- 1Oceanografía Biológica, Centro de Investigación Científica y de Educación Superior de Ensenada (CICESE), Ensenada, Mexico
- 2Department of Chemistry and Biochemistry, San Diego State University, San Diego, CA, United States
Recent research has shown that in aquatic systems pairs of prokaryote and eukaryote species exercise symbiotic exchanges of metabolites that are essential for the proliferation of either species. Using dinoflagellate Lingulodinium polyedrum cultures and a factorial design, we examined its growth at different concentrations of vitamin B1 (thiamine) and B12 (cobalamin). When both vitamins were at their lowest concentrations tested, 0.033 pM of B1 and 0.053 pM of B12 the growth was limited. When axenic L. polyedrum was co-cultured with the bacterium Dinoroseobacter shibae, a known B1 and B12 producer, then L. polyedrum grew at the same rate as in culture media supplemented with B1 and B12. In the L. polyedrum vitamin—limited culture (V-L), the abundance of attached and free-living D. shibae was higher than in the vitamin—replete (V-R) culture. In the V-R and V-L co-cultures the measured particulate B12 (pB12) concentration of attached and free-living D. shibae were in the range of 4.7 × 10−19 to 3 × 10−18 and 8.4 × 10−21 to1.2 × 10−19 (mol cell−1), respectively. Without B12 or B7 (biotin) added to the culture medium of a co-culture of L. polyedrum and D. shibae, the measured dissolved B12 (dB12) concentration was more than 60 pM higher than necessary for un-limited growth rates of L. polyedrum. In the same culture we measured B7 in the L. polyedrum particulate fraction (pB7; 4.7 × 10−19 to 9.4 × 10−19 mol cell−1). We suggest that in response to the production of B1 and B12 by D. shibae to supply L. polyedrum requeriments, the latter produced B7, which is required by D. shibae, and in our culture was only produced by L. polyedrum when D. shibae was present. We propose that D. shibae can control L. polyedrum through the release of B1 and B12, and L. polyedrum can control D. shibae through the release of B7. D. shibae is also auxotroph for niacin and 4-amino-benzoic acid, not provided by the culture medium. Therefore, L. polyedrum might affect a similar control through the release of these specific compounds and organic substrate necessary for the growth of D. shibae.
Introduction
Eukaryotic phytoplankton and bacteria have developed a number of interkingdom metabolic interactions. These include growth stimulators (Ferrier et al., 2002), potential toxin inducers (Green et al., 2004, 2006), cyst inducers (Adachi et al., 2003, 2004; Mayali et al., 2007), growth inhibitors (Hare et al., 2005), algicids (Doucette et al., 1999), and chemosensors (Miller et al., 2004). The interactions also include mutualistic trade-off of soluble factors, for example, iron siderophores (Amin et al., 2009) and vitamins (Croft et al., 2005; Wagner-Döbler et al., 2010; Kazamia et al., 2012; Cruz-López and Maske, 2016). B vitamins are essential growth factors for most marine eukaryotic phytoplankton because they are required for the activity of several enzymes in central metabolism. Vitamin B12 (cobalamin; hereafter B12) is essential for the biosynthesis of deoxyriboses from riboses, the reduction and transfer of single carbon fragments in many biochemical pathways, porphyrin and methionine biosynthesis, which is dominant in eukaryotic phytoplankton (Tang et al., 2010; Sañudo-Wilhelmy et al., 2014). Vitamin B1 (thiamin; hereafter B1) plays a major role for enzymes involved in primary carbon metabolism like the Krebs and Calvin-Benson cycles, and for branch-chain amino acid metabolism, like alanine, leucine and valine (Sañudo-Wilhelmy et al., 2014). Vitamin B7 (biotin; hereafter B7) is essential for several carboxylase enzymes, including acetyl coenzyme A (CoA) carboxylase, which is involved in the fatty acid biosynthesis, and pyruvate carboxylase, which catalyzes the first step in gluconeogenesis by converting pyruvate to oxaloacetate, hence universally required (Croft et al., 2006; Tang et al., 2010; Sañudo-Wilhelmy et al., 2014). Many phytoplankton species are natural B vitamin auxotrophs, meaning they lack the biosynthetic pathways to produce B vitamins and thus must acquire them from exogenous sources. Of the phytoplankton surveyed to date, 54% are B12 auxotrophs, 27% are B1 auxotrophs, and 8% are B7 auxotrophs (Croft et al., 2005; Tang et al., 2010). Because these auxotrophs are common in natural waters, they are useful to study to determine the roles that niche partitioning and species succession play in phytoplankton blooms (Helliwell, 2017).
Although B vitamin auxotrophy is not systematically distributed across the algal lineages, in particular, dinoflagellates have evolved obligate dependence on vitamins (Croft et al., 2006; Tang et al., 2010; Helliwell et al., 2011). Dinoflagellates form part of the eukaryotic phytoplankton and contribute significantly to the primary production of coastal areas (Moustafa et al., 2010), many dinoflagellates form blooms that can represent a potential threat to coastal ecosystems, public health, economies, and fisheries (Tang et al., 2010). Tang et al. (2010) reported that of the surveyed dinoflagellate species that are involved in harmful algal bloom events, 100% required B12, 78% required B1 and 32% B7.
Recent culture studies showed that for auxotrophic dinoflagellates a minimum of 2 × 10−5-5 × 10−4 pM of B12 and from 1.4 × 10−11 to 0.55 × 10−6 nM of B1 are required for growth (Tang et al., 2010). These concentrations are typically found in coastal waters where dinoflagellates thrive (Gobler et al., 2007; Panzeca et al., 2009; Sañudo-Wilhelmy et al., 2012). During bloom conditions, the B1 and B12 concentrations are variable, from 5 to 20 pM for both vitamins; these concentrations should be sufficient to support dinoflagellate growth (Gobler et al., 2007; Koch et al., 2011).
Most experimental physiology studies related to vitamins have focused on single-vitamin limitation, for example, the role of B12 at different concentrations (reviewed in Droop, 2007; Tang et al., 2010). Droop (1974) found threshold type limitation for phosphate and B12 in phytoplankton cultures. Other authors have reported concurrent limitation of vitamins with nitrogen (Gobler et al., 2007; Koch et al., 2011; Bertrand and Allen, 2012), iron (Panzeca et al., 2006; Bertrand et al., 2007, 2011; Koch et al., 2011) or CO2 (King et al., 2011). Although Panzeca et al. (2006), reported primary and secondary production limitation by Fe, B1, and B12 in coastal communities off the Antarctic, they did not investigate the potential for co-limitation of B12 with other essential vitamins.
Heterotrophic bacteria, archaea, and marine cyanobacteria are the only known source of B vitamins (Bonnet et al., 2010; Sañudo-Wilhelmy et al., 2014; Doxey et al., 2015), although, in early studies, some phytoplankton species have also been reported to produce B vitamins (Bednar and Holm-Hansen, 1964; Carlucci and Bowes, 1970a,b; Aaronson et al., 1971, 1977). Hence dinoflagellates would have to acquire their B vitamin from the environment either through phagotrophy (Jeong et al., 2005), active uptake from the dissolved fraction (Bertrand et al., 2007; Tang et al., 2010; Kazamia et al., 2012), or episymbiosis (Croft et al., 2005; Wagner-Döbler et al., 2010; Kazamia et al., 2012; Kuo and Lin, 2013; Xie et al., 2013; Cruz-López and Maske, 2016). Little is known currently about the relative contribution of these different mechanisms to acquire the different vitamins by auxotrophic dinoflagellates.
Previously we reported Lingulodinium polyedrum vitamin auxotrophy for B1 and B12 (Cruz-López and Maske, 2016), where a high concentration of one vitamin cannot overcome the lack of the other. This research left several questions open: At very low, ecologically relevant concentrations of both vitamins, can increasing the concentration of one support increased algal growth without an increase the in the other vitamin? Also, what is provided by the dinoflagellate to the bacteria in exchange for the necessary B-vitamins? Here we report the effect of vitamin B1 and B12 under multiple vitamin concentrations on growth rate. We also report the B7 and B12 cellular quotas for L. polyedrum in co-culture with the bacterium Dinoroseobacter shibae, a well-known B1 and B12 producing bacterium. Differently from our previous work concerning a co-culture of L. polyedrum with a bacterial community, the present work did not include auxotrophic bacteria that could compete with L. polyedrum for B1 and B12.
Materials and Methods
L. polyedrum Growth Conditions
Oceanic seawater was collected off Ensenada (31.671°N, 116.693°W; Ensenada, México) treated with activated charcoal, filtered through GF/F, and 0.22-mm pore-size cartridge filter (Pall Corporation) and stored in the dark at room temperature to age for at least 2 months. Aged seawater was sparged with CO2 (5 min per 1 L of seawater), autoclaved for 15 min and then equilibrated with air. L. polyedrum strain was isolated from Venice beach California and kindly provided by Avery Tatters (University of Southern California). Xenic L. polyedrum culture was grown in L1 medium (National Center for Marine Algae and Microbiota, NCMA, Maine, USA) prepared with aged oceanic water under 12:12 h light/dark cycle at an irradiance of 100 μmol m2 s−1, and a temperature of 20°C. To make the culture axenic, L. polyedrum culture was incubated with 1 mL of antibiotic solution (Penicillin, 5,000 U; Streptomycin, 5 mg mL−1; Neomycin, 10 mg mL−1. Sigma-Aldrich, P4083-100ML) for 50 mL of culture during 24 h, rinsed with the L1 medium, and repeated three times each step. Bacterial presence in the L. polyedrum culture was checked by staining with the nucleic acid-specific stain 4′,6-diamino-2-phenylindole (DAPI,1 μg mL−1) and quantification using epifluorescence microscopy (Axioskope II plus, Carl Zeiss, Oberkochen, Germany) connected by liquid light guide to a 175W xenon arc lamp (Lambda LS, Sutter), with optical filtering (Excitation, 360 nm/Dichroic, 395 nm/Emission, >397 nm; Semrock & Zeiss) under X100 objective lens (Plan-Apochromat, Carl Zeiss). The axenic status was documented after three antibiotic rounds, and sterile medium washes when we could not detect bacteria in the culture using epifluorescence microscopy.
Assessment of L. polyedrum Vitamin B1 and B12 Limiting Concentrations
To test the vitamin B1 and B12 limiting concentrations of L. polyedrum, cultures were grown in 50 mL cell culture flasks (BD Falcon™). Cultures were acclimated by six semi-continuous transfers during 6 weeks with an initial cell concentration of 1,300 cell L−1. The medium for L. polyedrum axenic cultures was supplemented with L1 and B1, B12 vitamins (Sigma-Aldrich) using a factorial experimental design combining 1) two vitamins (B1 + B12) and five vitamin concentrations ranging from 3.33 × 10−2 to 3.33 × 101 pM of B1 and 5.25 × 10−2 to 5.25 × 101 pM of B12 (Table 1). After acclimation, each culture and corresponding vitamin amendment were transferred and grown in 15 mL glass test tubes with silicon stoppers (n = 3) and placed randomly in transparent test tube holders to minimize bias in the data due to a heterogeneous light field in the incubator or shading by other cultures tubes. Cell growth rate was monitored by first mixing the cultures with an inclined rotating test tube holder (10 rpm) and then measuring in vivo Chlorophyll a (Chl a) fluorescence using a Turner Designs 10-000 fluorometer at the midpoint of the light phase. The specific growth rate (μ) was calculated according to the equation μ = ln (N2/N1)/(t2-t1): where N1 and N2 was in vivo Chl a fluorescence in relative units (r. u.) at time 1 (t1) and time 2 (t2), respectively. To calculate μ, we used the data obtained during the exponential phase of each growth curve on days 8, 10 and 12 after inoculation. in vivo Chl a fluorescence and cell counts were well correlated (Figure S1, r2 = 0.98). The specific growth rate was adjusted to a Monod-type model of specific growth rate vs. the initial B1 and B12 concentrations in the bioassays. A least square estimate of a Michaelis-Menten type kinetic was calculated with the PRISM 6.07v software (GraphPad, CA, USA).
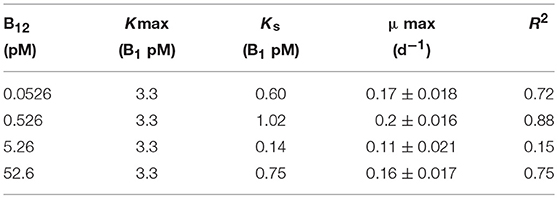
Table 1. Half-saturation constants (Ks) and maximum specific growth rates (μ) of L. polyedrum for B1 and B12 amendments.
Dinoflagellate-Bacterial Co-culture
The axenic bacterium culture D. shibae DFL-12T (NCMA, Maine, USA) was grown in oceanic seawater supplemented with yeast extract (2 g L−1) and peptone (1.25 g L−1). The co-culture was started by inoculating D. shibae cells to a final concentration of 106 cells mL−1 of an axenic, early exponential phase L. polyedrum culture in the L1 medium that was B1B7B12-supplemented (hereafter vitamin-replete, V-R) or B1B7B12 non-supplemented (hereafter vitamin-limited, V-L) medium and incubated under 12:12 h light/dark cycle, irradiance of 100 μmol m2 s−1, and a temperature of 20°C. The cultures were transferred weekly to fresh L1 medium at a ratio of 1:10 during 4 weeks before the experiment.
Sampling and Cell Fixation
All cultures were monitored for 15 d, and sampled every 2 days for cell counting by light microscopy. One milliliter of L. polyedrum cells from V-R and V-L cultures were harvested at lag (d2), exponential (d8) and stationary (d15) phase and fixed with paraformaldehyde-PBS at a final concentration of 1% for 12 h at 4°C. For attached bacteria, fixed cells were immobilized onto an 8.0 μm pore size, 25 mm-diameter Nucleopore filters (Whatman International, Ltd., Maidstone, England), and rinsed with phosphate-buffered saline (PBS, 0.1 M NaCl, 2 mM KCl, 4 mM Na2HPO4, pH 8.1; Palacios and Marín, 2008). For free-living bacteria, the fraction which passed through an 8.0 μm pore size filter was collected on 0.2 μm pore size, 25 mm-diameter Nucleopore filters (Whatman International, Ltd., Maidstone, England) and rinsed with PBS. The cells collected on the 8.0 μm filter were covered with 13 μL of low-melting-point agarose (0.05%, LMA) (BioRad, 161-3111) at 55°C, dried for 15 min at 37°C, then LMA was added again and the filter dried as previously. For all filtration steps, the pressure difference was <3.3 kPa to minimize cell damage.
Visual Observations
To visualize D. shibae cells attached to L. polyedrum cells, we used an epifluorescence microscope (Axioskope II plus, Carl Zeiss, Oberkochen, Germany; oil immersion X100 objective, Plan-Apochromat, Carl Zeiss; 175W xenon arc lamp; Lambda LS, Sutter connected through a liquid light guide) with a triple Sedat filter, a dichroic filter with three transmission bands. Excitation and emission spectra were controlled by filter wheels (Lambda 10-3, Sutter). Images were captured with a cooled CCD camera (Clara E, Andor) with 10 ms integration time. Optical stacks, 2.0 μm focal distance between images, were acquired with a computer controlled focusing stage (Focus Drive, Ludl Electronic Products, Hawthorne, NY, USA) and Micro-Manager (version 1.3.40, Vale Lab, UCSF) that controlled the filter selection and the focusing stage. The images were processed in ImageJ (Schneider et al., 2012).
Quantification of Dissolved and Particulate B12 (dB12, pB12)
For quantification of dB12 and pB12 we prepared four different fractions: 20 mL of L. polyedrum cells from V-R and V-L cultures were harvested at time zero, mid-exponential and stationary phase (n = 3). These samples were (a) pre-filtered (8.0 μm pore size, 25 mm-diameter Nuclepore filters) to harvest the fraction of pB12 of the L. polyedrum and attached D. shibae; (b) The filtrate of (a) was filtered again (0.4 μm pore size, 25 mm-diameter polycarbonate filter (Whatman International, Ltd., Maidstone, England) to recover the free-living D. shibae fraction. (c) The first filter (a) containing the L. polyedrum cells with attached D. shibae were treated with 10 mM N-acetyl cysteine (NAC; Sigma) (PBS−0.2 μM calcium chloride, 0.5 mM magnesium chloride, 15 mM glucose) for 1 h with agitation (70 rpm) at room temperature to detach adhered bacteria (Barr et al., 2013). Filtering this sample again with 0.8 μm collected L. polyedrum, cells without attached D. shibae. (d) The detached D. shibae cells were collected onto a 0.4 μm pore size, 25 mm-diameter polycarbonate filter.
Vitamin B12 was quantified by Enzyme-Linked Immunosorbent Assay, ELISA (Immunolab GmbH, B12-E01. Kassel, Germany) according to Zhu et al. (2011). These authors tested the ELISA with seawater samples and found close to 100 percent recovery of cyanocobalamin, methylcobalamin, hydroxycobalamin and coenzyme B12, but the method cannot distinguish between these different forms of B12.
For dB12, the filtered sample (0.2 μm) was pre-concentrated by solid phase (RP-C18) extraction according to Okbamichael and Sañudo-Wilhelmy (2004); the column was eluted with 5 mL methanol, the methanol was evaporated at 60°C with low vacuum, the sediment dissolved in ddH20 with a final volume of 1.5 mL, and 50 μL of the concentrate was injected into the ELISA well plate for quantification. Villegas-Mendoza et al. (in preparation) report a recovery efficiency of 85 percent using the above method for dissolved B12.
For the pB12, filters were cut for easier disintegration and transferred to bead beating tubes using 0.5 mm zirconia beads (biospec.com) with 1 mL of methanol (10:1 methanol:beads). Bead beating was conducted for 5 min followed by 5 min at −20°C, and repeated twice. The tubes were centrifuged at 5,000 rpm for 10 min and the supernatant transferred to a fresh tube. The methanol was evaporated at 60°C with vacuum, the sediment dissolved in ddH20 with a final volume of 1.5 mL, and 50 μL of the concentrate was injected into the ELISA well plate for quantification.
Quantification of Particulate B7 (pB7)
The samples were prepared for the solid extraction method described in Suffridge et al. (2017), with a slight modification. One hundred milliliters of axenic L. polyedrum culture (n = 3) was harvested at exponential phase using 8.0 μm pore size, 25 mm-diameter Nuclepore filters (Whatman International, Ltd. Maidstone, England). 100 mL of L. polyedrum + D. shibae culture (n = 3) was harvested at mid-exponential phase, and pre-filtered (a) to harvest the fraction of particulate B7 of the L. polyedrum and attached D. shibae using 8.0 μm pore size, 25 mm-diameter Nuclepore filter; (b) to collect the free-living D. shibae fraction the filtrate from (a) was filtered with 0.4 μm pore size, 25 mm-diameter polycarbonate filter (Whatman International, Ltd., Maidstone, England). The first filter (a) containing L. polyedrum cells with attached D. shibae was rinsed with 10 mL of Milli-Q water to detach adhered D. shibae. (c) Filtering this fraction again through 0.8 μm collected L. polyedrum cells without attached D. shibae. (d) The detached D. shibae cells passing through 8.0 μm filter were collected onto a 0.4 μm pore size, 25 mm-diameter polycarbonate filter.
Frozen filters containing the different fractions mentioned above were processed according to Suffridge et al. (2017) with slight modifications. In brief, frozen filters were placed in an autoclaved 1.5 mL Eppendorf tube with 1 mL of diluent (50% LC-MS grade acetonitrile, 50% water, 0.1% formic acid). The cells were lysed by repeated vortexing for 5 min followed by 1 min cooling on ice for a total period of 30 min. The cells were further sonicated for 5 min. The cell lysate was passed through 0.2 μm pore size, 25 mm-diameter polycarbonate filter (Whatman International, Ltd, Maidstone, England) to remove large particles and cell debris. The filters were washed with 0.5 mL of diluent to collect residual cell metabolites. An equal volume of chloroform was added to the filtrate; the mix was vigorously shaken for 1 min and then centrifuged for liquid phase extraction. The top aqueous phase was transferred to a new Eppendorf tube. The liquid phase extraction with chloroform was repeated twice. The residual aqueous phase was adjusted to 300–500 μL with the diluent, and then the sample was injected in triplicate in a LC-MS (Applied Biosystems API4000 SCIEX) for pB7 quantification. To obtain the efficiency of recovery, 100 ng mL−1 of B7 standard (Sigma-Aldrich-B4501) was added to three different samples.
D. shibae Frequency of Attachment on L. polyedrum
The frequency was established using the co-culture in exponential phase with modifications as follows: An initial V-R (V-R0) culture was followed for 15 d, then, the culture was transferred weakly to fresh L1 medium under semi-continuous culture conditions during 4 weeks; each consecutive culture was termed V-R1, V-R2, and V-R3. A parallel culture was started from the V-R0 culture under V-L conditions, this was termed V-L0, then the culture was transferred to a second V-L condition, and termed V-L1, and for the next transferred, add-back to a V-R condition, and termed V-L1 to V-. In each culture, a sample at mid-exponential phase was taken, and processed as described in Sampling and cell fixation, and Visual observations.
Statistical Analysis
One-way ANOVA was used to compare the specific growth rate during exponential phase at day 8 of L. polyedrum under V-R vs. V-L cultures, to compare the dB12 in V-R vs. V-L cultures, and to compare pB12 from attached and free-living D. shibae at lag, exponential and stationary phase. Since the distribution of the number of attached D. shibae on L. polyedrum cell had no Gaussian distribution, we used a Mann–Whitney test to assess the significance of the number of attached bacteria on L. polyedrum during mid-exponential phase at day 8. All analysis were performed using STATISTICA 7.1v software (Stat Soft Inc., USA).
Results
Quantitative Vitamin B1 and B12 Requirements
L. polyedrum has recently been shown to be vitamin B1 and B12 auxotroph but not to be B7 auxotroph (Cruz-López and Maske, 2016), following the pattern of the majority of the examined dinoflagellate species requiring these two vitamins (Tang et al., 2010). In a new set of batch culture experiments, we explored the growth limiting concentrations for this species using a factorial design for both vitamins. In these experiments, cultures exhibited a μmax of 0.18 ± 0.003 d−1 (n = 3) in the ranges of 0.33–3.3 pM for B1, and 0.053–0.53 pM for B12 (Figure 1). Vitamin half-saturation constants (Ks) for μmax were 3.3 pM for B1, and 0.53 pM for B12 (Table 1). Growth rates and biomass were strongly dependent on both vitamins and did not grow at concentrations of 0.033 and 0.053 pM of B1 and B12, respectively (Tables S1, S2).
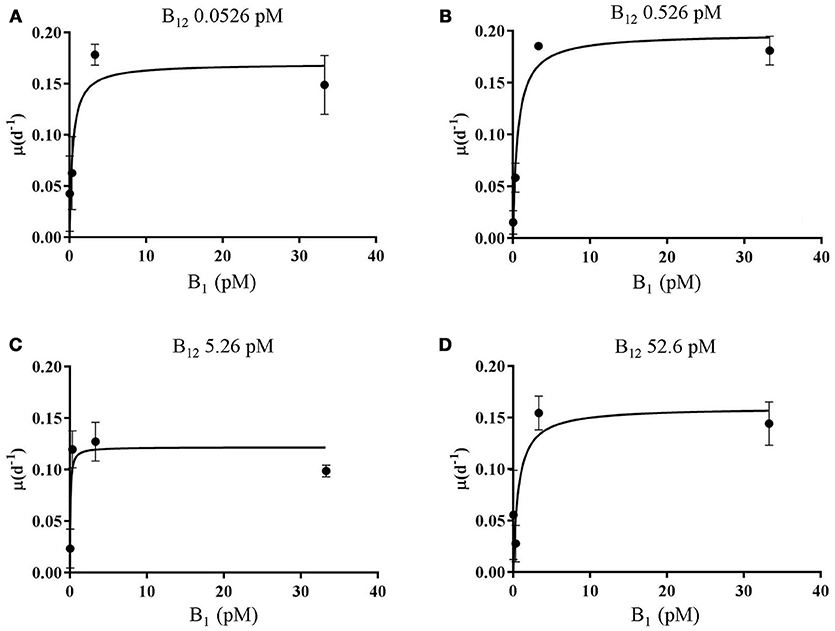
Figure 1. Vitamin B1 vs. B12 growth kinetics curve for L. polyedrum. The data plotted are from 16 (n = 3) independent experiments. L. polyedrum growth under (A) 0.0526, (B) 0.526, (C) 5.26, (D) 52.6 pM of B12, and 0.0333, 0.333, 3.33, and 33.3 pM of B1. Error bars represented one standard deviation (n = 3). A hyperbolic Michaelis–Menten model is plotted, Ks and μmax are summarized in Table 1.
dB12 and Cell Quotas of L. polyedrum and D. shibae
Dissolved B12 varied between cultures. During the lag phase, in the V-R culture, dB12 was 42.7 (±1.8) pM, whereas in the V-L culture dB12 was 29.9 (±5.3) pM. In exponential phase, in the V-R culture the dB12 was 43.1 (±8.6) pM, and in the V-L 65.3 (±24.3) pM (Table 1). During stationary phase, in the V-R culture dB12 was 37.6 (±3.1) pM, and in the V-L culture 372 (±2.4) pM. The dB12 was higher during lag phase in the V-R than the V-L culture, dB12 concentration in the V-R and V-L cultures were similar during stationary phase, but unexpectedly during exponential phase, the dB12 in the V-R culture was much lower than in the V-L culture.
The B12 cell quotas (mol cell−1) of L. polyedrum, attached and free-living D. shibae were calculated by dividing the pB12 concentration by cell abundance. Samples were taken at the lag, exponential and stationary phase of the V-R and V-L cultures. For L. polyedrum, during the lag phase, in the V-R and V-L culture, the pB12 were both the same, 2.7 × 10−17 mol cell−1, respectively (Table 2). During the exponential phase, in the V-R and V-L cultures, the cell quotas were similar at 8.9 × 10−18 (±1.05 × 10−18) and 9.9 × 10−18 (±1.1 × 10−18) mol cell−1, decreasing during the stationary phase to 5.8 × 10−18 (±5.6 × 10−19) and 5.0 × 10−18 (±7.1 × 10−19) mol cell−1 in V-R and V-L cultures, respectively. The cell quotas of L. polyedrum in both types of culture were similar and showed maximum dB12 concentrations during exponential phase.
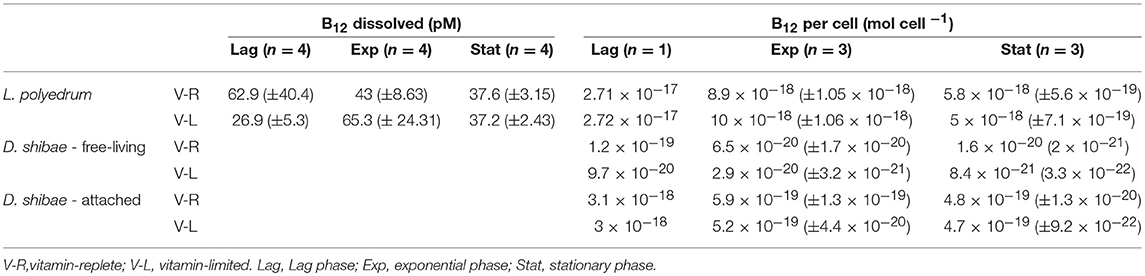
Table 2. Dissolved and B12 quotas expressed per cell (mol cell−1) for L. polyedrum and D. shibae in co-culture.
For free-living D. shibae, the estimates for the lag, exponential and stationary phase in the V-R culture, cell quotas were 1.2 × 10−19, 6.5 × 10−20 (±1.7 × 10−20) and 1.6 × 10−20 (2.1 × 10−21), and for the V-L culture, they were 9.7 × 10−20, 2.9 × 10−20 (±3.2 × 10−21) and 8.5 × 10−21 (3.3 × 10−22) mol cell−1. For the attached D. shibae, the estimates for the lag, exponential and stationary phase in V-R culture were 3.1 × 10−18, 5.9 × 10−19 (±1.3 × 10−19) and 4.9 × 10−19 (±1.3 × 10−20) mol cell−1, and for the V-L culture were 3.0 × 10−18, 5.3 × 10−19 (±4.4 × 10−20), 4.7 × 10−19 (±9.2 × 10−22) mol cell−1. The D. shibae B12 cell quotas of attached D. shibae were always much higher than those for free-living D. shibae by a factor 10 to 55 (Table 2).
B7 Cell Quotas of L. polyedrum and D. shibae
We could not detect pB7 in L. polyedrum axenic cultures, while in the xenic culture; L. polyedrum contained an average of 7.07 × 10−19 mol cell−1 of B7. As reported previously, D. shibae can thrive in two phenotypes, in attached mode or free-living; when we tried to quantify the free-living fraction, we could not detect any trace of pB7, whereas, in the attached fraction, we quantified an average of 1.8 × 10−19 (n = 2) mol cell−1 (Table 3).
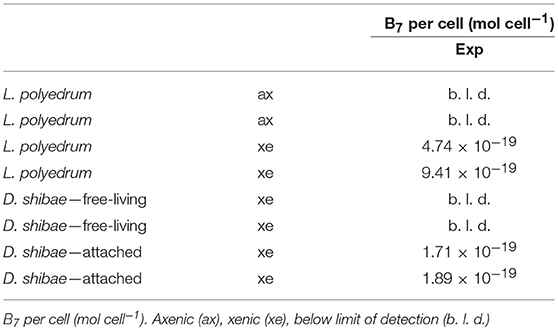
Table 3. B7 quotas expressed per cell (mol cell−1) for axenic L. polyedrum and with D. shibae in co-culture.
D. shibae Frequency of Attachment on L. polyedrum
We quantified the attachment of D. shibae to L. polyedrum cells by counting microscopically the number of attached bacteria to each L. polyedrum cell. Because 100 L. polyedrum cells were observed the frequency of bacteria per host cell in Figure 2 gives the relative frequency in percent. Figure 2 shows that in the V-R culture the average number of D. shibae bound to L. polyedrum was lower, compared with the V-L culture (p < 0.05, Mann-Whitney). In addition, the abundance of free-living D. shibae in the V-R culture was lower than in the V-L culture (Figure 3; p < 0.05, ANOVA).
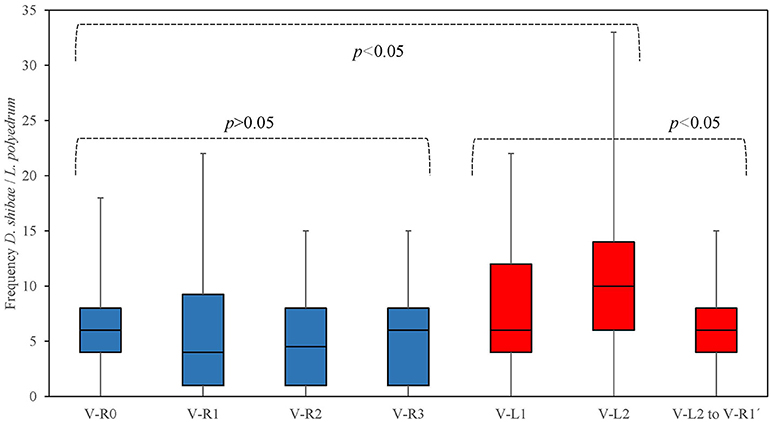
Figure 2. Frequency of D. shibae cells attached to single L. polyedrum cell in semi-continuous culture conditions. L. polyedrum in V-R culture (), and V-L culture (
).
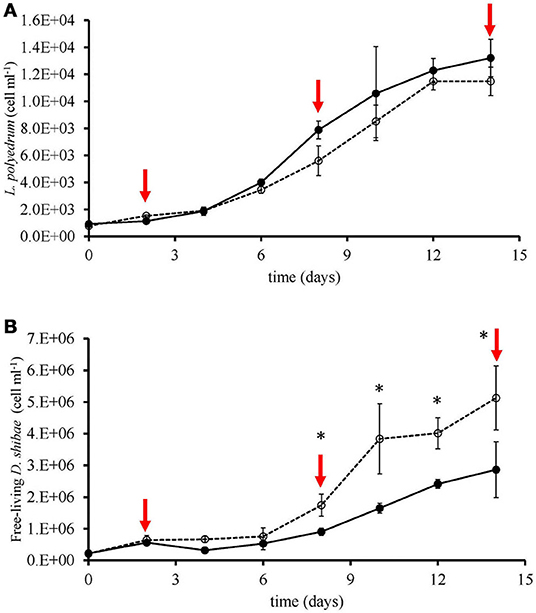
Figure 3. Growth of L. polyedrum and D. shibae under V-R (-•-) and V-L (-°-) cultures. (A) L. polyedrum abundance; red arrows denoted time-points of sampling. (B) Free-living D. shibae abundance. *p < 0.05 (ANOVA).
Discussion
Limiting Concentrations of B1 and B12
Our results show that B1 and B12 at the lowest concentrations tested limited the growth rate of L. polyedrum. The L. polyedrum strain examined in this study must possess high-affinity uptake systems for B1 and B12 to explain their low Ks values (Table 1). Our Ks estimate for B12 of 0.53 pM is in the range of values reported by Tang et al. (2010; Table 2) for six different phytoplankton species including two dinoflagellate species reported to have the lowest (0.02 pM) and highest Ks values (13.1 pM) for B12 in the list. Our Ks estimate for B1 of 3.3 pM is lower than the values reported by Tang et al. (2010; Table 2) for five species. In their list, the values of the three dinoflagellates ranged from 86 to 131 pM.
The uptake of B1 and B12 by phytoplankton communities has been documented in coastal areas (Gobler et al., 2007; Koch et al., 2011, 2012), but the potential for colimitation of B1 and B12 has not been reported. Given the slow growth rate of dinoflagellates, it is difficult to imagine that traditional bioassay experiments with natural populations probing vitamin limitation would yield conclusive results for dinoflagellates. But concurrent limitation of vitamins with other compounds have been reported (Panzeca et al., 2006; Bertrand et al., 2007, 2011; Gobler et al., 2007; King et al., 2011; Koch et al., 2011; Bertrand and Allen, 2012).
The limiting concentrations of B1 and B12 supporting maximum growth rates of L. polyedrum can be compared with measured in situ concentrations. In coastal systems dB12 ranges from undetectable to 87 pM (Sañudo-Wilhelmy et al., 2006, 2012; Panzeca et al., 2009) and for dB1 from undetectable to 200 pM (Gobler et al., 2007; Koch et al., 2012, 2013; Sañudo-Wilhelmy et al., 2012; Suffridge et al., 2017). This comparison suggests that B1 or B12 might limit the growth rate of L. polyedrum during certain stages of bloom formation. Koch et al. (2014), measured dissolved B1 and B12 concentrations inside and outside of dinoflagellate blooms, and found concentrations higher than the limiting concentrations reported in our study. They also reported that vitamin concentrations inside dinoflagellate blooms were lower than outside of blooms, which pointed to active uptake and the possibility of vitamin limitation. Further field data will have to show if the dinoflagellate bloom development can be limited by vitamin availability in coastal waters that are less eutrophic than their study area.
L. polyedrum and D. shibae Co-culture
Our experiments were planned after having shown B1 and B12 auxotrophy of L. polyedrum, including experimental evidence that co-cultured bacterial communities could overcome the vitamin limitation without supplying the bacteria with additional organic substrate in the culture medium (Cruz-López and Maske, 2016). Our previous experimental approach could not separate the vitamin auxotrophy of part of the bacterial community from the auxotrophy of L. polyedrum. In a new set of experiments, we co-cultured L. polyedrum with D. shibae, a bacterium reported to produce B1 and B12 (Wagner-Döbler et al., 2010). D. shibae has been shown to be B7 auxotroph (Biebl et al., 2005; Wang et al., 2014a) which combined well with L. polyedrum that was shown not to be B7 auxotroph (Cruz-López and Maske, 2016).
In the co-cultures, we calculated B12 cell quotas based on pB12 and cell abundances for L. polyedrum ranging from 5 × 10−18 to 2 × 10−17 mol cell−1 (Table 2). These cell quotas are comparable to 4 × 10−22 and 1.7 × 10−18, the values reported for dinoflagellates by Tang et al. (2010; Table 2). Our measured cell quotas show between one and two orders higher cellular B12 content for L. polyedrum than for both types of D. shibae phenotypes, attached and free-living. In our results, L. polyedrum had lower Ks but higher cell quotas compared with other dinoflagellate species (Tang et al., 2010); the former might provide an ecological advantage to L. polyedrum or partially compensate for the disadvantage of a high cell quota. The cell quota concentrations based on cell volumes are 2–3 orders of magnitude lower for L. polyedrum cells than for D. shibae cells (Table 1). Panzeca et al. (2009) quantified the concentration of B12 during a L. polyedrum bloom in a coastal, ranging from 2 to 61 pM, concentrations that should not be limiting for L. polyedrum. L. polyedrum abundance was not quantified at the time of B12 measurements, but at nearby locations at a later date the abundance was shown to be high, ranging from 1 × 106 to 6 × 106 (cell L−1) (Peña-Manjarrez et al., 2009).
According to Croft et al. (2005), a minimum of 10 ng L−1 of B12 is required for growth in culture by most phytoplankton species, while Tang et al. (2010) showed that HAB species need higher concentrations than non-bloom forming species. Most of the B12 quantification in the field or in culture studies measured the dissolved form, and only few studies related to dinoflagellates in the field (Carlucci, 1970; Gobler et al., 2007; Panzeca et al., 2009) or in cultures (Croft et al., 2005; Tang et al., 2010; Cruz-López and Maske, 2016). Recently Suffridge et al. (2017), quantified the particulate fraction for several B-type vitamins in the field and cultured marine bacteria, given previous cell quotas for marine phytoplankton or heterotrophic bacteria are based on uptake rates (reviewed in Droop, 2003; Bonnet et al., 2010).
To quantify the B12 in the different fractions, we used a highly sensitive enzyme-linked immunosorbent assay (ELISA) able to recognize all B12 forms (cyanocobalamin, methylcobalamin, hydroxocobalamin, and coenzyme B12), but unable to distinguish them individually. The cyanocobalamin produced by D. shibae can be used directly by the algal auxotroph without post-modifications (Helliwell et al., 2016). A likely explanation for our co-culture experimental results is that L. polyedrum acquired B1 and B12 vitamins from D. shibae in sufficient quantity to sustain the maximum growth over many generations; however, it had not been shown what D. shibae receives in return from L. polyedrum. For D. shibae to grow together with L. polyedrum, it would need organic substrate for biomass formation but in addition also specific compounds because D. shibae is auxotroph for B7, niacin and 4-amino-benzoic acid (Biebl et al., 2005). The production and release of niacin and 4-amino-benzoic acid by a dinoflagellate host was previously suggested by Wagner-Döbler et al. (2010) and Wang et al. (2014a) to explain their co-cultures of Prorocentrum minimum CCMP1329 with D. shibae in medium lacking B12 or any of the other essential vitamins mentioned above. However, the dinoflagellate P. minimum CCMP1329 is B7 auxotroph (Wagner-Döbler et al., 2010) and therefore B7 had to be supplied to the medium. In the case of L. polyedrum, B7 is not required (Cruz-López and Maske, 2016) and the culture medium was not supplemented with B7, niacin or 4-amino-benzoic acid. We assume that these organic growth factors could not be provided by the prepared seawater medium. We suggest that these three compounds were provided by L. polyedrum in the form of exudates in sufficient quantity to support the growth of the D. shibae population. In return, L. polyedrum thus promoted the production of B1 and B12. Due to technical limitations, we only measured B7 in the particulate fraction, but not in the dissolved fraction. Future research should quantify B7, niacin or 4-amino-benzoic acid in the dissolved fraction to better define the mutualistic relationship between D. shibae and L. polyedrum (Figure 4).
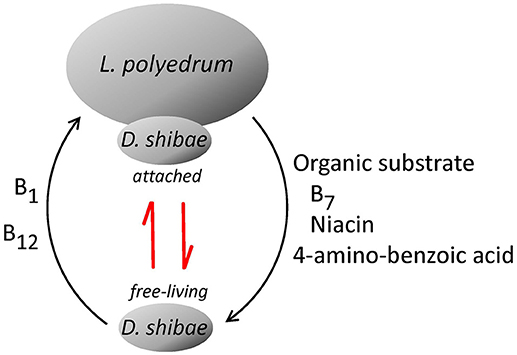
Figure 4. Proposed mutualism of L. polyedrum and D. shibae. Depending on the degree of B1 and B12 limitation L. polyedrum liberates growth promoters for D. shibae, for example, B7, niacin and 4-amino-benzoic acid. D. shibae responds with an increased tendency to attach to L. polyedrum cells and to produce B1 and B12. The red arrows indicate the kinetic between free-living and attached lifestyle of D. shibae based on the dissolved pool of B1 and B12.
On the other hand, the release of B7 from different phytoplankton species has been documented previously (Bednar and Holm-Hansen, 1964; Carlucci and Bowes, 1970a,b; Aaronson et al., 1971, 1977), cell quotas for dinoflagellates are based on uptake rates (Tang et al., 2010). Our pB7 results showed no pB7 in the L. polyedrum axenic culture, whereas in co-culture with D. shibae, L. polyedrum we found from 4.74 × 10−19 to 9.41 × 10−19 mol cell−1. Adjusting to pmol cell−1, our results are in the range (4.74 × 10−7 to 9.41 × 10−7) for the cell quotas reported for Gymnodinium instriatum L3, and two orders of magnitude higher than those reported for P. minimum CCMP696 and P. minimum PB3 (Tang et al., 2010).
For marine heterotrophic bacteria, the B7 production has been inferred, based on their available genomes, or in situ transcriptional studies (Luo and Moran, 2014; Sañudo-Wilhelmy et al., 2014; Gómez-Consarnau et al., 2018). Among the Roseobacter clade, of the 52 Roseobacter genomes analyzed by Luo and Moran (2014), 30 species were found to be auxotrophs for B7, including D. shibae (Biebl et al., 2005; Wienhausen et al., 2017; Gómez-Consarnau et al., 2018). Of these 30 Roseobacter species, 13 produce B1 and B12, and 17 only B12 (Table 4). In the case of D. shibae in co-culture with L. polyedrum, we could only detect B7 in the attached D. shibae phenotype, with cell quotas ranging from 1.72 × 10−7 to 1.9 × 10−7 pmol cell−1.
In a recent study, Suffridge et al. (2017), developed a method to quantify B-type vitamins in the dissolved and particulate fraction using cultures of marine bacteria. They obtained cell quotas of B7 for Synechococcus strain CC9311 (9.9 × 10−6 ± 8.1 × 10−6 mol cell−1), and for Vibrio AND4 (2.47 × 10−6 ± 1.36 × 10−6 mol cell−1). In our cultures, surprisingly the free-living D. shibae did not contain pB7. As was shown by Luo and Moran (2014), the B7 auxotrophy is a widespread phenomenon among the Roseobacter clade, although this auxotrophy is not always related to a symbiotic lifestyle with phytoplankton, which needs to be clarified with further culture and genomic studies.
D. shibae Frequency of Attachment on L. polyedrum
Eukaryotic phytoplankton and heterotrophic bacteria are interacting to fulfill the need for vitamins of the former and the need of excreted organic substrate of the latter. Different modes of exchange of vitamins and organic substrate are possible; vitamin-producing bacterial epibionts can be attached to the vitamin-auxotroph cells, or the vitamin-producing and consuming cells can be freely suspended, and the exchange of the dissolved compounds is maintained by turbulent and molecular diffusion at sufficiently high rates to supply the metabolic demand of the auxotrophs (Croft et al., 2005; Droop, 2007).
We used vitamin-replete and vitamin-limited cultures of L. polyedrum to produce contrasting host-bacterial culture conditions assuming that the V-L condition would promote the cellular attachment of bacteria and host. The frequency of D. shibae attached to L. polyedrum was higher under V-L condition compared to V-R condition (Figure 2; Table S3; Mann-Whitney, p < 0.005). When V-L cultures returned to V-R condition, the frequency of attachment reduced to average abundance. Higher attachment frequency under V-L condition suggests a more intense exchange of metabolites under growth culture conditions where this exchange would be essential to the growth of either population.
Because no carbon substrates were added to the culture medium that could have supported the growth of D. shibae, our results suggest that D. shibae was able to use dinoflagellate photosynthates as a carbon source in return for supplying the host with vitamin B1 and B12. As pointed out above L. polyedrum might have provided more specific growth enhancing substrates to D. shibae. Although in media without added vitamin D. shibae was found to be attached more frequently to L. polyedrum cells suggesting a synergy between both species, it leaves the question open about the mechanism of delivery. Vitamin could have either been exchanged in the dissolved form originating from free-living partners or by a more direct exchange of metabolites when both cell types are physically attached. Interestingly, in similar experiments but with a natural community of microbes instead of D. shibae, the frequency of attachment of microbes to L. polyedrum did not increase but the abundance of free-living bacteria in the co-culture increased (Cruz-López and Maske, 2016). For co-cultures of L. polyedrum with bacterial communities, the vitamin exchange and balance is more difficult to analyze because an unknown part of the bacterial community is also B12 auxotroph.
During the co-culture of L. polyedrum and D. shibae, we quantified the free-living and attached D. shibae cells in V-R and V-L cultures, and observed that the abundance of free-living D. shibae in the V-R culture was lower than in V-L culture (Figure 3B; ANOVA, p > 0.05), and that L. polyedrum in the V-R culture was lightly colonized in comparison with the V-L culture (Figure 2; Table S3; Mann-Whitney, p < 0.005). These two alternative lifestyles of D. shibae have been documented before (Biebl et al., 2005; Wagner-Döbler et al., 2010; Wang et al., 2014a), and are controlled by quorum sensing (QS) via CtrA signal transduction protein (Patzel et al., 2013; Wang et al., 2014b). Note, however, that increased bacterial attachment frequency under vitamin limitation coincided with an increased abundance of free-living bacteria, further work will be required to confirm that this phenotype is driven by nutrient exchange requirements.
Conclusion
Our data show that the B1 and B12 limiting concentrations for L. polyedrum growth are lower than typical concentrations found in coastal waters. In vitamin-depleted cultures of L. polyedrum, the limitation could be overcome by co-culture with D. shibae, because the bacterium was likely able to provide sufficient quantities of B1 and B12 to support the growth of L. polyedrum. In return, D. shibae was likely provided sufficient exudates by L. polyedrum to support its growth. Also, the mutualism between both partners may have extended beyond the supply of B1 and B12 and dissolved organic carbon. Since D. shibae is auxotrophic for B7, niacin and 4-amino-benzoic acid, L. polyedrum needed to provide sufficient quantities of these compounds to overcome D. shibae's auxotrophy. This mutualism was probably helped by the phenotypic ability of D. shibae to choose between a free-living or attached lifestyle, where the attached lifestyle could serve as a reservoir of B7 within this isogenic population.
Author Contributions
RC-L conceived, performed, and wrote the article. HM conceived and wrote the article. KY and NH performed and wrote the article.
Conflict of Interest Statement
The authors declare that the research was conducted in the absence of any commercial or financial relationships that could be construed as a potential conflict of interest.
Acknowledgments
This work was partially supported by the National Council of Science and Technology (CONACyT-México) project CB-2008-01 106003 (to HM), a Ph.D. scholarship, and a Postdoctoral fellowship for overseas to RCL (CONACyT-México). Additionally, we would like to thank Avery O. Tatters (University of Southern California) for kindly providing the L. polyedrum strain and Prof. Carl J. Carrano (San Diego State University) for allowing us to use his laboratory to prepare samples. We would like to show our gratitude to Sharon and Christopher A. Rhodes, the owners of Drug Delivery Experts LLC., for allowing us to use their facility to generate LC-MS data. Finally, we would like to thank the reviewers for constructive comments and suggestions.
Supplementary Material
The Supplementary Material for this article can be found online at: https://www.frontiersin.org/articles/10.3389/fmars.2018.00274/full#supplementary-material
References
Aaronson, S., DeAngelis, B., Frank, O., and Baker, H. (1971). Secretion of vitamins and amino acids into the environment by Ochromonas danica. J. Phycol. 7, 215–218.
Aaronson, S., Dhawale, S. W., and Patni, N. J. (1977). The cell content and secretion of water-soluble vitamins by several freshwater algae. Arch. Microbiol. 112, 57–59. doi: 10.1007/BF00446654
Adachi, M., Kanno, T., Okamoto, R., Itakura, S., Yamaguchi, M., and Nishijima, T. (2003). Population structure of Alexandrium (Dinophyceae) cyst formation-promoting bacteria in Hiroshima Bay, Japan. Appl. Environ. Microbiol. 69, 6560–6568. doi: 10.1128/AEM.69.11.6560-6568.2003
Adachi, M., Kanno, T., Okamoto, R., Shinozaki, A., Fujikawa-Adachi, K., and Nishijima, T. (2004). Jannaschia cystaugens sp. nov., an Alexandrium (Dinophyceae) cyst-formation-promoting bacterium from Hiroshima Bay, Japan. Int. J. Syst. Evol. Microbiol. 54, 1687–1692. doi: 10.1099/ijs.0.03029-0
Amin, S. A., Green, D. H., Hart, M. C., Küpper, F. C., Sunda, W. G., and Carrano, C. J. (2009). Photolysis of iron-siderophore chelates promotes bacterial-algal mutualism. Proc. Natl. Acad. Sci. U.S.A. 106, 17071–17076. doi: 10.1073/pnas.0905512106
Barr, J. J., Auro, R., Furlan, M., Whiteson, K. L., Erb, M. L., Pogliano, J., et al. (2013). Bacteriophage adhering to mucus provide a non-host-derived immunity. Proc. Natl. Acad. Sci. U.S.A. 110, 10771–10776. doi: 10.1073/pnas.1305923110
Bednar, T. W., and Holm-Hansen, O. (1964). Biotin liberation by the lichen alga Coccomyxa sp. and by Chlorella pyrenoidosa. Plant Cell Physiol. 5, 297–303.
Bertrand, E. M., and Allen, A. E. (2012). Influence of vitamin B auxotrophy on nitrogen metabolism in eukaryotic phytoplankton. Front. Microbiol. 3:375. doi: 10.3389/fmicb.2012.00375
Bertrand, E. M., Saito, M. A., Jeon, Y. J., and Neilan, B. A. (2011). Vitamin B12 biosynthesis gene diversity in the ross sea: the identification of a new group of putative polar B12 biosynthesizers. Environ. Microbiol. 13, 1285–1298. doi: 10.1111/j.1462-2920.2011.02428.x
Bertrand, E. M., Saito, M. A., Rose, J. M., Riesselman, C. R., Lohan, M. C., Noble, A. E., et al. (2007). Vitamin B12 and iron co-limitation of phytoplankton growth in the Ross Sea. Limnol. Oceanogr. 52, 1079–1093. doi: 10.4319/lo.2007.52.3.1079
Biebl, H., Allgaier, M., Tindall, B. J., Koblizek, M., Lünsdorf, H., Pukall, R., et al. (2005). Dinoroseobacter shibae gen. nov., sp. nov., a new aerobic phototrophic bacterium isolated from dinoflagellates. Int. J. Syst. Evol. Microbiol. 55, 1089–1096. doi: 10.1099/ijs.0.63511-0
Bonnet, S., Webb, E. A., Panzeca, C., Karl, D., Capone, D. G., and Sañudo-Wilhelmy, S. A. (2010). Vitamin B12 excretion by cultures of the marine cyanobacteria Crocosphaera and Synechococcus. Limnol. Oceanogr. 55, 1959–1964. doi: 10.4319/lo.2010.55.5.1959
Carlucci, A. F. (1970). “Part, I. I. Vitamin B12, thiamine, biotin,” in The Ecology of the Phytoplankton Off La Jolla, ed J. D. H. Strickland (Berkeley, CA: University of California Press), 23–31.
Carlucci, A. F., and Bowes, P. M. (1970a). Production of vitamin B12, thiamine, and biotin by phytoplankton. J. Phycol. 6, 351–357.
Carlucci, A. F., and Bowes, P. M. (1970b). Vitamin production and utilization by phytoplankton in mixed culture. J. Phycol. 6, 393–400.
Croft, M. T., Lawrence, A. D., Raux-Deery, E., Warren, M. J., and Smith, A. G. (2005). Algae acquire vitamin B12 through a symbiotic relationship with bacteria. Nature 438, 90–93. doi: 10.1038/nature04056
Croft, M. T., Warren, M. J., and Smith, A. G. (2006). Algae need their vitamins. Euk. Cell 5, 1175–1183. doi: 10.1128/ec.00097-06
Cruz-López, R., and Maske, H. (2016). The vitamin B1 and B12 required by the marine dinoflagellate Lingulodinium polyedrum can be provided by its associated bacterial community in culture. Front. Microbiol. 7:560. doi: 10.3389/fmicb.2016.00560
Doucette, G. J., McGovern, E. R., and Babinchak, J. A. (1999). Algicidal bacteria against Gymnodinium breve (Dynophyceae). I. Bacterial isolation and characterization of killing activity. J. Phycol. 35, 1447–1454. doi: 10.1046/j.1529-8817.1999.3561447.x
Doxey, A. C., Kurtz, D. A., Lynch, M. D. J., Sauder, L. A., and Neufeld, J. D. (2015). Aquatic metagenomes implicate Thaumarchaeota in global cobalamin production. ISME J. 9, 461–471. doi: 10.1038/ismej.2014.142
Droop, M. R. (1974). The nutrient status of algal cells in continuous culture. J. Mar. Biol. Ass. U.K. 54, 825–855. doi: 10.1017/S002531540005760X
Droop, M. R. (2003). In defence of the cell quota model of micro-algal growth. J. Plankton Res. 25, 103–107. doi: 10.1093/plankt/25.1.103
Droop, M. R. (2007). Vitamins, phytoplankton and bacteria: symbiosis or scavenging? J. Plankton Res. 29, 107–113. doi: 10.1093/plankt/fbm009
Ferrier, M., Martiny, J. L., and Rooney-Varga, J. N. (2002). Stimulation of Alexandrium fundyense growth by bacterial assemblages from the Bay of Fundy. J. Appl. Microb. 92, 706–716. doi: 10.1046/j.1365-2672.2002.01576.x
Gobler, C. J., Norman, C., Panzeca, C., Taylor, G. T., and Sañudo-Wilhelmy, S. A. (2007). Effect of B-vitamins (B1, B12) and inorganic nutrients on algal bloom dynamics in a coastal ecosystem. Aquat. Microb. Ecol. 49, 181–194. doi: 10.3354/ame01132
Gómez-Consarnau, L., Sachdeva, R., Gifford, S. M., Cutter, L. S., Fuhrman, J. A., Sañudo-Wilhelmy, S. A., et al. (2018). Mosaic patterns of B-vitamin synthesis and utilization in a natural marine microbial community. Environ. Microbiol. doi: 10.1111/1462-2920.14133. [Epub ahead of print].
Green, D. H., Bowman, J. P., Smith, E. A., Gutierrez, T., and Bolch, C. J. (2006). Marinobacter algicola sp. nov., isolated from laboratory cultures of paralytic shellfish toxin-producing dinoflagellates. Int. J. Syst. Evol. Microbiol. 56, 523–527. doi: 10.1099/ijs.0.63447-0
Green, D. H., Llewellyn, L. E., Negri, A. P., Blackburn, S. I., and Bolch, C. J. S. (2004). Phylogenetic and functional diversity of the cultivable bacterial community associated with the paralytic shellfish poisoning dinoflagellate Gymnodinium catenatum. FEMS. Microbiol. Ecol. 46, 345–357. doi: 10.1016/S0168-6496(03)00298-8
Hare, C. E., Demir, E., Coyne, K. J., Cary, S. C., Kirchman, D. L., and Hutchins, D. A. (2005). A bacterium that inhibits the growth of Pfiesteria piscicida and other dinoflagellates. Harm. Alg. 4, 221–234. doi: 10.1016/j.hal.2004.03.001
Helliwell, K. E. (2017). The roles of B vitamins in phytoplankton nutrition: new perspectives and prospects. New Phytol. 216, 62–68. doi: 10.1111/nph.14669
Helliwell, K. E., Lawrence, A. D., Holzer, A., Kudahl, U. J., Sasso, S., Kräutler, B., et al. (2016). Cyanobacteria and eukaryotic algae use different chemical variants of vitamin B12. Curr. Biol. 26, 999–1008. doi: 10.1016/j.cub.2016.02.041
Helliwell, K. E., Wheeler, G. L., Leptos, K. C., Goldsteins, R. E., and Smith, A. G. (2011). Insights into the evolution of vitamin B12 auxotrophy from sequenced algal genomes. Mol. Biol. Evol. 28, 2921–2933. doi: 10.1093/molbev/msr124
Jeong, H. J., Yoo, Y. D., Park, J. Y., Song, J. Y., Kim, S. T., Lee, S. H., et al. (2005). Feeding by phototrophic red-tide dinoflagellates: five species newly revealed and six species previously known to be mixotrophic. Aquat. Microb. Ecol. 40, 133–150. doi: 10.3354/ame040133
Kazamia, E., Czesnick, H., Nguyen, T. T., Croft, M. T., Sherwood, E., Sasso, S., et al. (2012). Mutualistic interactions between vitamin B(12)-dependent algae and heterotrophic bacteria exhibit regulation. Environ. Microbiol. 14, 1466–1476. doi: 10.1111/j.1462-2920.2012.02733.x
King, A. L., Sañudo-Wilhelmy, S. A., Leblanc, K., Hutchins, D. A., and Fu, F. (2011). CO2 and vitamin B12 interactions determine bioactive trace metal requirements of a subarctic Pacific diatom. ISME J. 5, 1388–1396. doi: 10.1038/ismej.2010.211
Koch, F., Burson, A., Tang, Y. Z., Collier, J. L., Fisher, N. S., Sañudo-Wilhelmy, S., et al. (2014). Alteration of plankton communities and biogeochemical cycles by harmful Cochlodinium polykrikoides (Dinophyceae) blooms. Harm. Alg. 33, 41–54. doi: 10.1016/j.hal.2014.01.003
Koch, F., Hattenrath-Lehmann, T. K., Goleski, J. A., Sañudo-Wilhelmy, S., Fisher, N. S., and Gobler, C. J. (2012). Vitamin B1 and B12 uptake and cycling by plankton communities in coastal ecosystems. Front. Microbiol. 3:363. doi: 10.3389/fmicb.2012.00363
Koch, F., Marcoval, A., Panzeca, C., Bruland, K. W., Sañudo-Wilhelmy, S. A., and Gobler, C. J. (2011). The effects of vitamin B12 on phytoplankton growth and community structure in the Gulf of Alaska. Limnol. Oceanogr. 3, 1023–1034. doi: 10.4319/lo.2011.56.3.1023
Koch, F., Sañudo-Wilhelmy, S. A., Fisher, N. S., and Gobler, C. J. (2013). Effect of vitamins B1 and B12 on bloom dynamics of the harmful brown tide alga, Aureococcus anophagefferens (Pelagophyceae). Limnol. Oceanogr. 58, 1761–1774. doi: 10.4319/lo.2013.58.5.1761
Kuo, R. C., and Lin, S. (2013). Ectobiotic and endobiotic bacteria associated with Eutreptiella sp. isolated from Long Island sound. Protist 164, 60–74. doi: 10.1016/j.protis.2012.08.004
Luo, H., and Moran, M. A. (2014). Evolutionary ecology of the marine Roseobacter clade. Microbiol. Mol. Biol. Biol. Rev. 78, 573–587. doi: 10.1128/MMBR.00020-14
Mayali, X., Franks, P. J. S., and Azam, F. (2007). Bacterial induction of temporary cyst formation by the dinoflagellate Lingulodinium polyedrum. Aquat. Microb. Ecol. 50, 51–62. doi: 10.3354/ame01143
Miller, T. R., Hnilicka, K., Dziedzic, A., Desplats, P., and Belas, R. (2004). Chemotaxis of Silicibacter sp. strain TM1040 toward dinoflagellate products. Appl. Environ. Microbiol. 70, 4692–4701. doi: 10.1128/AEM.70.8.4692-4701.2004
Moustafa, A., Evans, A. N., Kulis, D. M., Hackett, J. D., Erdner, D. L., Anderson, D. M., et al. (2010). Transcriptome profiling of a toxic dinoflagellate reveals a gene-rich protist and a potential impact on gene expression due to bacterial presence. PLoS ONE 5:e9688. doi: 10.1371/journal.pone.0009688
Okbamichael, M., and Sañudo-Wilhelmy, S. A. (2004). A new method for the determination of vitamin B12 in seawater. Anal. Chim. Acta 517, 33–38. doi: 10.1016/j.aca.2004.05.020
Palacios, L., and Marín, M. (2008). Enzymatic permeabilization of the thecate dinoflagellate Alexandrium minutum (Dinophyceae) yields detection of intracellularly associated bacteria via catalyzed reporter deposition-fluorescence in situ hybridization. Appl. Environ. Microbiol. 74, 2244–2247. doi: 10.1128/AEM.01144-07
Panzeca, C., Beck, A. J., Tovar-Sanchez, A., Segovia-Zavala, J., Taylor, G. T., Gobler, C. J., et al. (2009). Distribution of dissolved vitamin B12 and Co in coastal and open-ocean environments. Est. Coast. Shelf Sci. 85, 223–230. doi: 10.1016/j.ecss.2009.08.016
Panzeca, C., Tovar-Sanchez, A., Agusti, S., Reche, I., Duarte, C. M., Taylor, G. T., et al. (2006). B vitamins as regulators of phytoplankton dynamics. EOS Trans. Am. Geoph. Union 87, 593–596. doi: 10.1029/2006EO520001
Patzel, D., Wang, H., Buchholz, I., Rohde, M., Gröbe, L., Pradella, S., et al. (2013). You are what you talk: quorum sensing induces individual morphologies and cell division modes in Dinoroseobacter shibae. ISME J. 7, 2274–2286. doi: 10.1038/ismej.2013.107
Peña-Manjarrez, J. L., Gaxiola-Castro, G., and Helenes-Escamilla, J. (2009). Environmental factors influencing the variability of Lingulodnium polyedrum and Scrippsiella trochoidea (Dinophyceae) cyst production. Ciencias Marinas 35, 1–14. doi: 10.7773/cm.v35i1.1406
Sañudo-Wilhelmy, S. A., Cutter, L. S., Durazo, R., Smail, E. A., Gómez-Consarnau, L., Webb, E. A., et al. (2012). Multiple B-vitamin depletion in large areas of the coastal ocean. Proc. Natl. Acad. Sci. U.S.A. 109, 14041–14045. doi: 10.1073/pnas.1208755109
Sañudo-Wilhelmy, S. A., Gobler, C. J., Okbamichael, M., and Taylor, G. T. (2006). Regulation of phytoplankton dynamics by vitamin B12. Geophys. Res. Lett. 33:L04604. doi: 10.1029/2005GL025046
Sañudo-Wilhelmy, S. A., Gómez-Consarnau, L., Suffridge, C., and Webb, E. A. (2014). The role of B vitamins in marine biogeochemistry. Annu. Rev. Mar. Sci. 6, 339–367. doi: 10.1146/annurev-marine-120710-100912
Schneider, C. A., Rasband, W. S., and Eliceiri, K. W. (2012). NIH Image to ImageJ: 25 years of image analysis. Nat. Methods 9, 671–675. doi: 10.1038/nmeth.2089
Suffridge, C., Cutter, L., and Sañudo-Wilhelmy, S. A. (2017). A New analytical method for direct measurement of particulate and dissolved B-vitamins and their congeners in seawater. Front. Mar. Sci. 4:11. doi: 10.3389/fmars.2017.00011
Tang, Y. Z., Koch, F., and Gobler, C. J. (2010). Most harmful algal bloom species are vitamin B1 and B12 auxotrophs. Proc. Natl. Acad. Sci. U.S.A. 107, 20756–20761. doi: 10.1073/pnas.1009566107
Wagner-Döbler, I., Ballhausen, B., Berger, M., Brinkhoff, T., Buchholz, I., Bunk, B., et al. (2010). The complete genome sequence of the algal symbiont Dinoroseobacter shibae: a hitchhiker's guide to life in the sea. ISME J. 4, 61–77. doi: 10.1038/ismej.2009.94
Wang, H., Tomasch, J., Jarek, M., and Wagner-Döbler, I. (2014a). A dual-species co-cultivation system to study the interactions between Roseobacters and dinoflagellates. Front. Microbiol. 5:311. doi: 10.3389/fmicb.2014.00311
Wang, H., Ziesche, L., Frank, O., Michael, V., Martin, M., Petersen, J., et al. (2014b). The CtrA phosphorelay integrates differentiation and communication in the marine alphaproteobacterium Dinoroseobacter shibae. BMC Genomics 15:130. doi: 10.1186/1471-2164-15-130
Wienhausen, G., Noriega-Ortega, B. E., Niggemann, J., Dittmar, T., and Simon, M. (2017). The exometabolome of two model strains of the roseobacter group: a marketplace of microbial metabolites. Front. Microbiol. 8:1985. doi: 10.3389/fmicb.2017.01985
Xie, B., Bishop, S., Stessman, D., Wright, D., Spalding, M. H., and Halverson, L. J. (2013). Chlamydomonas reinhardtii thermal tolerance enhancement mediated by a mutualistic interaction with vitamin B12-producing bacteria. ISME J. 7, 1544–1555. doi: 10.1038/ismej.2013.43
Keywords: B vitamin auxotrophy, growth limitation, Dinoflagellate-bacteria interactions, dissolved B12, particulate B12, particulate B7
Citation: Cruz-López R, Maske H, Yarimizu K and Holland NA (2018) The B-Vitamin Mutualism Between the Dinoflagellate Lingulodinium polyedrum and the Bacterium Dinoroseobacter shibae. Front. Mar. Sci. 5:274. doi: 10.3389/fmars.2018.00274
Received: 01 March 2018; Accepted: 20 July 2018;
Published: 28 August 2018.
Edited by:
Stanley Chun Kwan Lau, Hong Kong University of Science and Technology, Hong KongReviewed by:
Meinhard Simon, University of Oldenburg, GermanyJohn Everett Parkinson, Oregon State University, United States
Copyright © 2018 Cruz-López, Maske, Yarimizu and Holland. This is an open-access article distributed under the terms of the Creative Commons Attribution License (CC BY). The use, distribution or reproduction in other forums is permitted, provided the original author(s) and the copyright owner(s) are credited and that the original publication in this journal is cited, in accordance with accepted academic practice. No use, distribution or reproduction is permitted which does not comply with these terms.
*Correspondence: Ricardo Cruz-López, cmljYXJkby5jcmxwQGdtYWlsLmNvbQ==
†Present Address: Ricardo Cruz-López, Department of Chemistry and Biochemistry, San Diego State University, San Diego, CA, United States