- Department of Marine Science, Incheon National University, Incheon, South Korea
Along the Texas-Louisiana continental shelf in the northern Gulf of Mexico (nGOM) is a region frequently referred to as the “Dead Zone” due to severe oxygen depletion caused by eutrophication. Owing to its characteristics, it is expected to be an oceanic source region for nitrous oxide (N2O) emissions to the atmosphere during the hypoxic events. However, there is little known about whether the nGOM is source or sink region for atmospheric N2O. Here, we estimate “mean-state” July (1988-2007) N2O fluxes from the nGOM, using simple modeling approach. The estimated mean N2O fluxes for July across the air-sea interface ranged from +0.9 ± 11.7 to +14.3 ± 15.0 μmol N2O m−2 d−1 with a mean value of +6.1 ± 9.0 μmol N2O m−2 d−1 (+: sea → air). Our estimates were in reasonable agreement with the few available summer measurements, and suggested that the nGOM hypoxic region acts as a source of atmospheric N2O during the month of July between 1985 and 2007. Local regions influenced by the Mississippi and Atchafalaya Rivers showed higher N2O fluxes to the atmosphere than other regions. If the area affected by nutrient loading and the resulting hypoxia expands, the nGOM may become an even stronger oceanic N2O “hot spot” source region. Therefore, future study, based on in-situ observations, is necessary to elucidate N2O dynamics in the nGOM hypoxic region.
Introduction
One of the most important nitrogen cycle processes directly linked to climate change is nitrous oxide (N2O) production, owing to its strong greenhouse effect and high ozone depletion potential (IPCC, 2013). Atmospheric N2O concentrations rapidly increased during the Anthropocene (~1760 to present) due to human activities (Weiss, 1981; Machida et al., 1995; Battle et al., 1996). Additionally, while land-based sources are important, the oceans are one of the most important sources for atmospheric N2O (Nevison et al., 1995, 2003; Seitzinger et al., 2000; Bange, 2006; Hirsch et al., 2006) accounting for ~35% (3.8 Tg N yr−1) of the total production from natural sources (11.0 Tg N yr−1) (IPCC, 2013). Although small in area, coastal regions are responsible for a significant fraction of the total oceanic N2O emissions (Bange et al., 1996; IPCC, 2013).
The northern Gulf of Mexico (nGOM) along the Texas-Louisiana continental shelf area (Figure 1) is frequently referred to as a “Dead Zone” due to severe oxygen depletion caused by eutrophication via river inputs (i.e., the Atchafalaya and Mississippi rivers), leading to high biological production and fast remineralization rates (Justić et al., 1995; Turner and Rabalais, 2004). As a result, hypoxia (i.e., [O2] ≤ 2 mg L−1≈63 μM) develops every summer season over extensive regions of the nGOM (Dagg et al., 2007). The limited dissolved N2O concentrations measurements available in the nGOM (available for September 2007, April, July, and August 2008 to date) have shown the area to be a significant source of atmospheric N2O (Visser, 2009; Walker et al., 2010). Since microbial N2O production is enhanced during hypoxic conditions and the hypoxic area has extended during recent years (Diaz and Rosenberg, 2008; Rabalais et al., 2009; Codispoti, 2010; Naqvi et al., 2010), the nGOM is assumed to be an oceanic source region for N2O emissions to the atmosphere. However, little is known about the temporal evolution of N2O fluxes in the nGOM hypoxic region. Further, the limited available observation records may be insufficient to reach conclusions about whether the nGOM is a net source region for atmospheric N2O because coastal oceans tend to display a high degree of variability (Gruber, 2015). High variability and limited observations are issues faced widely throughout the coastal oceans research community.
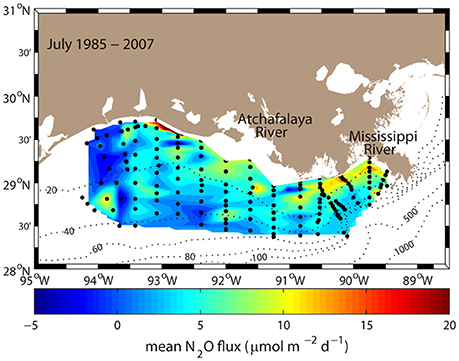
Figure 1. Spatial distribution of the July mean N2O flux averaged from 1985 to 2007 (excluding 1988–1990) with the bathymetry contours (black dotted lines; unit of meters) in the nGOM. Black dots indicate the hydrographic stations used in our analysis.
Recently, Kim et al. (2013) developed a simple model that estimates bottom-water N2O concentrations using an empirically based tri-linear ΔN2O (i.e., excess N2O, which is mainly determined by nitrification and denitrification as the major microbial production and consumption pathways) relationship associated with dissolved oxygen (O2) concentrations and presented the evolution of bottom-water N2O concentrations in the nGOM for the month of July between 1985 and 2007. However, they did not extend to the N2O flux study to determine whether the nGOM is a source (+: ocean → atmosphere) or sink (–: atmosphere → ocean) for atmospheric N2O. The way that the model data (i.e., the bottom-water N2O concentrations estimated for July 1985–2007) were combined along with the application of some further reasonable assumptions provides an opportunity to reconstruct a time-series of the N2O fluxes to the atmosphere from the nGOM. Therefore, the main purpose of this study was to (1) present an estimate of the temporal evolution of July N2O fluxes from the nGOM, and (2) determine whether the nGOM is a net source or sink region for atmospheric N2O for the month of July from 1985 to 2007. Note that our study is an extension of Kim et al. (2013) with a focus on July N2O flux dynamics in the nGOM.
Methods
Data, Approach, and Limitation
Recently, Kim et al. (2013) estimated the summer (July) bottom-water N2O concentrations using a conceptual N2O biogeochemical model, based on the summer Texas-Louisiana shelf-wide hydrographic datasets observed for the month of July between 1985 and 2007 (excluding July 1988-1990 due to lack of available data). The bottom-water N2O concentrations () were estimated using an empirically derived ΔN2O/O2 relationship as follows:
where ΔN2O is the concentration determined by microbial processes (i.e., nitrification and denitrification by bacteria and archaea), is the N2O atmospheric equilibrated value with atmosphere (details in Equation 4 below), AOU is the apparent oxygen utilization—the difference between the measured O2 concentration and the O2 equilibration value (i.e., ), the α coefficient indicates the relationship between ΔN2O and AOU, and the β and γ coefficients are the relationships between ΔN2O and the amount of denitrification (ΔNdeni) that is the loss of nitrate () as a consequence of denitrification. More information about the estimation of bottom-water N2O concentrations can be found in Kim et al. (2013).
To evaluate whether the nGOM is a net source or sink for atmospheric N2O during the month of July, we calculated the N2O fluxes for July from 1985 to 2007 from the nGOM using the July surface-water N2O concentrations derived from the results of bottom-water N2O concentrations simulated by Kim et al. (2013) in conjunction with the N2O exchange rates obtained from the air-sea gas formulations. The summer Texas-Louisiana shelf-wide hydrographic datasets used in our analysis are available at http://www.nodc.noaa.gov and http://www.aoml.noaa.gov.
During the summer, hypoxic conditions develop over extensive regions in the nGOM implying that the water-column stratification is strong. Visser (2009) reported that the mean surface and bottom N2O concentrations were 6.3 ± 0.9 and 11.0 ± 7.0 nmol L−1 in July 2008 (max bottom depth < ~30 m), respectively, indicating a large difference in the N2O concentration between the surface and bottom waters (i.e., ~57% less in surface waters compared to bottom waters). In this study, we estimated the surface N2O concentrations () as follows:
where is the estimated bottom-water N2O concentration by Kim et al. (2013).
Implicit in our approach is that the constant was uniformly applied to generate the surface-water N2O concentrations. In spite of this approach, our results may provide a baseline for future N2O studies in the nGOM, where is expected to be a significant N2O source (sea to air) region due to the extending/strengthening the hypoxic area (Rabalais et al., 2009; Visser, 2009). Therefore, we emphasize that here, the July N2O fluxes estimated from the following section are the mean values averaged from the hydrographic stations (i.e., , where i is the July estimate at one station, and n is the number of total estimates for individual year from 1985 to 2007), and thus could be roughly considered as an upper limit of the N2O fluxes for July in the nGOM. Although the measurements were collected during a different time window, our estimates were validated by comparing the available summer measurements in the study area to date (see Figure 2).
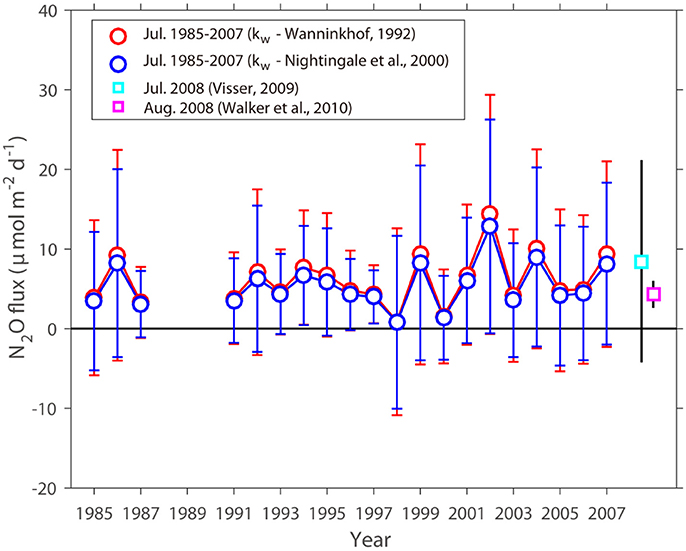
Figure 2. Temporal variation of the estimated July N2O flux during the 1985–2007 study period (excluding 1988–1990) in the nGOM. Red circles are the estimated July N2O fluxes from this study using the gas transfer velocity (kw) of Wanninkhof (1992), blue circles are the estimated N2O fluxes from this study using kw of Nightingale et al. (2000), the cyan square is the measured N2O fluxes in July 2008 by Visser (2009), and the pink square is the measured N2O flux in August 2008 by Walker et al. (2010). Error bars indicate the standard deviations from the mean.
Estimation of N2O Flux
We used the air-sea gas exchange equation to estimate the N2O flux as follows:
where kw is the gas transfer velocity (cm h−1), and is the concentration of N2O in equilibrium with the atmosphere and is calculated as:
where x′ is the mean atmospheric N2O dry mole fraction (ppb) for July, β is the N2O Bunsen solubility (mol L−1 atm−1) that is determined from the seawater temperature and salinity relationship (Weiss and Price, 1980), and P is the ambient pressure which was set to 1 atm. As further information, the atmospheric N2O dry mole fractions (i.e., x′) during the study period (July 1985–2007) specific to the nGOM did not exist, and therefore the mean atmospheric N2O dry mole fractions observed during this period over the full northern hemisphere NOAA/ESRL halocarbons program were used instead (http://www.esrl.noaa.gov/).
Several model approaches have been published to estimate the gas transfer velocity (kw) from wind speed (e.g., Liss and Merlivat, 1986; Wanninkhof, 1992; Wanninkhof and McGillis, 1999; Nightingale et al., 2000). To facilitate the comparison of our results with the previous studies, we follow the approach of Visser (2009) and Walker et al. (2010) who used the Wanninkhof (1992) and Nightingale et al. (2000) air-sea exchange models (kwW1992 and kwN2000, respectively) as follows:
both kw models require estimates of the wind speed at a 10 m height (U10) and Schmidt numbers (Sc). The Sc number was calculated as the kinematic viscosity of seawater (Siedler and Peters, 1986) divided by the diffusion coefficient of N2O in seawater (Rhee, 2000). The U10 wind speeds were not measured during the Texas-Louisiana shelf-wide hydrographic cruises. Instead, the mean wind speeds for each July from 1987 to 2007 were obtained for the nGOM from the NOAA Blend Sea Winds product database (BSW, http://www.ncdc.noaa.gov/). Using data from the BSW, we also computed a 20-year mean July U10 of 4.9 ± 0.5 m s−1 that was used for July 1985 and 1986 because no data were available from the BSW for these months.
The mean difference in the estimated July N2O flux between the two models was 0.7 μmol N2O m−2 d−1 (Table 1). The resulting July N2O flux estimates, which are discussed in the next section, satisfactory display the “mean-state” spatial distribution and temporal variation in the nGOM (Figures 1, 2) despite the strong variability expected in a coastal region and the potentially high degree of uncertainty due to implicit in our approach. The estimated N2O fluxes for the month of July 1985-2007 are summarized in Table 1.
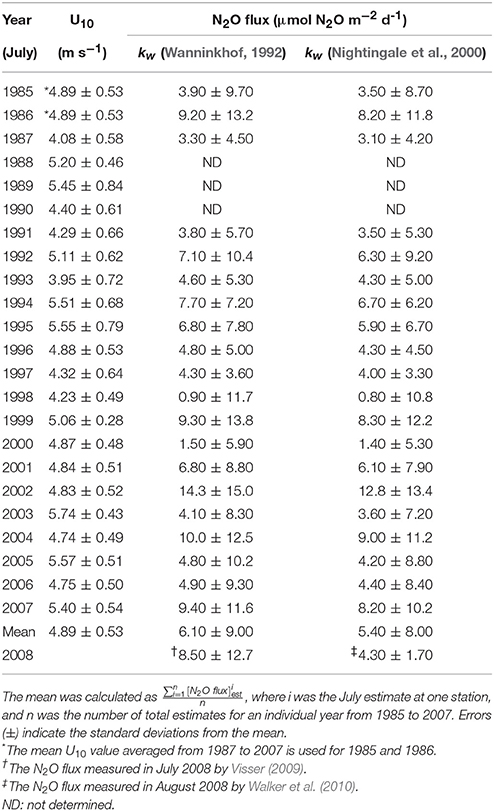
Table 1. Summary of July mean wind speeds (U10; m s−1) and estimated mean N2O fluxes for July in the nGOM.
Results and Discussion
Spatial and Temporal Dynamics of N2O Flux
To determine whether the nGOM is a net source or sink of atmospheric N2O during the summer, we reconstructed the temporal variation of the nGOM N2O fluxes for July 1985-2007 and included for comparison recent estimates based on direct measurements of dissolved N2O by Visser (2009) (+8.5 ± 12.7 μmol N2O m−2 d−1 in July 2008) and Walker et al. (2010) (+4.3 ± 1.7 in August 2008) (Figure 2). Additionally, Visser (2009) also reported N2O fluxes measured in September 2007 (+66.4 ± 46.7 μmol N2O m−2 d−1) and April 2008 (−5.2 ± 4.0 μmol N2O m−2 d−1). The estimated N2O fluxes for July across the air-sea interface ranged from +0.9 ± 11.7 (July 1998) to +14.3 ± 15.0 μmol N2O m−2 d−1 (July 2002) with a mean value of +6.1 ± 9.0 μmol N2O m−2 d−1 using (+0.8 ± 10.8 to +12.8 ± 13.4 μmol N2O m−2 d−1 with the mean value of +5.4±8.0 μmol N2O m−2 d−1 using ) (Table 1), equivalent to ~0.04–0.6 Gg N2O July−1 (G: Giga, 109) extrapolated to the size of study area (≈ 3.24 × 1010 m2). Our July estimates were in reasonable agreement with the measured Visser (2009) and Walker et al. (2010) summer nGOM values (i.e., +8.5 ± 12.7 and +4.3 ± 1.7 μmol N2O m−2 d−1 in July and August 2008, respectively) (Figure 2 and Table 1). None of the July estimates were as large as the Visser (2009) September 2007 value (i.e., +66.4 ± 46.7 μmol N2O m−2 d−1) and only one (i.e., −5.2 ± 4.0 μmol N2O m−2 d−1) indicated the possibility of a sink for spring as suggested by Visser (2009).
Under low oxygen conditions, denitrification ( → → N2O/N2), which is a dissimilatory process that uses nitrate as an electron acceptor instead of oxygen (Brandes et al., 2007), acts as a source (i.e., production under hypoxic condition: 0.14 < O2 ≤ 2 mg L−1) or a sink (i.e., consumption under suboxic-anoxic condition: 0 ≤ O2 ≤ 0.14 mg L−1) depending on the O2 concentrations (Naqvi et al., 2010; Kim et al., 2013). In July 1998, N2O consumption from denitrification was significant (Kim et al., 2013), therefore, the estimated N2O flux was the lowest during the study period (Figure 2 and Table 1). In July 1987 (3.3 ± 4.5 μmol N2O m−2 d−1 using ) and 2000 (1.5 ± 5.9 μmol N2O m−2 d−1 using ), the estimated N2O fluxes were relatively low compared to other years (Figure 2 and Table 1). These features may be explained by the magnitude of the remineralization (i.e., primary source for N2O production via nitrification process) in 1987 and 2000 that was lower than other study years (Kim and Min, 2013; Kim et al., 2013), due to the low biological production driven by less discharges from the Atchafalaya-Mississippi Rivers (sum of January to July) (Walker and Rabalais, 2006). For example, the total river discharge in 1987 and 2000 was 0.15 and 0.12 Sverdrup (Sv, which is a unit of water volume transport and is given as 106 m3 s−1), respectively, and was lower than the mean 0.19 ± 0.04 Sv Value. As a result, the sizes of hypoxia in July 1987 (6.7 × 109 m2) and 2000 (4.4 × 109 m2) were smaller compared to the mean value (14.4 ± 5.1 × 109 m2) averaged during the study periods (July 1985–2007) (https://gulfhypoxia.net/). In July 2002, the estimated N2O flux was highest value during the study periods (Figure 2 and Table 1). In contrast to 1998, denitrification acted as a source for N2O production in 2002, and also the size of hypoxia (22 × 109 m2) was the largest during the study periods, indicating that the magnitude of remineralization was high (Kim and Min, 2013). In addition, the total river discharge was relatively high (0.20 Sv). Overall, these conditions might have driven the highest estimated N2O flux in July 2002.
Further these nGOM July N2O flux estimates were comparable to those published for other marine O2-deficient systems (i.e., open-ocean hypoxic zones: 2.7–4.5 μmol N2O m−2 d−1, naturally-formed continental-margin hypoxic zones: 10–50 μmol N2O m−2 d−1, anthropogenically-formed hypoxic zones: 3.3–17.1 μmol N2O m−2 d−1, enclosed anoxic basins: 1.6–5.2 μmol N2O m−2 d−1) [see Table 3 of (Naqvi et al., 2010), and references therein]. The estimated July N2O fluxes suggest that the nGOM hypoxic region acts as a source of atmospheric N2O in July with a mean of 6.1 ± 9.0 μmol N2O m−2 d−1 using (5.4 ± 8.0 μmol N2O m−2 d−1 using ) (Table 1).
A contour map illustrating the spatial distribution of the mean July nGOM N2O fluxes averaged from 1985 to 2007 (excluding 1988–1990 due to the lack of available data) was used to investigate the spatially homogeneity (Figure 1). Relatively high N2O fluxes (> ~10 μmol N2O m−2 d−1) were distributed along inshore regions (< ~20 m isobaths), whereas relatively low N2O fluxes (< ~10 μmol N2O m−2 d−1) occupied a wider offshore area (> ~20 m isobaths). It is noted that the regions influenced by the Atchafalaya and Mississippi Rivers displayed particularly high N2O fluxes compared to other areas of the nGOM (Figure 1). Nutrient loading from river inputs stimulates phytoplankton blooms in these inshore areas downstream of the inflow (Walker and Rabalais, 2006). The anthropogenically supported blooms eventually lead to hypoxia through remineralization processes (Pakulski et al., 2000; Dagg and Breed, 2003; Fry et al., 2015). Remineralization (i.e., nitrification processes) is primarily responsible for the N2O production (> ~90%) (Kim et al., 2013), therefore, the distribution of the N2O fluxes largely reflect the magnitude of oxygen consumption in the nGOM.
Future N2O Emissions From the nGOM: A Potential Positive Climate Feedback Loop
A multitude of changes that are occurring in the nGOM may directly and/or indirectly influence prediction of future N2O emissions from the nGOM (Figure 3). Summer surface and bottom water temperatures have increased significantly in the study area over the period of 1985–2007 (Kim and Min, 2013). These increases in seawater temperature have enhanced stratification in the water column. Freshwater inflows have also increased into the nGOM (Justić et al., 2003, 2005), leading to coastal eutrophication due to increased nutrient loading. As a consequence of the increases in both stratification and eutrophication, the hypoxic layer thickness in the nGOM has increased (Obenour et al., 2013). The resulting nitrification and denitrification strengthening processes (Kim et al., 2013) that are yielding higher N2O concentrations under hypoxic conditions, may lead to further increases in N2O outgassing from the nGOM. The results presented here suggest that the nGOM is a net summertime source region (i.e., +: sea → air) for atmospheric N2O (Table 1) as a strong greenhouse gas. Based on a significant correlation between N2O production and the size of the hypoxic region (Kim et al., 2013), we expect that in the future, if the areas affected by nutrient loading and the resulting hypoxia expand, the nGOM may be even more effective oceanic N2O “hot spot” source region. Therefore, we suggest that regular surveys of dissolved N2O measurements in the nGOM are needed to improve N2O flux estimates from the nGOM and to decipher the production pathways.
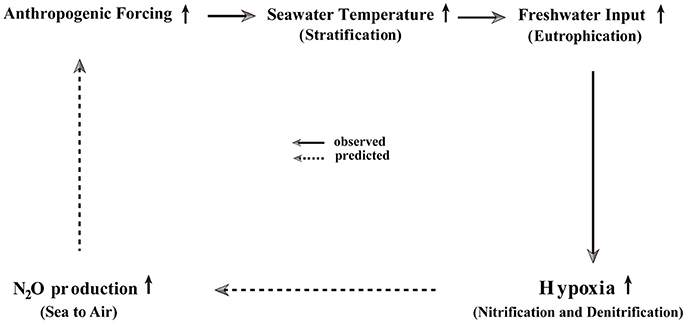
Figure 3. A conceptual feedback scheme for future N2O emission in the nGOM, based on physical changes currently occurring in the nGOM.
Summary
We estimated “mean-state” July (1988–2007) N2O fluxes from the nGOM and investigated whether the nGOM is a net source (+: sea to air) or a net sink (–: air to sea) for atmospheric N2O, using simple modeling approach. The estimated mean N2O fluxes for July across the air-sea interface ranged from +0.9 ± 11.7 to +14.3 ± 15.0 μmol N2O m−2 d−1 with a mean value of +6.1 ± 9.0 μmol N2O m−2 d−1 using (+0.8 ± 10.8 to +12.8 ± 13.4 μmol N2O m−2 d−1 with the mean value of +5.4 ± 8.0 μmol N2O m−2 d−1 using ) suggesting that the nGOM hypoxic region acts as a source of atmospheric N2O during July. Spatially, local regions influenced by the Mississippi and Atchafalaya Rivers showed higher N2O fluxes to the atmosphere than other regions. If the area affected by hypoxia expands (due to enhanced nitrification/denitrification processes), the nGOM may become an even stronger oceanic N2O “hot spot” source region. Although the present study results have some caveats, it behooves us to pay attention to future N2O dynamics in the nGOM.
Author Contributions
The author confirms being the sole contributor of this work and approved it for publication.
Funding
This study was supported by the National Research Foundation of Korea (NRF) grant funded by the Korea government (MSIP) (No. 2015R1C1A1A01052051).
Conflict of Interest Statement
The author declares that the research was conducted in the absence of any commercial or financial relationships that could be construed as a potential conflict of interest.
Acknowledgements
We thank all scientists responsible for the Texas-Louisiana shelf-wide cruises. We thank Drs. H. W. Bange (GEOMAR) and A. M. Macdonald (WHOI) for their valuable comments that improved our manuscript substantially.
References
Bange, H. W. (2006). New directions: the importance of oceanic nitrous oxide emissions. Atmos. Environ. 40, 198–199. doi: 10.1016/j.atmosenv.2005.09.030
Bange, H. W., Rapsomanikis, S., and Andreae, M. O. (1996). Nitrous oxide in coastal waters. Glob. Biogeochem. Cycles 10, 197–207. doi: 10.1029/95GB03834
Battle, M., Bender, M., Sowers, T., Tans, P. P., Butler, J. H., Elkins, J. W., et al. (1996). Atmospheric gas concentrations over the past century measured in air from firn at the South Pole. Nature 383, 231–235. doi: 10.1038/383231a0
Brandes, J. A., Devol, A. H., and Deutsch, C. (2007). New development in the marine nitrogen cycle. Chem. Rev. 107, 577–589. doi: 10.1021/cr050377t
Codispoti, L. A. (2010). Interesting times for marine N2O. Science 327, 1339–1340. doi: 10.1126/science.1184945
Dagg, M. J., Ammerman, J. W.R., Amon, M. W., Gardner, W. S., Green, R. E., and Lohrenz, S. E. (2007). A review of water column processes influencing hypoxia in the northern Gulf of Mexico. Estuar. Coasts 30, 735–752. doi: 10.1007/BF02841331
Dagg, M. J., and Breed, G. A. (2003). Biological effects of Mississippi River nitrogen on the northern Gulf of Mexico – a review and synthesis. J. Mar. Sys. 43, 133–152. doi: 10.1016/j.jmarsys.2003.09.002
Diaz, R. J., and Rosenberg, R. (2008). Spreading dead zones and consequences for marine ecosystems. Science 321, 926–929. doi: 10.1126/science.1156401
Fry, B., Justić, D., Riekenberg, P., Swenson, E. M., Turner, R. E., Wang, L., et al. (2015). Carbon dynamics on the Louisiana continental shelf and cross-shelf feeding of hypoxia. Estuar. Coasts. 38, 703–721. doi: 10.1007/s12237-014-9863-9
Hirsch, A. I., Michalak, A. M., Bruhwiler, L. M., Peters, W., Dlugokencky, E. J., and Tans, P. P. (2006). Inverse modeling estimates of the global nitrous oxide surface flux from 1998–2001. Glob. Biogeochem. Cycles 20:GB1008. doi: 10.1029/2004GB002443
IPCC (2013). Climate Change 2013: The Physical Science Basis. Contribution of Working Group I to the Fifth Assessment Report of the Intergovernmental Panel on Climate Change, Cambridge; New York, NY: Cambridge University Press.
Justić, D., Rabalais, N. N., and Turner, R. E. (1995). Stoichiometric nutrient balance and origin of coastal eutrophication. Mar. Pollut. Bull. 30, 41–46. doi: 10.1016/0025-326X(94)00105-I
Justić, D., Rabalais, N. N., and Turner, R. E. (2005). Coupling between climate variability and coastal eutrophication: evidence and outlook for the northern Gulf of Mexico. J. Sea Res. 54, 25–35. doi: 10.1016/j.seares.2005.02.008
Justić, D., Turner, R. E., and Rabalais, N. N. (2003). Climatic influences on riverine nitrate flux: implication for coastal marine eutrophication and hypoxia. Estuaries 26, 1–11. doi: 10.1007/BF02691688
Kim, I.-N., and Min, D.-H. (2013). Temporal variation of summertime denitrification rates in the Texas-Louisiana inner shelf region in the Gulf of Mexico: a modeling approach using the extended OMP analysis. Cont. Shelf Res. 66, 49–57. doi: 10.1016/j.csr.2013.07.005
Kim, I.-N., Lee, K., Bange, H. W., and Macdonald, A. M. (2013). Interannual variation in summer N2O concentration in the hypoxic region of the northern Gulf of Mexico, 1985–2007. Biogeosciences 10, 6783–6792. doi: 10.5194/bg-10-6783-2013
Liss, P. S., and Merlivat, L. (1986). “Air-sea gas exchange rates: introduction and synthesis,” in The Role of Air-Sea Exchange in Geochemical Cycling, eds P. Buat-Menard and D.Reidel (Dordrecht: Springer), 113–127.
Machida, T., Nakazawa, T., Fujii, Y., Aoki, S., and Watanabe, O. (1995). Increase in the atmospheric nitrous oxide concentration during the last 250 years. Geophys. Res. Lett. 22, 2921–2924. doi: 10.1029/95GL02822
Naqvi, S. W. A., Bange, H. W., Farias, L.P., Monteiro, M. S., Scranton, M. I., and Zhang, J. (2010). Marine hypoxia/anoxia as a source of CH4 and N2O. Biogeosciences 7, 2159–2190. doi: 10.5194/bg-7-2159-2010
Nevison, C., Butler, J. H., and Elkins, J. W. (2003). Global distribution of N2O and the ΔN2O-AOU yield in the subsurface ocean. Glob. Biogeochem. Cycles 17, 1119. doi: 10.1029/2003GB002068
Nevison, C. D., Weiss, R. F., and Erickson, D. J. III. (1995). Global oceanic emissions of nitrous oxide. J. Geophys. Res. 100, 15809–15820.
Nightingale, P. D., Malin, G., Law, C. S., Watson, A. J., Liss, P. S., Liddicoat, M. I., et al. (2000). In situ evaluation of air-sea gas exchange parameterizations using novel conservative and volatile tracers. Glob. Biogeochem. Cycles 14, 373–387. doi: 10.1029/1999GB900091
Obenour, D. R., Scavia, D., Rabalais, N. N., Turner, R. E., and Michalak, A. M. (2013). Retrospective analysis of midsummer hypoxic area and volume in the northern Gulf of Mexico, 1985-2011. Environ. Sci. Technol. 47, 9808–9815. doi: 10.1021/es400983g
Pakulski, J. D., Benner, R., Whitledge, T., Amon, R., Eadie, B., Cifuentes, L., et al. (2000). Microbial metabolism and nutrient cycling in the Mississippi and Atchafalaya River plumes. Estuar. Coast. Shelf Sci. 50, 173–184. doi: 10.1006/ecss.1999.0561
Rabalais, N. N., Turner, R. E., Díaz, R. J., and Justić, D. (2009). Global change and eutrophication of coastal waters, ICES J. Mar. Sci. 66, 1528–1537. doi: 10.1093/icesjms/fsp047
Rhee, T. S. (2000). The Process of Air-Water Gas Exchange and Its Application. Ph.D. thesis, Department of Oceanography, Texas AandM University, College Station, TX.
Seitzinger, S. P., Kroeze, C., and Styles, R. V. (2000). Global distribution of N2O emissions from aquatic systems: natural emissions and anthropogenic effects. Chemosphere Glob. Change Sci. 2, 267–279. doi: 10.1016/S1465-9972(00)00015-5
Siedler, G., and Peters, H. (1986).“Properties of seawater,” in Oceanography, ed J. Sündermann (New York, NY:Springer Verlag), 233–264.
Turner, R. E., and Rabalais, N. N. (2004). Suspended sediment, C, N, P, and Si yields from the Mississippi River basin. Hydrobiologia 511, 79–89. doi: 10.1023/B:HYDR.0000014031.12067.1a
Visser, L. A. (2009). Nitrous Oxide Production in the Gulf of Mexico Hypoxic Zone. M.S. thesis, Department of Oceanography, Texas A&M University, College Station, TX.
Walker, N. D., and Rabalais, N. N. (2006). Relationship among satellite chlorophyll a, river inputs, and hypoxia on the Louisiana continental shelf, Gulf of Mexico. Estuar. Coasts 29, 1081–1093. doi: 10.1007/BF02781811
Walker, J. T., Stow, C. A., and Geron, C. (2010). Nitrous oxide emissions from the Gulf of Mexico hypoxic zone. Environ. Sci. Technol. 44, 1617–1623. doi: 10.1021/es902058t
Wanninkhof, R. (1992). Relationship between wind speed and gas exchange over the ocean. J. Geophys. Res. 97, 7373–7382. doi: 10.1029/92JC00188
Wanninkhof, R., and McGillis, W. R. (1999). A cubic relationship between air-sea CO2 exchange and wind speed. Geophys. Res. Lett. 26, 1889–1892. doi: 10.1029/1999GL900363
Weiss, R. F. (1981). The temporal and spatial distribution of tropospheric nitrous oxide. J. Geophys. Res. 86, 7185–7195. doi: 10.1029/JC086iC08p07185
Keywords: the northern Gulf of Mexico, N2O, hypoxia, positive climate feedback, air-sea exchange
Citation: Kim I-N (2018) Estimating “Mean-State” July (1985–2007) N2O Fluxes in the Northern Gulf of Mexico Hypoxic Region: Variation, Distribution, and Implication. Front. Mar. Sci. 5:249. doi: 10.3389/fmars.2018.00249
Received: 22 December 2017; Accepted: 28 June 2018;
Published: 17 July 2018.
Edited by:
Sunil Kumar Singh, Physical Research Laboratory, IndiaReviewed by:
Rajesh Agnihotri, Birbal Sahni Institute of Paleosciences, IndiaSatya Prakash, Indian National Centre for Ocean Information Services, India
Copyright © 2018 Kim. This is an open-access article distributed under the terms of the Creative Commons Attribution License (CC BY). The use, distribution or reproduction in other forums is permitted, provided the original author(s) and the copyright owner(s) are credited and that the original publication in this journal is cited, in accordance with accepted academic practice. No use, distribution or reproduction is permitted which does not comply with these terms.
*Correspondence: Il-Nam Kim, aWxuYW1raW1AaW51LmFjLmty