- 1Department of Biological Oceanography, CICESE, Ensenada, Mexico
- 2Departamento de Oceanografía Biológica, Instituto de Investigaciones Oceanológicas, Universidad Autónoma de Baja California, Ensenada, Mexico
Recruitment is a fundamental step upon which all subsequent interactions within a community occur. We explored how the attenuation of physical conditions by seaweed plots comprised of either Chondracanthus canaliculatus, Pyropia perforata, Sylvetia compressa or a mixed aggregation, at varying densities (average 1,199, 816, and 408 in. m−2), affected recruitment of seaweeds and microphytobenthic organisms in the understory, and if physical factors modulate their abundance and distribution. We outplanted macroscopic seaweeds in the intertidal and measured changes in understory irradiance, particle retention, and bulk water flow. Both factors influenced physical conditions below the canopy. However, only canopy density had a significant effect on recruitment. The low-density canopy treatments had a greater abundance of seaweed recruits, with the opposite found for microphytobenthic organisms. The recruitment processes of seaweeds and microphytobenthic organisms, however, appeared to be independent of each other and were not due to competition. We conclude that it is crucial to consider microscale biological interactions, which are rarely addressed when assessing recruitment processes of benthic primary producers.
Introduction
Abiotic factors can affect both the distribution and abundance of organisms, thus modulating community structure (Crain and Bertness, 2006). In a recent contribution, Umanzor et al. (2017) explored how different seaweed aggregations influenced the abundance and distribution of understory microphytobenthic (MPB) organisms (benthic diatoms and cyanobacteria) on an exposed rocky intertidal. Results showed that the settlement of microphytobenthic organisms was modulated by the interaction between the species composition and the density of the seaweed aggregations, with branched morphologies at higher densities having higher particle retention and greater abundance of MPB organisms underneath their canopies. Authors also reported recruitment (here defined as early post- settlement sensu Vadas et al., 1992) of seaweeds, although there was no further analysis on recruitment patterns of seaweed spores across the treatments. Therefore, in this contribution, we repeated the experiment assessing the effect that macroscopic seaweeds as ecosystem engineers had on the recruitment of the microscopic stages of seaweeds (spores, gametophytes, and early sporophytes). We then evaluated the factors that could be affecting the patterns of distribution and abundance of seaweed recruits underneath manipulated canopies in the intertidal compared to MPB settlement.
Positive interactions and stress attenuation can play an important role in determining the establishment and survival of seaweed recruits (Bertness et al., 1999; Choi and Norton, 2005; Bennett and Wernberg, 2014). Overall, recruitment is the key process upon which all subsequent interactions within a community will occur. As such, variations in successful recruitment events can substantially influence the dynamics of adult populations (Woodin, 1991; Vadas et al., 1992). Evidence shows that there are multiple factors, both inherent and external to the species, that can influence successful settlement and recruitment of early stages. Inherent factors can include the number of propagules produced, growth rates and size of settling cells, germination and spore viability, and even the strength of adhesion by early propagules (Vadas et al., 1992).
On the other hand, external factors such as particle movement resulting in sedimentation, siltation, scour or increased turbidity might affect early settlers by either preventing or enhancing their survival. On the Great Barrier Reef, for example, increased sedimentation has significantly decreased the rates of recruitment, survival, growth, and regeneration of Sargassum sp. (Umar et al., 1998). Also, in a laboratory experiment, Watanabe et al. (2016) found that the adhesion rate of spores and the gametophyte survival and growth rates of Eisenia sp. (now Ecklonia) declined noticeably with increasing sedimentation rates. Particle movement resulting in complete burial or sand scour can, however, also have a positive outcome for some algal species such as Rhodomela, Penicillus, and Halimeda, allowing their colonization of areas where other species would not thrive (Hurd et al., 2014).
Moreover, substrate properties such as its topography and stability can also greatly enhance or reduce successful seaweed recruitment. In a controlled experiment, Callow et al. (2002) tested how varying microtopographies affected the settlement of Enteromorpha sp. spores. They found that lower profile topographies significantly reduced the abundance of settled spores. Contrarily, Schumacher et al. (2007) found that smooth surfaces enhanced spore settlement of Ulva sp. In fact, Linskens (1966) reported that algae propagules will either settle on smooth or rugose surfaces, depending on the species. Coupled with the substrate properties, water motion is another factor that has long been studied as critical in influencing settlement and survival of seaweed propagules (Vadas et al., 1992). Seaweed zygotes use a range of adhesive mechanisms for attachment, allowing them to either thrive in low or high wave-energy environments. Through field and laboratory experiments, Taylor and Schiel (2003) demonstrated that the “stickability” of Durvillaea antarctica zygotes allowed the species to attach immediately and firmly to surfaces exposed to different wave regimes, resulting in high rates of survival when compared to zygotes of Hormosira banksii and Cystophora torulosa.
For intertidal seaweeds, desiccation stress due to aerial exposure can also cause increased mortality of early stages. Brawley and Johnson (1991) showed that without the protection against water loss provided by parental canopies, a large percentage of seaweed early settlers would inevitably die. However, pre-existing canopies can also represent a stressful biotic force, which can also potentially influence recruitment success. Canopies can cause shading, sweep propagules away or prevent settlement entirely, outcompete them for space and nutrients, or cause chemical interferences (Sousa, 1979; McCourt, 1984; Brawley and Johnson, 1991), that in the short term will trigger high mortality rates of potential new settlers. Evidence for canopy inhibition of early-post settlement or recruitment has been recorded in succession and reproductive ecology studies in the intertidal zone, with overall recruitment increasing due to the attrition of canopy cover (Sousa, 1979; Robertson, 1987).
Together, seaweed aggregations and microphytobenthic biofilms can interact directly or indirectly by modifying biophysical parameters (Fong et al., 1993; Hardison et al., 2013), and thus a influence the recruitment of associated organisms. For example, Hardison et al. (2013) measured the independent and interactive effect that both the MPB and benthic macroalgae can have on the quality and quantity of sediment organic matter (SOM). They concluded that while the MPB increased the SOM liability, benthic macroalgae tend to decrease it. They also found that both groups influenced bacterial build-up that could have a further effect on hypoxia events, sulfide accumulation, mineralization or denitrification of shallow water systems. Bacterial build up can also be important in determining the abundance and distribution of a variety of organisms, as for many benthic invertebrates, larval settlement occurs in response to bacterial cues (Freckelton et al., 2017).
Despite the many contributions related to seaweed recruitment in the intertidal zone, few studies have simultaneously explored the recruitment and development of seaweeds and the MPB. In part, this could be attributed to the difficulty in obtaining in situ measurements from organisms of such small size, but also could be due to the complexity of characterizing the microenvironment they inhabit. However, because seaweed recruits and MPB colonize similar areas, we expect the abundance of their early stages to be limited or enhanced by the same physical factors. Consequently, we tested the following hypotheses: (1) seaweed recruitment shows a similar abundance and distribution underneath intertidal canopies of different species and densities and (2) seaweed recruitment follows the same pattern of distribution as the microphytobenthos underneath seaweed canopies. We constructed experimental quadrats consisting of Pyropia perforata (Agardh, 1883), Silvetia compressa (Agardh, 1848), Chondracanthus canaliculatus (Harvey, 1840), and a mixed assemblage comprised of the former three, at three densities. We then determined if seaweed recruitment was correlated to the attenuation of bulk water flow, particle transport, and irradiance driven by the experimental canopies.
Materials and Methods
Study Site
Experiments were conducted for a 15-day period on a rocky shore in Baja California (31° 51′ 41.6″ N and 116° 39′ 58.1″ W) during spring (2016) when the selected seaweeds (C. canaliculatus, Silvetia compressa, and P. perforata) were abundant. These species were selected because they are among the most common species in local intertidal sites, often forming dense beds or patches. This area has a semidiurnal tidal cycle with two low tides and two high tides of different heights per day (Umanzor et al., 2017). The experimental quadrats were fixed in the mid-intertidal zone, where seaweeds are generally abundant, but in a clear section allowing us to minimize effects caused by natural stands of seaweed. All quadrats remained submerged during high tides and the less extreme low tides.
Targeted Seaweeds
Chondracanthus canaliculatus is a corticated red alga, abundant from the mid to low rocky intertidal zones in Baja California. Although the species is perennial, it is particularly abundant during spring and summer when growth peaks (García-Lepe et al., 1997). The fucoid Silvetia compressa is a leathery brown alga, abundant in the upper and mid intertidal. It is a perennial species, which produces eggs ranging from 80 to 100 μm, showing peak recruitment periods throughout the year (Johnson and Brawley, 1998). Finally, P. perforata is a foliose red algae, abundant in the upper and mid intertidal, with peak abundance during spring and summer (Zertuche-González et al., 2000). In Baja California, the macroscopic stages of P. perforata can persist throughout the year. Both red algae can produce spores larger than 20 μM (Knight and Nelson, 1999; Maggs and Callow, 2003; Avila et al., 2011). At the time of the experiment, at least 50% of all blades were reproductive.
Experimental Design
To determine the effect that different canopies had on the recruitment of seaweeds compared to recruitment of MPB cells, we installed experimental quadrats in the intertidal zone. Quadrats consisted of monocultures of the selected species or a mixed tri-culture (including all three species) at three densities each. We assembled each quadrat using seaweed fragments of approximately 10 cm in lenght collected from the intertidal zone. Densities were assigned based on the maximum density of these species per square meter on a nearby rocky shore, quantified in situ before sample collection. In addition, control treatments with no algae were included. Quadrats were checked daily, and seaweeds showing any damage were immediately replaced by fresh ones to maintain the appropriate density for each treatment.
As each treatment included three replicates, the design comprised 48 quadrats total (Figure 1), following an orthogonal approach (Underwood, 1997). Quadrats consisted of 30 x 30 cm steel frames covered with plastic netting to which seaweeds and data collection devices were attached. Quadrats were individually secured to the intertidal using 2.5 kg lead weights. Settlement slides, particle collectors, light meters, and plaster cylinders were fixed underneath the canopy in each quadrat.
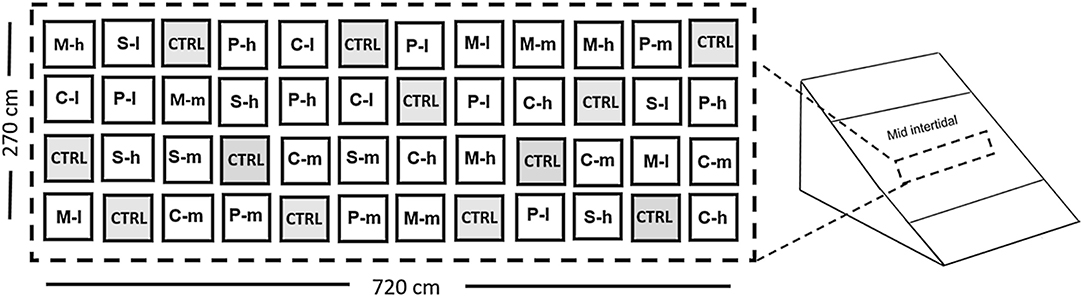
Figure 1. Experimental design comprising 48 randomly distributed quadrats including either Chondracanthus canaliculatus (C), Silvetia compressa (S), Pyropia perforata (P), or the mixed assemblage (M) at a given density: high (h), medium (m), low (l), or control (CTRL). Quadrats were assembled with ropes cultured with fragments of the selected seaweeds.
Physical Variable Sampling
Relative measurements of light levels were obtained using light meters (ONSET™ computer corp., Ma, USA) attached below the canopies and programmed to record every 15 min for a 7-day period. We calculated particle retention below the canopies by dry weight differences of two synthetic fiber pads (25 × 75 mm, initial weight 1.362 ± 0.003 g) per quadrat. Pads were collected after a 48 h period underneath the canopies. After collection, fiber pads were oven dried at 70°C for 60 h and then weighed three times (Sartorius, Germany ± 0.0001 g) to obtain the average weight per day per quadrat. A proxy measure of the relative bulk water flow underneath the canopies was acquired using the dissolution of plaster. Two cylindrical plaster bars (1 × 8 cm, initial weight 10.422 ± 0.005) were installed per quadrat and subsequently removed after 48 h. The bars were then oven dried at 70°C for 72 h before weighing them three times to obtain an average per quadrat. The difference in dry weight before and after deployment allows a relative estimate of bulk water flow based on the dissolution of plaster in a given area over a standardized time when compared to a control with no water motion (Komatsu and Kawai, 1992).
Microscopic Seaweed and Microphytobenthic Recruitment
To assess recruitment by seaweed microscopic stages and the microphytobenthos, a transparent polycarbonate slide (25 × 75 × 3 mm) was fixed underneath every canopy treatment and collected after a 15-day period. After collection, slides were placed individually in Petri dishes containing filtered (1 μM) seawater and immediately fixed with Lugol's solution (1%) for direct cell counting at 400x with an inverted microscope (Zeiss AxioObserver, Germany). We divided each slide into 10 equally sized sections from which a photograph was taken. We used all photographs for recruitment quantification and identification. For seaweed recruitment, we considered spores, gametophytes, and early sporophytes, regardless of size. When possible, we further classified them as red, brown or green. For the MPB, we only considered cells bigger than 20 μm because we could not photograph smaller cells with enough detail to ensure their correct identification.
Data Analysis
Density (high, medium, low, and control) and species composition (S. compressa, C. canaliculatus, P. perforata, and mixed culture) were considered categorical and independent factors. Natural log transformations were conducted as required to satisfy the assumptions (Underwood, 1997). Normality (Shapiro-Wilk test), independence of variables (Durvin-Watson test) and homogeneity of variances (Cochran's test) were confirmed per factor and level.
The iterative effect of the two categorical factors on bulk water flow, particle retention, irradiance, and seaweed and MPB recruitment was used in an ANOVA by least mean squares at an alpha value of 0.05. Post-hoc (Tukey test) comparisons were conducted where differences were found. Also, simple and multiple regressions were performed to identify which environmental factor or combined factors resulted in significant predictors of the abundance of both groups and to determine if any correlation existed between them. Outputs and raw data of the variables measured in this manuscript are available through the figshare repository (http://figshare.com), doi: 10.6084/m9.figshare.5797137.
Results
Overall, the differences in the ability of the canopies to attenuate intertidal physical conditions seemed to influence the abundance of seaweed recruitment underneath the canopies directly. In general, higher abundances of seaweed recruits occurred underneath canopy treatments with the least attenuated physical conditions, whereas the MPB showed the lowest abundance under these conditions.
Algal species and canopy density influenced the measured physical parameters below the canopies. There was no significant effect of species composition on water bulk flow. There was, however, a significant effect of density on water flow (p < 0.001; Table 1). Plaster bars underneath the high and medium density treatments had significantly lower dissolution than the low density and control treatments (Tukey p < 0.001), suggesting there was significantly less bulk water flow underneath the canopies of higher densities (Table 2).
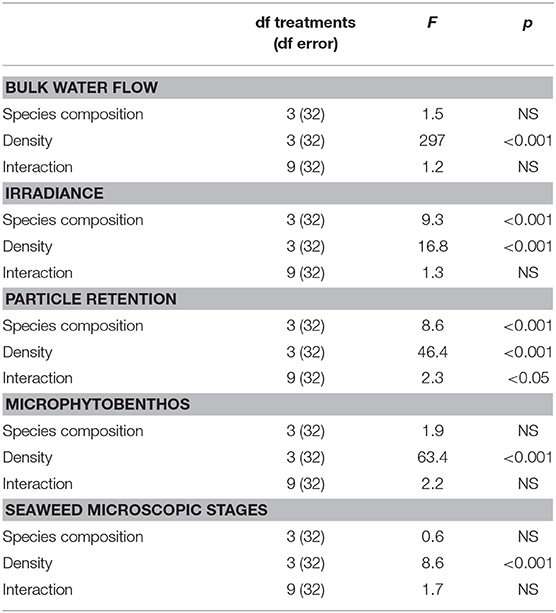
Table 1. Plaster bar erosion, irradiance, particle retention, and abundance of microphytobenthic organisms and microscopic stages of seaweeds based on species composition, density, and their interaction, using a two-factor crossed ANOVA.
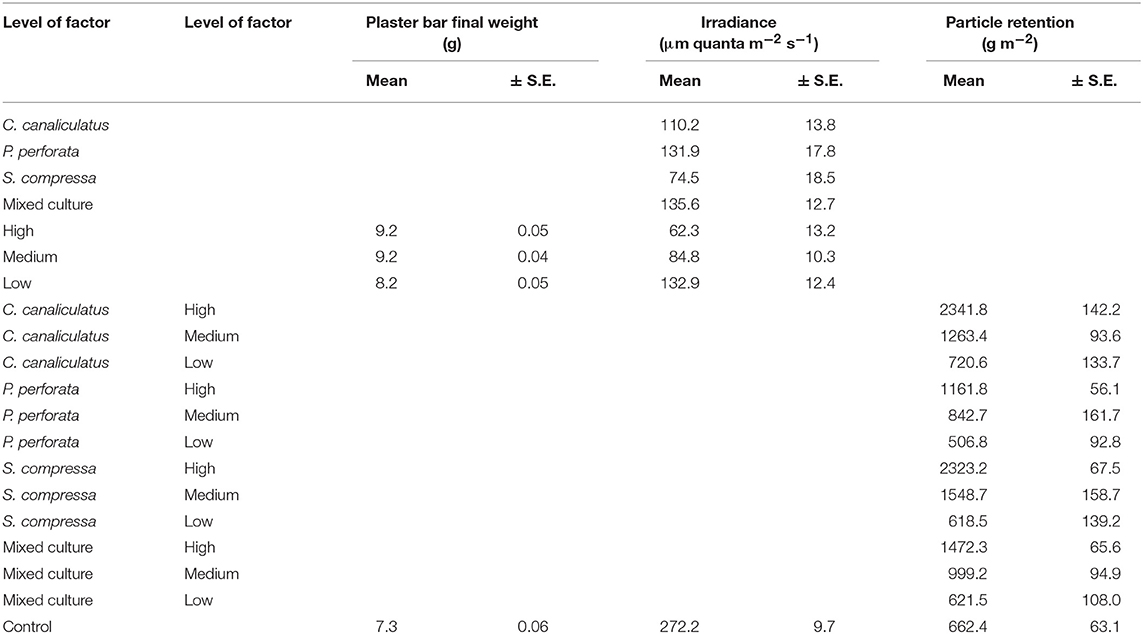
Table 2. Measures of plaster bars final weight, irradiance, and particle retention as a function of species composition, density and their interaction.
Furthermore, there was no significant interaction effect between species and density on the attenuation of irradiance below the canopies, yet there was an effect driven separately by each factor (p < 0.001; Table 1). The species Silvetia compressa and overall the high and medium density treatments attenuated irradiance the most (Tukey p < 0.05; Table 2). There was also a significant interaction between species composition and density on particle retention below the canopy (p < 0.05 Table 1). Synthetic fiber pads below the S. compressa and C. canaliculatus canopies at high densities retained more particles than other treatments (Tukey p < 0.001; Table 2).
On the other hand, only density treatments showed a significant effect on the abundance of seaweed and MPB recruitment underneath the canopies (p < 0.001, Table 1). However, both groups had contrasting distributions. Diatoms were abundant underneath higher density treatments (Figure 2), while seaweed recruits were abundant underneath lower density treatments (Figure 3).
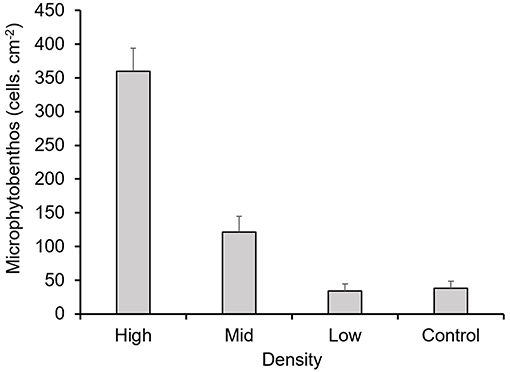
Figure 2. Abundance of microphytobenthic organisms settled underneath the experimental canopies. Mean values ± one standard error.
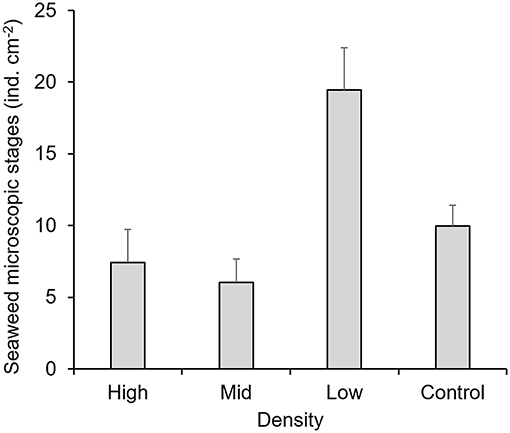
Figure 3. Abundance of microscopic stages of seaweeds settled underneath the canopy treatments. Mean values ± one standard error.
Early sporophytes of brown seaweeds were the most abundant seaweed microscopic stage found and often grew close to one another. Red algal spores were second in abundance and showed a more isolated distribution, with few to no other seaweeds or microphytobenthic organisms settled next to them (Figure 4). Conversely, benthic diatoms were the dominant organism within the MPB with Cocconeis spp. representing 89% of the settlers and often forming biofilm mats. Fewer representatives of other benthic diatoms, such as Navicula sp. (10%), Climacosphenia sp. (< 1%), and cyanobacteria, such as Chroococcus sp. (< 1%), were present at lower abundances (Figure 5).
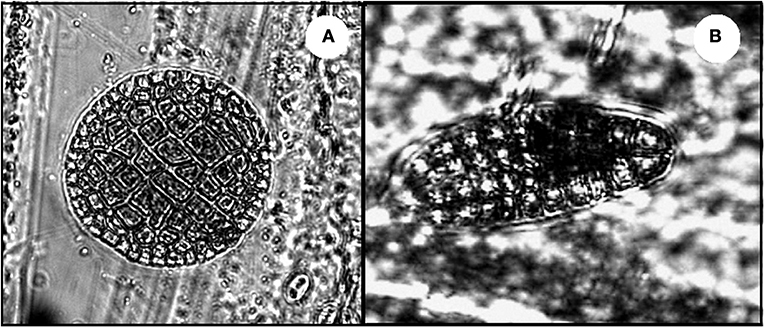
Figure 4. Microscopic stages of seaweeds settled underneath the canopies. (A) Spore of red seaweed and (B) sporophyte of brown seaweed.
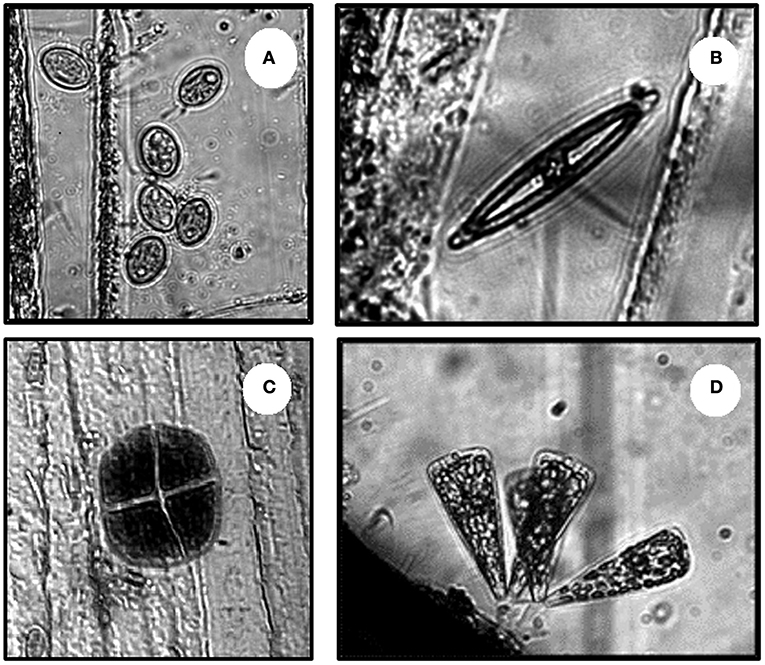
Figure 5. Microphytobenthic organisms settled underneath the canopies. (A) Cocconeis sp., (B) Navicula sp., (C) Chroococcus sp., and (D) Climacosphenia sp.
Surprisingly, none of the physical factors used in the regression analyses explained the variability in the abundance of seaweed recruits (Table 3) (r2 = 0.047, p = NS). However, the regression analyses did show that particle retention by the seaweed canopy aggregations explained 64% of the variability in the abundance of MPB cells in the understory (n = 48, p < 0.001).
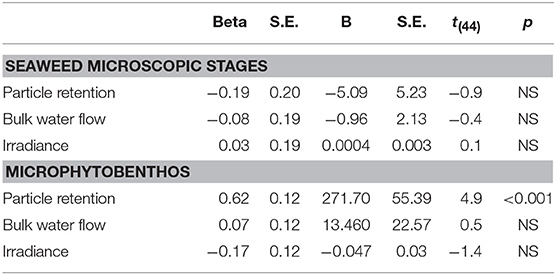
Table 3. Regression summary for the recruitment of seaweed microscopic stages (p = NS) and microphytobenthic cells (r2 = 0.64, p < 0.001) and underneath the canopy.
Discussion
Overall, our results indicate that the attenuation of physical factors driven by macroscopic seaweeds influences the abundance and distribution of seaweed and MPB recruits differently. We found a greater abundance of MPB cells underneath the most attenuated canopies and a greater abundance of seaweed settlers underneath the least attenuated canopies. In contrast to the MPB, particle retention did not appear to affect seaweed recruitment significantly. Moreover, no relationship with the physical factors measured herein explained the abundance of seaweed recruits. We neither find any relationship between the distribution patterns of MPB cells and seaweed recruits. The distribution and abundance patterns might suggest competition for space, light or nutrients, due to their apparently inverse, but not significantly related distribution, however no relationship was found to evidence this. Huang and Boney (1984) experimentally demonstrated that although MPB cells can outcompete juvenile brown and red seaweeds, both groups could also coexist with no competition between them, which seems to be the case in our study.
At least 50% of the blades in our experimental quadrats were fertile during the study period, coinciding with the reproductive periods described for these species in the region (Pacheco-Ruiz et al., 1989; García-Lepe et al., 1997; Johnson and Brawley, 1998; Zertuche-González et al., 2000). Many of the germlings or spores measured in this study could have resulted from self-seeding in our quadrats, which might be related to the relatively high abundance of brown and red seaweed microscopic stages underneath the canopies. The non-motile eggs of the fucoid seaweeds are within the largest reproductive cells among seaweeds. Although spores released from our outplants might sink relatively faster into the water than the MPB (Okuda and Neushul, 1981), thus facilitating colonization of available substrate, many could have been lost to mortality (Santelices, 1990; Hurd et al., 2014) or could have been impeded from settling by the high canopy densities of our treatments. In fact, a number of studies have reported settlement of recruits to be inhibited by previously established seaweeds; either due to the physical barrier thalli represent (McCourt, 1984), a sweeping effect (Fletcher and Callow, 1992; Johnson and Brawley, 1998), or by particular traits of the canopies (Brawley and Johnson, 1991). C. canaliculatus, for example, can form dense mats that can trap enough sediment which prevents subsequent settlement by algal spores (Sousa, 1979; Sousa et al., 1981). Moreover, studies show that seaweed recruits also respond to the chemical properties of the microenvironment. For instance, flagellated spores can detect and respond to a variety of inorganic and organic nutrients, swimming toward or away from microhabitats with nutrients at concentrations that enhance or inhibit growth (Amsler et al., 1992; Maggs and Callow, 2003; Hurd et al., 2014). It is possible that inhibitory cues by our experimental canopies could have deterred green motile spores from settling, therefore explaining their relatively low abundance in our experimental treatments.
Early colonizers play a key role in ecology because they can modify the physicochemical conditions of their environment. In turn, they can furthermodulate settlement of other organisms (Dobretsov, 2008; Orvain et al., 2015). In fact, benthic diatoms are among the first algae to settle on new substrate, and it is suggested that they may affect the settlement of later successional organisms (Amsler et al., 1992). Research shows that together, the MPB and bacteria can form biofilms that can influence the adhesion of seaweed spores by modifying the chemical characteristics of the settlement surface (Callow and Callow, 2006; Ma et al., 2010). This effect, however, is not always consistent, as the strength of adhesion depends on the species of seaweeds tested, their stage of development and the physicochemical properties of the substrate (Mieszkin et al., 2013). Although this study was repeated a year later, similarly to Umanzor et al. (2017), we found that diatoms of the genus Cocconeis almost solely colonized all understories. This result provides further evidence of the relevance of particle retention for the settlement of benthic organisms, as particle transport and deposition significantly influence their establishment, survival, and development (Eckman and Duggins, 1991; Morrow and Carpenter, 2008). Even though we only considered diatoms and cyanobacteria within these films, bacteria were also most likely abundant.
It is possible that MPB organisms were already attached to the seaweed canopies as epiphytes, and that the transfer to the seabed occurred directly underneath the high-density treatments. In contrast to seaweed propagules, the MPB can grow exponentially and cover a large area in a relatively short time, from a few founding cells (Blanchard et al., 2001), facilitating rapid colonization of new substrate. Such developmental and colonization attributes could have resulted in the differences in the abundance and distribution of the MPB and seaweed recruits measured underneath the canopies. It is also possible that grazers could have influenced the recruitment process of both the MPB and seaweed microscopic stages. Experimental studies show that grazers and filter feeders can ingest and digest algal spores, affecting the pattern of dispersal and settlement on rocky shores (Buschmann and Santelices, 1897; Eckman and Duggins, 1991). Also, Coleman et al. (2006) showed that limpet grazers affected diversity and biomass of intertidal seaweeds across a latitudinal gradient. However, in this study, we did not witness any grazers on our settlement slides. Nonetheless, our experiment did not consider any exclusion caging, therefore, selective feeding underneath the treatment canopies could have potentially influenced the outcome of the experiment, though we do believe this to be unlikely.
We conclude that although both seaweed microscopic stages and MPB cells inhabit similar microhabitats, microscale processes appear to affect their recruitment in different ways. As shown here, the presence of macroscopic seaweeds can greatly influence the settlement and distribution of a variety of benthic organisms. Microscale changes promoted by seaweeds with different morphologies and at different densities can have profound effects on the settlement of associated organisms and these effects could trigger changes at a larger scale that should not be underestimated. Although intertidal seaweed aggregations seem to modulate a contrasting response in understory microphytobenthic and seaweed recruitment, more experimental work considering longer periods and control environments is required to determine the reciprocal effects that the MPB and seaweed microscopic stages might have on each other. Determining the identity of the recruits and if there is an effect by bacterial films and chemical cues is also recommended. Complex interactions in intertidal dynamics, the small size of the target organisms, and the characteristics of the microenvironment they inhabit all make it difficult to obtain in situ measurements of the potential feedback loops between seaweeds and microphytobenthic communities. Nonetheless, the interactions between seaweed aggregations and other benthic microorganisms need further focus as the ecological effects driven by changes in the recruitment of primary producers can have significant further consequences on the dynamics of the overall ecosystem.
Author Contributions
SU was responsible for data collection and processing. LL contributed to the experimental design and writing of the manuscript. JZ-G contributed with intellectual inputs and data processing.
Funding
This project was supported by CONACYT under Grant 221662 to LL and a scholarship from CONACYT (CVU 576942) to the first author. The Coastal Complexity Crew: Towards a paradigm shift for the near-shore ocean by exploring the biophysical complexity of spatial-temporal scales in coastal productivity.
Conflict of Interest Statement
The authors declare that the research was conducted in the absence of any commercial or financial relationships that could be construed as a potential conflict of interest.
Acknowledgments
We would like to thank Dr. David Siqueiros and Alberto Galvez for their feedback in identifying and photographing the microorganisms. We also appreciate the contributions by J. Manuel Guzman and the volunteers who helped to set up the experiment in such a challenging intertidal.
References
Amsler, C. D., Reed, D. C., and Neushul, M. (1992). The microclimate inhabited by macroalgal propagules. Br. Phycol. J. 27, 253–270. doi: 10.1080/00071619200650251
Avila, M., Piel, M. I., Caceres, J. H., and Alveal, K. (2011). Cultivation of the red alga Chondracanthus chamissoi: sexual reproduction and seedling production in culture under controlled conditions. J. Appl. Phycol. 23, 529–536. doi: 10.1007/s10811-010-9628-1
Bennett, S., and Wernberg, T. (2014). Canopy facilitates seaweed recruitment on subtidal temperate reefs. J. Ecol. 102, 1462–1470. doi: 10.1111/1365-2745.12302
Bertness, M. D., Leonard, G. H., Levine, J. M., Schmidt, P. R., and Ingraham, A. O. (1999). Testing the relative contribution of positive and negative interactions in rocky intertidal communities. Ecology 80, 2711–2726.
Blanchard, G. F., Guarini, J. M., Orvain, F., and Sauriau, P. G. (2001). Dynamic behaviour of benthic microalgal biomass in intertidal mudflats. J. Exp. Mar. Bio. Ecol. 264, 85–100. doi: 10.1016/S0022-0981(01)00312-4
Brawley, S. H., and Johnson, L. E. (1991). Survival of fucoid embryos in the intertidal zone depends upon developmental stage and microhabitat. J. Phycol. 27, 179–1991.
Buschmann, A. H., and Santelices, B. (1897). Micrograzers and spore release in Iridaea laminarioides Bory (Rhodophyta: Gigartinales). J. Exp. Biol. Ecol. 108, 171–179.
Callow, J. A., and Callow, M. E. (2006). “Progress in molecular and subcellular biology: marine molecular bio- technology,” in Progress in Molecular and Subcellular Biology: Marine Molecular Bio- Technology, eds N. Fusetani and A. S. Clare (Berlin: Springer-Verlag), 141–169.
Callow, M. E., Jennings, A. R., Brennan, A. B., Seegert, C. E., Gibson, A., Wilson, L., et al. (2002). Microtopographic cues for settlement of zoospores of the green fouling alga enteromorpha. Biofouling 18, 229–236. doi: 10.1080/08927010290014908
Choi, H. G., and Norton, T. A. (2005). Competition and facilitation between germlings of Ascophyllum nodosum and Fucus vesiculosus. Mar. Biol. 147, 525–532. doi: 10.1007/s00227-005-1593-x
Coleman, R. A., Underwood, A. J., Benedetti-Cecchi, L., Aberg, P., Arenas, F., Arrontes, J., et al. (2006). A continental scale evaluation of the role of limpet grazing on rocky shores. Oecologia 147, 556–564. doi: 10.1007/s00442-005-0296-9
Crain, C. M., and Bertness, M. D. (2006). Ecosystem engineering across environmental gradients: implications for conservation and management. Bioscience 56, 211–218. doi: 10.1641/0006-3568(2006)056[0211:EEAEGI]2.0.CO;2
Dobretsov, S. (2008). “Inhibition and induction of marine biofouling by biofilms,” in Marine and Industrial Biofouling, eds H C. Flemming, P.S. Murthy, R. Venkatesan, and K. Cooksey (Berlin: Springer), 293-313. doi: 10.1007/7142_2008_10
Eckman, J. E., and Duggins, D. O. (1991). Life and death beneath macrophyte canopies: effects of understory kelps on growth rates and survival of marine, benthic suspension feeders. Oecologia 87, 473–487.
Fletcher, R. L., and Callow, M. E. (1992). The settlement, attachment and establishment of marine algal spores. Br. Phycol. J. 27, 303–329. doi: 10.1080/00071619200650281
Fong, P., Donohoe, R. M., and Zedler, J. B. (1993). Competition with macroalgae and benthic cyanobacterial mats limits phytoplankton abundance in experimental microcosms. Mar. Ecol. Prog. Ser. 100, 97–102. doi: 10.3354/meps100097
Freckelton, M. L., Nedved, B. T., and Hadfield, M. G. (2017). Induction of invertebrate larval settlement; different bacteria, different mechanisms? Sci. Rep. 7:42557. doi: 10.1038/srep42557
García-Lepe, M. G., Ballesteros-Grijalva, G., Zertuche-González, J. A., and Chee-Barragán, A. (1997). Annual variation in size and reproductive phenology of the red alga Chondracanthus canaliculatus (Harvey) Guiry at Punta San Isidro, Baja California, Mexico. Ciencias Mar. 23, 449–462.
Hardison, A. K., Canuel, E. A., Anderson, I. C., Tobias, C. R., Veuger, B., and Waters, M. N. (2013). Microphytobenthos and benthic macroalgae determine sediment organic matter composition in shallow photic sediments. Biogeosciences 10, 5571–5588. doi: 10.5194/bg-10-5571-2013
Huang, R., and Boney, A. D. (1984). Growth interactions between littoral diatoms and juvenile marine algae. J. Exp. Mar. Bio. Ecol. 81, 21–45. doi: 10.1016/0022-0981(84)90222-3
Hurd, C. L., Harrison, P. J., Bischof, K., and Lobban, C. S. (2014). Seaweed Ecology and Physiology, 2nd Edn. Cambridge: Cambridge University Press.
Johnson, L. E., and Brawley, S. H. (1998). Dispersal and recruitment of a canopy-forming intertidal alga: the relative roles of propagule availability and post-settlement processes. Oecologia 117, 517–526. doi: 10.1007/s004420050688
Knight, G. A., and Nelson, W. A. (1999). An evaluation of characters obtained from life history studies for distinguishing New Zealand Porphyra species. J. Appl. Phycol. 11, 411–419. doi: 10.1023/A:1008175226816
Komatsu, T., and Kawai, H. (1992). Measurements of time-averaged intensity of water motion with plaster balls. J. Oceanogr. 48, 353–365.
Ma, Y., Liu, P., Zhang, Y., Cao, S., Li, D., and Chen, W. (2010). Inhibition of spore germination of Ulva pertusa by the marine bacterium Pseudoalteromonas haloplanktis CI4. Acta Oceanol. Sin. 29, 69–78. doi: 10.1007/s13131-010-0009-z
Maggs, C. A., and Callow, M. E. (2003). “Algal spores,” in Encyclopedia of Life Sciences (Nature Publishing Group), 1–6. doi: 10.1038/npg.els.0000311
McCourt, R. M. (1984). Seasonal patterns of abundance, distributions and phenology in relation to growth strategies of three Sargassum species. J. Exp. Biol. Ecol. 74, 171–156.
Mieszkin, S., Callow, M. E., and Callow, J. A. (2013). Interactions between microbial biofilms and marine fouling algae: a mini review. Biofouling 29, 1097–1113. doi: 10.1080/08927014.2013.828712
Morrow, K. M., and Carpenter, R. C. (2008). Macroalgal morphology mediates particle capture by the corallimorpharian Corynactis californica. Mar. Biol. 155, 273–280. doi: 10.1007/s00227-008-1023-y
Orvain, F., Martinez, A.-S., Desoche, E., and Claquin, P. (2015). “Chemical interaction between epilitic microphytobenthic biofilm and larval development of the sea urchin Paracentrotus lividus,” in Proceedings of Congress on Artificial Reefs: From Materials to Ecosystems (Caen), 239–247.
Pacheco-Ruiz, I., García-Esquivel, Z., and Aguilar-Rosas, L. E. (1989). Spore discharge in the carragenophyte Gigartina canaliculata Harvey (Rhodophyta, Gigartinales). J. Exp. Mar. Bio. Ecol. 126, 293–299. doi: 10.1016/0022-0981(89)90194-9
Robertson, B. L. (1987). Reproductive ecology and canopy structure of Fucus spiralis L. Bot. Mar. 30, 475–482.
Santelices, B. (1990). Patterns of reproduction, dispersal and recruitment in seaweeds. Oceanogr. Mar. Biol. Ann. Rev. 28, 177–276.
Schumacher, J. F., Carman, M. L., Estes, T. G., Feinberg, A. W., Wilson, L. H., Callow, M. E., et al. (2007). Engineered antifouling microtopographies – effect of feature size, geometry, and roughness on settlement of zoospores of the green alga Ulva. Biofouling 23, 55–62. doi: 10.1080/08927010601136957
Sousa, W. P. (1979). Experimental investigations of disturbance and ecological succession in a rocky intertidal algal community. Ecol. Monogr. 49, 227–254. doi: 10.2307/1942484
Sousa, W. P., Schroeter, S. C., and Gaines, S. D. (1981). Latitudinal variation in intertidal algal community structure: the influence of grazing and vegetative propagation. Oecologia 48, 297–307. doi: 10.1007/BF00346486.
Taylor, D. I., and Schiel, D. R. (2003). Wave-related mortality in zygotes of habitat-forming algae from different exposures in southern New Zealand: the importance of “stickability”. J. Exp. Mar. Bio. Ecol. 290, 229–245. doi: 10.1016/S0022-0981(03)00094-7
Umanzor, S., Ladah, L., and Zertuche-González, J. A. (2017). The influence of species, density, and diversity of macroalgal aggregations on microphytobenthic settlement. J. Phycol. 53, 1060–1071. doi: 10.1111/jpy.12565
Umar, M. J., Mc Cook, L. J., and Price, I. R. (1998). Effects of sediment deposition on the seaweed Sargassum on a fringing coral reef. Coral Reefs 17, 169–177.
Underwood, A. (1997). Experiments in Ecology: Their Logical Design and Interpretation Using Analysis of Variance, 1st Edn. Cambridge: Cambridge University Press.
Vadas, R. L., Johnson, J. S., and Norton, T. A. (1992). Recruitment and mortality of early post-settlement stages of benthic algae. Br. Phycol. J. 27, 331–351. doi: 10.1080/00071619200650291
Watanabe, H., Ito, M., Matsumoto, A., and Arakawa, H. (2016). Effects of sediment influx on the settlement and survival of canopy-forming macrophytes. Sci. Rep. 6:18677. doi: 10.1038/srep18677
Woodin, S. A. (1991). Recruitment of infauna: positive or negative cues? Integr. Comp. Biol. 31, 797–807. doi: 10.1093/icb/31.6.797
Keywords: bioengineers, rocky intertidal, seaweed spores, sporophytes, understory settlement
Citation: Umanzor S, Ladah L and Zertuche-González JA (2018) Intertidal Seaweeds Modulate a Contrasting Response in Understory Seaweed and Microphytobenthic Early Recruitment. Front. Mar. Sci. 5:296. doi: 10.3389/fmars.2018.00296
Received: 05 February 2018; Accepted: 03 August 2018;
Published: 03 September 2018.
Edited by:
Marco Ghisalberti, University of Western Australia, AustraliaReviewed by:
Anna R. Armitage, Texas A&M University at Galveston, United StatesDavid Ian Taylor, Cawthron Institute, New Zealand
Copyright © 2018 Umanzor, Ladah and Zertuche-González. This is an open-access article distributed under the terms of the Creative Commons Attribution License (CC BY). The use, distribution or reproduction in other forums is permitted, provided the original author(s) and the copyright owner(s) are credited and that the original publication in this journal is cited, in accordance with accepted academic practice. No use, distribution or reproduction is permitted which does not comply with these terms.
*Correspondence: Lydia Ladah, bGxhZGFoQGNpY2VzZS5teA==
†Present Address: Schery Umanzor, Department of Ecology and Evolutionary Biology, University of Connecticut, Stamford, CT, United States