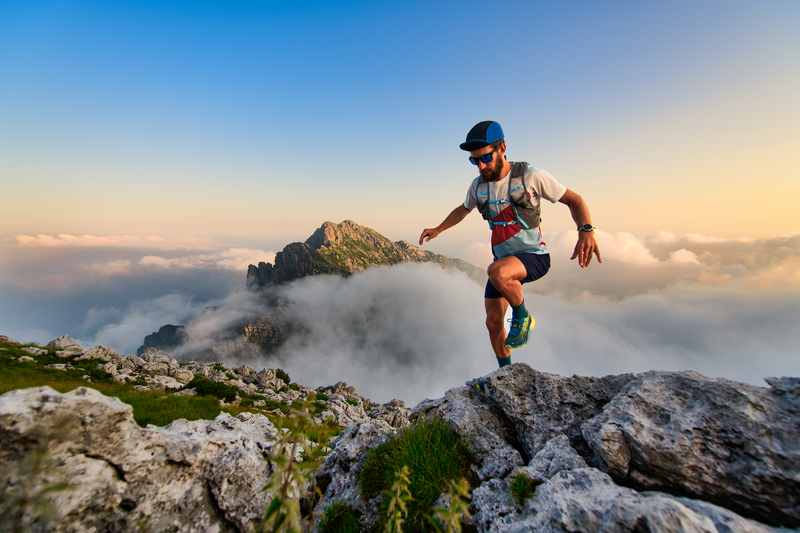
94% of researchers rate our articles as excellent or good
Learn more about the work of our research integrity team to safeguard the quality of each article we publish.
Find out more
ORIGINAL RESEARCH article
Front. Mar. Sci. , 12 April 2018
Sec. Deep-Sea Environments and Ecology
Volume 5 - 2018 | https://doi.org/10.3389/fmars.2018.00129
There is the potential for climate change to interact with pollution in all of the Earth's oceans. In the fjords of Norway, mine tailings are released into fjords generating suspended sediment plumes that impact deep-sea ecosystems. These same deep-sea ecosystems are expected to undergo periodic warming as climate change increases the frequency of down-welling events in fjords. It remains unknown how a polluted deep-sea ecosystem would respond to down-welling because multiple stressors will often interact in unpredictable ways. Here, we exposed two deep-sea foundation species; the gorgonian coral Primnoa resedaeformis and the demosponge Geodia atlantica to suspended sediment (10 mg L−1) and acute warming (+5°C) in a factorial mesocosm experiment for 40 days. Physiology (respiration, nutrient flux) and cellular responses (lysosomal cell stability) were measured for both the coral and sponge. Exposure to elevated suspended sediment reduced metabolism, supressed silicate uptake and induced cellular instability of the sponge G. atlantica. However, combining sediment with warming caused G. atlantica to respire and excrete nitrogen at a greater rate. For the coral P. resedaeformis, suspended sediments reduced O:N ratios after 40 days, however, warming had a greater effect on P. resedaeformis physiology compared to sediment. Warming increased respiration, nitrogen excretion, and cellular instability which resulted in lower O:N ratios. We argue that suspended sediment and warming can act alone and also interact to cause significant harm to deep-sea biota, however responses are likely to be species-specific. Warming and pollution could interact in the deep-sea to cause mortality to the coral P. resedaeformis and to a lesser extent, the sponge G. atlantica. As foundation species, reducing the abundance of deep sea corals and sponges would likely impact the ecosystems they support.
Ninety five percent of the Earth's ocean floor is below more than 200 m of water (Sweetman et al., 2017). Known as the “deep-sea,” these regions remain relatively unstudied due in large part to the high cost and logistical difficulty of gaining access. The past paucity of research and limited knowledge of deep-sea habitats have not prevented industries from extracting resources and disposing of waste in the deep-sea, for example the release of mining wastes into fjords, oil and gas drilling, and extensive bottom trawling (Fosså et al., 2002; Althaus et al., 2009; Purser and Thomsen, 2012). In addition to the ecological impacts caused by such activities, some deep-sea ecosystems will soon experience rising sea water temperatures (Collins et al., 2013; Sweetman et al., 2017). With advancing technology, researchers have an increasing ability to study the potential for interacting effects of multiple anthropogenic stressors on deep sea organisms, and their ecosystem functioning.
Many deep-sea ecosystems rely on the services performed by a few “foundation” species (Dayton, 1972; Bruno and Bertness, 2001; Levin and Dayton, 2009). These foundation species are abundant and provide services such as habitat and nutrient cycling that are fundamental to the structure of the ecosystem (Ellison et al., 2005). In some deep-sea ecoystems, demosponges and gorgonian corals are foundation species that can grow into large tree shaped structures arranged like forests on the seafloor (Krieger and Wing, 2002; Buhl-Mortensen et al., 2010; Hogg et al., 2010; Kutti et al., 2013). Up to 114 invertebrate species have been found associated with gorgonian corals (Buhl-Mortensen and Mortensen, 2004, 2005) and over 240 species are associated with sponge forests, including commercial fish species and other megafauna (Klitgaard, 1995; Metaxas and Davis, 2005). Such numbers are almost certainly underestimates of the full biotic diversity associated with these key species (Buhl-Mortensen and Mortensen, 2004, 2005). In addition to forming habitat, sponges, corals and their associated fauna play major roles in nitrogen cycling and carbon fixation in the deep-sea (Jiménez and Ribes, 2007; Danovaro et al., 2008, 2014; Hoffmann et al., 2009; Cathalot et al., 2015).
The Norwegian fjords are hot spots for deep-sea biota (Mortensen et al., 1995; Kutti et al., 2013, 2014), but are also convenient places for the disposal of inert mine waste; a practice that has been occurring for decades (Olsgard and Hasle, 1993; Kvassnes and Iversen, 2013). The mine waste released into fjords smothers benthic communities in the immediate vicinity, but also causes high levels of suspended sediment in the water column for kilometers away from the discharge site (Allan, 1995; Bakke et al., 2013; Brooks et al., 2015). This type of suspended sediment is known to impact benthic soft sediment habitats (Mevenkamp et al., 2017), however, knowledge of its effects on other deep-sea environments remains limited (Bakke et al., 2013). Chronic exposure to suspended sediment from other sources such as terrestrial run-off, or bottom trawling is known to affect the distribution of sponges (Bannister et al., 2011) and elevate their metabolic rates (Bannister et al., 2012; Tjensvoll et al., 2013; Kutti et al., 2015; Fang et al., in press). Furthermore, exposure to elevated sediments will induce cellular damage in deep-sea sponges and corals (Roberts and Cairns, 2014; Edge et al., 2016).
In addition to the waste from extractive activities, some deep-sea ecosystems will experience rising sea water temperatures in the near future (Collins et al., 2013) which will place extra physiological demands on ectothermic organisms (Pörtner and Farrell, 2008). Down-welling events cause warm surface water to be drawn into the deeper regions of the ocean, and raise the ambient temperature 4–5°C above average. Such events are likely to become more common and intense in the future oceans, especially near the coast (Collins et al., 2013). Such down-welling events were recorded in 2006 and 2008 at Tisler reef in a southern Norwegian fjord (Guihen et al., 2012), a location that was known to support large sponge and coral assemblages (Wisshak and Rüggeberg, 2006). During this down-welling event the ambient temperature was elevated by 4–5°C (to a maximum water temperature of 12°C) for 2 weeks and a concurrent mass mortality of the sponge Geodia barretti was observed (Guihen et al., 2012). This mortality may not be a result of the elevated temperature alone, given that acute (2 weeks) temperature elevations to 12°C are expected to be within the tolerance of Geodia species (Strand et al., 2017). However, multiple concurrent stressors were not investigated by Strand et al. (2017) and it is possible that elevated temperature has interacted with other environmental or biological stressors to cause the observed sponge mortality. When two or more environmental stressors combine, they will often interact in non-linear ways to cause unpredictable effects on ecosystems and organisms (Folt et al., 1999; Crain et al., 2008; Sokolova, 2013).
In the deep-sea, suspended sediment from mine waste can travel for kilometers away from the discharge site (Bakke et al., 2013), raising the likelihood of mine waste being caught in a down-welling cycle. A deep-sea ecosystem subjected to suspended sediment could experience a down-welling event that triggers an interaction among stressors. The demosponge Geodia atlantica (Stephens, 1915) and the coral Primnoa resedaeformis (Gunnerus, 1763) are foundation species distributed throughout the northern Atlantic Ocean, and are prevalent throughout Norwegian fjords generally at depths >200 m (Klitgaard and Tendal, 2004; Buhl-Mortensen et al., 2015) where they create habitat and recycle nutrients that are essential for associated biota (Metaxas and Davis, 2005; Jiménez and Ribes, 2007; Danovaro et al., 2008, 2014; Hoffmann et al., 2009; Buhl-Mortensen et al., 2010; Cathalot et al., 2015). In this study, we aimed to test how deep-sea foundation species like octocorals and demosponges would respond if they were to experience a down-welling event in an area of elevated suspended sediment. We exposed the sponge G. atlantica and the octocoral P. resedaeformis to suspended sediment and elevated temperature in a manipulative mesocosm experiment and measured the physiology (respiration, nutrient flux) and cellular responses (lysosomal cell stability) for both the coral and sponge. It was predicted that both organisms would experience cellular and physiological disruptions from each stressor and their interaction.
To test the single and combined effects of suspended sediment and warming on the sponge and coral, organisms were exposed together in mesocosms to orthogonal combinations of ambient and elevated temperature and sediment (n = 5 mesocosms for each combination of treatments). After 26, 33, and 40 days in experimental treatments, physiological (respiration, nutrient flux) and cellular (lysosomal stability) responses of the sponge and coral were measured. This study was carried out in accordance with the requirements of Norwegian law regarding the ethical treatment of animals.
To obtain experimental sponges, explants of G. atlantica were cultivated from 10 adult donor sponges. Donor G. atlantica were collected from a depth of 190–200 m from Nakken Reef in Langenuen Fjord, Norway in January 2015 (60° 02′ 32.7″N, 5° 19′ 27.0″E) using a remotely operated vehicle operated from the research vessel Håkon Mosby (Figure 1). Cultivation techniques for G. atlantica were implemented from standard published methodologies (Hoffmann et al., 2003; Kutti et al., 2015; Strand et al., 2017). Briefly, whole adult G. atlantica were cut into 4 cm3 pieces where they were left to heal and grow in commercial mussel lanterns at a depth of 200 m in an adjacent fjord (60° 2′ 17″ N, 05° 27′ 15″ E) for 8 months (see Kutti et al., 2015). Following 8 months healing time, the sponge explants were collected in September 2015 and transported to the Institute of Marine Research, Austevoll Research Station (Hordaland, Norway). Each explant was given an identifying number and placed into 600 L flow-through aquaria at the Austevoll Research Station for a period of 2 months to acclimate to conditions prior to experimental exposure. The flowthrough aquaria used sand filtered seawater drawn from a depth of 160 m from the adjacent Langenuen Fjord (60° 5′ 16.91″N, 5° 16′ 9.84″E), where it then flowed through tanks at a rate of 2,500 L h−1 tank−1. For all experiments food was not added to the tanks. Sand filtered seawater at a high flow rate was relied upon to supply food, previous studies using these facilities have shown that the sand filtered seawater contains sufficient particles < 10 μm for a food supply (e.g., Kutti et al., 2015; Strand et al., 2017).
Figure 1. Sponge and coral forest at Nakken reef, Norway. Circle (A) indicates a P. resdeaformis colony, and circle (B) indicates a G. atlantica holobiont. The image was captured at a depth of 200 m using a ROV operated from the ship Håkon Mosby.
To obtain experimental corals, 15 large (>1 m from base to tips of distal branches) colonies of P. resedaeformis were collected from depth of 200 m at Nakken Reef in Langenuen Fjord, Norway, in September 2015 (60° 02′ 32.7″N, 5° 19′ 27.0″E) using a remotely operated vehicle operated from the research vessel Håkon Mosby (Figure 1). Once collected, colonies were transported in seawater to the laboratory where they were fragmented from the distal branches into 150 mm long pieces that each contained >50 polyps. The fragments were then placed into weighted bases, given an identifying number, and kept in aforementioned flowthrough aquaria with G. atlantica explants for a period of 2 months to acclimate to conditions prior to experimental exposure.
Crushed granite rock (i.e., simulated inert mine tailings) was collected from a producer of artificial sand near the city of Bergen (Fana Stein A/S). Collected sediment was then sieved using stainless steel laboratory sieves to obtain the fine fraction of sediment (<63 μm). This sediment particle size was used because the finer fraction is likely to remain suspended in the water column (McCave, 1975), and therefore, would more accurately represent the suspended mine tailings in fjords. The fine fraction of sediment was then combusted at 450°C for 4 h to eliminate any organic content of the sediment and then stored in airtight containers at room temperature until use.
All experiments were conducted at the Austevoll Research Station (Hordaland, Norway) during November–December 2015. Fragments of P. resedaeformis and explants of G. atlantica were kept together in 40 L flow through aquarium tanks, of which there were 20 in total (mesocosms) throughout the duration of the experiment. Following acclimation, G. altanicta explants were each blotted dry on paper towel, and weighed on an electronic balance (± 0.001 g; Supplementary Material); P. resedaeformis was not weighed in order to minimize disturbance. Fragments of P. resedaeformis and G. altanicta explants were then randomly distributed among mesocosms, each containing four fragments of coral, and four explants of G. altanicta. Seawater in the mesocosm tanks was the same as used above for acclimation, at a flow rate of 50 L h−1 tank−1.
The sponge G. atlantica and the coral P. resedaeformis were exposed together in five replicate (n = 5) mesocosms per each of four nominal experimental treatments; control (sand filtered seawater; 7°C), suspended sediment (10 mg L−1 crushed rock; 7°C), warming (sand filtered seawater; 13°C), and a combined sediment and warming treatment (referred to as sediment + warming; 10 mg L−1 crushed rock; 13°C). To cause an acute temperature rise (simulated down-welling), the temperature was not increased in the elevated temperature treatments until the 26th day of the experiment. Of the total 20 mesocosms, 10 were held at control conditions (sand filtered seawater; 7°C) and 10 at elevated suspended sediment (10 mg L−1 crushed rock; 7°C) for 26 days. After 26 days, 5 control mesocosms and 5 elevated suspended sediment mesocosms were warmed by incrementally increasing the temperature over 2 days to the desired experimental temperature (sand filtered seawater; 13°C). They then remained at 13°C until the end of the experiment (a further 14 days).
Ambient temperatures in the control, and suspended sediment tanks were unaltered from the external environment and remained at 7 ± 1°C. Warming treatments were maintained by two heat exchangers warming the incoming seawater to 13 ± 1°C. The two heat exchanges were both used to heat each elevated temperature treatment (i.e., one heater was used to heat some replicates in the warming treatment and some in the sediment + warming treatment), this was done to prevent one heater heating all replicates in one treatment. Individuals were sampled at three time points throughout the experiment; 26, 33, and 40 days after commencement of exposure. Individuals in the two warming treatments were exposed to elevated temperature only after 26 days since the beginning of the experiment, therefore, at 26 days, no measurements were taken for organisms in warming treatments.
Suspended sediment during the exposure period was manipulated using the model of Anthony (1999). Briefly; two 80 L conical header tanks were used to suspend the fine sediment and create a stock solution prior to dosage to replicate tanks. Header tanks were filled with ambient sand filtered seawater, and then a known amount of pre-ashed crushed rock (<63 μm) was mixed in to create a stock solution. Recirculating pumps kept the crushed rock resuspended in the header tanks. Sediment stock solution was delivered in a cycle of 12 h in every 24 h to randomly assigned replicate tanks. As with the heaters, each header tank supplied suspended sediment to both the sediment and the combined sediment + warming treatment to avoid one treatment receiving sediment from one header tank. Tidal, current and weather patterns are suggested to influence the distribution of sediment from mine waste around release sites (Kutti et al., 2015), and therefore we used a cyclic exposure of organisms to suspended sediment. Sediment was delivered into mesocosms via peristatic dosage pumps [IWAKI (EW/Y)] pumping a predetermined volume of stock solution at the required rate to give a constant nominal concentration of 10 mg L−1 in each replicate suspended sediment treatment tank. Sediment concentrations in each replicate tank were determined daily during the time that sediment exposure was occurring. This was done by measuring the absorbance of a random sample from each tank using UV spectroscopy (Shimadzu UV 160) at 660 nm and comparing the absorbance to standards made from a dilution series of stock sediment suspension at a known concentration (Tompkins-MacDonald and Leys, 2008). Mean (± s.e.m., n = 5) measured sediment concentrations in the sediment and the combined sediment + warming treatments over the entire experimental period were 11.33 ± 0.74 mg L−1, and 11.22 ± 0.75 mg L−1 respectively.
The oxygen and nutrient fluxes of P. resedaeformis and G. atlantica were measured using closed incubation chambers on the sampling days 26, 33, and 40, after the 12 h suspended sediment treatments cycles. At each time point, one individual explant of sponge or branch of coral was randomly selected from each replicate mesocosm for physiological measurements. A 500 mL airtight chamber was placed over the top of each selected organism, capturing seawater to fill the chamber, and forming a seal against the bottom of the tank. This was done without disturbing the organism (for further details on the respiration chambers see Fang et al., in press). Each chamber was fitted with a magnetic stirrer to generate water movement. Oxygen probes (PreSens needle probe NTH-PST1, AS1 Ltd., Regensburg, Germany) were inserted into the chambers and oxygen concentrations were measured throughout the incubation period. Probes were calibrated using a two-point calibration (0 and 100%) and all measurements were done at the experimental temperature in the replicate tank (7°C, or the warming treatment temperature of 13°C). Water samples for nutrient measurements were taken at the beginning and end of incubations using pre-combusted (400°C, 4 h) glass pipettes, placed into new polyethylene sample jars, and preserved with chloroform. The exact incubation time of approximately 3 h for sponges and 12 h for corals was recorded (O2 concentrations were never depleted below 70%), and was used to calculate rates of oxygen uptake and nutrient uptake and release. These incubation times were observed from pilot experiments (unpublished) that showed organisms this size needed about 3 h for sponges and 12 h for corals to reduce oxygen by 20–30%. A “blank” chamber was run concurrently per treatment receiving the same sampling process (including all nutrient and oxygen measurements) as all other chambers, only without an organism inside. This was done to quantify the background changes in all measured variables during an incubation (raw data can be found in Supplementary Data), so that any background changes could be subtracted and rates could be standardized as in Equation 1 (below). All incubations were run in the dark.
Nitrogen release and silicate uptake rates by sponges were determined by measuring the concentration of three nitrogenous waste products that are produced by sponge holobionts; ammonium, nitrate and nitrite ( + ; Hoffmann et al., 2009) and the concentration of silicate () at the beginning and end of incubations. Like all invertebrates, G. atlantica produces ammonium as its primary nitrogenous waste, however, there are symbiotic bacteria contained in the internal and external surfaces of the sponge that oxidize the ammonium to nitrate and nitrite (Hoffmann et al., 2009). Therefore, the total release of nitrogen by sponges was calculated as the sum of the measured ammonium, nitrate and nitrite ( + ). Levels of nitrite () in before and after samples of sponge incubations were below detection levels (<0.06 μmol L−1) and therefore not used in calculating nitrogen release. Nitrogen release by corals was determined by measuring ammonium concentrations (the main source of nitrogenous waste in corals), at the beginning and end of incubations (Szmant et al., 1990). O:N ratios (oxygen uptake vs. nitrogen release) were calculated on a molar basis. This molar ratio can provide a metabolic index that provides information about which catabolic substrates are being used for energy production. This ratio has been used previously on corals and cold water sponges (Szmant et al., 1990; Morley et al., 2016). A greater proportion of nitrogen indicates the organism is using more protein for energy catabolism (Wright, 1995). The molar equivalent of O2 change as calculated per equation 1 (below) was divided by the rate of nitrogen ( for corals and + for sponges) change as calculated by Equation 1 (below).
Following incubations, individual sponge explants and coral branches were removed from the chambers, and coral branches were removed from their stands. Coral branches and sponge explants were then blotted on paper towel, weighed on an electronic balance (± 0.001 g) and then had their volume estimated by placing each sponge or coral in a measuring cylinder with a known volume of seawater; the volume of seawater displaced was recorded and used as the volume of the organism in Equation 1 (below). The viscera of each coral polyp was then removed by cutting the individual polyp away from the calcified stem (that forms the branch like structure of the colony), and then gently removing the polyp from the sclerites using a scalpel before lysosomal stability analysis. To prepare for lysosomal analysis and standardize to dry weight, sponge explants were dissected to remove a small (10 × 10 mm) cube of tissue for lysosomal stability analysis. The remaining sponge was weighed on an electronic balance (± 0.001 g), dried at 70°C for 72 h and weighed again. To estimate dry weights for sponges, the relationship between sponge's wet weight (after dissection) and dry weight was estimated using linear regression (y = 0.171x + 0.4366; R2 = 0.83; P < 0.001). The equation was then used to estimate dry weights of sponges from their original whole wet weight.
All chemical analyses of nutrients were carried out by the Chemical Laboratory at the Institute of Marine Research, (Bergen, Norway) following standard methods with an Alpkem Flow Solution IV autoanalyser (RFA Methodology, 1989; Kérouel and Aminot, 1997; Holmes et al., 1999). Rates of change in nutrients and oxygen during incubations were determined by subtracting the beginning concentration from the end concentration. This change was then standardized to wet weight (coral; only wet weight could be used because the whole colony from each replicate was sacrificed for lysosomal analysis) or estimated dry weight (sponge) using equation 1 modified from Bayne (1999):
Equation 1. Rate standardization calculation.
Where; Vstand h−1 is the measured variable normalized for time (h) and g−1 of dry tissue mass, Vol is the volume of the respiratory chamber minus the volume of the organism (L), ΔVmeas is the change in concentration of the measured variable (μmol or mg), Δt is the whole incubation time (h), Blank is the rate of change in the chamber measured concurrently without an organism, Wb is the tissue mass (dry for sponge or wet for corals) at time of sampling (g).
To determine the cellular membrane stability as a proxy for cellular health of the coral and sponge, the lysosomal membrane stability assay was used. The lysosomal membrane stability assay has been adapted to a wide range of bivalves (e.g., Ringwood et al., 1998; Edge et al., 2012) and recently has been adapted for use on Geodia sponges exposed to sediment (Edge et al., 2016) and thermal stress (Strand et al., 2017). The lysosomal membrane stability in tissue cells was quantified with a neutral red retention assay previously described in detail (Ringwood et al., 1998, 2004; Edge et al., 2012). Briefly, cells from the sponge tissue and coral polyp viscera were extracted by tissue disaggregation to form a suspension. After filtering and washing, the cell suspensions were incubated with a neutral red dye for 60 min. The neutral red retention by lysosomes (6–12 μm) in cells was determined by examining cell suspensions under a light microscope (Olympus 400x). Cells with neutral red sequestered in lysosomes were scored as stable, and those with neutral red leaking into the cytoplasm were scored as destabilized. At least 50 cells were scored for each sample, and data were expressed as the percent of cells with neutral red leaking into the cytosol (% unstable lysosomes).
All data collected at the 26 day sampling time were analyzed using a single factor (Sediment; two levels nominal 0 or 10 mg L−1, n = 5) analysis of variance (Anova). Data collected at the 33 and 40 day sampling times were analyzed using a two factor Anova, where the first factor, Temperature (two levels, nominal 7 or 13°C), was fixed and orthogonal, and the second factor, Sediment (two levels, nominal 0 or 10 mg L−1) was also fixed and orthogonal (n = 5). All data were tested for homogeneity of variances prior to analysis using Cochran's test with α < 0.05). Data collected for P. resedaeformis at 26 days for O:N analysis and 33 days for respiration were transformed by a natural log (lnx) to satisfy the assumption of homogeneity of variances, all other data met this assumption without transformation. All post hoc tests were conducted using SNK tests on the significant factor or interaction of interest (Sokal and Rohlf, 1995). Data analysis was completed using GMAV-5 for Windows (Underwood et al., 2002).
Silicate uptake by G. atlantica was not affected by sediment treatments after 26 days. However, silicate uptake was significantly reduced by suspended sediment after 33 days of exposure [Anova, F(1, 16) = 4.12; P = 0.05]. Silicate uptake was high, although not significantly different, in the elevated temperature treatment after 33 days. After 40 days, silicate uptake by G. atlantica was significantly greater in the control treatment (where silicate uptake was twice as high) compared to the other three treatments [Anova, sediment × temperature interaction F(1, 16) = 7.7; P = 0.01; SNK post-hoc; Figure 2A, Table 1].
Figure 2. Mean (±SE) physiological results of G. atlantica measured after experimental exposure. (A) mean (n = 5) silicate uptake rate (μmol g−1 h−1) after 26, 33, and 40 days respectively; (B), nitrogen release rate (μmol N g−1 h−1) after 26, 33, and 40 days respectively; (C), oxygen uptake rate (μmol O2 g−1 h−1) after 26, 33, and 40 days respectively; and (D), ratio of oxygen uptake to nitrogen release in the form of nitrate and ammonia (O:N; μmol g−1 h−1) of G. atlantica following 26, 33, and 40 days exposure to experimental treatments of; control conditions (no manipulation of variables; 7°C), suspended sediment (10 mg L−1; 7°C), elevated temperature (13°C) and combined suspended sediment; elevated temperature (10 mg L−1; 13°C). Latin letters x – y, roman numerals i-ii, and Greek letters α-β indicate significant differences (P < 0.05) among means as indicated by post-hoc SNK tests after 26, 33, and 40 days in treatments respectively (n = 5).
Table 1. Summary of physiological measurements for of G. atlantica and P. resedaeformis following 26, 33, and 40 days exposure to experimental treatments of; control conditions (no manipulation of variables; 7°C), suspended sediment (10 mg L−1; 7°C), elevated temperature (13°C) and combined suspended sediment; elevated temperature (10 mg L−1; 13°C).
Experimental treatments did not affect the release of nitrogen in the form of nitrate and ammonium by G. atlantica at 26 or 33 days, however, at 40 days there was a significant sediment × temperature interaction [Anova, F(1, 16) = 6.66 P = 0.02]. The sediment + warming treatment had an approximately 50% increase in mean nitrogen release compared to the three other experimental treatments (SNK post-hoc; Figure 2B).
The respiration rate of G. atlantica as indicated by oxygen uptake was not significantly affected by sediment or warming after 26 and 33 days of exposure. After 40 days, there was a significant [Anova, F(1, 16) = 10.1; P = 0.006] temperature × sediment interaction on respiration rate. When exposed to either suspended sediment or warming, the respiration rate of G. atlantica was half the rate of sponges in the control or the sediment + warming treatment (SNK post-hoc; Figure 2C).
The ratio of oxygen uptake to nitrogen release (O:N) of G. atlantica was significantly reduced by suspended sediment [Anova, F(1, 8) = 30.98; P < 0.001] after 26 days. After 33 days the O:N ratio of sponges was reduced compared to the control under both warming [Anova, F(1, 16) = 11.50; P < 0.01], and suspended sediment [Anova, F(1, 16) = 10.9; P = 0.006] as single stressors, however these treatments did not significantly interact. After 40 days, the control treatment had a greater O:N ratio compared to the other 3 treatments [Anova, temperature × sediment interaction F(1, 16) = 7.85; P = 0.01; SNK post-hoc; Figure 2D].
After 26 days exposure to an elevated sediment treatment there was a small but significant increase in the mean percentage of unstable lysosomes in G. atlantica cells when compared to background levels [approximately 4% increase; Anova, F(1, 8) = 6.11; P = 0.025]. After 33 and 40 days there was also a small (approximately 5% for both time points) but significant increase in the percentage of unstable lysosomes of sponges exposed to warming [Anova, 33 days F(1, 16) = 14.6; P = 0.0017, 40 days; F(1, 16) = 7.48; P = 0.014]. There were no effects of sediment on lysosomal stability at 33 or 40 days, and the two stressors did not interact at any time points (Table 2).
Table 2. Summary of mean (± s.e.m.) lysosomal stability results for G. atlantica and P. resedaeformis following 26, 33, and 40 days exposure to experimental treatments of; control conditions (no manipulation of variables; 7°C), suspended sediment (10 mg L−1; 7°C), elevated temperature (13°C) and combined suspended sediment; elevated temperature (10 mg L−1; 13°C).
There were no effects of the sediment on the release of ammonia by P. resedaeformis after 26 days of exposure (Figure 3A, Table 1). However, the release rate of nitrogen by P. resedeaformis in the form of ammonium was significantly increased by warming after both 33 [Anova, F(1, 16) = 16.41; P < 0.001] and 40 days [Anova, F(1, 16) = 48.64; P < 0.0001] in experimental treatments. Nitrogen release rates of P. resedaeformis were more than three times greater when exposed to warming compared to the control treatment.
Figure 3. Mean (± SE) physiological results of P. resedaeformis measured after experimental exposure. (A) mean ammonium release (μmol g−1 h−1) after 26, 33, and 40 days respectively; (B) O:N (; μmol g−1 h−1) ratio of P. resedaeformis following 26, 33, and 40 days respectively; and (C) oxygen uptake rate (μmol O2 g−1 h−1) after 26, 33, and 40 days respectively exposure to experimental treatments of; control conditions (no manipulation of variables; 7°C), suspended sediment (10 mg L−1; 7°C), elevated temperature (13°C) and combined suspended sediment; elevated temperature (10 mg L−1; 13°C). Roman numerals i-ii, and Greek letters α - γ indicate significant differences (P < 0.05) among means as indicated by post-hoc SNK tests after 33, and 40 days in treatments respectively (n = 5).
Treatments did not interact to affect the respiration of P. resedaeformis at any time points, and respiration was also not significantly affected after the first 26 days of exposure to sediment. After 33 days, both warming [Anova, F(1, 16) = 17.84; P < 0.001] and sediment [Anova, F(1, 16) = 5.17; P = 0.037] as single stressors increased the rate at which P. resedaeformis respired (Figure 3B). After 40 days, the respiration rate of P. resedaeformis was only increased by warming [Anova, F(1, 16) = 42.63; P < 0.0001, Figure 3B].
There were no effects of sediment on O:N ratios at 26 days. Warming caused a significant and large [Anova, F(1, 16) = 6.43; P = 0.02] increase in the proportion of nitrogen excreted by P. resedaeformis relative to the oxygen consumed which resulted in a lower O:N ratio after 33 days in treatments. Sediment had no effect on the O:N ratio at 33 days and the stressors did not interact. At 40 days, the O:N ratio of P. resedaeformis was reduced by a sediment × temperature interaction [Anova, F(1, 16) = 5.81; P = 0.024]. The control treatment had the highest O:N ratio, which was greater than all other treatments. The O:N ratio of corals in the sediment treatment were lower than the control by approximately 30%. Further, the O:N ratio of corals in the warming and sediment + warming treatments were significantly lower than both the control and sediment treatment. Corals in these treatments were approximately 60% lower than the control but were not different to each other (SNK post-hoc; Figure 3C).
Warming caused a small but significant increase (approximately 10% increase compared to background) in the percentage of unstable lysosomes of P. resedaeformis after 33 days [Anova, F(1, 16) = 7.56; P = 0.018]. The percentage of unstable lysosomes was not different among treatments and remained at a background level of approximately 20% at both 26 and 40 days (Table 2).
Using controlled mesocosm studies, we have observed for the first time how multiple stressors can influence the physiology of two key deep-sea foundation species. Our results highlight that both G. atlantica and P. resedaeformis can be affected by suspended sediment and acute warming as single, and combined stressors. We found that when G. atlantica and P. resedaeformis were exposed to a combination of suspended sediment and warming, these stressors did not always interact. Whether stressors interacted, and if so, their direction (e.g., additive, synergistic or antagonistic) depended on the time exposed and the variables measured.
Sediment and warming interacted to increase the release of nitrogen in G. atlantica, however, in most cases the effects of the combined sediment + warming treatment did not exceed the effects of the stressors on their own. For P. resedaeformis, exposure to both suspended sediment and warming as single stressors caused the coral to consume oxygen and excrete nitrogen at a greater rate, resulting in lower O:N ratios. Once again, the effects of the sediment + warming treatment rarely exceeded that of the single stressors. We argue that warming and suspended sediment can lower the potential for growth and increase the chances of mortality for these deep-sea foundation species. There is a lack of knowledge surrounding environmental change and the consequences for deep-sea fauna compared to their shallow living relatives; this study provides a unique insight into how pollution may interact with down-welling to affect the ecophysiology of deep-sea foundation species.
Geodia atlantica was sensitive to both elevated suspended sediment and warming. At a cellular level, the stability of lysosomal membranes was compromised after 26 days exposure to suspended sediment, with greater cellular instability at elevated compared to control treatments, an effect that was not observed again at the 33 or 40-day measurement times. This may indicate a capacity for G. atlantica to acclimate to low levels of uncontaminated suspended sediments over a prolonged exposure time; something that was observed in the closely related sponge G. barretti when exposed to low levels of natural sediments (<30 mg L−1), where lysosomal destabilization decreased with increasing time of exposure (Edge et al., 2016). The effect of sediment at 26 days but no other time points may potentially indicate some acclimation, however it may also be because the lysosomal stability test that we used is not sensitive enough to detect low level stress, and is most effective at determining toxic contamination (Ringwood et al., 1998).
Sponges pump water through a system of canals to oxygenate tissues, filter food, and excrete waste products into their surrounding environment. Sponges also retain and use silicate from the water to produce skeletons that give themselves a three dimensional shape and structure (Uriz et al., 2003). Lowering pumping rates have been suggested as a self-preservation tactic used by sponges in order to avoid further damage and clogging when exposed to elevated suspended sediment (Koopmans et al., 2011; Kutti et al., 2015). Furthermore, recent studies on sponges from the Geodia genus have demonstrated that these sponges are sensitive to sediment (Tjensvoll et al., 2013) and respiration was reduced when exposed to different types and concentrations of suspended sediments, indicting a capacity to differentiate between sediment types and react accordingly (Kutti et al., 2015).
We did not directly measure the pumping rates of G. atlantica, however, changes in respiration can indicate changes in pumping as the two functions are related (Bell et al., 2015). It was found that at 26 and 33 days there was a trend for lower respiration under suspended sediment, and after 40 days respiration was significantly lowered under elevated sediment or warming. These results suggest; as single stressors, warming and sediment may lower the pumping of G. atlantica. Further, these effects did not manifest instantly (to a statistically significant level), but rather it took time for the sponge to react to treatments; previous studies have shown that sponges of the Geodia genus can take up to 29 days to respond to low concentrations of suspended sediment (Kutti et al., 2015). Conversely, this was not the case in the warming treatment after 33 days and the warming + sediment treatment after 40 days. After 33 days in the warming treatment both nitrogen release and respiration were not different to the control, and there was a trend for them to be increased. This may be due to a shock effect of warming causing an increase in respiration and possibly pumping. The closely related G. barretti was found to increase respiration two-fold after exposure to warming (11°C) for only 1 day (Strand et al., 2017). After 14 days in a warming treatment, Strand et al. (2017) found the respiration of G. barretti to decrease to half its previous level. We did not observe a trend for a similar respiration increase in the warming + sediment treatment at 33 days, which was perhaps due to an effect of the sediment (Koopmans et al., 2011; Kutti et al., 2015). After 40 days, the sediment + warming treatment interacted to cause an increase in the sponge's respiration to a level that was also not different to the control, but greater than the warming and sediment treatments. Furthermore, this interaction caused an increase in nitrogen release to a level greater than all other treatments. This result could be attributed to elevated energy demand from chronic exposure to interacting stressors; and is example that the interactive effects of these stressors do not always behave in a linear fashion.
Oxygen is necessary for the production of energy and nitrogen is a by-product of this process; however the ratios in which these elements are used and excreted depends on the catabolic substrate. Increases in nitrogen release by the sponge holobiont and a lower O:N ratio may indicate that a greater proportion of sponge metabolism is being met by protein rather than carbohydrates and lipids. This shift in nitrogen flux could also be caused by changes in the symbiotic bacteria that inhabit sponges. Bacterial compositions were not measured in this study, however, in the closely related sponge G. baretti, elevated temperature was found to have no effect on the sponge's microbiota assemblage (Strand et al., 2017). If this result isn't due to a change in symbiotic bacteria, then G. atlantica may be using protein as an energy source. If this were the case, then it would indicate that the sponge is stressed, needs quick energy and may be food limited (Wright, 1995). A rise in energetic demand can be met through mitochondrial energy production, however, this can produce excess reactive oxygen by-products which cause damage to cellular membranes and contribute to cellular instability. This may explain the greater percentage of unstable lysosomes in the warming treatment (Zhang et al., 2006).
Sponges retain and use silicate from the water to produce skeletons that give themselves a three dimensional shape and structure (Uriz et al., 2003), but this process of biomineralisation is energy intensive (Simkiss and Wilbur, 2012). The rate at which sponges absorbed silicate was significantly affected by experimental treatments, however, patterns were not always consistent among treatments and time points. We observed lower silicate uptake after both 33 and 40 days in suspended sediment treatments; an effect that may be explained by a greater energetic demand that was met by a trade-off to decrease silicate deposition, and increase metabolic energy production. Such trade-offs that sacrifice biomineralisation for homeostasis are common (Sokolova et al., 2012; Ivanina et al., 2013; McCoy and Ragazzola, 2014). Alternatively, this may be due to physical blocking of sponge channels by sediment that is impeding the sponge from extracting silicate (Bell et al., 2015). Silicate uptake by G. atlantica was observed to significantly increase in the elevated temperature treatment (13°C; no sediment) after 33 days. This result is counter to previous and subsequent patterns, however, it coincided with greater rates of respiration and nitrogen release; which may indicate that sponges were pumping at a greater rate under elevated temperature and had high energy requirements. Warming has been shown in previous studies to have no effect on silicate uptake on the closely related Geodia baretti (Strand et al., 2017), however, Strand et al. (2017) did observe an increase in oxygen consumption, nitrogen release and cellular instability.
The metabolic rate of cold water sponges can be plastic in response to seasonal change or pollution events (Kutti et al., 2015; Morley et al., 2016) and the nature of this plasticity can be species specific (Morley et al., 2016). Antarctic cold water sponges of Suberites sp. have been shown to increase their metabolic rates during summer which coincides with an increase in O:N ratios (Morley et al., 2016). However, other Antarctic sponges such as Dendrilla Antarctica have a lower metabolic rate in summer which coincides with a lower O:N ratio (Morley et al., 2016). Here, G. atlantica has shown plasticity to deal with a stressful environment by potentially down regulating energy intensive processes such as depositing silicate, upregulating metabolic activity, and utilizing a greater proportion of protein in their metabolism. The closely related sponge G. barretti has been described as resilient to the low levels of suspended sediment used here (Kutti et al., 2015), but also resilient to warming of 5°C (Strand et al., 2017). We observed that G. atlantica may have less energy available for growth under suspended sediment, however pulses of warming do not interact with sediment to cause death or severe tissue loss to G. atlantica. Further studies are needed to determine if longer term exposure to these multiple stressors may have different effects.
We found that exposing the deep-sea coral P. resedaeformis to treatments of suspended sediment and warming caused changes in physiology, and these changes were exacerbated in some cases by combining sediment with warming. How the coral P. resedaeformis reacts to abiotic stressors is largely unknown, however, other deep-sea corals are sensitive to chemicals, sediment and elevated temperature (e.g., Dodds et al., 2007; Ransome et al., 2014; DeLeo et al., 2016). The sensitivity and low resilience of these corals is further compounded by their slow growth rates and longevity (Andrews et al., 2002).
Excess suspended sediment can influence the distribution and growth of shallow water scleractinian coral reefs (Stoddart, 1969; Hubbard and Pocock, 1972; Rogers, 1990). It's been suggested that gorgonian corals are more tolerant to suspended sediment compared to scleractin corals because their tree-like morphology helps to prevent the accumulation of sediment on polyps (Marszalek, 1981). We did not compare the relative resilience of P. resedaeformis to other types of deep-sea corals, however, we did find that suspended sediment and elevated temperature increased respiration and reduced their O:N ratios when each was present as a sole stressor (after 40 days for sediment and 33 days for warming), placing the coral under greater energetic demand. The lowering of the O:N ratio further suggests that this energetic demand is not being met entirely by the catabolism of carbohydrates, but the catabolism of proteins is occurring (Wright, 1995; Gori et al., 2016). An increased energetic demand caused by suspended sediment is likely to be associated with the active removal of sediment from the polyps. Corals can achieve this by multiple methods including the use of their tentacles and cilia (Rogers, 1990; Zetsche et al., 2016a), but also the production of mucus to slough sediment off the polyp surface (Hubbard and Pocock, 1972). Other cold water corals like Lophelia pertusa are known to produce mucus (Zetsche et al., 2016b) in response to excess suspended sediment. The protein synthesis required for mucus production has been estimated to account from 8 to 45 % of expended energy in some corals (Edmunds and Davies, 1986; Crossland, 1987; Brown and Bythell, 2005). Corals are more likely to rely on active methods of sediment removal such as secreting mucus (Rogers, 1990) in areas of low water movement, like the deep-sea. While we did not quantify the amount of mucus produced; mucus production by P. resedaeformis may account for a large portion of the increased energetic demand in sediment treatments. We observed effects of sediment on P. resedaeformis respiration and O:N ratios after 40 days, but not before. This may be due to the chronic effect of sediment in the low concentrations used. Previous studies have shown that corals can be effective at removing sediment, however, cilia exhaustion can occur beyond a threshold, and energy reserves can be depleted (Brown and Bythell, 2005; Zetsche et al., 2016a).
Similar to the effects of suspended sediment, we also found negative effects of warming on P. resedaeformis. Warming was found to cause a doubling in respiration, a four-fold increase in nitrogen release, lower O:N ratios, and lysosomal destabilization. Taken together, these results indicate that P. resedaeformis was likely to be experiencing stress at elevated temperature. Warming has been shown in other studies to permit infection by opportunistic parasites and disease, which can cause tissue loss and death of gorgonian corals (Cerrano et al., 2000). Deep-sea corals are azooxanthellate (i.e., lacking symbiotic dinoflagellates) therefore, they are not susceptible to the bleaching that afflicts photosynthetic corals in shallow water environments (Brown, 1997; Hoegh-Guldberg, 1999). Despite the absence of symbiotic dinoflagellates, we found that the physiology of P. resedaeformis was dependent on warming. We observed a ≈100% increase in the amount of oxygen consumed by P. resedaeformis in the warming treatment. Another cold water coral with a similar temperature tolerance; L. pertusa was found to increase respiration by ≈50% when temperature was raised from 9 to 11°C (Dodds et al., 2007). The authors suggested in this case that L. pertusa was sensitive to warming (Dodds et al., 2007); a finding that may be also extended to P. resedaeformis. Elevated temperature can reduce the oxygen transporting capacity of an organism, to a point where oxygen deficiency defines their tolerance to warming (Pörtner, 2001, 2002). This limited oxygen supply at the upper thresholds of temperature tolerance can cause invertebrates to increase their anaerobic metabolism (Pörtner, 2001, 2002). Anaerobic metabolism requires protein as a catabolic substrate, which produces nitrogen as a waste product (De Zwaan and Zandee, 1972; de Zwaan and Wijsman, 1976). This process may explain why we found corals to excrete a greater amount of nitrogen and have lower O:N ratios at elevated temperature.
Temperature is an important factor in limiting the distribution of P. resedaeformis in the northern Atlantic Ocean, with this octocoral occurring in habitats with an average of 5.3°C, however P. resedaeformis has been recorded in the wild at temperatures as high as 12°C (Mortensen and Buhl-Mortensen, 2004). This is only 1°C lower than we used in our study, where we observed P. resedaeformis to be likely stressed at 13°C. Given that P. resedaeformis can survive in the wild at 12°C (Mortensen and Buhl-Mortensen, 2004) the effects that we observed may be associated with the rapid onset of elevated temperature, rather than an inability to survive at these temperatures if given adequate acclimation. Our study is the first to our knowledge to test the physiological responses of P. resedaeformis to warming. Our results support the suggestion by Mortensen and Buhl-Mortensen (2004) that 12−13°C is the upper limit of P. resedaeformis thermal tolerance, however further studies would be required to accurately test this limit.
The sediment used in our study was chosen to represent inert mine waste that is released into fjords, testing the effects of physical stress. Real-world mine waste, however, is often not inert but contains harmful chemicals of both a natural or human origin (Bakke et al., 2013). Natural compounds found in mine waste like heavy metals, can have impacts on biota that reach beyond the physical effects of sediment that we observed (Loring and Asmund, 1989). Furthermore, mine waste and the by-products of oil drilling and exploration will often contain man made substances like flocculants, drilling muds and produced water (Neff et al., 2011; Bakke et al., 2013). These substances are toxic, and can cause significant harm to deep-sea biota including sponges and corals (Bakke et al., 2013; Kutti et al., 2015; DeLeo et al., 2016; Edge et al., 2016; Fang et al., in press). If we had included sediment containing toxic compounds in our study, it's likely that the sponge and coral would have experienced more damage to their cells, a greater metabolic shift and even death.
Here we have tested how a common deep-sea sponge (G. atlantica) and octocoral (P. resedaeformis) responded to simulated sediment exposure and warming in a laboratory setting. Studies such as this are beginning to expand our knowledge surrounding deep-sea fauna and environmental change; however, here we have only “scratched the surface” by investigating a small fraction of deep-sea biodiversity and potential combining stressors. Furthermore, we have shown that their responses can be highly species specific, which makes it even more difficult to generalize results among other deep-sea fauna. Future studies should focus on the effects of chemical contaminants associated with disposing mine wastes and drill cuttings and their effects on different species of deep-sea fauna over longer periods of exposure, and perhaps investigate the effects on a community rather than species level. We conclude that sediment and warming can interact in some cases to lower the potential for growth and increase the chance of mortality for some deep-sea foundation species. A loss in abundance or biomass of these species would likely affect deep-sea ecosystems, resulting in lower abundance and diversity of associated fauna, as well as a reduced capacity for benthic–pelagic coupling and carbon cycling in the deep-sea (Dayton, 1972; Bruno and Bertness, 2001).
This study was undertaken and designed by ES, RB, TK, and JF. All authors were involved in the interpretation of data and the writing of this manuscript.
This project was partially financed through the Institute of Marine Research on project grant no. 14515 and the Norwegian Research Council on project grant no. 225283 awarded to RB, and the FIMITA project of FRAM–High North Research Centre for Climate and Environment awarded to TK.
The authors declare that the research was conducted in the absence of any commercial or financial relationships that could be construed as a potential conflict of interest.
The authors wish to acknowledge the help of Cathinka Krogness for her generous help with the collection of data, the station manager and staff at the Institute of Marine Research's Research station in Austevoll, Norway, and the IMR chemistry lab for their analysis of samples. We would like to thank the two reviewers for their comments that improved this paper.
The Supplementary Material for this article can be found online at: https://www.frontiersin.org/articles/10.3389/fmars.2018.00129/full#supplementary-material
Allan, R. J. (1995). “Impact of mining activities on the terrestrial and aquatic environment with emphasis on mitigation and remedial measures,” in Heavy Metals, eds U. Förstner, W. Salomons, and P. Mader (Berlin: Springer), 119–140.
Althaus, F., Williams, A., Schlacher, T. A., Kloser, R. J., Green, M. A., Barker, B. A., et al. (2009). Impacts of bottom trawling on deep-coral ecosystems of seamounts are long-lasting. Mar. Ecol. Prog. Ser. 397, 279–294. doi: 10.3354/meps08248
Andrews, A. H., Cordes, E. E., Mahoney, M. M., Munk, K., Coale, K. H., Cailliet, G. M., et al. (2002). Age, growth and radiometric age validation of a deep-sea, habitat-forming gorgonian (Primnoa resedaeformis) from the Gulf of Alaska. Hydrobiologia 471, 101–110. doi: 10.1023/A:1016501320206
Anthony, K. (1999). A tank system for studying benthic aquatic organisms at predictable levels of turbidity and sedimentation: case study examining coral growth. Limnol. Oceanogr. 44, 1415–1422. doi: 10.4319/lo.1999.44.6.1415
Bakke, T., Klungsøyr, J., and Sanni, S. (2013). Environmental impacts of produced water and drilling waste discharges from the Norwegian offshore petroleum industry. Mar. Env. Res. 92, 154–169. doi: 10.1016/j.marenvres.2013.09.012
Bannister, R. J., Battershill, C. N., and de Nys, R. (2011). Demographic variability and long-term change in a coral reef sponge along a cross-shelf gradient of the great barrier reef. Mar. Freshwater Res. 61, 389–396. doi: 10.1071/MF09067
Bannister, R. J., Battershill, C. N., and de Nys, R. (2012). Suspended sediment grain size and mineralogy across the continental shelf of the Great Barrier Reef: impacts on the physiology of a coral reef sponge. Continent. Shelf Res. 32, 86–95. doi: 10.1016/j.csr.2011.10.018
Bayne, B. (1999). Physiological components of growth differences between individual oysters (Crassostrea gigas) and a comparison with Saccostrea commercialis. Physiol. Biochem. Zool. 72, 705–713.
Bell, J. J., McGrath, E., Biggerstaff, A., Bates, T., Bennett, H., Marlow, J., et al. (2015). Sediment impacts on marine sponges. Mar. poll. Bull. 94, 5–13. doi: 10.1016/j.marpolbul.2015.03.030
Brooks, S. J., Harman, C., Hultman, M. T., and Berge, J. A. (2015). Integrated biomarker assessment of the effects of tailing discharges from an iron ore mine using blue mussels (Mytilus spp.). Sci. Total Environ. 524, 104–114. doi: 10.1016/j.scitotenv.2015.03.135
Brown, B., and Bythell, J. (2005). Perspectives on mucus secretion in reef corals. Mar. Ecol. Prog. Ser. 296, 291–309. doi: 10.3354/meps296291
Brown, B. E. (1997). Coral bleaching: causes and consequences. Coral Reefs 16, S129–S138. doi: 10.1007/s003380050249
Bruno, J. F., and Bertness, M. D. (2001). “Habitat modification and facilitation in benthic marine communities” in. Marine Community Ecology, eds M. D. Bertness et al. (Sinauer), 201–218.
Buhl-Mortensen, L., Vanreusel, A., Gooday, A. J., Levin, L. A., Priede, I. G., Buhl-Mortensen, P., et al. (2010). Biological structures as a source of habitat heterogeneity and biodiversity on the deep ocean margins. Mar. Ecol. 31, 21–50. doi: 10.1111/j.1439-0485.2010.00359.x
Buhl-Mortensen, L., and Mortensen, P. B. (2004). Crustaceans associated with the deep-water gorgonian corals Paragorgia arborea (L., 1758) and Primnoa resedaeformis (Gunn., 1763). J. Natur. Hist. 38, 1233–1247. doi: 10.1080/0022293031000155205
Buhl-Mortensen, L., and Mortensen, P. B. (2005). “Distribution and diversity of species associated with deep-sea gorgonian corals off Atlantic Canada,” in Cold-water corals and ecosystems, eds A. Freiwald and J. M. Roberts (Berlin: Springer), 849–879.
Buhl-Mortensen, L., Olafsdottir, S. H., Buhl-Mortensen, P., Burgos, J. M., and Ragnarsson, S. A. (2015). Distribution of nine cold-water coral species (Scleractinia and Gorgonacea) in the cold temperate North Atlantic: effects of bathymetry and hydrography. Hydrobiologia 759, 39–61. doi: 10.1007/s10750-014-2116-x
Cathalot, C., Van Oevelen, D., Cox, T. J., Kutti, T., Lavaleye, M., Duineveld, G., et al. (2015). Cold-water coral reefs and adjacent sponge grounds: hotspots of benthic respiration and organic carbon cycling in the deep-sea. Front. Mar. Sci. 2:37. doi: 10.3389/fmars.2015.00037
Cerrano, C., Bavestrello, G., Bianchi, C. N., Cattaneo-Vietti, R., Bava, S., Morganti, C., et al. (2000). A catastrophic mass-mortality episode of gorgonians and other organisms in the Ligurian Sea (North-western Mediterranean), summer 1999. Ecol. Lett. 3, 284–293. doi: 10.1046/j.1461-0248.2000.00152.x
Collins, M., Knutti, R., Arblaster, J., Dufresne, J.-L., Fichefet, T., Friedlingstein, P., et al. (2013). “Long-term climate change: projections, commitments and irreversibility,” in Climate Change 2013: The Physical Science Basis. Contribution of Working Group I to the Fifth Assessment Report of the Intergovernmental Panel on Climate Change, eds T. F. Stocker, D. Qin, G.-K. Plattner, M. Tignor, S. K. Allen, J. Boschung, A. Nauels, Y. Xia, V. Bex, and P. M. Midgley (New York, NY: Cambridge University Press), 1029–1136.
Crain, C. M., Kroeker, K., and Halpern, B. S. (2008). Interactive and cumulative effects of multiple human stressors in marine systems. Ecol. Lett. 11, 1304–1315. doi: 10.1111/j.1461-0248.2008.01253.x
Crossland, C. (1987). In situ release of mucus and DOC-lipid from the corals Acropora variabilis and Stylophora pistillata in different light regimes. Coral Reefs 6, 35–42. doi: 10.1007/BF00302210
Danovaro, R., Gambi, C., Dell'Anno, A., Corinaldesi, C., Fraschetti, S., Vanreusel, A., et al. (2008). Exponential decline of deep-sea ecosystem functioning linked to benthic biodiversity loss. Curr. Biol. 18, 1–8. doi: 10.1016/j.cub.2007.11.056
Danovaro, R., Snelgrove, P. V., and Tyler, P. (2014). Challenging the paradigms of deep-sea ecology. Trends Ecol. Evolut. 29, 465–475. doi: 10.1016/j.tree.2014.06.002
Dayton, P. K. (1972). “Toward an understanding of community resilience and the potential effects of enrichments to the benthos at McMurdo Sound, Antarctica,” in Proceedings of the Colloquium on Conservation Problems in Antarctica, (Lawrence, KS: Allen Press), 81–95.
DeLeo, D. M., Ruiz-Ramos, D. V., Baums, I. B., and Cordes, E. E. (2016). Response of deep-water corals to oil and chemical dispersant exposure. Deep Sea Res. Part II Topic. Stud. Oceanogr. 129, 137–147. doi: 10.1016/j.dsr2.2015.02.028
de Zwaan, A., and Wijsman, T. (1976). Anaerobic metabolism in bivalvia (Mollusca) characteristics of anaerobic metabolism. Comp. Bioch. Physiol. B Comp. Biochem. 54, 313–323.
De Zwaan, A., and Zandee, D. (1972). The utilization of glycogen and accumulation of some intermediates during anaerobiosis in Mytilus edulis L. Comp. Bioch. Physiol. B Comp. Biochem. 43, 47–54.
Dodds, L. A., Roberts, J. M., Taylor, A. C., and Marubini, F. (2007). Metabolic tolerance of the cold-water coral Lophelia pertusa (Scleractinia) to temperature and dissolved oxygen change. J. Exp. Mar. Biol. Ecol. 349, 205–214. doi: 10.1016/j.jembe.2007.05.013
Edge, K. J., Johnston, E. L., Dafforn, K. A., Simpson, S. L., Kutti, T., and Bannister, R. J. (2016). Sub-lethal effects of water-based drilling muds on the deep-water sponge Geodia barretti. Environ. Poll. 212, 525–534. doi: 10.1016/j.envpol.2016.02.047
Edge, K. J., Johnston, E. L., Roach, A. C., and Ringwood, A. H. (2012). Indicators of environmental stress: cellular biomarkers and reproductive responses in the Sydney rock oyster (Saccostrea glomerata). Ecotoxicol. 21, 1415–1425. doi: 10.1007/s10646-012-0895-2
Edmunds, P., and Davies, P. S. (1986). An energy budget for Porites porites (Scleractinia). Mar. Biol. 92, 339–347.
Ellison, A. M., Bank, M. S., Clinton, B. D., Colburn, E. A., Elliott, K., Ford, C. R., et al. (2005). Loss of foundation species: consequences for the structure and dynamics of forested ecosystems. Front. Ecol. Environ. 3, 479–486. doi: 10.1890/1540-9295(2005)003[0479:LOFSCF]2.0.CO;2
Fang, J. K. H., Rooks, C. A., Krogness, C. M., Kutti, T., Hoffmann, F., and Bannister, R. J. (in press). Impact of particulate sediment, bentonite barite (oil-drilling waste) on net fluxes of oxygen nitrogen in Arctic-boreal sponges. Environ. Poll.
Folt, C., Chen, C., Moore, M., and Burnaford, J. (1999). Synergism and antagonism among multiple stressors. Limnol. Oceanog. 44, 864–877. doi: 10.4319/lo.1999.44.3_part_2.0864
Fosså, J. H., Mortensen, P., and Furevik, D. M. (2002). The deep-water coral Lophelia pertusa in Norwegian waters: distribution and fishery impacts. Hydrobiologia 471, 1–12. doi: 10.1023/A:1016504430684
Gori, A., Ferrier-Pagès, C., Hennige, S. J., Murray, F., Rottier, C., Wicks, L. C., et al. (2016). Physiological response of the cold-water coral Desmophyllum dianthus to thermal stress and ocean acidification. PeerJ 4:e1606. doi: 10.7717/peerj.1606
Guihen, D., White, M., and Lundälv, T. (2012). Temperature shocks and ecological implications at a cold-water coral reef. Mar. Biodivers. Rec. 5:e68. doi: 10.1017/S1755267212000413
Hoegh-Guldberg, O. (1999). Climate change, coral bleaching and the future of the world's coral reefs. Mar. Freshwater Res. 50, 839–866. doi: 10.1071/MF99078
Hoffmann, F., Radax, R., Woebken, D., Holtappels, M., Lavik, G., Rapp, H. T., et al. (2009). Complex nitrogen cycling in the sponge Geodia barretti. Environ. Microbiol. 11, 2228–2243. doi: 10.1111/j.1462-2920.2009.01944.x
Hoffmann, F., Rapp, H. T., Zöller, T., and Reitner, J. (2003). Growth and regeneration in cultivated fragments of the boreal deep water sponge Geodia barretti Bowerbank, 1858 (Geodiidae, Tetractinellida, Demospongiae). J. Biotech. 100, 109–118. doi: 10.1016/S0168-1656(02)00258-4
Hogg, M. M., Tendal, O. S., Conway, K. W., Pomponi, S. A., Van Soest, R. W. M., Gutt, J., et al. (2010). Deep-Sea Sponge Grounds: Reservoirs of Biodiversity. UNEP-WCMC Biodiversity Series No. 32. Cambridge, UK: UNEP-WCMC.
Holmes, R. M., Aminot, A., Kérouel, R., Hooker, B. A., and Peterson, B. J. (1999). A simple and precise method for measuring ammonium in marine and freshwater ecosystems. Can. J. Fish. Aquat. Sci. 56, 1801–1808.
Hubbard, J. A., and Pocock, Y. P. (1972). Sediment rejection by recent scleractinian corals: a key to palaeo-environmental reconstruction. Geol. Rundsch. 61, 598–626. doi: 10.1007/BF01896337
Ivanina, A. V., Dickinson, G. H., Matoo, O. B., Bagwe, R., Dickinson, A., Beniash, E., et al. (2013). Interactive effects of elevated temperature and CO2 levels on energy metabolism and biomineralization of marine bivalves Crassostrea virginica and Mercenaria mercenaria. Comp. Biochem. Physiol,. Part A Mol. Integr. Physiol. 166, 101–111. doi: 10.1016/j.cbpa.2013.05.016
Jiménez, E., and Ribes, M. (2007). Sponges as a source of dissolved inorganic nitrogen: nitrification mediated by temperate sponges. Limnol. Oceanog. 52, 948–958. doi: 10.4319/lo.2007.52.3.0948
Kérouel, R., and Aminot, A. (1997). Fluorometric determination of ammonia in sea and estuarine waters by direct segmented flow analysis. Mar. Chem. 57, 265–275. doi: 10.1016/S0304-4203(97)00040-6
Klitgaard, A. (1995). The fauna associated with outer shelf and upper slope sponges (Porifera, Demospongiae) at the Faroe Islands, northeastern Atlantic. Sarsia 80, 1–22. doi: 10.1080/00364827.1995.10413574
Klitgaard, A. B., and Tendal, O. S. (2004). Distribution and species composition of mass occurrences of large-sized sponges in the northeast Atlantic. Prog. Oceanog. 61, 57–98. doi: 10.1016/j.pocean.2004.06.002
Koopmans, M., Van Rijswijk, P., Martens, D., Egorova-Zachernyuk, T., Middelburg, J., and Wijffels, R. (2011). Carbon conversion and metabolic rate in two marine sponges. Mar. Biol. 158, 9–20. doi: 10.1007/s00227-010-1538-x
Krieger, K. J., and Wing, B. L. (2002). Megafauna associations with deepwater corals (Primnoa spp.) in the Gulf of Alaska. Hydrobiologia 471, 83–90. doi: 10.1023/A:1016597119297
Kutti, T., Bannister, R. J., and Foss,å, J. H. (2013). Community structure and ecological function of deep-water sponge grounds in the Traenadypet MPA—Northern Norwegian continental shelf. Cont. Shelf Res. 69, 21–30. doi: 10.1016/j.csr.2013.09.011
Kutti, T., Bannister, R. J., Fosså, J. H., Krogness, C. M., Tjensvoll, I., and Søvik, G. (2015). Metabolic responses of the deep-water sponge Geodia barretti to suspended bottom sediment, simulated mine tailings and drill cuttings. J. Exp. Mar. Biol. Ecol. 473, 64–72. doi: 10.1016/j.jembe.2015.07.017
Kutti, T., Bergstad, O. A., Fosså, J. H., and Helle, K. (2014). Cold-water coral mounds and sponge-beds as habitats for demersal fish on the Norwegian shelf. Deep Sea Res. Part II Topic. Stud. Oceanogr. 99, 122–133. doi: 10.1016/j.dsr2.2013.07.021
Kvassnes, A. J. S., and Iversen, E. (2013). Waste sites from mines in Norwegian Fjords. Mineralproduksjon 3, A27–A38. Available online at: http://mineralproduksjon.no/wp-content/uploads/2017/03/MP3-D-VA-Kvassnes-PRINT.pdf
Levin, L. A., and Dayton, P. K. (2009). Ecological theory and continental margins: where shallow meets deep. Trend. Ecol. Evol. 24, 606–617. doi: 10.1016/j.tree.2009.04.012
Loring, D. H., and Asmund, G. (1989). Heavy metal contamination of a Greenland fjord system by mine wastes. Environ. Geol. Water Sci. 14, 61–71. doi: 10.1007/BF01740586
Marszalek, D. S. (1981). “Impact of dredging on a subtropical reef community, southeast Florida, U. S. A.” in 4. International Coral Reef Symposium, Manila, (Philippines), 18–22.
McCave, I. (1975). Vertical flux of particles in the ocean. Deep Sea Res. Oceanogr. Abstr. 22, 491–502. doi: 10.1016/0011-7471(75)90022-4
McCoy, S. J., and Ragazzola, F. (2014). Skeletal trade-offs in coralline algae in response to ocean acidification. Nat. Clim. Chan. 4, 719–723. doi: 10.1038/nclimate2273
Metaxas, A., and Davis, J. (2005). Megafauna associated with assemblages of deep-water gorgonian corals in Northeast Channel, off Nova Scotia, Canada. J. Mar. Biol. Assoc. U. K. 85, 1381–1390. doi: 10.1017/S0025315405012567
Mevenkamp, L., Stratmann, T., Guilini, K., Moodley, L., van Oevelen, D., Vanreusel, A., et al. (2017). Impaired short-term functioning of a benthic community from a deep Norwegian fjord following deposition of mine tailings and sediments. Front. Mar. Sci. 4:169.doi: 10.3389/fmars.2017.00169
Morley, S. A., Berman, J., Barnes, D. K., de Juan Carbonell, C., Downey, R. V., and Peck, L. S. (2016). Extreme phenotypic plasticity in metabolic physiology of Antarctic demosponges. Front. Ecol. Evol. 3:157. doi: 10.3389/fevo.2015.00157
Mortensen, P. B., and Buhl-Mortensen, L. (2004). Distribution of deep-water gorgonian corals in relation to benthic habitat features in the Northeast Channel (Atlantic Canada). Mar. Biol. 144, 1223–1238. doi: 10.1007/s00227-003-1280-8
Mortensen, P. B., Hovland, M., Brattegard, T., and Farestveit, R. (1995). Deep water bioherms of the scleractinian coral Lophelia pertusa (L.) at 64° N on the Norwegian shelf: structure and associated megafauna. Sarsia 80, 145–158. doi: 10.1080/00364827.1995.10413586
Neff, J., Lee, K., and DeBlois, E. M. (2011). “Produced water: overview of composition, fates, and effects,” in Produced Water, eds J. Neff and K. Lee (New York, NY: Springer), 3–54.
Olsgard, F., and Hasle, J. R. (1993). Impact of waste from titanium mining on benthic fauna. J. Exp. Mar. Biol. Ecol. 172, 185–213.
Pörtner, H. (2001). Climate change and temperature-dependent biogeography: oxygen limitation of thermal tolerance in animals. Naturwissenschaften 88, 137–146. doi: 10.1007/s001140100216
Pörtner, H.-O. (2002). Climate variations and the physiological basis of temperature dependent biogeography: systemic to molecular hierarchy of thermal tolerance in animals. Comp. Biochem. Physiol. A Mol. Integr. Physiol. 132, 739–761. doi: 10.1016/S1095-6433(02)00045-4
Pörtner, H. O., and Farrell, A. P. (2008). Physiology and climate change. Science 322, 690–692. doi: 10.1126/science.1163156
Purser, A., and Thomsen, L. (2012). Monitoring strategies for drill cutting discharge in the vicinity of cold-water coral ecosystems. Mar. Poll. Bull. 64, 2309–2316. doi: 10.1016/j.marpolbul.2012.08.003
Ransome, E., Rowley, S. J., Thomas, S., Tait, K., and Munn, C. B. (2014). Disturbance to conserved bacterial communities in the cold-water gorgonian coral Eunicella verrucosa. FEMS Microbiol. Ecol. 90, 404–416. doi: 10.1111/1574-6941.12398
RFA Methodology (1989). Nitrate + Nitrite Nitrogen. A303-S170 Revision 6–89, College Station, TX: Alpkem.
Ringwood, A. H., Conners, D. E., and Hoguet, J. (1998). Effects of natural and anthropogenic stressors on lysosomal destabilization in oysters Crassostrea virginica. Mar. Ecol. Prog. Ser. 166, 163–171.
Ringwood, A. H., Hoguet, J., Keppler, C., and Gielazyn, M. (2004). Linkages between cellular biomarker responses and reproductive success in oysters–Crassostrea virginica. Mar. Env. Res. 58, 151–155. doi: 10.1016/j.marenvres.2004.03.010
Roberts, J. M., and Cairns, S. D. (2014). Cold-water corals in a changing ocean. Curr. Opin. Environ. Sustain. 7, 118–126. doi: 10.1016/j.cosust.2014.01.004
Rogers, C. S. (1990). Responses of coral reefs and reef organisms to sedimentation. Mar. Ecol. Prog. Ser. 62, 185–202.
Sokal, R. R., and Rohlf, F. J. (1995). Biometry: The Principles and Practice of Statistics in Biological Research. New York, NY: WH Freeman.
Sokolova, I. M. (2013). Energy-limited tolerance to stress as a conceptual framework to integrate the effects of multiple stressors. Integ. Comp. Biol. 53, 597-608. doi: 10.1093/icb/ict028
Sokolova, I. M., Frederich, M., Bagwe, R., Lannig, G., and Sukhotin, A. A. (2012). Energy homeostasis as an integrative tool for assessing limits of environmental stress tolerance in aquatic invertebrates. Mar. Environ. Res. 79, 1–15. doi: 10.1016/j.marenvres.2012.04.003
Stoddart, D. R. (1969). Ecology and morphology of recent coral reefs. Biol. Rev. 44, 433–498. doi: 10.1111/j.1469-185X.1969.tb00609.x
Strand, R., Whalan, S., Webster, N. S., Kutti, T., Fang, J. K. H., Luter, H. M., et al. (2017). The response of a boreal deep-sea sponge holobiont to acute thermal stress. Sci. Rep. 7:1660. doi: 10.1038/s41598-017-01091-x
Sweetman, A. K., Thurber, A. R., Smith, C. R., Levin, L. A., Mora, C., and Wei, C. L. (2017). Major impacts of climate change on deep-sea benthic ecosystems. Elem Sci Anth. 5:4. doi: 10.1525/elementa.203
Szmant, A. M., Ferrer, L. M., and FitzGerald, L. M. (1990). Nitrogen excretion and O: N ratios in reef corals: evidence for conservation of nitrogen. Mar. Biol. 104, 119–127. doi: 10.1007/BF01313165
Tjensvoll, I., Kutti, T., Fosså, J. H., and Bannister, R. (2013). Rapid respiratory responses of the deep-water sponge Geodia barretti exposed to suspended sediments. Aquat. Biol. 19, 65-73. doi: 10.3354/ab00522
Tompkins-MacDonald, G. J., and Leys, S. P. (2008). Glass sponges arrest pumping in response to sediment: implications for the physiology of the hexactinellid conduction system. Mar. Biol. 154:973. doi: 10.1007/s00227-008-0987-y
Underwood, A., Chapman, M., and Richards, S. (2002). GMAV-5 for Windows. An Analysis of Variance Programme, Centre for Research on Ecological Impacts of Coastal Cities. Marine Ecology Laboratories, University of Sydney Australia.
Uriz, M. J., Turon, X., Becerro, M. A., and Agell, G. (2003). Siliceous spicules and skeleton frameworks in sponges: origin, diversity, ultrastructural patterns, and biological functions. Micros. Res. Tech. 62, 279–299. doi: 10.1002/jemt.10395
Wisshak, M., and Rüggeberg, A. (2006). Colonisation and bioerosion of experimental substrates by benthic foraminiferans from euphotic to aphotic depths (Kosterfjord, SW Sweden). Facies 52, 1–17. doi: 10.1007/s10347-005-0033-1
Wright, P. A. (1995). Nitrogen excretion: three end products, many physiological roles. J. Exp. Biol. 198, 273–281.
Zetsche, E.-M., Baussant, T., and Van Oevelen, D. (2016a). Ciliary removal of particles by the cold-water coral Lophelia pertusa. Coral Encount. 31, 53–56. Available online at: http://www.vliz.be/imisdocs/publications/06/295606.pdf
Zetsche, E.-M., Baussant, T., Meysman, F. J. R., and van Oevelen, D. (2016b). Direct Visualization of Mucus Production by the Cold-Water Coral Lophelia pertusa with Digital Holographic Microscopy. PLoS ONE 11:e0146766. doi: 10.1371/journal.pone.0146766
Keywords: deep-sea, multiple stressors, climate change, mine waste, stress, suspended sediment, down-welling
Citation: Scanes E, Kutti T, Fang JKH, Johnston EL, Ross PM and Bannister RJ (2018) Mine Waste and Acute Warming Induce Energetic Stress in the Deep-Sea Sponge Geodia atlantica and Coral Primnoa resedeaformis; Results From a Mesocosm Study. Front. Mar. Sci. 5:129. doi: 10.3389/fmars.2018.00129
Received: 01 December 2017; Accepted: 27 March 2018;
Published: 12 April 2018.
Edited by:
Telmo Morato, University of the Azores, PortugalReviewed by:
Ann I. Larsson, University of Gothenburg, SwedenCopyright © 2018 Scanes, Kutti, Fang, Johnston, Ross and Bannister. This is an open-access article distributed under the terms of the Creative Commons Attribution License (CC BY). The use, distribution or reproduction in other forums is permitted, provided the original author(s) and the copyright owner are credited and that the original publication in this journal is cited, in accordance with accepted academic practice. No use, distribution or reproduction is permitted which does not comply with these terms.
*Correspondence: Elliot Scanes, ZWxsaW90LnNjYW5lc0BzeWRuZXkuZWR1LmF1
Disclaimer: All claims expressed in this article are solely those of the authors and do not necessarily represent those of their affiliated organizations, or those of the publisher, the editors and the reviewers. Any product that may be evaluated in this article or claim that may be made by its manufacturer is not guaranteed or endorsed by the publisher.
Research integrity at Frontiers
Learn more about the work of our research integrity team to safeguard the quality of each article we publish.