- 1Centre for Marine and Environmental Research, University of Algarve, Faro, Portugal
- 2Systemic Physiological and Ecotoxicological Research, Department of Biology, University of Antwerp, Antwerp, Belgium
- 3Man-Technology-Environment Research Centre (MTM), School of Science and Technology, Örebro University, Örebro, Sweden
- 4UMR CNRS 5805 EPOC, University of Bordeaux, Talence, France
Although microplastics (MPs) are distributed globally in the marine environment, a great deal of unknowns relating to their ecotoxicological effects on the marine biota remains. Due to their lipophilic nature, microplastics have the potential to adsorb persistent organic pollutants present in contaminated regions, which may increase their detrimental impact once assimilated by organisms. This study investigates the ecotoxicological effects of exposure to low-density polyethylene (LDPE) microplastics (11–13 μm), with and without adsorbed contaminants (benzo[a]pyrene—BaP and perfluorooctane sulfonic acid—PFOS), in the peppery furrow shell clam, Scrobicularia plana. Environmentally relevant concentrations of contaminants (BaP−16.87 ± 0.22 μg g−1 and PFOS−70.22 ± 12.41 μg g−1) were adsorbed to microplastics to evaluate the potential role of plastic particles as a source of chemical contamination once ingested. S. plana were exposed to microplastics, at a concentration of 1 mg L−1, in a water-sediment exposure setup for 14 days. Clams were sampled at the beginning of the experiment (day 0) and after 3, 7, and 14 days. BaP accumulation, in whole clam tissues, was analyzed. A multi-biomarker assessment was conducted in the gills, digestive gland, and haemolymph of clams to clarify the effects of exposure. This included the quantification of antioxidant (superoxide dismutase, catalase, glutathione peroxidase) and biotransformation (glutathione-S-transferases) enzyme activities, oxidative damage (lipid peroxidation levels), genotoxicity (single and double strand DNA breaks), and neurotoxicity (acetylcholinesterase activity). Results suggest a potential mechanical injury of gills caused by ingestion of microplastics that may also affect the analyzed biomarkers. The digestive gland seems less affected by mechanical damage caused by virgin microplastic exposure, with the MPs-adsorbed BaP and PFOS exerting a negative influence over the assessed biomarkers in this tissue.
Introduction
Advances in plastic production have resulted in more versatile, lightweight, durable, and cheap plastics, which have become incorporated in every part of our day-to-day lives (Andrady and Neal, 2009). Yet, plastic is now a ubiquitous, long lasting source of litter on the planet (Barnes et al., 2009). Since mass production of plastic began in the 1940's, annual production has increased from ~5 Mt in the 1950's to 322 Mt in 2015 (Plastics-the Facts, 2016). Low density polyethylene (LDPE) is used in the production of reusable bags and agricultural films, with its predominant use in the food packaging industry (Andrady, 2003; Plastics-the Facts, 2016).
Macroplastics (>5 mm) and their effect on the marine environment have been studied for some years (Cole et al., 2011). Due to their large size, being clearly visible they create “eyesores” on the landscape and in the oceans as well as entangling and endangering marine life. Estimates of the amount of plastic currently residing in the oceans varies from 7,000 t (Cózar et al., 2014), to over 250,000 t (Eriksen et al., 2014). In recent years microplastics (MPs; <5 mm) have become an area of concern due to their ubiquitous distribution in the marine environment, occurring in all geographical regions of the Oceans, including the Arctic (Obbard et al., 2014), and Antarctica (Lusher, 2015). The presence of microplastics have been reported in a wide range of marine habitats - beach sediments (Thompson et al., 2004), subtidal and benthic sediments (Barnes et al., 2009), deep-sea sediments (Fischer et al., 2015), the water column, surface waters (Hidalgo-Ruz et al., 2012), near densely populated areas (Barnes, 2005), and even in remote island atolls where no input or production of plastics occurs (McDermid and McMullen, 2004). Properties of plastic polymers, such as density, surface charge and aggregation potential, as well as abiotic (oxidation, weathering, and vertical mixing) and biotic factors (biofouling) all contribute to the ubiquitous distribution of MPs within the oceans (Lusher, 2015). Microplastics have been reported in 61% of Portuguese water samples with higher concentrations in Costa Vincentina (0.036 particles m−3) and Lisbon (0.033 particles m−3) compared to the Algarve (0.014 particles m−3) and Aveiro (0.002 particles m−3) (Frias et al., 2014). Microplastic resin pellets (3–6 mm, 5% > 5 mm) represented 53% of total marine debris collected (1,289 items m−2, 30 g m−2) in another study on the Portuguese coastline, with 98% of marine debris being identified as plastic (Antunes et al., 2013). In both cases, higher microplastic abundances were reported in proximity to urban, industrial, and shipping areas.
MP ingestion, or uptake by other means, has been reported in a variety of marine species including plankton, invertebrates, fish, sea birds, marine mammals, and turtles (e.g., Browne et al., 2008; Moore, 2008; von Moos et al., 2012; Galgani et al., 2014; Avio et al., 2015, 2017; Lusher, 2015; Setälä et al., 2016; Lourenço et al., 2017; Germanov et al., 2018; Kolandhasamy et al., 2018). MPs may enter the base of the food chain through ingestion or adsorption by phytoplankton and/or zooplankton (Lusher, 2015) and trophic transfer has been reported (e.g., Farrell and Nelson, 2013). Commercial marine species which are eaten whole, such as shrimps and bivalves, constitute a potential transfer pathway of MPs from the marine environment to humans (Lusher, 2015; Rochman et al., 2015; Santillo et al., 2017).
MP particle size plays an important role in their biological fate within the marine environment. Impacts on the marine biota may vary across the size spectrum of MPs. Large microplastics (2–5 mm) may take more time to pass from the stomach of organisms, having the potential to be retained in the digestive system. Toxicant adsorption, dependent on polymer type, may occur with increased exposure time to plastics. Feeding and digestion may occur with particles in the upper end of the size spectrum (1–2 mm) (Lusher, 2015). Small marine invertebrates have been shown to actively ingest and egest particles <20 μm (Thompson et al., 2004; Lee et al., 2013). Smaller size microplastics have larger effects on organisms at the cellular level. In the micro- to nanometer range microplastics have been shown to translocate and pass into cellular membranes, including the haemolymph (Browne et al., 2008; Ribeiro et al., 2017), and the lysosomal system (von Moos et al., 2012) of marine invertebrates.
Many additives are added in the production of plastics, which give them various desirable qualities and enhance their performance (Andrady and Neal, 2009). Many of the additives have known or suspected toxicity, containing persistent organic pollutants (POPs), synthetic organic compounds of anthropogenic origin. POPs are chemically stable and do not easily degrade in the environment. Plastics are known to sorb hydrophobic chemical contaminants from the surrounding sea water, and have been shown to concentrate them (Mato et al., 2001; Rios et al., 2007; Barnes et al., 2009; Wu et al., 2016; Zhang et al., 2018). Degradation of MPs to smaller plastic particle sizes adds more surface area to sorb contaminants. The combination of increased surface area due to weathering, long exposure times in the marine environment, and the hydrophobicity of organic xenobiotics may facilitate adsorption of these contaminants to MPs at concentrations significantly higher than those detected in seawater (Ogata et al., 2009; Antunes et al., 2013). Hence, MPs are vehicles for organic pollutants to enter marine organisms (Besseling et al., 2013; Bakir et al., 2014, 2016; Chua et al., 2014; Ziccardi et al., 2016; Hartmann et al., 2017; Rainieri et al., 2018) Under laboratory conditions, various polymer particles have been shown to adsorb chemical pollutants from the surrounding environment, with PE, PVC, PP, and PS displaying high sorption capacity for polycyclic aromatic hydrocarbons (PAHs), dichlorodiphenyltrichloroethane (DDT), hexachlorocyclohexanes, chlorinated benzenes, musks, pharmaceuticals, personal care products (Bakir et al., 2012; Lee et al., 2014; Wu et al., 2016; Ziccardi et al., 2016; Hartmann et al., 2017; Zhang et al., 2018). Various organic pollutants, including PAHs, polychlorinated biphenyls (PCBs), organo-halogenated pesticides, nonylphenol, and dioxins have been detected in plastic pellets from beaches worldwide (Ogata et al., 2009; Avio et al., 2015). Contamination in, and concentration of pollutants on MPs are of great importance as MPs may be ingested by marine organisms with contaminants having the potential to desorb and accumulate in fatty tissues due to their lipophilic nature, posing a long-term risk to the environment (Mato et al., 2001; Rios et al., 2007; Besseling et al., 2013; Avio et al., 2015; Bakir et al., 2016; Ziccardi et al., 2016; Batel et al., 2018). Exposure to organic chemicals adsorbed to MPs revealed biomarker responses at cellular and sub-cellular level, such as alterations in oxidative stress, immune and neurological responses, and gene expression profiles (Browne et al., 2013; Oliveira et al., 2013; Rochman et al., 2014; Avio et al., 2015; Paul-Pont et al., 2016). In mussels exposed to PS MPs with adsorbed fluoranthene, an increase in reactive oxygen species production in hemocytes was noted, along with high activities of anti-oxidant and glutathione-related enzymes (Paul-Pont et al., 2016). In another study with mussels exposed to PS and PE with adsorbed pyrene, negative, and greater effects were noted, when comparing with MPs without adsorbed chemicals, inducing changes in immune responses, antioxidant enzyme activities, neurotoxic, and genotoxic effects (Avio et al., 2015).
Known for its pro-carcinogenic properties, benzo[a]pyrene (BaP) (C20H12) is thought to be one of the most toxic PAHs and is classified by the International Agency for Research on Cancer as a group 1 human carcinogen (IARC, 2011; Liu et al., 2015; Châtel et al., 2017). Formed through the incomplete pyrolysis of combustible organic material, the main sources are anthropogenically derived from fossil fuel combustion, waste incineration, and oil spills. PAHs emitted, as soot or gas, to the atmosphere, enter the marine environment through rain and surface run-off (Antunes et al., 2013; Liu et al., 2015; Châtel et al., 2017). BaP is ubiquitously distributed in coastal and marine environment (Antunes et al., 2013; Liu et al., 2015; Châtel et al., 2017) and has been used as a model to investigate the effects and metabolic pathways of PAHs in marine organisms (Liu et al., 2015).
Perfluorooctane sulfonic acid (PFOS) has been classed as an emerging chemical of concern, along with its precursors—perfluoroalkylated acids, and is classified as a POP under the Stockholm Convention (Paul et al., 2009). Environmental contamination of PFOS may occur in two ways, either through direct release to the environment during manufacture and application, or indirectly, through degradation from precursor compounds. Under environmental conditions PFOS does not hydrolyse, photolyze, or biodegrade. Considered a widespread contaminant, PFOS has been recorded globally in seawater, human blood, and in the biota (Giesy and Kannan, 2001; Kannan et al., 2001; Yamashita et al., 2005). The chemical properties of PFOS, including high water solubility and negligible vapor pressure, imply that it will reside in surface waters once released into the environment (Paul et al., 2009). Although limited information is available on the volume of PFOS, and its precursors, released into the environment, empirical oceanographic data estimates that ~235–1,770 t of PFOS currently reside in oceanic surface waters (Paul et al., 2009). PFOS has been shown to bioaccumulate and biomagnify to higher trophic levels (Giesy and Kannan, 2001). Unlike other POPs, PFOS does not accumulate in fatty tissues, but binds to the protein albumin, mainly present in blood, liver, and eggs. As such the behavior of PFOS within the body is similar to that of fatty acids, with hydrophobic interactions playing a role in bioaccumulation (de Vos et al., 2008).
Scrobicularia plana is an environmentally relevant species to use as a bioindicator for evaluating the health status of coastal and estuarine ecosystems (Mouneyrac et al., 2008), playing a key role in their structure and functioning (Châtel et al., 2017). As burrowing deposit filter feeders, clams can assimilate particles, and associated contaminants, from both the sediments and the water column. Being positioned at the base of the food web, the clam is an important food source for crabs, fish, birds, and increasingly for human consumption (Rodríguez-Rúa et al., 2003; Langston et al., 2005, 2007).
In the present study, a battery of biochemical, cellular, and physiological biomarkers was analyzed in order to characterize the ecotoxicological potential of both virgin and contaminated LDPE microplastics, in the gills, digestive gland, and haemocytes of the peppery furrow shell clam, S. plana. Environmentally relevant concentrations of known marine contaminants, benzo[a]pyrene (BaP), and perfluorooctane sulfonic acid (PFOS), were adsorbed to microplastics to address both the potential for plastic particles to act as a vector of chemical exposure once ingested, and evaluate the effect of each respective contaminant. To clarify any effects of exposure, a set of biomarkers were employed, including the quantification of antioxidant (superoxide dismutase—SOD, catalase—CAT, glutathione peroxidase—GPx) and biotransformation (Glutathione-S-transferases—GST) enzyme activities, which play a role in detoxification under conditions of oxidative stress; lipid peroxidation (LPO) levels, indicative of oxidative damage; single and double strand DNA breaks to evaluate genotoxicity; the activity of the enzyme acetylcholinesterase (AChE), involved in neuro- and neuromuscular transmission; and the condition index, evaluated to assess the overall health status of the organisms.
Materials and Methods
Preparation and Characterization of Microplastics
Non fluorescent, low-density PE microparticles (0.96 g cm−3), MPP-635G (11–13 μm) were purchased from Micro Powders Inc. (NY-USA). Microplastics were spiked with BaP using 125 g L−1 of plastic, weighed into separate 250 mL narrow-mouth Septa bottles (Thermo scientific) filled with double-deionized water. Benzo[a]pyrene, CAS 50-32-8 (purity ≥ 96%) was purchased from Sigma Aldrich, and a concentration of 2,500 μg L−1 was used. Bottles were placed on a rotary shaker for two days at the lowest speed (20 rpm). Samples were filtered using a ceramic funnel and glass microfiber filters (1.0 μm, Whatman® glass microfiber filters, GE Healthcare Life Sciences). Samples were rinsed with double-deionized water, dried by vacuum evaporation and extracted in hexane (≥98%, SupraSolv). Extracts were sonicated and centrifuged at 2000 RCF. Extracts were filtrated trough fiberglass and transferred to toluene (purity 96%, SOLVECO). The sample volume was reduced to 500 μL using a nitrogen stream. Concentrations of BaP were quantified using a high-resolution GC-MS system (Micromass Autopspec Ultima), separation on a 30 m (0.25 mm i.d., 25 μm film thickness) DB-5MS column (J&W Scientific, Folsom, USA). Details about the instrumental method can be found in Larsson et al. (2013). As an internal standard, benzo[a]pyrene-d12 in toluene was added to the vial. Quantification was performed against perylene-d12 recovery standard, dissolved in toluene and purchased from Chiron. Final concentration of BaP adsorbed to LDPE (11–13 μm) MP was 16.87 (±0.22) μg g−1.
For the preparation of PFOS-spiked MPs, 50 g L−1 of plastic was weighed into a 1 L polypropylene bottle filled with 500 mL of double-deionized water. Heptadecafluorooctanesulfonic acid potassium salt (CAS 2785-37-3, purity ≥98%) was purchased from Sigma-Aldrich (Germany) and a concentration of 20 mg L−1 was used. The bottles were placed on a rotary shaker at the lowest speed (20 rpm) for 7 days. Filtration of the samples was performed using a funnel and glass microfiber filter (1.0 μm, Whatman® glass microfiber filters, GE Healthcare Life Sciences). Samples were rinsed with double-deionized water and dried by vacuum evaporation on a ceramic funnel. MPs were extracted in methanol (>99.9% purity, Fisher Scientific) by ultra-sonication followed by centrifugation (7000 RCF). Extracts were filtrated trough out a polyethylene syringe (Norm-Ject®, 5 mL, ref.4050-000V0) with a filter of 0.2 μm (AcrodiscGHP, 13 mm, 0.2 μm). Recovery standard was added with a mobile phase methanol and ammonium acetate (Fluka, Steinheim, Germany), 40%/60% (v/v). Analysis was performed on an Acquity UPLC system coupled to a Xevo TQ-S quadrupole mass spectrometer (Waters Corporation, Milford, U.S.A.). PFOS were separated on 100 mm Acquity BEH C18 column (2.1, 1.7 mm). Detailed description of LC method and instrumental settings can be found in the supplementary information of the publication by Eriksson et al. (2016). Final concentration of PFOS adsorbed to LDPE (11–13 μm) MP was 70.22 (±12.41) μg g−1.
Experimental Design
Clams (S. plana, 4 cm ± 0.5 cm) were collected from Cabanas de Tavira, Ribeira do Almargem, Southern Portugal (N 37°7′59.75′′W 7 36′34.95′′), transported to the laboratory alive, and acclimatized for 5–7 days in natural seawater, at constant aeration with a photoperiod of 12 h light to 12 h dark. Collection occurred in early February, during the period of sexual inactivity. Post acclimation, clams were transferred to 25 L aquaria containing a proportion of 1:4 of sediment/ seawater. The height of the water column was 18 cm above the 5 cm of sediment layer. Sediment, previously collected from the top 30 cm at the same site, was passed through a 4 mm sieve to remove any macro-organisms and debris, and dried at 65°C for 48 h to remove organic matter, volatile compounds, and water (Maranho et al., 2014). Sediment was rehydrated, to the same original sediment moisture content (%) as when collected, calculated by the difference in the wet weight of a known volume of sediment after reaching constant dry weight at 65°C.
The exposure experiment consisted of 8 aquaria (each containing 85 clams), with 4 treatments (control, virgin LDPE, BaP contaminated LDPE, and PFOS contaminated LDPE) in a duplicate design. All aquaria, excluding the two controls, were exposed to LDPE microplastics (11–13 μm) at a concentration of 1 mg L−1 for a duration of 14 days. The exposure to microplastics was conducted by adding microplastics to the water column. The mixing of microplastics in the entire water column was possible (and visible) by the relatively strong aeration supplied to each aquarium.
Water was changed every 72 h, with the subsequent re-application of microplastics. Abiotic parameters; water temperature (19.31 ± 0.21°C), oxygen saturation (95.78 ± 1.77%), salinity (34 ± 1 ppt), and pH (8.05 ± 0.04), were measured using a multiparametric probe (ODEON V3.3.0). Mortality was observed in all aquaria, with the highest being in the control and LDPE treatments (7%), followed by LDPE+PFOS (5%), and LDPE+BaP (3%) treatments. Food was not supplied during the exposure period to minimize interactions of microplastics with other suspended particles. Glass Pasteur pipettes were attached to the end of plastic aeration tubes to avoid plastic contamination.
Individuals were randomly sampled from each aquarium before the addition of microplastics (day 0), and after 3, 7, and 14 days of exposure. Haemolymph was extracted from the posterior adductor muscle of S. plana at each sampling time, using a 1.5 mm sterile hypodermic syringe with an attached needle and used for genotoxic analysis. Gills and digestive gland tissues were dissected immediately, flash-frozen in liquid nitrogen and stored at −80°C for later analysis. Whole clams, to be used for chemical analysis, were frozen at −20°C for chemical analysis. Microplastics contents were not analyzed in the clams tissues.
Tissue Chemical Analysis
BaP was quantified in the whole soft tissues of freeze-dried clams using the method previously described by De Witte et al. (2014), with some modifications. 6 clams per treatment and per time (at days 0 and 14) were analyzed. Briefly, the samples were extracted by accelerated solvent extraction (Dionex, ASE350), using a mixture of hexane and acetone 3:1 at 100°C. After two evaporation steps, 5 μL of each sample was injected on a GC (Agilent 7890A) equipped with a PTV-injector (Gertsel, 6495-U). An Agilent 5975C MS-detector with electron impact ionization in single-ion mode was used for detection. As an internal standard, chrysene-d12 in toluene was added to the vial. Quantification was performed against benzo[a]pyrene-d12 recovery standard, dissolved in iso-octane and purchased from LGC-standards. The analysis is accredited by BELAC under the ISO/IEC 17025 standard, with a quantification limit of 1.65 ng g−1 dw.
Condition Index
The gravimetric condition index (CI) was assessed in 6 individuals per treatment, at each sampling time (day 0, 3, 7, and 14 of exposure), to determine the physiological status of both control and exposed clams. Tissues were dried at 80°C until a constant dry weight was achieved. The CI was estimated by calculating the percentage (%) of the ratio between dry weight of the soft tissues (g) and the dry weight (g) of the shell (Walne, 1976).
DNA Damage
DNA damage was determined in 6 individuals per treatment and per time (pre-exposure and 14 days exposure) using a slightly modified alkaline comet assay (Singh et al., 1988) as described by Almeida et al. (2013).
Post extraction, haemolymph cells were centrifuged at 3,000 rpm for 3 min at 4°C, and the supernatant removed. The DNA pellet was re-suspended in phosphate buffered saline (PBS) solution and low melting point agarose (LMA 0.65%, dissolved in Kenny's salt solution). DNA cell suspensions were cast on microscope slides previously coated with normal melting point agarose (NMA 0.65%, dissolved in Tris-acetate EDTA). One slide, with two replicate agarose gels embedded with cells, was prepared per sample. Slides were immersed in Lysis buffer (2.5 M NaCl, 100 mM EDTA, 10 mM Tris, 1% Sarcosil, 10% Dimethylsulfoxide, 1% Triton X 100, pH 10) at 4°C in the dark for 1 h, enabling cell lysis. Microscope slides were rinsed with ultrapure water (Milli-Q), placed in an electrophoresis chamber, submerged in buffer (300 mM NaOH, 1 mM EDTA, ultrapure water, pH > 13, at 4°C) for 15 min prior to running the current, to allow DNA to unwind. Electrophoresis was run under the following conditions: 25 V, 300 mA, for 5 min. Slides were removed, immersed in neutralization solution (0.4 mM Tris, pH 7.5), rinsed with ultrapure water, and allowed to dry, in the dark at room temperature. Once dry, slides were stained with 4,6-diamidino-2-phenylindole (DAPI). Each well was examined using an optical fluorescence microscope (Axiovert S100), under 400x magnification. 25 photographs of randomly chosen, individual cell nuclei were taken from each well (50 for each slide/sample), using a camera (Sony) attached to the microscope. Photographs were analyzed using the Komet 5.5 image analysis system (Kinetic Imaging Ltd). DNA damage was quantified by measuring the displacement between the genetic material of the cell nucleus (“comet head”) and the migrating comet “tail” with both comet tail length and olive tail moment parameters assessed. Results are expresses as mean ± standard deviation (SD).
Antioxidant and Biotransformation Enzyme Activity
Enzyme activities (SOD, CAT, GPx, and GST) were assessed in the gills and digestive gland tissues of 6 individuals per treatment, at each sampling time (0, 3, 7, and 14 days). Tissues were defrosted, weighed and homogenized, on ice, in 5 mL of Tris sucrose buffer (Sucrose 0.5 M, Tris 20 mM, KCL 0.5 M, DTT 1 M, EDTA 1 mM, at pH 7.6). The homogenate was centrifuged at 500 × g, at 4°C for 15 min. The supernatant was separated and re-centrifuged at 12,000 × g, at 4°C for 45 min. The cytosolic fraction was divided into 5 aliquots, stored in Eppendorf tubes and frozen at −80°C for the determination of SOD, CAT, GPx, and GST activities as well as total protein concentrations. Percentage inhibition in the reduction of cytochrome c by the superoxide anion generated by the xanthine/hypoxanthine system, measured at 550 nm (McCord and Fridovich, 1969) was used to determine SOD activity, expressed in Units (U) mg−1 of total protein concentration, where 1 U of activity corresponds to the amount of sample required to cause 50% inhibition. CAT activity was determined by measuring the consumption of hydrogen peroxide (H2O2) at 240 nm, as described by Greenwald (1987), with results expressed as μmol min−1 mg−1 of total protein concentration. GPx activity was determined through the reduction in NADPH, in the presence of glutathione reductase (GR) and reduced glutathione (GSH) using a cumene hydroperoxide probe (Lawrence and Burk, 1978). Measured at 340 nm, the decrease in NADPH is directly proportional to GPx activity. Results are expressed as nmol min−1 mg−1 of total protein concentration. GST activity was determined by the conjugation of 1-chloro 2,4 dinitrobenzene (CDNB) with reduced glutathione (GSH) with the resulting increase in absorbance measured at 340 nm (Habig and Jakoby, 1981). Results are expressed in nmol CDNB min−1 mg of total protein concentration.
Acetylcholinesterase (AChE) Activity
AChE activity was assessed in the gills of 6 individuals per treatment, at each sampling time (day 0, 3, 7, and 14) following the protocol modified from Ellman's colorimetric method (Ellman et al., 1961). Tissues were defrosted, weighed and homogenized, on ice, in 5 mL of Tris HCL buffer (100 mM, pH 8.0) and 50 μL of Triton—X 100 (0.1%). The homogenate was centrifuged at 12,000 × g, at 4°C for 30 min. Acetylthiocholine degradation rate was determined through the increase in 5-mercapto-2-nitrobenzoate, a compound of yellow color produced due to the non-enzymatic reaction of thiocoline with 5,5′-dithio-bis (2- nitrobenzoic acid). Absorbance was read at 405 nm and results expressed in nmol ACTC min−1 mg of total protein concentration.
Lipid Peroxidation (LPO)
LPO levels were quantified in gill and digestive gland tissues of 6 clams per treatment, at each sampling time (0, 3, 7, and 14 days of exposure) following the colorimetric method described by Erdelmeier et al. (1998). Tissues were defrosted, weighed and homogenized on ice, in 5 mL of Tris HCL buffer (0.02 M, pH 8.6) and 50 μL of butylated hydroxytoluene solution (BHT). The homogenate was centrifuged at 30,000 × g, at 4°C for 45 min. Lipid peroxidation levels were determined through the quantification of malondialdehyde (MDA) and 4-hydroxyalkenals (4-HNE) concentrations upon decomposition by polyunsaturated fatty acid peroxides. Supernatant (200 μL) was mixed with 1-methyl-2-phenylindone diluted in methanol (650 μL), and methanesulfonic acid (150 μL, 15.4 M), and incubated at 45°C for 60 min. Following incubation, the mixture was centrifuged at 15,000 × g, at 4°C for 10 min. Absorbance was read at 586 nm and results expressed in nmol of MDA mg −1 of total protein concentration.
Total Protein Concentration
Total protein concentrations were determined in the cytosolic fraction of gill and digestive gland tissues, of 6 individuals per treatment and per sampling time (0, 3, 7, and 14 days), post homogenisation, using the Bradford Assay (Bradford, 1976). Concentrations (mg ml−1) were used to normalize enzyme activities and LPO levels.
Statistical Analysis
Analyses were performed using R 3.3.1 software (R Core Team, 2016). Data are expressed as mean ± SD. Biomarker results were compared using two-way ANOVA, with polymer type and time as variables. Significant ANOVA results were analyzed using Tukey's HSD test. Chemical concentrations in tissues were compared using the non-parametric Mann–Witney U-test. A p-value ≤ 0.05 was considered statistically significant. Principal component analysis (PCA) was used to evaluate the relationship between biomarkers, BaP concentration, and the different treatments along the exposure period per contaminated LDPE MP.
Results
Condition Index
The CI of the organisms' pre-exposure (day 0) was 7.7 ± 2.7%. No significant differences were found between control (7.6 ± 0.4%) and virgin MP treatments (7.7 ± 0.3%) (p > 0.05), nor between virgin MP and contaminated MP treatments (LDPE+BaP 7.4 ± 0.5%, LDPE+PFOS 7.4 ± 0.9%, p > 0.05). No significant changes were observed between sampling times of the same treatment (p > 0.05). Results, expressed as treatment means ± SD, indicate that S. plana clams remained in good health for the duration of the experiment.
Virgin LDPE MP
Genotoxic effects, analyzed using the alkaline comet assay, for control and virgin LDPE treatments are displayed in Figure 1, with results expressed as comet tail length and olive tail moment. No significant differences were found between virgin LDPE and control treatments, nor between different time points of the same treatment, when quantifying DNA damage through the resulting comet tail length or OTM (Figure 1) (p > 0.05).
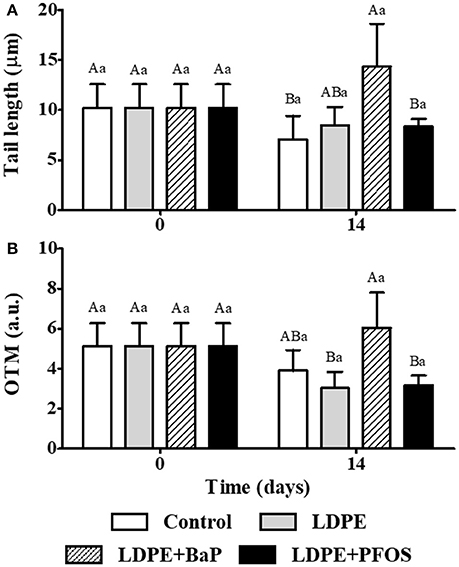
Figure 1. DNA damage (mean ± SD) in the haemocytes of S. plana, expressed as Tail length (μm) (A) and OTM (B) for control, virgin LDPE, LDPE+BaP, and LDPE+PFOS treatments. Different capital letters indicate a significant difference between treatments within the same time. Different lowercase letters indicate a significant difference for the same treatment between times (p < 0.05). a.u. = arbitrary units.
No significant differences were found between virgin LDPE and control treatments, neither through time, when quantifying SOD activity in the gills of S. plana (p > 0.05) (Figure 2A). A significant difference in digestive gland SOD activity occurred between treatments with the control displaying significantly higher activities than the virgin LDPE after 3 days of exposure (p < 0.05). SOD activity in the digestive gland of virgin LDPE treatments remained unchanged over time (p > 0.05) (Figure 3A).
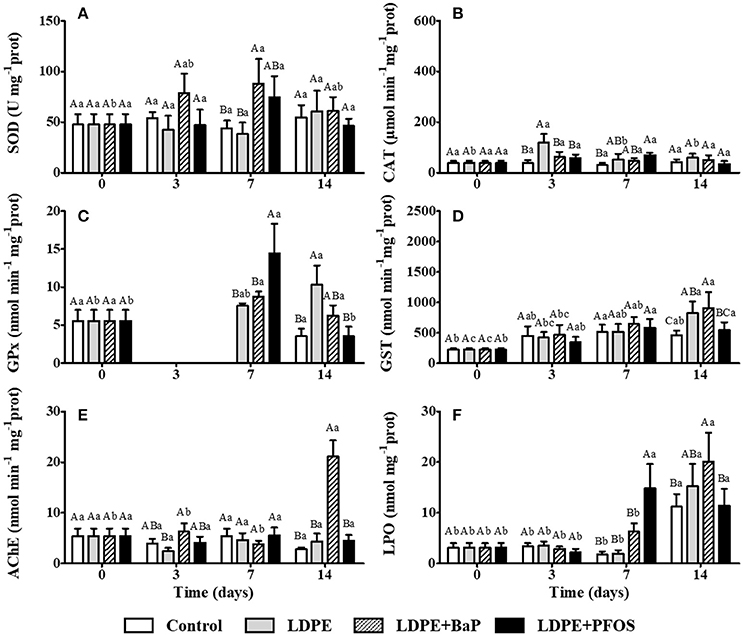
Figure 2. SOD (A), CAT (B), GPx (C), GST (D), AChE (E), and LPO (F) activities/levels (mean ± SD) in gill tissues of S. plana for control, virgin LDPE, LDPE+BaP, and LDPE+PFOS treatments. Different capital letters indicate a significant difference between treatments within the same time. Different lowercase letters indicate a significant difference for the same treatment between times (p < 0.05).
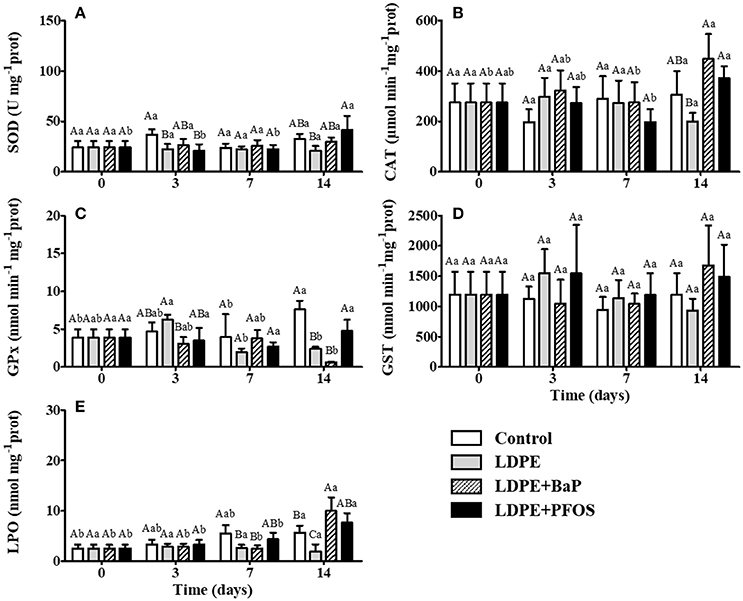
Figure 3. SOD (A), CAT (B), GPx (C), GST (D), and LPO (E) activities/levels (mean ± SD) in digestive gland tissues of S. plana for control, virgin LDPE, LDPE+BaP, and LDPE+PFOS treatments. Different capital letters indicate a significant difference between treatments within the same time. Different lowercase letters indicate a significant difference for the same treatment between times (p < 0.05).
A tissue specific response is observed in CAT activity, with a 2 to 3-fold higher activity in the digestive glands relative to gills, for both treatments (control and virgin LDPE) (Figures 2B, 3B). Exposure to virgin LDPE caused a significant increase in CAT activity in gill tissues after 3 days, significantly differing to the control at this time (p < 0.05) (Figure 2B). CAT activity in the gills subsequently decreased at day 7 and 14, with a significant difference occurring between tissues exposed to virgin LDPE after 3 days of exposure and both these times (p < 0.05). No significant differences were observed in CAT activity of digestive gland tissues between control and virgin LDPE treatments, nor over time for either treatment (p > 0.05) (Figure 3B).
GPx activity was highly variable among tissues and treatments, being null in gill tissues at day 3 for all treatments and control at day 7. A significant increase in GPx activity was observed in the gill tissues of virgin LDPE treatment after 14 days of exposure, relative to both pre-exposure and the control at day 14 (p < 0.05) (Figure 2C). In the digestive gland, a significant decrease in GPx activity in virgin LDPE treatments is observed after 7 days, remaining with similar levels at day 14 (p < 0.05), when compared to day 3 (Figure 3C).
GST activity increases steadily in gill tissues of organisms exposed to virgin LDPE during the experimental period (Figure 2D). Relative to pre-exposure, a significant increase in GST activity in the gills is first observed after 7 days (p < 0.05), with a significant difference in activity occurring between day 14 and pre-exposure and day 3 (p < 0.05). Significantly higher GST activity in the gills occur in virgin LDPE relative to control after 14 days of exposure (p < 0.05) (Figure 2D). No significant differences in GST activity in the digestive gland tissues were observed between treatments within the same exposure time, neither through time for each treatment (p > 0.05) (Figure 3D).
No significant differences were observed in AChE activities of gill tissues between control and virgin LDPE treatments, nor over time for either treatment (p > 0.05) (Figure 2E).
LPO levels in gill tissues remained stable for both control and virgin LDPE exposed treatments, until day 14 when a significant increase occurred, with both increasing simultaneously (p < 0.05) (Figure 2F). Levels significantly differ at this time for both treatments in respect to all previous times (p < 0.05). No significant difference in LPO levels in gill tissues occurred between control and virgin LDPE treatments over the 2-week experimental period (p > 0.05) (Figure 2F). LPO levels in digestive gland tissues remained stable for the duration of the experiment in virgin LDPE exposed treatments (p > 0.05) (Figure 3E). Significantly lower LPO levels were observed in digestive gland tissues exposed to virgin LDPE on days 7 and 14 when compared to controls (p < 0.05) (Figure 3E).
BaP Contaminated LDPE MP
Background BaP contamination (<1.6 ng g−1 dw) occurred in whole tissues of clams from all treatments (Figure 4), with levels remaining stable over time in both control and virgin LDPE treatments (p > 0.05). A significant increase in BaP concentration in whole tissues occurred after 14 days exposure to LDPE+BaP (p < 0.05), reaching 7.3 ± 2.0 ng g−1 dw (Figure 4).
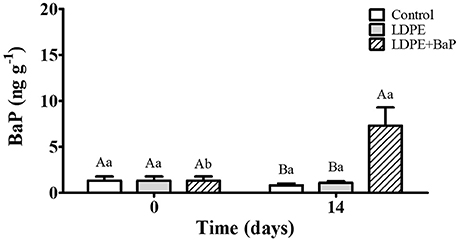
Figure 4. S. plana whole tissue BaP concentrations (ng g−1) in control, virgin LDPE, and LDPE+BaP treatments. Different capital letters indicate a significant difference between treatments within the same time. Different lowercase letters indicate a significant difference for the same treatment between times (p < 0.05).
The tail length of haemocyte cells was significantly higher in LDPE+BaP treatments when compared to control after 14 days exposure (p < 0.05) (Figure 1A). When quantifying DNA damage through OTM, significantly higher OTM values were observed in LDPE+BaP treatments after 14 days of exposure when compared to virgin LDPE (p < 0.05) (Figure 1B) No significant differences were observed between times for LDPE+BaP treatments for both tail length and OTM (p > 0.05).
An increase in SOD activity in the gills of S. plana was noted after 3 days of exposure to LDPE+BaP, being significantly higher after 7 days when compared to control and virgin LDPE treatments (p < 0.05) (Figure 2A). This increase in activity was also significantly higher when compared to time 0 (p < 0.05). SOD activity remained stable in digestive gland tissues of LDPE+BaP treatments for the duration of the experiment (p > 0.05) (Figure 3A).
CAT activity in the gills of organisms exposed to LDPE+BaP remained stable during the experiment (p > 0.05) (Figure 2B). A significantly higher activity occurs in virgin LDPE when compared to LDPE+BaP treatments after 3 days of exposure (p < 0.05). A significant increase in CAT activity in digestive gland tissues of organisms exposed to LDPE+BaP was noted after 14 days exposure, when compared to virgin LDPE (p < 0.05) (Figure 3B). The increase in CAT activity in digestive gland was also significantly higher in LDPE+BaP treatment after 14 days exposure when compared to the start and day 7 of exposure (p < 0.05).
Exposure to LDPE+BaP did not induce a significant response in GPx activity in gill tissues through time nor relative to control and virgin LDPE (p > 0.05) (Figure 2C). A significant decrease in GPx activity in the digestive gland tissues of LDPE+BaP treatments occurs after 14 days exposure, when compared with pre-exposure values and to control at this time (p < 0.05) (Figure 3C). Significantly lower GPx activities were noted after 3 days exposure in LDPE+BaP when compared to virgin LDPE (p < 0.05) (Figure 3C).
Exposure to LDPE+BaP induces a steady increase through time in GST activity in gill tissues, with a significant increase observed after 7 and 14 days, when compared to pre-exposure (p < 0.05) (Figure 2D). After 14 days GST activities in gill tissues are significantly higher in both virgin LDPE and LDPE+BaP treatments than the control (p < 0.05). GST activity in the digestive gland tissues remained stable over time in each respective treatment and no significant differences were found between treatment per exposure time (p > 0.05) (Figure 3D).
A significant increase in AChE activity in LDPE+BaP treatments at day 14 of exposure was noted when compared to other exposure times (p < 0.05) (Figure 2E). At this time, LDPE+BaP AChE activity is significantly higher when compared with both virgin LDPE and control treatments (p < 0.05).
Exposure to LDPE+BaP induced a significant increase in LPO levels after 14 days in both gill and digestive gland tissues (p < 0.05) (Figures 2F, 3E). Levels at this time significantly differ to all previous times of LDPE+BaP exposure for both tissues (p < 0.05). Significantly higher LPO levels after 14 days occurred between LDPE+BaP when compared to control treatments in the gills (p < 0.05) (Figure 2F), and between LDPE+BaP and both the control and virgin LDPE treatments in the digestive gland tissues (p < 0.05) (Figure 3E).
Principal component analysis was applied to BaP concentration and biomarkers data for days 0 and 14, for all treatments (control, virgin LDPE, and LDPE+BaP) (Figure 5). The two principal components represent 87.3% of variation within the data, with PC1 accounting for 54.6% and PC2 32.7%. A clear separation exists between the control (T0 and T14) and LDPE+BaP, and also for virgin LDPE treatment, after 14 days exposure. PC1 is negatively determined by treatment LDPE+BaP after 14 days exposure, with the remaining treatments being placed on the positive side of the axis. BaP concentration is positively related with LDPE+BaP treatment T14, as most of the biomarkers analyzed, except GPx. Results obtained for AChE, tail length, and also for LPO and CAT in the digestive gland are strongly related to the concentration of BaP, determined in whole tissue. A differential tissue response is noted with biomarkers results for digestive gland remaining on the positive side of PC2 axis, while biomarkers data for gills remain on the negative side of PC2. Control treatments appear on the positive side of axis for PC2, while virgin LDPE remains on the negative side of the axis that is positively influenced by most of the biomarkers responses for gills (Figure 5).
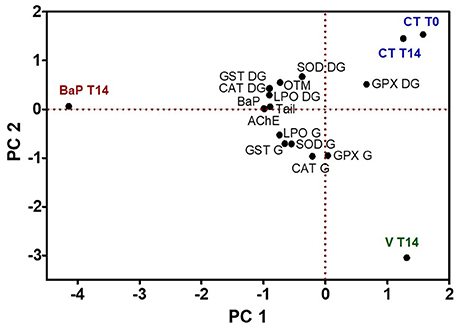
Figure 5. Principle component analysis (PCA) of a battery of biomarkers in the gills (G), digestive glands (DG), and haemocytes (expressed as Tail and OTM) of S. plana, in control (CT), virgin LDPE (V), and LDPE+BaP (BaP) treatments, over the duration of the experiment, where T0 = day 1 and T14 = day 14.
PFOS Contaminated LDPE MP
No significant differences were observed between control and LDPE+PFOS treatments, nor between virgin LDPE and LDPE+PFOS treatments when quantifying DNA damage through the resulting comet tail length or OTM (p > 0.05) (Figure 1). LDPE+PFOS treatment after 14 days did not show any significant difference when compared with pre-exposure (p > 0.05).
A slight increase in SOD activity in the gill tissues was observed following 7 days exposure to LDPE+PFOS but no significant differences were noted when compared to pre-exposure, neither with control or virgin LDPE treatments (p > 0.05) (Figure 2A). SOD was significantly induced in digestive gland tissues after 14 days exposure to LDPE+PFOS when compared to previous exposure times (p < 0.05), with activity being significantly higher than that of the virgin LDPE treatment at this time (p < 0.05) (Figure 3A).
CAT activity in the gills of organisms exposed to LDPE+PFOS remained stable during the experiment (p > 0.05) (Figure 2B). A significant difference in activity occurs between virgin LDPE and LDPE+PFOS treatments in gill tissues after 3 days of exposure, following an increase of activity in tissues exposed to virgin LDPE (p < 0.05). After 7 days exposure, a significant difference is observed in CAT activity, of gill tissues, between the control and LDPE+PFOS (p < 0.05) (Figure 2B). A significant difference in CAT activity in the digestive gland of organisms exposed to LDPE+PFOS occurs between day 7 and 14 of exposure, with higher activities occurring at day 14 (p < 0.05), yet activities at these times are comparable to both day 3 and pre-exposure levels (p > 0.05) (Figure 3B). After 14 days of exposure significantly higher CAT activity levels were found when compared to virgin LDPE (p < 0.05) (Figure 3B).
GPx activity in gill tissues of virgin LDPE and LDPE+PFOS treatments significantly differ after 7 days exposure, with LDPE+PFOS inducing an increase in activity relative to virgin LDPE (p < 0.05) (Figure 2C). Activity is significantly reduced in gill tissues for LDPE+PFOS treatment by day 14 when compared to day 7, with GPx activity comparable to pre-exposure levels (p < 0.05). A significant difference in GPx activity in the gills occurs between virgin LDPE and LDPE+PFOS at day 14 (p < 0.05) (Figure 2C). GPx activities remain stable for the duration of the experiment in the digestive glands of organisms exposed to LDPE+PFOS (p > 0.05) (Figure 3C). On day 14 significantly higher GPx activities are observed in digestive gland tissues of LDPE+PFOS when compared to virgin LDPE (p < 0.05) (Figure 3C).
When compared to pre-exposure, LDPE+PFOS treatment induces a significant increase in GST activity in gill tissues after 7 days exposure with levels remaining high at day 14 (p < 0.05) (Figure 2D). GST activities remain stable for the duration of the experiment in the digestive glands of organisms exposed to LDPE+PFOS (p > 0.05) (Figure 3D). No significant differences are observed in digestive gland tissues between, control, virgin LDPE, and LDPE+PFOS treatments (p > 0.05) (Figure 3D).
No significant difference in AChE activity in the gills of S. plana exposed to LDPE+PFOS occurred during the experimental period (p > 0.05) (Figure 2E). Likewise, no significant differences were observed in AChE activity between the different treatments at any exposure time (p > 0.05) (Figure 2E).
LPO levels are comparable in both gill and digestive gland tissues until day 7 of exposure when a > 2-fold increase in LPO levels is observed in the gills of LDPE+PFOS treatments (Figures 2F, 3E). LPO levels in gill tissues at this time are significantly higher than control and virgin LDPE treatments (p < 0.05) (Figure 2F). In gills, LPO stabilizes between day 7 and day 14 of exposure to LDPE+PFOS, being significantly higher when compared to both pre-exposure and day 3 (p < 0.05) (Figure 2F). LPO levels remain stable in digestive gland tissues of LDPE+PFOS treatments until 14 days of exposure when a significant increase is observed (p < 0.05) (Figure 3E). LPO levels in digestive gland tissues of LDPE+PFOS treatments are significantly higher at this time than in that of virgin LDPE treatments (p < 0.05) (Figure 3E).
Principal component analysis was applied to all biomarkers data for days 0 and 14, for all treatments (control, virgin LDPE, and LDPE+PFOS) (Figure 6). 87.2% of the variation within the data is explained by two principal components, with PC1 accounting for 53.3 and PC2 33.9%. PC1 is positively determined by virgin LDPE treatment after 14 days exposure, with the remaining treatments being placed on the opposite side of the axis. A clear tissue specific biomarkers response is noted given the separation by tissues observed in the PCA, with gills biomarkers placed on the positive side of the PC1 axis and digestive gland biomarkers agglomerated on the negative side of PC1 axis. Gill biomarkers are positively related to virgin LDPE after 14 days of exposure, while digestive gland biomarkers are positively related to LDPE+PFOS after 14 days exposure, along PC1 axis. AchE and DNA damage biomarkers have a similar response among them, and positively related with pre-exposure (CT T0) and the positive side of PC2 axis (Figure 6).
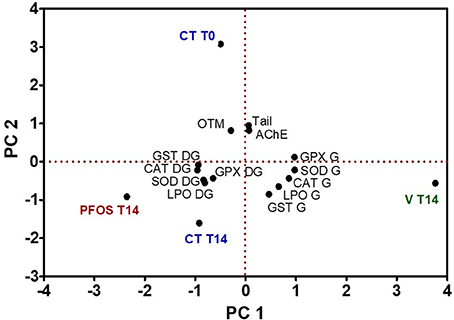
Figure 6. Principle component analysis (PCA) of a battery of biomarkers in the gills (G), digestive glands (DG), and haemocytes (expressed as Tail and OTM) of S. plana, in control (CT), virgin LDPE (V), and LDPE+PFOS (PFOS) treatments, over the duration of the experiment, where T0 = day 1 and T14 = day 14.
Discussion
Microplastics are a major contaminant in the marine environment and are bioavailable to benthic organisms. Deposition of low density plastics may be biologically mediated, through the formation of biofilms, through excretion in fecal pellets, or caught in marine snow (Lobelle and Cunliffe, 2011; Zalasiewicz et al., 2016). Deposition of low density plastics may also occur because of density changes resulting from mineral adsorption while in the water column (Corcoran et al., 2015). Little information has been reported on the environmental concentrations of microplastics < 50 μm in size (Ribeiro et al., 2017). As such, the question of an environmentally relevant concentration of microplastics to be used in laboratory studies may also vary. A concentration of 1 mg L−1 was decided upon to encompass measured environmental concentrations of MP in both sediment and water compartments of the world's Oceans.
A battery of biomarkers was used to assess the biological effects and toxicity of LDPE microplastics in the gills, digestive glands, and haemocytes of S. plana. The potential for microplastics to act as a vector for chemical exposure was investigated by comparing biomarker activities between organisms exposed to virgin LDPE and contaminated LDPE microplastics.
No significant enhancement in DNA strand breaks were detected in the haemocytes of S. plana clams exposed to virgin LDPE MP or to LDPE+PFOS MP (Figure 1). There is some evidence to suggest that exposure to LDPE+BaP MP induces DNA damage after 14 days, yet the strength of these results is low. The comet assay has been proven to be a robust, sensitive and cost-effective tool for assessing genotoxicity in haemocyte cells of S. plana (Petridis et al., 2009). Studies have indicated the genotoxic potential of both virgin polystyrene MP in S. plana, and virgin PE MP in M. galloprovincialis, through a significant increase in DNA strand breaks in haemocyte cells (Avio et al., 2015; Ribeiro et al., 2017).
Results indicate a time- and tissue-dependent oxidative stress response that varies depending on treatment used and biomarker investigated (Figures 2, 3). Antioxidant defence systems, comprised of antioxidant enzymes such as superoxide dismutase (SOD), catalase (CAT), and glutathione peroxidase (GPx), play a crucial role in maintaining cellular homeostasis by the removal of ROS (Jo et al., 2008).
A significant increase in SOD activity is observed in gill tissues exposed to LDPE+BaP after 7 days when compared with pre-exposure and with virgin MP treatment (Figure 2A). Such an effect is only observed in the digestive gland tissues of organisms exposed to LDPE+PFOS after 14 days (Figure 3A). An increase in SOD activity is indicative of the first line of defence in protecting tissues against oxidative stress. SOD, found in every living organism that consumes oxygen, catalyzes the partitioning of the superoxide anion radical (O2•−1) into hydrogen peroxide (H2O2) and water, thus reducing the potential for oxidative damage to occur (Jo et al., 2008). Results in the gill tissues of contaminated MP treatments are comparable to those reported for virgin PS exposure in the gills of S. plana by Ribeiro et al. (2017). Exposure to virgin LDPE does not induce the same time dependent increase in SOD activity, in either tissue, as exposure to virgin PS at the same concentration, 1 mg L−1 (Ribeiro et al., 2017). As SOD activity remained stable for the duration of the experiment in both gill and digestive gland tissues exposed to virgin MP, and did not significantly differ to that of the control in the majority of cases, it may be hypothesized that the observed increase results from the toxicity of the adsorbed contaminants.
The extent to which microplastics act as a vector for contaminants once ingested by organisms within the marine environment is still unclear, as in field conditions organisms may accumulate the same chemical contaminants from other sources (Oliveira et al., 2013). Laboratory studies have demonstrated the desorption of contaminants from ingested microplastics, including PCBs from ingested microplastics in shearwater chicks (Teuten et al., 2009), yet it must be noted that in field conditions environmental contaminants will rarely exist solely, but do so as a mixture with varying concentrations. Before guideline values for the environmental risk assessment of plastic debris can be produced, further research is needed into the adsorption and desorption kinetics of common marine contaminants to various plastic polymers, and their effects on the marine biota once ingested. The ecological impact of MP ingestion may be significantly increased by desorption of toxicants. Desorption of chemicals from ingested MP is dependent on the residence time of the particles (Engler, 2012), which in turn is dependent on the particle size (Lusher, 2015). PS MP particles have been shown to accumulate in the digestive gland and gill tissues of S. plana in a laboratory experiment by Ribeiro et al. (2017), residing in both tissues for the 1 week depuration period investigated.
A 5-fold higher activity of CAT in the digestive gland when compared to gills was noted in all treatments and times (Figures 2B, 3B). The antioxidant enzyme CAT, prevents cellular damage from ROS by reducing both endo- and exogenous sources of H2O2 to H2O (Oliveira et al., 2009; Solé et al., 2009). CAT induced activity was not observed in gill tissues of the LDPE+BaP MP treatment, but an increase was noted after 7 days of exposure to LDPE+PFOS when compared to control. In the digestive gland an increase in CAT activity was noted after 14 days for both LDPE+BaP and LDPE+PFOS when compared to virgin LDPE. Moreover, a significant induction in CAT activity in gill tissues occurred after 3 days exposure to virgin LDPE MP, with the activity being subsequently reduced over time reaching levels comparable to pre-exposure by day 14. Previous studies have observed an inhibition of CAT activity in response to MP exposure, both in the digestive gland of S. plana exposed to PS MP, and in the digestive gland of M. galloprovincialis exposed to both virgin PE and PS, and pyrene contaminated PE and PS (Avio et al., 2015; Ribeiro et al., 2017). It may be argued that CAT is not the antioxidant defence mechanism used by S. plana in response to PE MP exposure, or that 2 weeks exposure to both virgin LDPE and contaminated LDPE MP, under the experimental conditions observed, is not sufficient to induce a significant response.
A defence mechanism reaction to oxidative stress is suggested by the significant increase in GPx activity in the gills of virgin LDPE MP treatment by day 14 (Figure 2C). A significant increase followed by a subsequent decrease in activity is observed in the gills of LDPE+PFOS treatments (Figure 3C). Glutathione peroxidases which catalyze the reduction of metabolically produced H2O2 to H2O and O2, have been proven to be a sensitive biomarker in revealing pro-oxidant challenges, even at low levels of environmental contamination (Jo et al., 2008; Regoli and Giuliani, 2014). Previous studies have shown that an inhibition in GPx may occur in response to elevated toxicity levels. Such inhibition was demonstrated in mussels (M. galloprovincialis) exposed to virgin and pyrene contaminated PE and PS MP (Avio et al., 2015). A similar trend was observed in whole tissues of S. plana exposed to mercury, with higher mercury levels inhibiting GPx activity (Ahmad et al., 2011). This hypothesis—that an inhibition of GPx activity occurs due to the inability to process excess ROS—may explain the antagonistic effects observed in the fore-mentioned treatments.
A tissue dependent response was observed in regard to GST, with the digestive gland displaying little variation in activity (Figures 2D, 3D). An increase in GST activity in gill tissues is observed in all MP treatments over time, although less pronounced in LDPE+PFOS. Gill tissues, being the major organ involved in filtration, have been demonstrated to be the first site of MP particle uptake in mussels (Browne et al., 2008; von Moos et al., 2012; Ribeiro et al., 2017), with S. plana also ingesting particles through the inhalant siphon, which are subsequently transported to the mouth and digestive gland (Hughes, 1969). A similar increase in GST activity in the gills of S. plana has been described by Ribeiro et al. (2017) in response to PS MP exposure. GST activity has also been reported to increase in the gills of M. galloprovincialis following exposure to the persistent organic pollutant pp'DDE (2,2-bis-(p-chlorphenyl)-1,1-dichlorethylene), a metabolite of DDT (Khessiba et al., 2001). The phase two enzymes, glutathione-S-transferases (GST), play an important role in cellular protection against various xenobiotics and toxic endogenous substances by converting reactive lipophilic molecules into non-reactive water-soluble molecules which can be excreted by the organism (Hoarau et al., 2002). As a significant increase in GST activity in gill tissues of contaminated LDPE MP treatments compared to virgin LDPE MP is not observed, it may be hypothesized that synergistic effects of ingestion and chemical exposure did not occur, indicating that the overall increase in activity with time results from the physical ingestion of MP, rather than the chemical toxicity of the respective contaminants.
It may be hypothesized, from the overall results on oxidative stress biomarkers, that ROS are produced as a result of short term exposure to both virgin LDPE and contaminated microplastics. Such stress may lead to lipid peroxidation, protein denaturation as well as cellular and DNA damage (Jo et al., 2008).
Inhibition of AChE activity did not occur in any of the MP exposed treatments (Figure 2E), with LDPE+BaP MP treatments showing a significant increase after 14 days of exposure. In contrast, a reduction in AChE activity in gill tissues of S. plana was observed following 2 weeks exposure to polystyrene microplastics at similar concentrations (Ribeiro et al., 2017). Previous studies have indicated that a reduction in AChE activity in the gills of mussels (M. galloprovincialis) occurred upon 7 days exposure to polystyrene and polyethylene microplastics, with and without pyrene contamination (Avio et al., 2015). Comparisons between these, and other MP feeding experiments, are confounded by numerous factors: different species used, different polymer concentrations used (1.5 g L−1 by Avio et al., 2015 compared to 1 mg L−1 in this experiment, a difference of 1500x), different polymer type (when comparing polyethylene, densities, and sizes differ), different contaminants used and different exposure times. Exposure to polyethylene microplastics, with and without added pyrene have also shown to significantly reduce AChE activity in juveniles of the common goby, Pomatoschistus microps (Oliveira et al., 2013). No significant changes occur in AChE activity in either virgin LDPE or LDPE+PFOS MP treatments, suggesting that a neurotoxic potential of LDPE MP without adsorbed contaminants does not exist under the experimental conditions of the present study.
There is a clear effect of lipid peroxidation in the gills of organisms exposed to LDPE+BaP by day 7, when compared to both control and virgin LDPE, with a similar effect in the digestive gland of organisms exposed for 14 days to LDPE+adsorbed chemicals (Figures 2F, 3E). An increase in lipid peroxidation may result from inefficient oxidative stress reduction mechanisms in the processing of excess ROS. This may, in part, explain the inhibition of both CAT and GPx, due to an inability to respond to elevated ROS levels. In contrast to the results of this experiment, levels of oxidative damage were reduced in the gills of S. plana exposed to PS MP at similar concentrations, and showed no significant changes over time in digestive gland tissues (Ribeiro et al., 2017). No increase in LPO levels was reported in tissues of the common goby, P. microps, following exposure to both PE MP and pyrene contaminated PE MP, at a concentration of 18.4 and 184 μg L−1 (Oliveira et al., 2013).
Results from the PCA for BaP (Figure 5), taking into account the biomarkers analyzed, and the determined concentration of BaP in the tissues, reveal that most biomarkers are more positively related with the LDPE+BaP treatment than to virgin LDPE. Still, it appears that gills are more influenced by virgin LDPE than are digestive gland tissues. Similarly, in the PCA for PFOS (Figure 6), virgin LDPE is in the opposite side of LDPE+PFOS in the axis for PC1, with gills biomarkers more related to virgin LDPE and digestive gland more related to LDPE+PFOS. This may be a result of potential mechanical injury of gills that may also affect the analyzed biomarkers, but more importantly that digestive glands are apparently less affected by potential mechanical damage cause by microplastics alone but more influenced by the presence of the contaminants.
Conclusion
A tissue specific response in oxidative stress was observed due to LDPE MP exposure, with each respective LDPE+BaP and LDPE+PFOS exhibiting varied response per biomarker investigated. Oxidative damage was observed in all microplastics, with adsorbed BaP and PFOS, treatments in both digestive gland and gill tissues. The digestive gland seems less affected by mechanical damage caused by virgin LDPE exposure, with the MP-adsorbed BaP and PFOS exerting a negative influence over the assessed biomarkers in this tissue. It is clear that further research is needed into the ecotoxicological effects of MP in the marine environment with the potential for MP with adsorbed chemicals to desorb once ingested and their subsequent mode of action within organisms being of particular concern.
Author Contributions
SO: Performed the experiment and analyses, analyzed the data, and wrote the manuscript; NM and MB: Designed the experiment and contributed to the data analysis and to the writing of the manuscript; SA: Conducted biomarker analyses; TF: Contributed the running of the experiment, analyses, and to the writing of the manuscript; SK and BC: Prepared and characterized the contaminated MPs and contributed to the writing of the manuscript; CC: Performed the chemical analysis and contributed to the writing.
Funding
This work was developed under the EPHEMARE project (Ecotoxicological effects of microplastics in marine ecosystems), supported by national funding agencies in the framework of JPI Oceans (FCT JPIOCEANS/0005/2015; BELSPO; FWO; FORMAS; SwAM). NM and MB further acknowledge the support from FCT through the grant UID/MAR/00350/2013 attributed to CIMA, University of Algarve.
Conflict of Interest Statement
The authors declare that the research was conducted in the absence of any commercial or financial relationships that could be construed as a potential conflict of interest.
Acknowledgments
We would like to thank Dr Bavo De Witte for his skillful collaboration to the BaP analysis, and Daphné Deloof, for performing BaP extraction and quantification.
References
Ahmad, I., Mohmood, I., Mieiro, C., Coelho, J., Pacheco, M., Santos, M., et al. (2011). Lipid peroxidation vs. antioxidant modulation in the bivalve Scrobicularia plana in response to environmental mercury—Organ specificities and age effect. Aquat. Toxicol. 103, 150–158. doi: 10.1016/j.aquatox.2011.02.017
Almeida, C., Pereira, C., Gomes, T., Cardoso, C., Bebianno, M. J., and Cravo, A. (2013). Genotoxicity in two bivalve species from a coastal lagoon in the south of Portugal. Mar. Environ. Res. 89, 29–38. doi: 10.1016/j.marenvres.2013.04.008
Andrady, A. L., and Neal, M. A. (2009). Applications and societal benefits of plastics. Philos. Trans. R. Soc. B Biol. Sci. 364, 1977–1984. doi: 10.1098/rstb.2008.0304
Antunes, J., Frias, J., Micaelo, A., and Sobral, P. (2013). Resin pellets from beaches of the Portuguese coast and adsorbed persistent organic pollutants. Estuar. Coast. Shelf Sci. 130, 62–69. doi: 10.1016/j.ecss.2013.06.016
Avio, C. G., Gorbi, S., and Regoli, F. (2017). Plastics and microplastics in the oceans: from emerging pollutants to emerged threat. Mar. Environ. Res. 128, 2–11. doi: 10.1016/j.marenvres.2016.05.012
Avio, C. G., Gorbi, S., Milan, M., Benedetti, M., Fattorini, D., d'Errico, G., et al. (2015). Pollutants bioavailability and toxicological risk from microplastics to marine mussels. Environ. Pollut. 198, 211–222. doi: 10.1016/j.envpol.2014.12.021
Bakir, A., O'Connor, I. A., Rowland, S. J., Hendriks, A. J., and Thompson, R. C. (2016). Relative importance of microplastics as a pathway for the transfer of hydrophobic organic chemicals to marine life. Environ. Pollut. 219, 56–65. doi: 10.1016/j.envpol.2016.09.046
Bakir, A., Rowland, S. J., and Thompson, R. C. (2014). Transport of persistent organic pollutants by microplastics in estuarine conditions. Estuar. Coast. Shelf Sci. 140, 14–21. doi: 10.1016/j.ecss.2014.01.004
Bakir, A., Rowland, S. J., and Thompson, R. C. (2012). Competitive sorption of persistent organic pollutants onto microplastics in the marine environment. Mar. Pollut. Bull. 64, 2782–2789. doi: 10.1016/j.marpolbul.2012.09.010
Barnes, D. (2005). Remote islands reveal rapid rise of southern hemisphere Sea Debris. Sci. World J. 5, 915–921. doi: 10.1100/tsw.2005.120
Barnes, D. K., Galgani, F., Thompson, R. C., and Barlaz, M. (2009). Accumulation and fragmentation of plastic debris in global environments. Philos. Trans. R. Soc. B Biol. Sci. 364, 1985–1998. doi: 10.1098/rstb.2008.0205
Batel, A., Borchert, F., Reinwald, H., Erdinger, L., and Braunbeck, T. (2018). Microplastic accumulation patterns and transfer of benzo[a]pyrene to adult zebrafish (Danio rerio) gills and zebrafish embryos. Environ. Pollut. 235, 918–930. doi: 10.1016/j.envpol.2018.01.028
Besseling, E., Wegner, A., Foekema, E. M., van den Heuvel-Greve, M. J., and Koelmans, A. A. (2013). Effects of microplastic on fitness and PCB bioaccumulation by the lugworm Arenicola marina (L.). Environ. Sci. Technol. 47, 593–600. doi: 10.1021/es302763x
Bradford, M. (1976). A rapid and sensitive method for the quantitation of microgram quantities of protein utilizing the principle of protein-dye binding. Anal. Biochem. 72, 248–254. doi: 10.1016/0003-2697(76)90527-3
Browne, M. A., Niven, S. J., Galloway, T. S., Rowland, S. J., and Thompson, R. C. (2013). Microplastic moves pollutants and additives to worms, reducing functions linked to health and biodiversity. Curr. Biol. 23, 2388–2392. doi: 10.1016/j.cub.2013.10.012
Browne, M. A., Dissanayake, A., Galloway, T. S., Lowe, D. M., and Thompson, R. C. (2008). Ingested microscopic plastic translocates to the circulatory system of the mussel, Mytilus edulis(L.). Environ. Sci. Technol. 42, 5026–5031. doi: 10.1021/es800249a
Châtel, A., Bruneau, M., Lièvre, C., Goupil, A., and Mouneyrac, C. (2017). Spermatozoa: a relevant biological target for genotoxicity assessment of contaminants in the estuarine bivalve Scrobicularia plana. Mar. Pollut. Bull. 116, 488–490. doi: 10.1016/j.marpolbul.2016.12.062
Chua, E. M., Shimeta, J., Nugegoda, D., Morrison, P. D., and Clarke, B. O. (2014). Assimilation of polybrominated diphenyl ethers from microplastics by the marine amphipod, Allorchestes compressa. Environ. Sci. Technol. 48, 8127–8134. doi: 10.1021/es405717z
Cole, M., Lindeque, P., Halsband, C., and Galloway, T. (2011). Microplastics as contaminants in the marine environment: a review. Mar. Pollut. Bull. 62, 2588–2597. doi: 10.1016/j.marpolbul.2011.09.025
Corcoran, P. L., Norris, T., Ceccanese, T., Walzak, M. J., Helm, P. A., and Marvin, C. H. (2015). Hidden plastics of Lake Ontario, Canada and their potential preservation in the sediment record. Environ. Pollut. 204, 17–25. doi: 10.1016/j.envpol.2015.04.009
Cózar, A., Echevarría, F., González-Gordillo, J. I., Irigoien, X., Úbeda, B., and Hernández-León, S. (2014). Plastic debris in the open ocean. Proc. Natl. Acad. Sci. U.S.A. 111, 10239–10244. doi: 10.1073/pnas.1314705111
de Vos, M. G., Huijbregts, M. A., van den Heuvel-Greve, M. J., Vethaak, A. D., Van de Vijver, K. I., Leonards, P. E., et al. (2008). Accumulation of perfluorooctane sulfonate (PFOS) in the food chain of the Western Scheldt estuary: comparing field measurements with kinetic modeling. Chemosphere 70, 1766–1773. doi: 10.1016/j.chemosphere.2007.08.038
De Witte, B. L., Devriese, L., Bekaert, K., Hoffman, S., Vandermeersch, G. Cooreman, K., et al. (2014). Quality assessment of the blue mussel (Mytilus edulis): comparison between commercial and wild types. Mar. Pollut. Bull. 85, 146–155. doi: 10.1016/j.marpolbul.2014.06.006.
Ellman, G. L., Courtney, K. D., Andres, V., and Featherstone, R. (1961). A new and rapid colorimetric determination of acetylcholinesterase activity. Biochem. Pharmacol. 7, 88–95. doi: 10.1016/0006-2952(61)90145-9
Engler, R. (2012). The complex interaction between marine debris and toxic chemicals in the Ocean. Environ. Sci. Technol. 46, 12302–12315. doi: 10.1021/es3027105
Erdelmeier, I., Gérard-Monnier, D., Yadan, J., and Chaudière, J. (1998). Reactions of N -Methyl-2-phenylindole with Malondialdehyde and 4-Hydroxyalkenals. Mechanistic aspects of the colorimetric assay of lipid peroxidation. Chem. Res. Toxicol. 11, 1184–1194. doi: 10.1021/tx970180z
Eriksen, M., Lebreton, L. C., Carson, H. S., Thiel, M., Moore, C. J., Borerro, J. C., et al. (2014). Plastic pollution in the world's oceans: more than 5 trillion plastic pieces weighing over 250,000 tons afloat at sea. PLoS ONE 9:e111913. doi: 10.1371/journal.pone.0111913
Eriksson, U., Roos, A., Lind, K., Hope, A., and Ekblad, A. (2016). Comparison of PFASs contamination in the freshwater and terrestrial environments by analysis of eggs from osprey (Pandion haliaetus), tawny owl (Strix aluco), and common kestrel (Falco tinnunculus). Environ. Res. 149, 40–47. doi: 10.1016/j.envres.2016.04.038
Farrell, P., and Nelson, K. (2013). Trophic level transfer of microplastic: Mytilus edulis (L.) to Carcinus maenas (L.). Environ. Pollut. 177, 1–3. doi: 10.1016/j.envpol.2013.01.046
Fischer, V., Elsner, N., Brenke, N., Schwabe, E., and Brandt, A. (2015). Plastic pollution of the Kuril–Kamchatka Trench area (NW pacific). Deep Sea Res. Part II Top. Stud. Oceanogr. 111, 399–405. doi: 10.1016/j.dsr2.2014.08.012
Frias, J. P, Otero, V., and Sobral, P. (2014). Evidence of microplastics in samples of zooplankton from Portuguese coastal waters. Mar. Environ. Res. 95, 89–95. doi: 10.1016/j.marenvres.2014.01.001
Galgani, F., Claro, F., Depledge, M., and Fossi, C. (2014). Monitoring the impact of litter in large vertebrates in the Mediterranean Sea within the European Marine Strategy framework directive: constraints, specificities and recommendations. Mar. Environ. Res. 100, 3–9. doi: 10.1016/j.marenvres.2014.02.003
Germanov, E. S., Marshall, A. D., Bejder, L., Fossi, M. C., and Loneragan, N. R. (2018). Microplastics: no small problem for filter-feeding Megafauna. Trends Ecol. Evol. 33, 227–232. doi: 10.1016/j.tree.2018.01.005
Giesy, J. P., and Kannan, K. (2001). Global distribution of perfluorooctane sulfonate in wildlife. Environ. Sci. Technol. 35, 1339–1342. doi: 10.1021/es001834k
Greenwald, R. (1987). Handbook of methods for oxygen radical research. Free Radic. Biol. Med. 3:161. doi: 10.1016/S0891-5849(87)80012-6
Habig, W., and Jakoby, W. (1981). [51] Assays for differentiation of glutathione S-Transferases. Meth. Enzymol. 77, 398–405. doi: 10.1016/S0076-6879(81)77053-8
Hartmann, N. B., Rist, S., Bodin, J., Jensen, L. H., Schmidt, S. N., Mayer, P., et al. (2017). Microplastics as vectors for environmental contaminants: exploring sorption, desorption, and transfer to biota. Integr. Environ. Assess. Manag. 13, 488–493. doi: 10.1002/ieam.1904
Hidalgo-Ruz, V., Gutow, L., Thompson, R. C., and Thiel, M. (2012). Microplastics in the marine environment: a review of the methods used for identification and quantification. Environ. Sci. Technol. 46, 3060–3075. doi: 10.1021/es2031505
Hoarau, P., Garello, G., Gnassia-Barelli, M., Romeo, M., and Girard, J. (2002). Purification and partial characterization of seven glutathione S -transferase isoforms from the clam Ruditapes decussatus. Eur. J. Biochem. 269, 4359–4366. doi: 10.1046/j.1432-1033.2002.03141.x
Hughes, R. (1969). A study of feeding in Scrobicularia plana. J. Mar. Biol. Assoc. U.K. 49:805. doi: 10.1017/S0025315400037309
IARC (2011). Monographs on the Evaluation of Carcinogenic Risks to Humans, a Review of Human Carcinogens. Lyon: International Agency for Research on Cancer.
Jo, P. G., Choi, Y. K., and Choi, C. Y. (2008). Cloning and mRNA expression of antioxidant enzymes in the Pacific oyster, Crassostrea gigas in response to cadmium exposure. Comp. Biochem. Physiol. Part 147, 460–469. doi: 10.1016/j.cbpc.2008.02.001
Kannan, K., Koistinen, J., Beckmen, K., Evans, T., Gorzelany, J. F., Hansen, K. J., et al. (2001). Accumulation of perfluorooctane sulfonate in marine mammals. Environ. Sci. Technol. 35, 1593–1598. doi: 10.1021/es001873w
Khessiba, A., Hoarau, P., Gnassia-Barelli, M., Aissa, P., and Roméo, M. (2001). Biochemical response of the mussel Mytilus galloprovincialis from Bizerta (Tunisia) to chemical pollutant exposure. Arch. Environ. Contam. Toxicol. 40, 222–229. doi: 10.1007/s002440010166
Kolandhasamy, P., Su, L., Li, J., Qu, X., Jabeen, K., and Shi, H. (2018). Adherence of microplastics to soft tissue of mussels: a novel way to uptake microplastics beyond ingestion. Sci. Tot. Environ. 610–611, 635–640. doi: 10.1016/j.scitotenv.2017.08.053
Langston, W., Burt, G., and Chesman, B. (2007). Feminisation of male clams Scrobicularia plana from estuaries in Southwest UK and its induction by endocrine-disrupting chemicals. Mar. Ecol. Prog. Ser. 333, 173–184. doi: 10.3354/meps333173
Langston, W., Burt, G., Chesman, B., and Vane, C. (2005). Partitioning, bioavailability and effects of oestrogens and xeno-oestrogens in the aquatic environment. J. Mar. Biol. Assoc. U.K. 85, 1–31. doi: 10.1017/S0025315405010787h
Larsson, M., Hagberg, J., Rotander, A., Van Bavel, B., and and, Engwall, M. (2013). Chemical and bioanalytical characterisation of PAHs in risk assessment of remediated PAH-contaminated soils. Environ. Sci. Pollut. Res. 20, 8511–8520. doi: 10.1007/s11356-013-1787-6
Lawrence, R. A., and Burk, R. F. (1978). Species, tissue and subcellular distribution of non se-dependent glutathione peroxidase activity. J. Nutr. 108, 211–215.
Lee, H., Shim, W. J., and Kwon, J. H. (2014). Sorption capacity of plastic debris for hydrophobic organic chemicals. Sci. Tot. Environ. 470–471, 1545–1552. doi: 10.1016/j.scitotenv.2013.08.023
Lee, K. W., Shim, W. J., Kwon, O. Y., and Kang, J. H. (2013). Size-dependent effects of micro polystyrene particles in the marine copepod Tigriopus japonicus. Environ. Sci. Technol. 47, 11278–11283. doi: 10.1021/es401932b
Liu, T., Pan, L., Jin, Q., and Cai, Y. (2015). Differential gene expression analysis of benzo(a)pyrene toxicity in the clam, Ruditapes philippinarum. Ecotoxicol. Environ. Saf. 115, 126–136. doi: 10.1016/j.ecoenv.2015.02.007
Lobelle, D., and Cunliffe, M. (2011). Early microbial biofilm formation on marine plastic debris. Mar. Pollut. Bull. 62, 197–200. doi: 10.1016/j.marpolbul.2010.10.013
Lourenço, P. M., Serra-Gonçalves, C., Ferreira, J. L., Catry, T., and Granadeiro, J. P. (2017). Plastic and other microfibers in sediments, macroinvertebrates and shorebirds from three intertidal wetlands of southern Europe and west Africa. Environ. Pollut. 231, 123–133. doi: 10.1016/j.envpol.2017.07.103
Lusher, A. (2015). “Microplastics in the marine environment: distribution, interactions and effects,” in Marine Anthropogenic Litter, eds M. Bergmann, L. Gutow, and M. Klages (Berlin: Springer), 245–308.
Maranho, L. A., Baena-Nogueras, R. M., Lara-Martín, P. A., DelValls, T. A., and Martín-Díaz, M. L. (2014). Bioavailability, oxidative stress, neurotoxicity and genotoxicity of pharmaceuticals bound to marine sediments. The use of the polychaete Hediste diversicolor as bioindicator species. Environ. Res. 134, 353–365. doi: 10.1016/j.envres.2014.08.014
Mato, Y., Isobe, T., Takada, H., Kanehiro, H., Ohtake, C., and Kaminuma, T. (2001). Plastic resin pellets as a transport medium for toxic chemicals in the marine environment. Environ. Sci. Technol. 35, 318–324. doi: 10.1021/es0010498
McCord, J. M., and Fridovich, I. (1969). Superoxide dismutase an enzymic function for erythrocuprein (Hemocuprein). Biol. Chem. 244, 6049–6055.
McDermid, K. J., and McMullen, T. L. (2004). Quantitative analysis of small-plastic debris on beaches in the Hawaiian archipelago. Mar. Pollut. Bull. 48, 790–794 doi: 10.1016/j.marpolbul.2003.10.017
Moore, C. (2008). Synthetic polymers in the marine environment: a rapidly increasing, long-term threat. Environ. Res. 108, 131–139. doi: 10.1016/j.envres.2008.07.025
Mouneyrac, C., Linot, S., Amiard, J. C., Amiard-Triquet, C., Métais, I., Durou, C., et al. (2008). Biological indices, energy reserves, steroid hormones and sexual maturity in the infaunal bivalve Scrobicularia plana from three sites differing by their level of contamination. Gen. Comp. Endocrinol. 157, 133–141. doi: 10.1016/j.ygcen.2008.04.010
Obbard, R., Sadri, S., Wong, Y., Khitun, A., Baker, I., and Thompson, R. (2014). Global warming releases microplastic legacy frozen in Arctic Sea ice. Earth's Future 2, 315–320. doi: 10.1002/2014EF000240
Ogata, Y., Takada, H., Mizukawa, K., Hirai, H., Iwasa, S., Endo, S., et al. (2009). International pellet watch: global monitoring of persistent organic pollutants (POPs) in coastal waters. 1. Initial phase data on PCBs, DDTs, and HCHs. Mar. Pollut. Bull. 58, 1437–1446. doi: 10.1016/j.marpolbul.2009.06.014
Oliveira, M., Maria, V. L., Ahmad, I., Serafim, A., Bebianno, M. J., Pacheco, M., et al. (2009). Contamination assessment of a coastal lagoon (Ria de Aveiro, Portugal) using defence and damage biochemical indicators in gill of Liza aurata – An integrated biomarker approach. Environ. Pollut. 157, 959–967. doi: 10.1016/j.envpol.2008.10.019
Oliveira, M., Ribeiro, A., Hylland, K., and Guilhermino, L. (2013). Single and combined effects of microplastics and pyrene on juveniles (0+ group) of the common goby Pomatoschistus microps (Teleostei, Gobiidae). Ecol. Indic. 34, 641–647. doi: 10.1016/j.ecolind.2013.06.019
Paul, A. G., Jones, K.C., and Sweetman, A. J. (2009). A first global production, emission, and environmental inventory for perfluorooctane sulfonate. Environ. Sci. Technol. 43, 386–392. doi: 10.1021/es802216n
Paul-Pont, I., Lacroix, C., González Fernández, C., Hégaret, H., Lambert, C., Le Goïc, N., et al. (2016). Exposure of marine mussels Mytilus spp. to polystyrene microplastics: toxicity and influence on fluoranthene bioaccumulation. Environ. Pollut. 216, 724–737. doi: 10.1016/j.envpol.2016.06.039
Petridis, P., Jha, A. N., and Langston, W. J. (2009). Measurements of the genotoxic potential of (xeno-) oestrogens in the bivalve mollusc Scrobicularia plana, using the Comet assay. Aquat. Toxicol. 94, 8–15. doi: 10.1016/j.aquatox.2009.05.010
Rainieri, S., Conlledo, N., Larsen, B. K., Granby, K., and Barranco, A. (2018). Combined effects of microplastics and chemical contaminants on the organ toxicity of zebrafish (Danio rerio). Environ. Res. 162, 135–143. doi: 10.1016/j.envres.2017.12.019
R Core Team (2016). R: A Language and Environment for Statistical Computing. Vienna: R Foundation for Statistical Computing.
Regoli, F., and Giuliani, M. (2014). Oxidative pathways of chemical toxicity and oxidative stress biomarkers in marine organisms. Mar. Environ. Res. 93, 106–117. doi: 10.1016/j.marenvres.2013.07.006
Ribeiro, F., Garcia, A. R., Pereira, B. P., Fonseca, M., Mestre, N. C., Fonseca, T. G., et al. (2017). Microplastics effects in Scrobicularia plana. Mar. Pollut. Bull. 122, 379–391. doi: 10.1016/j.marpolbul.2017.06.078
Rios, L. M., Moore, C., and Jones, P. R. (2007). Persistent organic pollutants carried by synthetic polymers in the ocean environment. Mar. Pollut. Bull. 54, 1230–1237. doi: 10.1016/j.marpolbul.2007.03.022
Rochman, C. M., Kurobe, T., Flores, I., and Teh, S. J. (2014). Early warning signs of endocrine disruption in adult fish from the ingestion of polyethylene with and without sorbed chemical pollutants from the marine environment. Sci. Tot. Environ. 493, 656–661. doi: 10.1016/j.scitotenv.2014.06.051
Rochman, C. M., Tahir, A., Williams, S. L., Baxa, D. V., Lam, R., Miller, J. T., et al. (2015). Anthropogenic debris in seafood: plastic debris and fibers from textiles in fish and bivalves sold for human consumption. Sci. Rep. 5:14340. doi: 10.1038/srep14340
Rodríguez-Rúa, A., Prado, M., Romero, Z., and Bruzón, M. (2003). The gametogenic cycle of Scrobicularia plana (da Costa, 1778) (Mollusc: Bivalve) in Guadalquivir estuary (Cádiz, SW Spain). Aquaculture 217, 157–166. doi: 10.1016/S0044-8486(02)00052-2
Santillo, D., Miller, K., and Johnston, P. (2017). Microplastics as contaminants in commercially important seafood species. Integr. Environ. Assess. Manag. 13, 516–521. doi: 10.1002/ieam.1909
Setälä, O., Norkko, J., and Lehtiniemi, M. (2016). Feeding type affects microplastic ingestion in a coastal invertebrate community. Mar. Pollut. Bull. 102, 95–101. doi: 10.1016/j.marpolbul.2015.11.053
Singh, N. P., McCoy, M. T., Tice, R. R., and Schneider, E. L. (1988). A simple technique for quantitation of low levels of DNA damage in individual cells. Exp. Cell Res. 175, 184–191. doi: 10.1016/0014-4827(88)90265-0
Solé, M., Kopecka-Pilarczyk, J., and Blasco, J. (2009). Pollution biomarkers in two estuarine invertebrates, Nereis diversicolor and Scrobicularia plana, from a Marsh ecosystem in SW Spain. Environ. Int. 35, 523–531. doi: 10.1016/j.envint.2008.09.013
Teuten, E. L., Saquing, J. M., Knappe, D. R., Barlaz, M. A., Jonsson, S., Björn, A., et al. (2009). Transport and release of chemicals from plastics to the environment and to wildlife. Philos. Trans. R. Soc. B Biol. Sci. 364, 2027–2045. doi: 10.1098/rstb.2008.0284
Thompson, R. C, Olsen, Y., Mitchell, R. P., Davis, A., Rowland, S. J., John, A. W., et al. (2004). Lost at sea: where is all the plastic? Science 304, 838–838. doi: 10.1126/science.1094559
von Moos, N., Burkhardt-Holm, P., and Köhler, A. (2012). Uptake and effects of microplastics on cells and tissue of the blue mussel Mytilus edulis L. after an experimental exposure. Environ. Sci. Technol. 46, 11327–11335. doi: 10.1021/es302332w
Walne, P. (1976). Experiments on the culture in the sea of the butterfish Venerupis decussata L. Aquaculture 8, 371–381. doi: 10.1016/0044-8486(76)90119-8
Wu, C., Zhang, K., Huang, X., and Liu, J. (2016). Sorption of pharmaceuticals and personal care products to polyethylene debris. Environ. Sci. Pollut. Res. 23, 8819–8826. doi: 10.1007/s11356-016-6121-7
Yamashita, N., Kannan, K., Taniyasu, S., Horii, Y., Petrick, G., and Gamo, T. (2005). A global survey of perfluorinated acids in oceans. Mar. Pollut. Bull. 51, 658–668. doi: 10.1016/j.marpolbul.2005.04.026
Zalasiewicz, J., Waters, C., Ivar do Sul, J., Corcoran, P., Barnosky, A., Cearreta, A., et al. (2016). The geological cycle of plastics and their use as a stratigraphic indicator of the Anthropocene. Anthropocene 13, 4–17. doi: 10.1016/j.ancene.2016.01.002
Zhang, X., Zheng, M., Wang, L., Lou, Y., Shi, L., and Jiang, S. (2018). Sorption of three synthetic musks by microplastics. Mar. Pollut. Bull. 126, 606–609. doi: 10.1016/j.marpolbul.2017.09.025
Keywords: polyethylene, benzo[a]pyrene, perfluorooctane sulfonic acid, DNA damage, oxidative stress, neurotoxicity, oxidative damage
Citation: O'Donovan S, Mestre NC, Abel S, Fonseca TG, Carteny CC, Cormier B, Keiter SH and Bebianno MJ (2018) Ecotoxicological Effects of Chemical Contaminants Adsorbed to Microplastics in the Clam Scrobicularia plana. Front. Mar. Sci. 5:143. doi: 10.3389/fmars.2018.00143
Received: 15 January 2018; Accepted: 10 April 2018;
Published: 26 April 2018.
Edited by:
Ricardo Beiras, University of Vigo, SpainReviewed by:
Leticia Vidal-Liñán, Instituto Español de Oceanografía (IEO), SpainJuan Campillo, Instituto Español de Oceanografía (IEO), Spain
Copyright © 2018 O'Donovan, Mestre, Abel, Fonseca, Carteny, Cormier, Keiter and Bebianno. This is an open-access article distributed under the terms of the Creative Commons Attribution License (CC BY). The use, distribution or reproduction in other forums is permitted, provided the original author(s) and the copyright owner are credited and that the original publication in this journal is cited, in accordance with accepted academic practice. No use, distribution or reproduction is permitted which does not comply with these terms.
*Correspondence: Maria J. Bebianno, bWJlYmlhbkB1YWxnLnB0