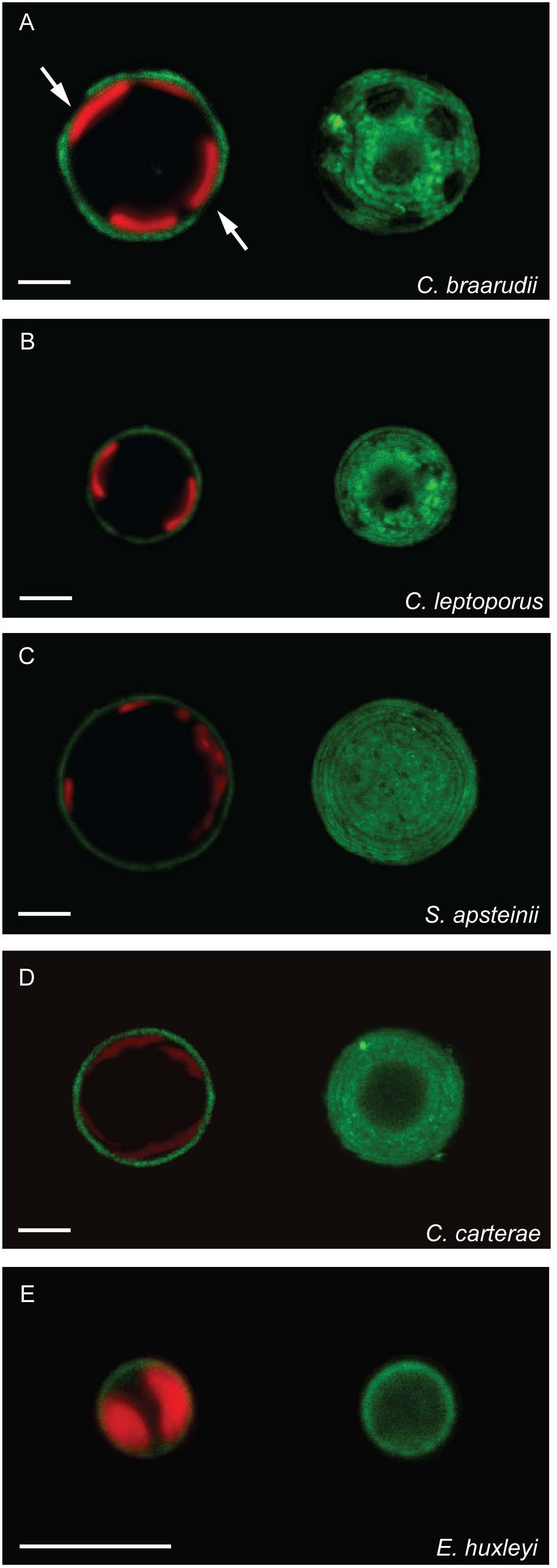
FIGURE 2. A polysaccharide layer on the cell surface of decalcified coccolithophores. Confocal microscopy imaging of decalcified coccolithophores stained with the fluorescent lectin FITC-ConA (green). Chlorophyll autofluorescence is also shown (red). The staining revealed a layer of polysaccharide on the cell surface of all five species. An individual confocal image (left) and a 3D reconstruction from a Z-stack (right) are shown for each species. (A) C. braarudii exhibits regular ellipsoidal intervals in the FITC-ConA staining. (B) C. leptoporus shows some irregularities in the FITC-ConA staining, (C–E) all other species have a smooth consistent layer. Scale bars represent 5 μm.
To investigate the interaction of this polysaccharide layer with the coccosphere, we also imaged FITC-ConA staining in calcified cells. All five species exhibited positive staining (Figure 3 and Table 1). Images of fully calcified C. braarudii cells revealed additional staining patterns with similar dimensions to coccoliths (Figure 3A). To confirm whether this was due in part to a polysaccharide coating on the coccoliths, we imaged discarded C. braarudii coccoliths. These were positively stained by FITC-ConA, indicating a coating of polysaccharide (Figures 1a, 3B SEM for comparison). Treatment of discarded coccoliths with sodium hypochlorite to remove organic material prevented fluorescent staining with FITC-ConA, indicating that the lectin staining of the coccolith related to exogenous organic material (Supplementary Figure 1). Staining of cells with an alternative fluorophore (Texas Red-ConA) ensured that the fluorescence observed with FITC-ConA was not due to cellular autofluorescence or non-specific binding of FITC. We observed identical staining patterns in cells treated with FITC-ConA and Texas Red-ConA (Supplementary Figure 2).
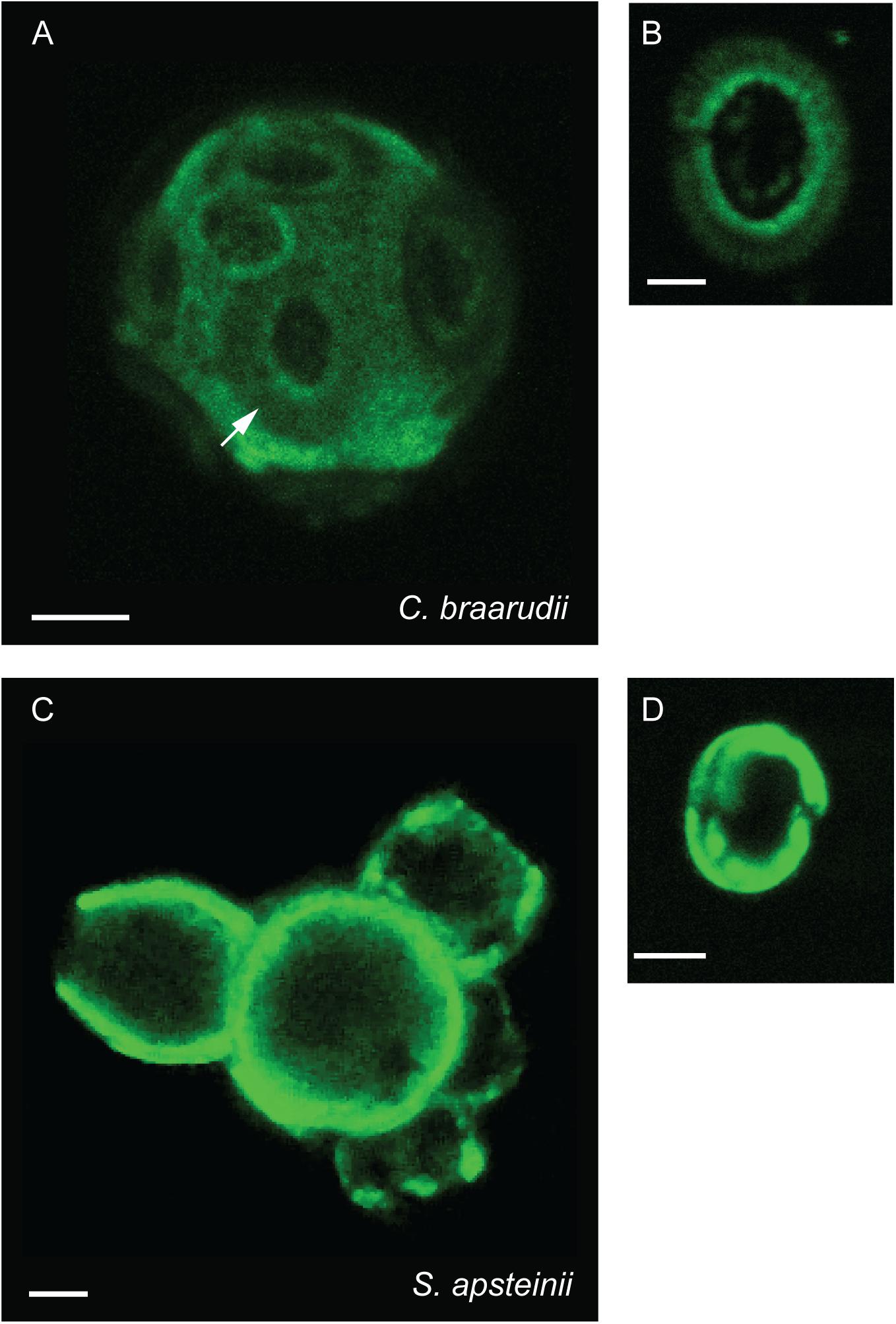
FIGURE 3. Polysaccharides associated with coccosphere. Confocal microscopy imaging of calcified coccolithophores stained with the lectin FITC-ConA. (A) z-stack projection of a calcified C. braarudii cell. Distinct coccolith shaped details are apparent (arrowed). (B) Discarded coccolith from C. braarudii. (C) z-Stack projection of a calcified S. apsteinii cell showing polysaccharide associated with the coccoliths (arrowed). (D) Discarded murolith from S. apsteinii. Scale bars represent 5 μm (A,C) and 2 μm (B,D).
Calcified cells exhibited FITC-ConA staining around the cell body and lopadoliths (Figure 3C). The large size of the lopadoliths in S. apsteinii cells enabled very clear imaging of the polysaccharide coating on the coccoliths. Staining of muroliths within the coccosphere was not clear, although imaging of discarded muroliths revealed distinct ConA staining (Figure 3D). Our combined observations indicate that extracellular polysaccharides both surround the coccoliths and form an organic layer around the cell in multiple species. It is important to note that the polysaccharides observed in each location are not necessarily identical and could exhibit some degree of biochemical variability. Indeed, the lectins may bind to multiple components within the organic layer surrounding the cell, including amorphous polysaccharide, organic scales and fibrillar material, as all of these components are composed of polysaccharide (Drescher et al., 2012).
Localization of Extracellular Polysaccharides Using Wheat Germ Agglutinin
To further examine the nature of the polysaccharides surrounding the cell and the coccoliths, we stained each species with an additional lectin, WGA, which binds predominately to N-acetyl-D-glucosamine and sialic acid residues. Interestingly, FITC-WGA staining was negative in all species except S. apsteinii (Table 1). Calcified S. apsteinii cells stained with WGA revealed a polysaccharide coating on lopadoliths and muroliths, as well as a polysaccharide layer surrounding the cell body (Figures 4A,B). In decalcified cells, we observed that extracellular polysaccharide could be observed that retained the shape of lopadoliths (Figures 4C,D). It is likely that these polysaccharides were associated with the coccoliths prior to decalcification, either externally and/or within the coccolith (intracoccolith polysaccharide). The observed staining pattern suggests that S. apsteinii produces a polysaccharide that also contains N-acetyl-D-glucosamine and/or sialic acid residues and therefore differs in composition from those of the other four species.
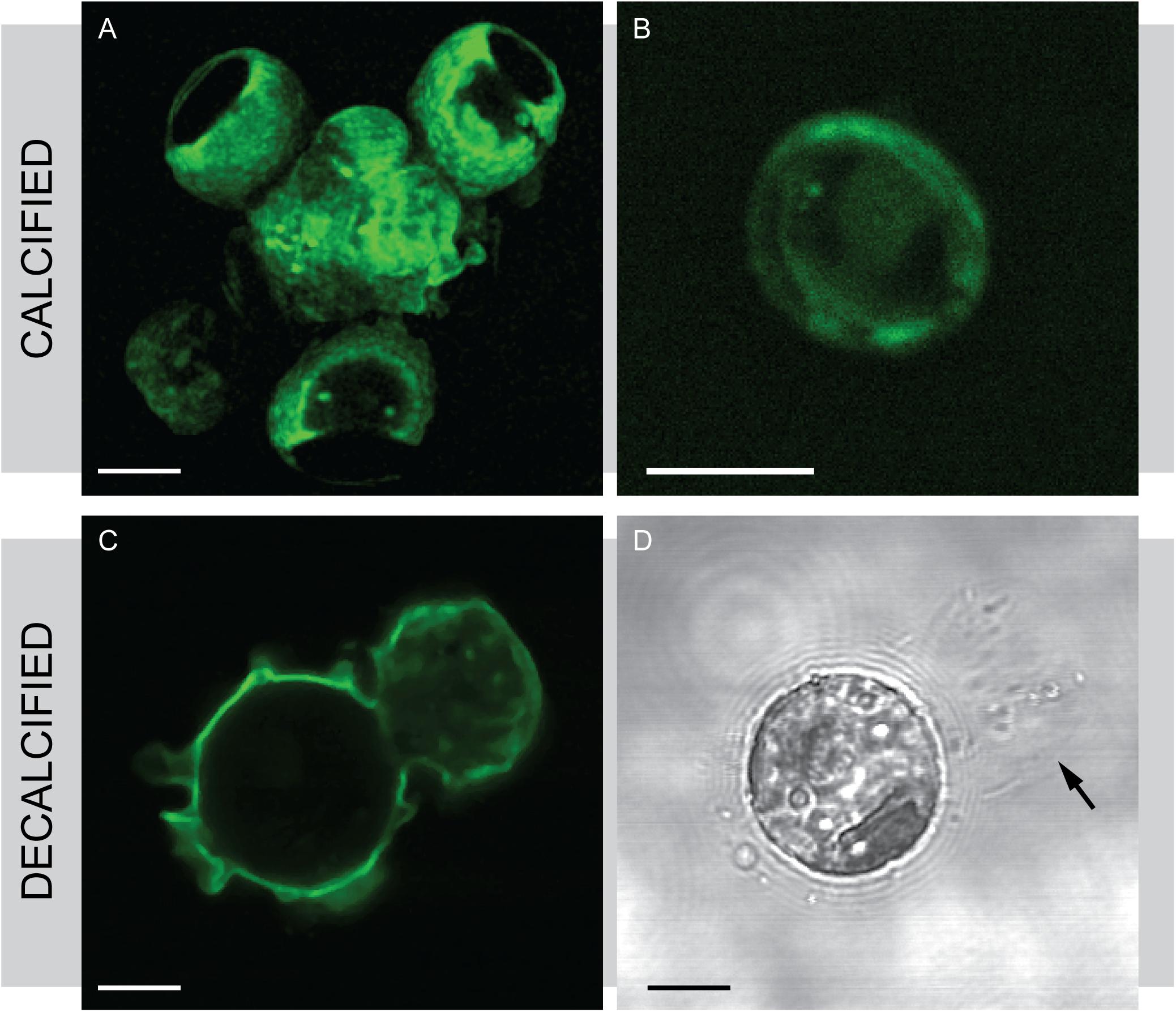
FIGURE 4. Wheat germ agglutinin (WGA) staining in S. apsteinii. Confocal microscopy imaging of FITC-WGA staining in S. apsteinii. (A) FITC-WGA positively stains lopadoliths, muroliths and an extracellular layer surrounding a fully calcified cell. (B) A discarded murolith. (C) FITC-WGA staining of a decalcified cell reveals a polysaccharide residue that closely resembles the shape of a lopadolith. (D) Transmitted light image corresponding to (C). Residual polysaccharide from a dissolved lopadolith can be seen (arrowed). Scale bars represent 5 μm.
To identify if the two lectins applied to S. apsteinii localized to different regions of the extracellular polysaccharide, we utilized FITC-ConA and Texas Red-WGA simultaneously on the same sample of decalcified cells. We found that both lectins positively stain the cell body (Figure 5). In addition, Texas Red-WGA stained polysaccharide left after dissolution of the lopadolith. As the residual polysaccharide was not stained by FITC-ConA, the WGA-positive residues may be found in intracoccolith polysaccharides revealed after decalcification.
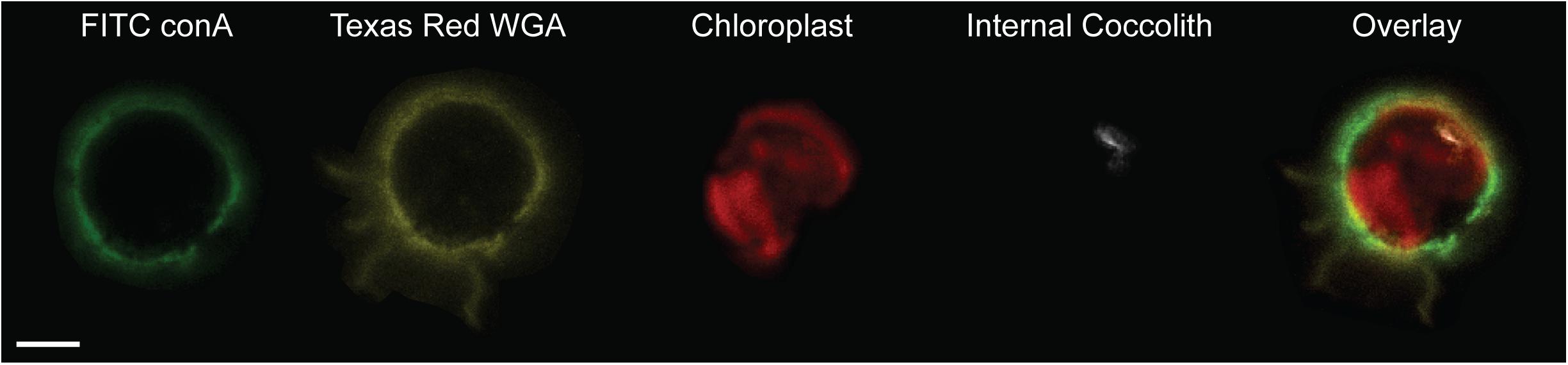
FIGURE 5. Differential localization of ConA and WGA lectin stains in S. apsteinii. Confocal sections of a decalcified S. apsteinii cell stained simultaneously with FITC-ConA and Texas Red-WGA. Both lectins localize around the cell body but Texas Red-WGA staining is additionally found on the residual polysaccharides from the dissolved lopadoliths (yellow). Chlorophyll autfluorescence (red) and reflectance of the internal coccolith (white) is also shown. Scale bar represents 5 μm.
Polysaccharide Extraction and Composition
To examine whether the extracellular polysaccharides identified by lectin staining exhibited significant variability in their biochemical composition, we analyzed polysaccharides from each species by gas chromatography mass spectrometry (GC–MS). We reasoned that it would not be possible to easily separate extracellular polysaccharide from intracellular polysaccharides in whole cell fractions. As we had previously determined that discarded coccoliths are coated in ConA-binding polysaccharide (Figures 3C,D), we isolated coccolith fractions from each species using density centrifugation through Percoll gradients. FITC-ConA staining after density centrifugation confirmed that coccoliths isolated in this manner retain their polysaccharide coating (Supplementary Figure 3). This fraction therefore contains polysaccharides that coat the coccolith, but may also contain polysaccharide components associated with the organic layer around the cell. As the extracellular polysaccharides surrounding coccolithophores are likely to be poorly water-soluble, insoluble polysaccharides were extracted from the coccoliths using 0.1 M EDTA to dissolve calcite and were then washed with 80% ethanol to remove any remaining soluble components.
GC–MS analysis of the monosaccharide residues released by acid hydrolysis of the extracellular polysaccharide fractions recovered from coccoliths revealed distinct differences between species (Figure 6). The results are expressed as the percentage of the monosaccharide and uronic acid residues that could be positively identified. It is possible that rare or unusual sugars could also contribute to the total polysaccharide in these extracts that have been not included in our analysis. We also did not attempt to differentiate between isomers and so it is likely that the galactose peak contains D-galactose and L-galactose, as L-galactose residues are common in many algal polysaccharides (Percival, 1970; Fichtinger-Schepman et al., 1979). L-Mannose has also been previously identified in coccolithophore polysaccharides (Fichtinger-Schepman et al., 1979). The GC–MS analysis also did not allow us to determine whether the polysaccharides were sulfated.
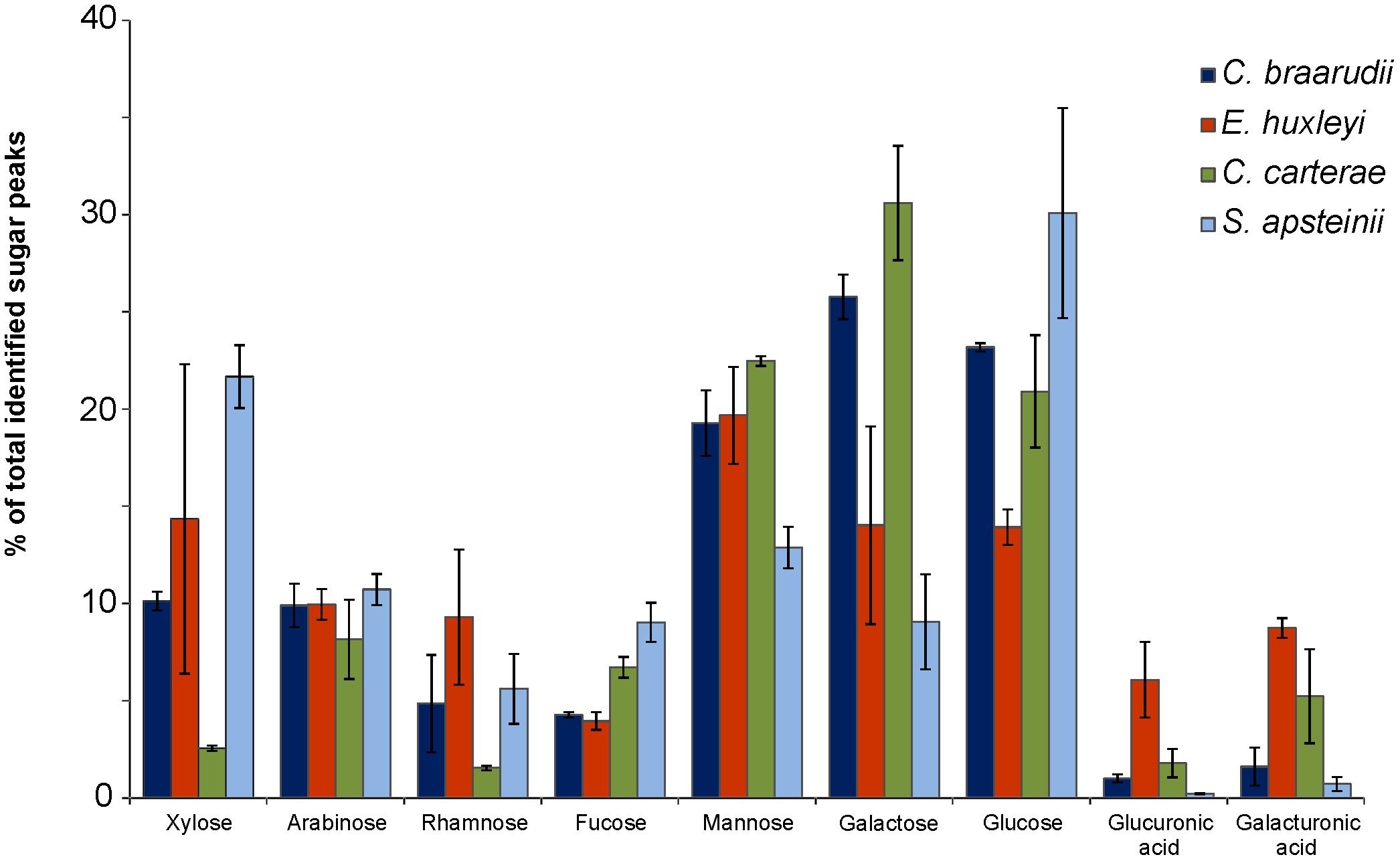
FIGURE 6. Biochemical composition of coccolith-associated polysaccharides (CAPs). Monosaccharides and uronic acids released by acid hydrolysis of 0.1 M EDTA insoluble polysaccharides extracted from purified coccolith fractions from four coccolithophore species (C. braarudii, E. huxleyi, C. carterae, S. apsteinii). Data are presented as the proportion of total identified residues following GC–MS analysis of trimethylsilyl derivatives. n = 3. Error bars represent standard errors.
The polysaccharide fractions isolated from C. carterae were notably lower in xylose (2.6 ± 0.1% SE of total polysaccharide) and rhamnose (1.5 ± 0.1% SE) than the other species (Figure 6). Polysaccharides from S. apsteinii had higher proportions of xylose (21.7 ± 1.6% SE) but lower proportions of galactose (9.1 ± 2.4% SE). However, the most striking differences were found in the uronic acid content, both glucuronic acid and galacturonic acid. Polysaccharides from E. huxleyi and C. carterae were relatively high in uronic acids, containing 14.8 ± 2.3% SE and 7.0 ± 2.6% SE respectively. In contrast the uronic acid content of the polysaccharides isolated from C. braarudii and S. apsteinii were much lower, representing 2.6 ± 1.2 and 0.9 ± 0.4%, respectively of the identified monosaccharide residues.
Production and Role of Extracellular Polysaccharides in C. braarudii
The distinctive morphology of the extracellular polysaccharide observed in decalcified C. braarudii cells (Figure 2A) led us to examine this in greater detail. As the oval-shaped regions are preserved even after decalcification, it suggests that the polysaccharide-rich organic layer in C. braarudii possesses structural properties that may contribute to the organization of the coccosphere. We have previously shown that the non-stained regions in the organic layer are reduced in number or absent when coccolith production is disrupted by treatment with germanium (Ge) (Walker et al., 2018), suggesting that they represent apertures in the polysaccharide layer corresponding to the position of the coccoliths. To test this hypothesis we partially decalcified C. braarudii cells so that we could image the position of the coccoliths in the polysaccharide layer. The non-stained regions correspond exactly to the position of the central-area of the coccoliths (i.e., the inner section excluding the shields) (Figures 7A–C). It is therefore likely that the positioning of the coccoliths in the polysaccharide-rich organic layer causes the formation of the ellipsoidal apertures in this layer.
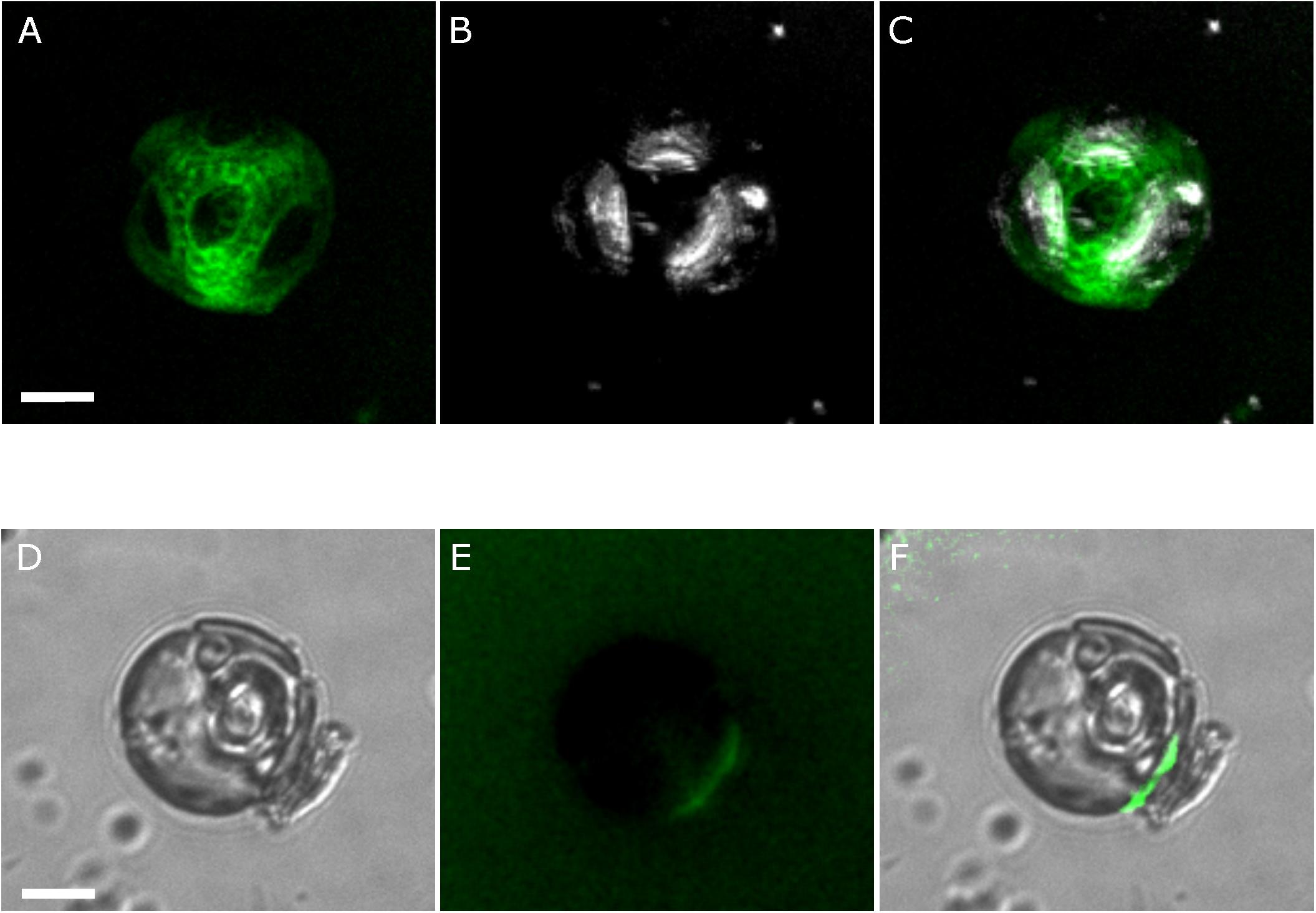
FIGURE 7. Detailed characterization of the extracellular polysaccharide layer in C. braarudii. (A) A partially-decalcified C. braarudii cell stained with the lectin FITC-ConA (green). A 3D projection generated from a Z-stack is shown. (B) The same cell showing the position of three remaining coccoliths (white). (C) Merged image indicating that the ellipsoidal intervals in the FITC-ConA staining correspond to the position of the three remaining coccoliths on the cell surface. An additional coccolith corresponding to the central ellipsoid region has become detached. (D) Differential interference contrast microscopy image of a recalcifying decalcified C. braarudii cell. The position of an internal coccolith and a newly-secreted external coccolith can be seen. (E) Epifluorescence image of the same cell stained with FITC-ConA showing a region of polysaccharide at the cell surface corresponding to the position of the coccolith. Note that this cell has lost its external polysaccharide layer. (F) Merged image. Scale bars represent 5 μm.
Further imaging of decalcified cells demonstrated that a small proportion of cells have lost the polysaccharide-rich organic layer, presumably due to the experimental manipulations associated with decalcification (Supplementary Figure 4). Several cells were observed where the polysaccharide layer was partially detached (n = 3), allowing us to confirm that this layer possesses considerable structural integrity even when dissociated from the cell (Supplementary Figure 4).
The occurrence of decalcified cells lacking a polysaccharide layer allowed us to examine how this layer was formed during coccolith secretion. When these cells were allowed to re-calcify, we found that the newly produced coccoliths exhibited a localized region of FITC-ConA stained polysaccharide on the underside of the coccolith (Figures 7D–F). The data suggests that the insoluble polysaccharide is produced internally and extruded with the coccolith, an observation that correlates with previous polysaccharide studies (van der Wal et al., 1983a,b; Marsh, 1994). The organic layer therefore appears to be formed by the aggregation of polysaccharide-rich material secreted with each coccolith, rather than pre-formed as a complete layer. The close association of the polysaccharide with the underside of the coccolith may indicate that these polysaccharides are involved in adhering the coccoliths to the cell surface, aiding the formation and structure of the complete coccosphere.
Like most phytoplankton cells, the surface area of a coccolithophore cell increases substantially as its volume increases throughout the cell cycle. The cell must therefore continuously produce new coccoliths to ensure that its surface area remains fully covered. This suggests that each new coccolith must be secreted through the existing polysaccharide layer, which must therefore retain a substantial flexibility in order to incorporate this coccolith in to the coccosphere. To determine whether coccoliths were secreted through the organic layer, we imaged coccolith secretion in decalcified cells in which this layer remained intact. The newly-secreted coccoliths were observed external to the organic layer, indicating that they can pass through the layer (Supplementary Figure 5). In addition, coccoliths produced in these cells were coated with polysaccharide on both the underside and the topside of the coccolith. We hypothesize that the coccolith is further coated with polysaccharide as it moves through the existing polysaccharide layer on the cell surface.
Discussion
Previous studies have largely focussed on the ability of CAPs to modulate the precipitation of calcite within the CV, with little research conducted on extracellular role of these polysaccharides. Here we show that polysaccharide material is extruded with the coccolith, which coats the coccolith and contributes to an organic layer associated with the coccosphere. The term CAP has been used to describe polysaccharides found in four distinct cellular locations (Figure 8 and Supplementary Table 1). These are intracoccolith polysaccharides, polysaccharides within the CV actively involved in the modulation of calcite precipitation, polysaccharides coating the surface of coccoliths and polysaccharides covering the cell body. Whilst it is likely that there is a degree of overlap in the nature of these polysaccharides (for example, polysaccharides involved in calcite precipitation and those found within the coccolith are likely to be identical), there may be important differences between them and we suggest that it is important to define the localization of the polysaccharide when using the term CAPs.
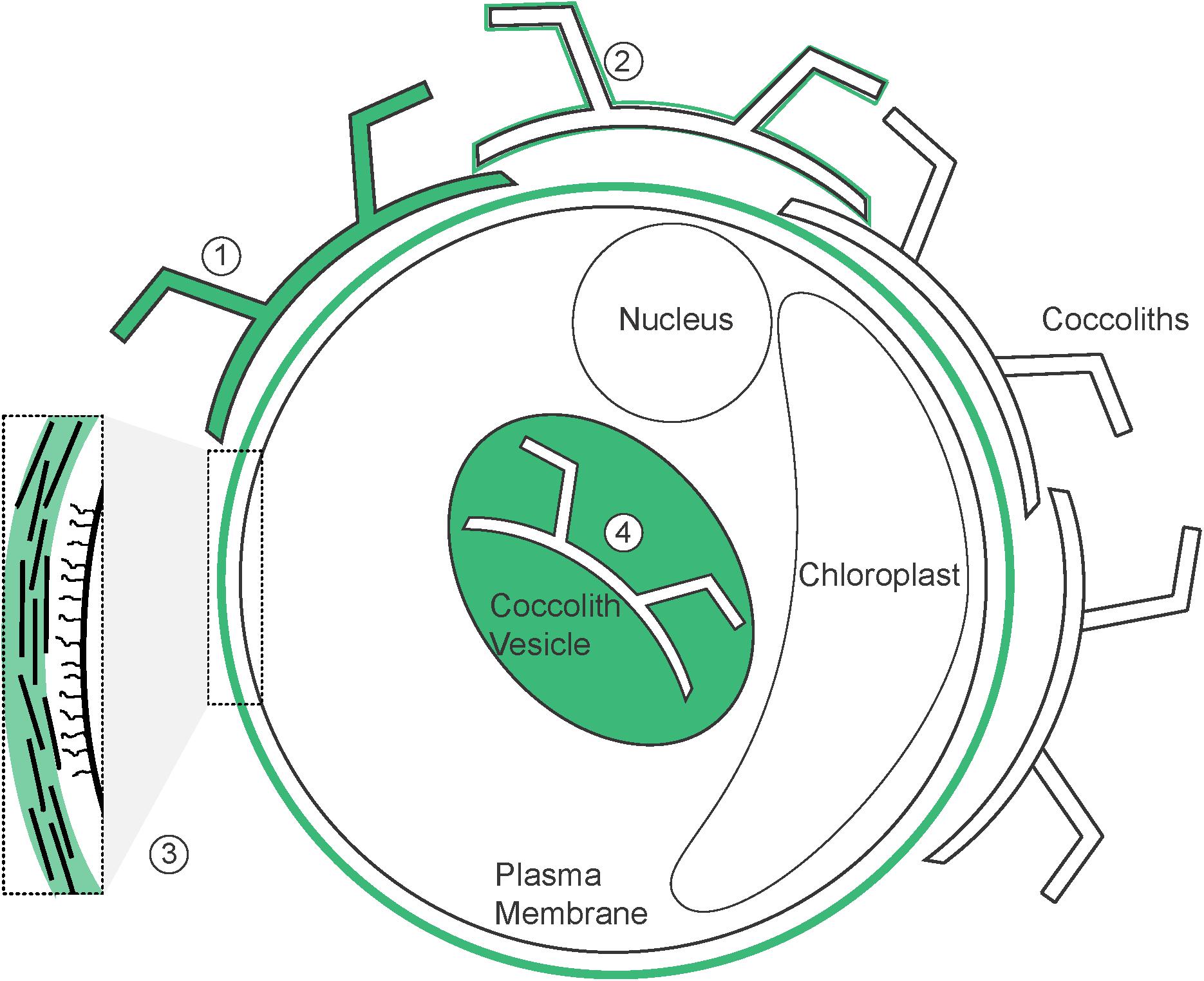
FIGURE 8. Potential localization of CAPs. Schematic illustration of a coccolithophore cell (based on C. braarudii) demonstrating the localization of CAPs identified both in previous research and in this study (highlighted in green). (1) Intracoccolith; (2) coccolith surface; (3) organic layer; (4) coccolith vesicle. Inset shows details of the components of the organic layer; including amorphous polysaccharide (green), organic scales and fibrillar material.
There is some evidence from C. carterae of differentiation of CAPs, even within the CV. Two CAPs (PS1 and PS2) are involved in nucleation of calcite crystal and formation of the protococcolith ring, with the highly acidic PS2 in particular acting as a high capacity Ca2+ buffer to modulate nucleation (Marsh, 2003). Further growth of the coccolith requires the presence of a third polysaccharide, the galacturonomannan PS3 (Marsh et al., 2002). PS2 and PS3 contribute directly to calcite nucleation and mutants that do not produce either polysaccharide exhibit significant defects in coccolith formation (Marsh and Dickinson, 1997; Marsh, 2003). The mature coccolith acquires a coating of polysaccharide comprising of PS1 and PS2 prior to secretion, indicating that polysaccharides that contribute to calcite precipitation in C. carterae also contribute to the coating on external coccoliths (Marsh, 2003). Thus, the extracellular polysaccharides that we have visualized using fluorescent lectins may also be involved in calcite precipitation. The direct association of fluorescently-labeled polysaccharide with newly secreted coccoliths in C. braarudii supports the presence of this polysaccharide in the CV.
Our analyses of the biochemical composition of the polysaccharides extracted from coccoliths indicated that they exhibit some similarities to intracoccolith polysaccharides. Lee et al. (2016) demonstrated that EDTA-soluble intracoccolith polysaccharides derived from E. huxleyi had a much higher uronic acid content (up to 60%) than those derived from C. braarudii (27%). We found a similar trend in the EDTA-insoluble polysaccharide isolated from coccoliths, although the uronic acids represented a lower proportion of the monosaccharides released by acid hydrolysis. This indicates that there is some overlap in the biochemical nature of these polysaccharides, but more detailed examination will be required to determine whether the polysaccharides present in each fraction are structurally similar.
Our research highlights extracellular roles for CAPs that are additional to their roles in modulation of calcite precipitation. The layer of insoluble polysaccharide found on the exterior of the coccolith likely offers a degree of protection from the environment. A previous study suggests that the organic covering prevents dissolution of the calcite in unfavorable conditions (Henriksen et al., 2004). Recent studies have shown that polysaccharides extracted from coccolith-containing ancient sediments (c. 70 Ma) were still functional in protecting coccoliths from dissolution (Sand et al., 2014). The predicted decrease in the calcite saturation state of future oceans will increase the potential for dissolution of calcite structures (Tyrrell et al., 2008), necessitating the requirement for a better understanding of the protective properties of this organic coating.
In addition to protecting coccoliths from dissolution, extracellular polysaccharide appears to play an important role in the formation and organization of the coccosphere. The lectin staining observed around the cell may reflect binding to multiple polysaccharide-rich components within this layer, such as amorphous polysaccharide, organic scales and fibrillar material. In C. braarudii, the polysaccharide material secreted with the coccolith acts to adhere the newly-produced coccolith to the cell surface. Presumably, the continued secretion of multiple coccoliths results in the formation of a complete organic layer around the cell that both ensures the adhesion of the coccoliths to the cell and allows them to be held in position relative to each other. The role of this extracellular organic layer in organizing the coccosphere is clearly visualized in C. braarudii, where the polysaccharide material has a defined structure not seen in other species. Apertures in this layer correspond with the position of the coccoliths, allowing the interlocking coccoliths to be held in a single layer around the cell (Walker et al., 2018). Observations from TEM studies indicate that the organic scales are absent from the central regions underlying coccoliths (Taylor et al., 2007). This distribution suggests that organic scales form a major component of the organic layer visualized by FITC-ConA staining. The presence of organic scales in this layer is likely to contribute to its structural rigidity, but still allow sufficient flexibility for coccoliths to be secreted through the layer. C. braarudii cells always maintain a complete coccosphere as their cell volume increases, comprising of 8–20 coccoliths in healthy cultures (Gibbs et al., 2013; Durak et al., 2016). The rigidity observed in the organic layer may therefore relate to the need for precise organization of the highly ordered interlocking coccoliths. However, the polysaccharide-rich organic layer around C. leptoporus did not exhibit a similar arrangement to C. braarudii, which is surprising given the similar nature of the coccospheres in these species (∼15 coccolith cell-1) (Langer et al., 2006; Liu et al., 2010).
The distribution of organic scales in S. apsteinii cells is similar to C. braarudii in that they are also absent from the areas underlying the coccoliths (Drescher et al., 2012). However, in decalcified S. apsteinii cells the polysaccharide material stained by lectins has a smooth appearance, without apertures corresponding to the position of the coccoliths. It may be that the non-interlocking nature of the coccoliths in S. apsteinii (both lopadoliths and muroliths) results in a much more fluid arrangement of the coccosphere and so any patterns in the distribution of the organic layer are lost following decalcification. The differential staining of lopadoliths in S. apsteinii is also an interesting area for further research as this was the only species included in the study with a dimorphic coccosphere (two coccolith types). The presence of WGA-binding residues within the lopadoliths may relate to unique properties of these CAPs that are associated with the formation or structural maintenance of these much larger coccoliths.
The absence of defined features in the polysaccharide-rich organic layers around E. huxleyi and C. carterae cells may reflect the less ordered nature of their coccospheres and a greater turnover of coccoliths within these coccospheres. For example, E. huxleyi produces between 23 and 36 coccoliths per cell in optimal growing conditions and as many as 20 of these are discarded (Paasche, 1998, 1999, 2001). Organic scales are also absent from E. huxleyi, which will likely alter the structural properties of its organic layer considerably. As the arrangement of the coccospheres in these species is less rigid, the organic layer may act primarily to adhere the coccoliths to the cell, rather than hold them in a specific position. The ability of the coccoliths to move flexibly within a layer of polysaccharide is important for the ability of E. huxleyi coccospheres to resist a mechanical load, suggesting that the polysaccharide layer contributes directly to the protective role of coccosphere (Jaya et al., 2016).
The diversity in the composition and functional roles of CAPs between species is important when the energetic cost of polysaccharide production is considered. Recent estimates suggest that the single intracoccolith CAPs produced by E. huxleyi and C. braarudii require 7 and 0.2% of total cellular fixed organic carbon respectively (Monteiro et al., 2016). In contrast, production of multiple CV-associated CAPs in C. carterae was calculated to cost of 50% of the total fixed organic carbon, a significantly higher cost of production (Brownlee and Taylor, 2004). The large range of these values reflects different assumptions over the role of CAPs. The former estimates are based primarily on the amounts of intracoccolith CAPs recovered from purified coccoliths, whereas the latter calculation assumes CAPs are involved in the stoichiometric delivery of Ca2+ to the CV. Our results clearly indicate that the extracellular polysaccharide components constitute a major additional sink for fixed carbon associated with coccolith production that has so far not been included in these estimates.
In this study we have expanded on the current understanding of CAPs and their role within coccolithophore calcification. CAPs are a group of polysaccharides involved in coccolith precipitation, coccolith adhesion, organization, and protection of the coccosphere. We have visualized extracellular polysaccharide material coating the coccoliths and contributing to the formation of an organic layer around the cell. These differing roles suggest that CAPs likely exhibit significant diversity in function and composition that remains to be fully understood. However, it is imperative that their abundance and role should be taken in to account in considerations of energy budgeting and response of coccolithophores to future ocean conditions.
Author Contributions
CW performed all experimental analyses. SH provided additional imaging of extracellular polysaccharides. AT supported SEM analysis. DS and NS contributed to GC–MS analysis of coccolith polysaccharides. GW, CB, and CW designed the study. CW, CB, AT, GL, and GW wrote the manuscript.
Funding
The authors acknowledge funding from NERC SPITFIRE DTP studentship to CW. GW and CB acknowledge support from NERC (NE/N011708/1) and the European Research Council (ERC-ADG 670390). CW was additionally supported by the Gillings Graduate Exchange Programme (University of Southampton/University of North Carolina Wilmington). AT acknowledges NSF support (NSFGEO-NERC-1638838).
Conflict of Interest Statement
The authors declare that the research was conducted in the absence of any commercial or financial relationships that could be construed as a potential conflict of interest.
Supplementary Material
The Supplementary Material for this article can be found online at: https://www.frontiersin.org/articles/10.3389/fmars.2018.00306/full#supplementary-material
References
Borman, A. H., De Jong, E. W., Huizinga, M., Kok, D. J., Westbroek, P., and Bosch, L. (1982). The role in CaCO3 crystallization of an Acid Ca2+-Binding polysaccharide associated with coccoliths of Emiliania huxleyi. Eur. J. Biochem. 129, 179–183. doi: 10.1111/j.1432-1033.1982.tb07037.x
Borman, A. H., De Jong, E. W., Thierry, R., Westbroek, P., and Bosch, L. (1987). Coccolith-associated polysaccharides from cells of Emiliania huxleyi (Haptophyceae). J. Phycol. 23, 118–125. doi: 10.1111/j.1529-8817.1987.tb04433.x
Brownlee, C., and Taylor, A. (2004). “Calcification in coccolithophores: a cellular perspective,” in Coccolithophores: From Molecular Processes to Global Impact, eds H. Thierstein and J. Young (New York, NY:: Springer), 31–49.
Daniels, C. J., Sheward, R. M., and Poulton, A. J. (2014). Biogeochemical implications of comparative growth rates of Emiliania huxleyi and Coccolithus species. Biogeosciences 11, 6915–6925. doi: 10.5194/bg-11-6915-2014
De Jong, E. W., Bosch, L., and Westbroek, P. (1976). Isolation and characterization of a Ca2+ binding polysaccharide associated with Coccoliths of Emiliania huxleyi (Lohmann) Kamptner. Eur. J. Biochem. 70, 611–621. doi: 10.1111/j.1432-1033.1976.tb11052.x
Drescher, B., Dillaman, R. M., and Taylor, A. R. (2012). Coccolithogenesis in Scyphosphaera apsteinii (Prymnesiophyceae). J. Phycol. 48, 1343–1361. doi: 10.1111/j.1529-8817.2012.01227.x
Durak, G. M., Taylor, A. R., Walker, C. E., Probert, I., De Vargas, C., Audic, S., et al. (2016). A role for diatom-like silicon transporters in calcifying coccolithophores. Nat. Commun. 7:10543. doi: 10.1038/ncomms10543
Engel, A., Delille, B., Jacquet, S., Riebesell, U., Rochelle-Newall, E., Terbruggen, A., et al. (2004). Transparent exopolymer particles and dissolved organic carbon production by Emiliania huxleyi exposed to different CO2 concentrations: a mesocosm experiment. Aquat. Microb. Ecol. 34, 93–104. doi: 10.3354/ame034093
Fichtinger-Schepman, A. M. J., Kamerling, J. P., Vliegenthart, J. F. G., Dejong, E. W., Bosch, L., and Westbroek, P. (1979). Composition of a methylated, acidic polysaccharide associated with coccoliths of Emiliania-huxleyi (Lohmann) Kamptner. Carbohydr. Res. 69, 181–189. doi: 10.1016/S0008-6215(00)85763-8
Gal, A., Wirth, R., Kopka, J., Fratzl, P., Faivre, D., and Scheffel, A. (2016). Macromolecular recognition directs calcium ions to coccolith mineralization sites. Science 353, 590–593. doi: 10.1126/science.aaf7889
Gibbs, S. J., Poulton, A. J., Brown, P. R., Daniels, C. J., Hopkins, J., Young, J. R., et al. (2013). Species-specific growth response of coccolithophores to palaeocene–eocene environmental change. Nat. Geosci. 6, 218–222. doi: 10.1038/ngeo1719
Guillard, R. R. L., and Ryther, J. H. (1962). Studies of marine planktonic diatoms. Can. J. Microbiol. 8, 229–239. doi: 10.1139/m62-029
Harrison, P. J., Waters, R. E., and Taylor, F. (1980). A broad spectrum artificial sea water medium for coastal and open ocean phytoplankton. J. Phycol. 16, 28–35. doi: 10.1111/j.1529-8817.1980.tb00724.x
Henriksen, K., and Stipp, S. L. S. (2009). Controlling biomineralization: the effect of solution composition on coccolith polysaccharide functionality. Cryst. Growth Des. 9, 2088–2097. doi: 10.1021/cg8004272
Henriksen, K., Young, J., Bown, P., and Stipp, S. (2004). Coccolith biomineralisation studied with atomic force microscopy. Palaeontology 47, 725–743.
Hirokawa, Y., Fujiwara, S., and Tsuzuki, M. (2005). Three types of acidic polysaccharides associated with coccolith of Pleurochrysis haptonemofera: comparison with Pleurochrysis carterae and analysis using fluorescein-isothiocyanate-labeled lectins. Mar. Biotechnol. 7, 634–644. doi: 10.1007/s10126-004-5148-9
Jaya, B. N., Hoffmann, R., Kirchlechner, C., Dehm, G., Scheu, C., and Langer, G. (2016). Coccospheres confer mechanical protection: new evidence for an old hypothesis. Acta Biomater. 42, 258–264. doi: 10.1016/j.actbio.2016.07.036
Kayano, K., Saruwatari, K., Kogure, T., and Shiraiwa, Y. (2011). Effect of coccolith polysaccharides isolated from the coccolithophorid, Emiliania huxleyi, on calcite crystal formation in in vitro CaCO3 crystallization. Mar. Biotechnol. 13, 83–92. doi: 10.1007/s10126-010-9272-4
Langer, G., Geisen, M., Baumann, K.-H., Kläs, J., Riebesell, U., Thoms, S., et al. (2006). Species-specific responses of calcifying algae to changing seawater carbonate chemistry. Geochem. Geophys. Geosyst. 7:Q09006. doi: 10.1029/2005GC001227
Lee, R. B. Y., Mavridou, D. A. I., Papadakos, G., Mcclelland, H. L. O., and Rickaby, R. E. M. (2016). The uronic acid content of coccolith-associated polysaccharides provides insight into coccolithogenesis and past climate. Nat. Commun 7:13144. doi: 10.1038/ncomms13144
Liu, H., Aris-Brosou, S., Probert, I., and De Vargas, C. (2010). A time line of the environmental genetics of the haptophytes. Mol. Biol. Evol. 27, 161–176. doi: 10.1093/molbev/msp222
Marsh, M. (1994). Polyanion-mediated mineralization — assembly and reorganization of acidic polysaccharides in the Golgi system of a coccolithophorid alga during mineral deposition. Protoplasma 177, 108–122. doi: 10.1007/BF01378985
Marsh, M. E. (2003). Regulation of CaCO3 formation in coccolithophores. Comp. Biochem. Physiol. B Biochem. Mol. Biol. 136, 743–754. doi: 10.1016/S1096-4959(03)00180-5
Marsh, M. E., and Dickinson, D. P. (1997). Polyanion-mediated mineralization — mineralization in coccolithophore (Pleurochrysis carterae) variants which do not express PS2, the most abundant and acidic mineral-associated polyanion in wild-type cells. Protoplasma 199, 9–17. doi: 10.1007/BF02539801
Marsh, M. E., Ridall, A. L., Azadi, P., and Duke, P. J. (2002). Galacturonomannan and Golgi-derived membrane linked to growth and shaping of biogenic calcite. J. Struct. Biol. 139, 39–45. doi: 10.1016/S1047-8477(02)00503-8
Monteiro, F. M., Bach, L. T., Brownlee, C., Bown, P., Rickaby, R. E. M., Poulton, A. J., et al. (2016). Why marine phytoplankton calcify. Sci. Adv. 2:e1501822. doi: 10.1126/sciadv.1501822
Outka, D. E., and Williams, D. C. (1971). Sequential coccolith morphogenesis in Hymenomonas-carterae. J. Protozool. 18, 285–297. doi: 10.1111/j.1550-7408.1971.tb03319.x
Paasche, E. (1998). Roles of nitrogen and phosphorus in coccolith formation in Emiliania huxleyi (Prymnesiophyceae). Eur. J. Phycol. 33, 33–42. doi: 10.1080/09670269810001736513
Paasche, E. (1999). Reduced coccolith calcite production under light-limited growth: a comparative study of three clones of Emiliania huxleyi (Prymnesiophyceae). Phycologia 38, 508–516. doi: 10.2216/i0031-8884-38-6-508.1
Paasche, E. (2001). A review of the coccolithophorid Emiliania huxleyi (Prymnesiophyceae), with particular reference to growth, coccolith formation, and calcification-photosynthesis interactions. Phycologia 40, 503–529. doi: 10.2216/i0031-8884-40-6-503.1
Percival, E. (1970). “Algal polysaccharides,” in The Carbohydrates. Chemistry and Biochemistry, ed. W. Pigman (New York, NY: Academic Press), 537–569.
Pienaar, R. N. (1994). “Ultrastructure and calcification of coccolithophores,” in Coccolithophores, eds A. Winter and W. G. Siesser (Cambridge: Cambridge University Press), 13–38.
Rost, B., and Riebesell, U. (2004). “Coccolithophores and the biological pump: responses to environmental changes,” in Coccolithophores: From Molecular Process to Global Impact, eds H. Thierstein and J. Young (Heidelberg: Springer), 99–126.
Sand, K., Pedersen, C., Sjoberg, S., Nielsen, J., Makovicky, E., and Stipp, S. (2014). Biomineralization: long-term effectiveness of polysaccharides on the growth and dissolution of calcite. Cryst. Growth Des. 14, 5486–5494. doi: 10.1021/cg5006743
Taylor, A. R., Brownlee, C., and Wheeler, G. (2017). Coccolithophore cell biology: chalking up progress. Ann. Rev. Mar. Sci. 9, 283–310. doi: 10.1146/annurev-marine-122414-034032
Taylor, A. R., Russell, M. A., Harper, G. M., Collins, T. F. T., and Brownlee, C. (2007). Dynamics of formation and secretion of heterococcoliths by Coccolithus pelagicuss sp.braarudii. Eur. J. Phycol. 42, 125–136. doi: 10.1080/09670260601159346
Tyrrell, T., Schneider, B., Charalampopoulou, A., and Riebesell, U. (2008). Coccolithophores and calcite saturation state in the Baltic and Black Seas. Biogeosciences 5, 485–494. doi: 10.5194/bg-5-485-2008
van der Wal, P., De jong, E. W., Westbroek, P., Debruijn, W. C., and Mulder-stapel, A. A. (1983a). Polysaccharide localization, coccolith formation, and golgi dynamics in the coccolithophorid Hymenomonas carterae. J. Ultrastr. Res. 85, 139–158.
van der Wal, P., De Jong, E. W., Westbroek, P., De Bruijn, W., and Mulder-Stapel, A. (1983b). Ultrastructural polysaccharide localization in calcifying and naked cells of the coccolithophorid Emiliania huxleyi. Protoplasma 118, 157–168. doi: 10.1007/BF01293073
Walker, C. E., Taylor, A. R., Langer, G., Heath, S., Probert, I., Tyrrell, T., et al. (2018). The requirement for calcification differs between ecologically important coccolithophore species. New Phytol. doi: 10.1111/nph.15272
Westbroek, P., Brown, C. W., Bleijswijk, J. V., Brownlee, C., Brummer, G. J., Conte, M., et al. (1993). A model system approach to biological climate forcing. The example of Emiliania huxleyi. Glob. Planet. Chang. 8, 27–46. doi: 10.1016/0921-8181(93)90061-R
Westbroek, P., De Jong, E., Dam, W., and Bosch, L. (1973). Soluble intracrystalline polysaccharides from coccoliths of Coccolithus huxleyi (Lohmann) kamptner (I). Calcif. Tissue Int. 12, 227–238. doi: 10.1007/BF02013737
Young, J., Geisen, M., Cros, L., Kleijne, A., Sprengel, C., Probert, I., et al. (2003). A guide to extant coccolithophore taxonomy. Int. Nannoplankton Assoc. 1:125.
Young, J. R., Davis, S. A., Bown, P. R., and Mann, S. (1999). Coccolith ultrastructure and biomineralisation. J. Struct. Biol. 126, 195–215. doi: 10.1006/jsbi.1999.4132
Keywords: coccolithophore, calcification, Coccolithus braarudii, polysaccharide, lectin
Citation: Walker CE, Heath S, Salmon DL, Smirnoff N, Langer G, Taylor AR, Brownlee C and Wheeler GL (2018) An Extracellular Polysaccharide-Rich Organic Layer Contributes to Organization of the Coccosphere in Coccolithophores. Front. Mar. Sci. 5:306. doi: 10.3389/fmars.2018.00306
Received: 24 May 2018; Accepted: 10 August 2018;
Published: 30 August 2018.
Edited by:
Alfred Portius Wheeler, Clemson University, United StatesReviewed by:
Yoshihiro Shiraiwa, University of Tsukuba, JapanKarina Krarup Sand, University of Copenhagen, Denmark
Copyright © 2018 Walker, Heath, Salmon, Smirnoff, Langer, Taylor, Brownlee and Wheeler. This is an open-access article distributed under the terms of the Creative Commons Attribution License (CC BY). The use, distribution or reproduction in other forums is permitted, provided the original author(s) and the copyright owner(s) are credited and that the original publication in this journal is cited, in accordance with accepted academic practice. No use, distribution or reproduction is permitted which does not comply with these terms.
*Correspondence: Glen L. Wheeler, Z2x3QG1iYS5hYy51aw==