- 1Unité Biologie des Organismes et Ecosystèmes Aquatiques, Muséum National d'Histoire Naturelle, Centre National de la Recherche Scientifique (UMR-7208), Sorbonne Université, Institut de Recherche pour le Développement, Université de Caen Normandie, Université des Antilles, Paris, France
- 2Sorbonne Université, Centre National de la Recherche Scientifique (UMR-7574), Collège de France, Laboratoire de Chimie de la Matière Condensée de Paris, Paris, France
- 3Sorbonne Université, Centre National de la Recherche Scientifique (UMR-7588), Institut des Nanosciences de Paris, Paris, France
- 4Université du Maine, Centre National de la Recherche Scientifique (UMR-6283), Institut des Molécules et Matériaux du Mans, Le Mans, France
- 5Université Paris Diderot, Paris, France
Light is important for the growth, behavior, and development of both phototrophic and autotrophic organisms. A large diversity of organisms used silica-based materials as internal and external structures. Nano-scaled well-organized silica biomaterials are characterized by a low refractive index and an extremely low absorption coefficient in the visible range, which make them interesting for optical studies. Recent studies on silica materials from glass sponges and diatoms, have pointed out very interesting optical properties, such as light waveguiding, diffraction, focusing, and photoluminescence. Light guiding and focusing have been shown to be coupled properties found in spicule of glass sponge or shells of diatoms. Moreover, most of these interesting studies have used purified biomaterials and the properties have addressed in non-aquatic environments, first in order to enhance the index contrast in the structure and second to enhance the spectral distribution. Although there is many evidences that silica biomaterials can present interesting optical properties that might be used for industrial purposes, it is important to emphases that the results were obtained from a few numbers of species. Due to the key roles of light for a large number of marine organisms, the development of experiments with living organisms along with field studies are require to better improve our understanding of the physiological and structural roles played by silica structures.
Introduction
Silica biominerals are the results of various internal or external biological processes that lead to the formation of composite materials with a large variety of composition, hierarchical structures, and probably functions. Silicon biomineralization capability is encountered in various organisms from bacteria to humans, including organisms that belong to the Archaeplastida as well as a large diversity of other photosynthetic and non-photosynthetic protists (i.e., unicellular organisms that are free-living or aggregated into simple colonies, such as eukaryotic algae, protozoans, or slime molds; Raven and Giordano, 2009; Ehrlich et al., 2010a).
The formation of these silica structures might however contribute to the protection of cell in such harsh environment. In terrestrial environments, a large number of land plants were known to mobilize significant quantities of silica in different tissues, where silica might serve as a structural scaffolding element, and can be involved in defense against herbivores and pathogens (Liu et al., 2017; Luyckx et al., 2017; Wang et al., 2017), or against various abiotic stresses (Cooke and Leishman, 2016). Silica minerals where also shown to contribute to optimize the light regime in the leaf (Pierantoni et al., 2017), and proposed to be involve in protecting the land plants against UV-radiation (Schaller et al., 2013).
In the marine environment, the silica frustule made by diatoms which can present extraordinary mechanical strength (Hamm et al., 2003; Aitken et al., 2016), contribute to their mechanical defense against grazers and it was shown that the silicification level can influence interactions between diatoms and grazers (Pondaven et al., 2007; Schultes et al., 2010; Liu et al., 2016), for example heterotrophic dinoflagellates preferentially feed on diatoms with low silica content (Zhang et al., 2017). The overall density of the silica wall also affects the overall sinking rate of diatoms, and help the cells in escaping from predators and parasites, avoiding high light intensity, or finding new resource areas (Raven and Waite, 2004). The silica spicules provide support and defense to marine sponges (Burns and Ilan, 2003; Rohde and Schupp, 2011), correspond to an import feature that can serve as anchoring structure to hold on the sea floor (Ehrlich et al., 2010b; Monn et al., 2015; Monn and Kesari, 2017). However, the exact roles and properties of such silica structures for light perception, UV protection or signal transduction has been rarely investigated in vivo. One of the reason is the difficulty to develop precise measurement with living cells also because of the interferences of the organic matter but also the large impacts of organelles like the chloroplast which can greatly interfere with light and fluorescence signal analyses. Nevertheless, understanding the physical properties of purified silica-based biomaterials which is important to novel develop industrial applications, might also give some insight to better understand their putative biological. The interactions between light and silica material is the focus of this review starting from some theoretical background to the recent advances in this field.
Theoretical Background
Light Guidance and Reflection
In order to understand guiding light ability, fundamental physical principles on the injection of light into materials structure and propagation of electromagnetic waves must be recalled. Light propagation over long distances is based on the total reflection phenomenon. When a light ray falls on a diopter that is a plan surface separating two different media of different refractive index n1 and n2, a part of this beam is reflected and leaves again in the medium of incidence at an angle θR equal to that of incidence θi, while the remaining part continues its path in the second medium at an angle θr different from the angle of incidence, so called refracted beam. The famous law of Snell-Descartes connects these different angles:
After the diopter, the refracted ray deviates from the normal one with an angle that depends on the two indexes values difference (Figures 1A,B). When the incidence angle exceeds a critical θc value (calculated by dividing n2 by n1), the refracted light is tangential to the surface: there is no longer refraction and the light is totally reflected (Figure 1C). For this exact angle, it is said the wave is evanescent and propagates by damping rapidly along the surface.
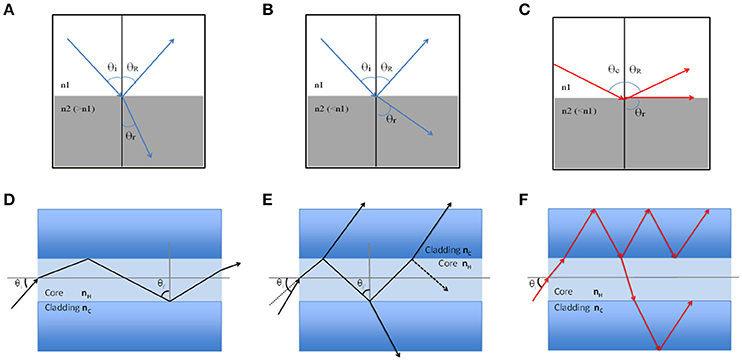
Figure 1. Schematic representation of light reflection and guiding. (A) Schematic light refraction between two different media with θi < θc and n2 > n1; (B) Light refraction with θi < θc and n2 < n1; (C) Total light reflection with θi ≥ θc that indicates the evanescent field; (D) Light in-coupling at one fiber end with θi > θc. It propagates without losses all along the fiber; (E) Light in-coupling with θi < θc. Part of the wave penetrates the cladding and can escape in the outer medium; (F) Cladding modes, the wave penetrates and propagates in the cladding.
Now for an optical fiber which consists of a core presenting an nH index slightly higher than nc the cladding index, when a light wave penetrates into the core at an angle >θc it propagates from one end to the other without any loss (Figure 1D). If the incidence angle at the fiber inlet is too high, part of the beam is transmitted through the cladding at each reflection, and the wave is quickly damped (Figure 1E). As is often the case for fibers in the air or immersed, the index of the external environment is lower than that of the cladding, the light can penetrate and spread in the cladding (Figure 1F), so called “cladding modes.” Optical fibers are characterized by their numerical aperture (N.A) that define light cone acceptance of the fiber, where the critical angle is related to the indexes of the core and the cladding by:
If a ray of light penetrates the fiber by its cone, then it will be guided by internal total reflection, otherwise, it will not be guided.
Light in Photonic Structures
We shall also briefly recall the fundamental principles of photonics and show how it differs from wave optics. As the name suggests, photonics is the art of manipulating photons. We therefore use the corpuscular model of light, established by Einstein and Planck in the early twentieth century. In this model light is a flux of particles called photons characterized by their energy E, spin S, and wave vector k. The wave vector is related to the momentum that contains all information on the direction of propagation. Well-known relations relate these quantities to the different characteristics of the electromagnetic field in the wave model:
where ν is the frequency of the wave and λ its wavelength. The spin is connected to the circular polarization of the wave, but we will not use this quantity in these reminders.
In an interaction between light and matter, matter is characterized by its complex index
where n is the refractive index of the material. The imaginary part km will be considered as negligible in our purpose. In periodically structured materials also called “photonic crystals” (Johnson et al., 1999; Johnson and Joannopoulos, 2001) a proper way to deal with, is to consider the periodic material as a particle of a lattice constant “a” and a wave vector |Ka| = 2π/a. In the same way, a wave of spatial period λ can be represented as a particle (photon) of wave vector |k| = 2π/λ. The interaction between these two particles is then treated as an elastic shock satisfying the conservation principles of energy, momentum, and angular momentum. Quantum mechanics shows that momentum exchanges are quantified and occur with K multiples. An incident photon of wave vector k will emerge from the structure with a wave vector k′ such that:
In linear optics, k and k′ having the same modulus, this relation (5) allows an easy diffraction wave direction determination. Moreover, each time |k| is a multiple of |Ka| there is an exchange of momentum where light can no longer propagate in the structure and it is totally reflected. The consequence is the appearance of forbidden frequency bands whose width depends on the contrast of the index of the materials. This mechanical approach of the light-matter interaction allows the diffractive and interferential phenomenon explanation.
Light Propagation in Glass Sponge Materials
A huge variety of marine and freshwater sponges have been described, with an estimated diversity in the order of 9,000 species (Van Soest et al., 2012), and trace fossil record down to the lowest Cambrian (Chang et al., 2017). Among them the Demospongiae (> 7,000 species) and the Hexactinellida (~ 600 species) can present silica spicules of different structure and morphology, ranging from a few microns (microscleres), to millimeters and up to several meters long (megascleres).
Spicule structure investigations reported that silica spheres are arranged in microscopic concentric rings bind together by organic matrix that elaborate laminated spicules. However, the number and the thickness of the layers depend not only on the diameter of the spicule, but also on the species considered, and on the age of the sponge. In Euplectella sp. spicules are assembled into bundles and form macroscopic cylindrical square-lattice consolidated by diagonal ridges (Figures 2A,B). In this species the alternation between the central and the surrounding silica materials was show to present light collecting effects and to allow strong mechanical rigidity and stability (Aizenberg et al., 2004, 2005). Ptychographic nano-tomography methods were used for deeper investigation of the internal structure of anchoring spicule from Euplectella aspergillum (Birkbak et al., 2016). This technic revealed that the central filament is composed of organic (protein volume fraction ~70%) and highly mineralized part, which exhibits circular symmetry. But interestingly, the axial filament was shown to be slightly offset from the spicule's central axis (Birkbak et al., 2016). Recently, other analyses of the structure and composition of the spicules of Suberites domuncula and Tethya aurantium showed that axial filament of both species is a three-dimensional crystal lattice of six-fold symmetry, with silica nano-spheres embedded within the organic lattice. Their axial filament found to play a scaffolding role in the final morphology of the spicule due to its enhanced growth in [001] direction (Werner et al., 2017).
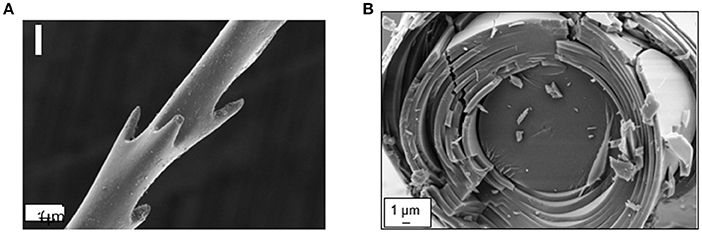
Figure 2. (A) Cross-section Scanning Electron Microscopy image of Euplectella aspergilium spicule. (B) Zoomed SEM image on spicule's edge showing part of the core region and the concentric silica layers. The multilayer set (silica + organic) surrounding the core of the spicule, forms a homogeneous medium of an effective refractive index different from spicule core refractive index.
Studies on the needle-shaped structures called strongyloxea spicules (axisymmetric silica rods) of Tethya aurantia have shown that spicule's tapering structures enhanced the buckling resistance compared to the same cylindrical structure (Monn and Kesari, 2017). Strongyloxea spicules have a strong Young's modulus of 72 GPa, which might be explained by the gathering of spicules into bundles to increase mechanical properties. It was also proposed that structure-property connection is also related to buckling resistance, and spicule's tapering structures can be considered as an optimal form for this purpose (Monn et al., 2015; Monn and Kesari, 2017). Overall, spicules have been shown to present remarkable mechanical properties in different axes as buckling resistance and toughness enhancement.
Due to their resemblances to manmade fiber optics, spicules are interesting materials to investigate their optical properties, and to our knowledge the first investigations of optical properties of biosilica were performed with purified frustules (Gaino and Sara, 1994; Cattaneo-Vietti et al., 1996). Optical properties of Hyalonema sieboldi spicule have been studied by Nd:YAG laser pulses by second harmonic pulses method. It shows by laser irradiation that the fluorescence intensity of spicules increases in the long wavelength range and show a maximum at 770 nm. Saturation and large fluorescence lifetimes were also reported (Kul'chin et al., 2009). Recently, a study by femtosecond (fs)-pulsed laser method, demonstrated the optical properties of Sericolophus hawaiicus spicules. Such spicules were assumed to act as a natural supercontinuum generator that involves wavelengths between 650 and 900 nm and a maximum spectral propagation at λ = 750 nm. This optical property was assumed to be due to its bio-composite nature combining mineral biosilica and organic matrix, including chitin (Ehrlich et al., 2016).
Alternatively, fabrication of an artificial spicules by bio-mimicry have been reported along with their waveguiding ability (Polini et al., 2012). It's believed that spicules made by natural glass sponges or by biomimetic approaches have a great potential for applications in optics and might “replace” industrial fiber optics due to their advantages of low cost and low temperature fabrication (for reviews see Müller et al., 2006, 2009).
Optical Properties of Diatoms Thecae
Diatoms are unicellular eukaryotic microalgae. They are represented in nature with a large variety of species and shapes, circular, pennate, cylindrical, and star shape. Since longtime, diatoms attracted the attention of scientists to address their important ecological roles (i.e., in carbon and silicon biogeochemical cycles Armbrust, 2009) and to describe their Si-biomineralization process (Hildebrand, 2008; Kroger, 2008; Ehrlich and Witkowski, 2015; Hildebrand and Lerch, 2015). Their fascinating silica shell architecture called frustule has evolved along millions of years to ensure the vitality of the cell by protecting it from predators and infections, ensuring metabolic exchange, maintaining the cellular integrity, and eventually interacting with incident light. Frustules present pillbox like shapes comprising two valves (epivalve and hypovalve) connected by porous silica rings named the girdle bands. Periodicity of the porous structure, symmetry, and the number of porous layers is species dependent.
Frustules: Interest for Optical Studies
Among the entire diatom studied, material scientists have focused on Coscinodiscus species, probably because of their relatively large cell size in the range of 100–200 μm width. Coscinodiscus present a highly structured frustule not only in two dimensions but also in 3-D (Sumper, 2002; Sar et al., 2008; Romann et al., 2015a,b; Aitken et al., 2016; Xing et al., 2017). The valve consists of three overlapping silica layers; the external side named cribrum, it includes smaller porous silica connections called cribellum and finally a third internal layer named foramen. The cribrum is connected to the foramen by silica walls named areola (Figure 3). The areola shows a honeycomb-like structure whereas foramen displays a hexagonal array of circular pores. More, foramen holes are aligned at the same time with the hexagonal pattern of areola and cribrum pores. It is also believed that the formation of these patterns is the result of a self-organized phase separation process (Sumper, 2002). There is no doubt that such porous biosilica nano-structured patterns have been found to be very interesting for optics and photonics investigations.
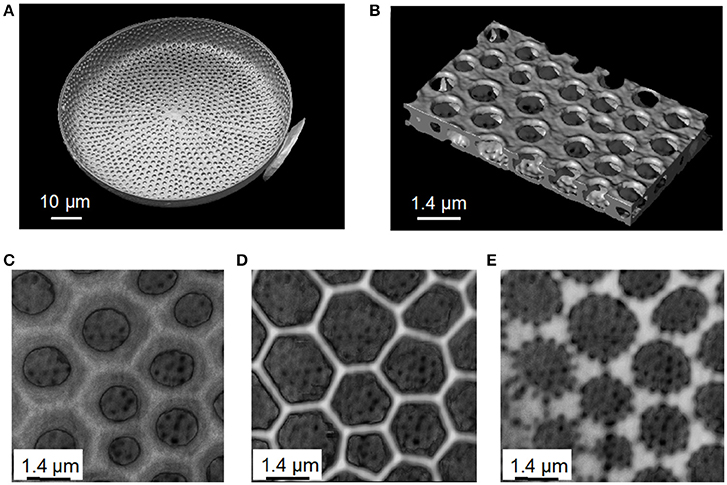
Figure 3. Images of Coscinodiscus sp. diatom valve from X-Ray tomography data obtained at the European Synchrotron Radiation Facility (ESRF). (A) A complete valve view from the cellular face. On the right we see part of the needle used as a support to handle and rotate the valve face to the X-Ray beam. (B) 3D reconstruction image of the structure of the valve, where the up-side, foramen, is in the interior side of the cell. Foramen represents a periodic pores layer leading to a hexagonal network. (C) The areola layer connecting the Foramen (big holes inside the valve) to the cribrum. (D) The cribrum layer of the valve. (E) The cribellum layer.
From an optical point of view two features are important to consider: the physical dimension of the periodic structure (number of porous silica layers, thickness, and holes diameters) and the refractive index contrast made by the complementarity of silica (refractive index n ≈ 1.45) and air pores (n = 1) in the visible range. Indeed, most studies have been performed from purified materials and in air conditions, in order to ensure a relatively good refractive index contrast needed to enable and quantify some optical properties (i.e., light diffraction through silica/air patterns). The scale of holes, of specific layers should be in the order of light wavelengths, which then allow interaction with incoming wavelengths whether diffracting, scattering or guiding them. In addition, some diatom valves, such as the one from Coscinodiscus, present a periodic pattern of holes in one directional slab (alternation of slica/air holes) resembling to a slab waveguide photonic crystal. Periodicity and porosity in the structure enable light-frustule interaction and can be regarded as particle (photon)-particle (matter) interaction as described in the theoretical part before, so then incoming wavelengths can be diffracted, scattered, or guided.
Light Focusing and Concentration
The frustule of the diatom can be considered as a nano-structured biosilica shell that can act as a photonic crystal because of its hierarchical structure, which enable to envision several applications as for example micro-lenses, optical filters, and waveguides. Table 1 summarizes most of recent studies reported in literature on the optical properties of diatoms frustules.
Light concentration and focusing ability of centric frustules was first studied for Coscinodiscus wailesii by illuminating a single valve by a red laser beam. It was demonstrated that the initial beam diameter of 100 μm was reduced about 12 times (~ 10 μm diameter) at a distance around 104 μm from the valve position (De Stefano et al., 2007). This lens behavior was attributed to a coherent superposition of the transmitted unfocused wave fronts through the quasi-regular areolae of the valve. In 2010, it was further suggested that the light confinement could be attributed to the regular pore pattern and the superposition of diffracted wave fronts (De Tommasi et al., 2010). However, it was also shown for C. wailesii valve that the focusing distance from the reference's valve position (named z*) is wavelength dependent, where by illuminating with laser beam of 532, 557, 582, and 633 nm wavelengths result in the focused distances at 130, 115, 110, and 105 μm, respectively (De Tommasi et al., 2010). Furthermore, similar focusing behavior was shown for the centric single valve of Arachnoidiscus sp. where a 633 nm laser beam was confined at 163 μm from the valve (Ferrara et al., 2014).
These results suggest that light propagation through the valve doesn't only depend on the interaction with the silica itself but likely on the spatial distribution of the pores in the periodic structure, therefore centric valve can be considered as multifocal lens. Multifocal lens and waveguide ability by confocal hyper-spectral imaging was investigated for two centric diatoms Cosinodiscus centralis and C. wailesii, it was shown, at several wavelengths (485, 535, 625, and 675 nm), that multiple light cones were transmitted through the centric valve (Romann et al., 2015a,b). These authors also shown that light convergence depends on the valve orientation, where the intensity of transmitted light decreases strongly when the incident light illuminate the internal side of the valve (i.e., the foramen side), whereas the light intensity increases upon illumination of external side (i.e., the cribrum side). Furthermore, tilting the incident light of 10° doesn't change the concentration behavior (Romann et al., 2015a,b). All of these reported strengthen the light focusing ability of valves from centric species.
A recent study by Chen et al. highlighted the light trapping effect of Cosinodiscus sp. species in photovoltaic application (Chen et al., 2015). For this purpose, Coscinodiscus sp. valves were deposited by floating assembly method on a 50 nm layer of low band-gap polymer PTB7:PC71BM photovoltaic active material. Absorption spectra of polymer coated diatoms sample showed a broadband enhancement (between 400 and 700 nm) estimated to be about 28% comparing to diatoms free sample. Moreover, in the same study, RCWA and FDTD simulation methods were applied to evaluate transmitted light behavior over the active layer. Results suggested that placing diatoms internal plate faced to the top of the PV active layer enhances the absorption efficiency by a factor of 1.41 from 380 to 800 nm. In addition, it was shown that this enhancement effect is the combination of the enhancement contribution by each separated layer in the valve's structure (Chen et al., 2015).
The light focusing, guiding, and trapping ability of frustules described above highlight the photonic crystal behavior of silica shells. T. Fuhrmann et al. were the first to perform band diagram simulations for valve and girdle bands of a centric species Cosinodiscus granii (Fuhrmann et al., 2004). Generated crystal photonics modes depend on the periodicity of the structure (lattice constant of each silica grid) the slab thickness and the incident wavelength. For C. granii valve and girdle bands influence incident light by coupling it into waveguides with distinct photonic crystal modes, for example red light was shown to be coupled in the valve but not in the girdle. It is also believed that to be able to exhibit a complete 2-D photonic crystal band-gaps, a refractive index contrast in the material of about 2 is needed (Knight, 2003). Kieu et al. reported the optical properties of a C. wailesii valve fixed on the top of an optical fiber by the external valve's side, by irradiating with coherent supercontinuum broadband laser (400–1,700 nm) a region of ~20 μm (Kieu et al., 2014). Then they studied the diffracted and transmitted light through the valve as a function of the lattice constant in the periodic structure. When irradiated by wavelengths at different angles, the hexagonal pattern generated colorful transmitted patterns. As a consequence, a white spotlight was observed on the screen without the valve in the setup, while changing the irradiated spot area on the valve changed its color. In addition, it was shown that the hexagonal colorful patterns resulted from the interaction with the periodic structure (Kieu et al., 2014).
All the above-mentioned studies were performed with frustules from centric diatoms, therefore with radial symmetry, so what about cylindrical frustules? Recently, the photonic crystalline characteristics of the cylindrical frustule of the diatom Melosira variance were reported (Yoneda et al., 2016). Clean frustules were studied with a microfiber spectroscopic method. The frustule was put on a micromanipulator and illuminated by a 10 μm sophisticated fiber optic end connected to a 300 W Xe lamp (300–1,100 nm) and transmitted light was collected and analyzed with a spectrometer. This method showed a fine space resolution due to the deployed micro-scale, which make it suitable to study the photonic crystallinity. When a frustule was irradiated through its internal side a transmittance valley was observed between 400 and 500 nm, while when it was irradiated perpendicular to the silica side wall, no particular valleys was observed. The authors concluded that transmitted spectra observed were related to the periodicity of the refractive index in the structure, and that the photonic crystalline characteristics assist light absorption but depending on the orientation (Yoneda et al., 2016).
Light Filtration
Frustules have also been investigated for their capability in protecting the cells against harmful wavelengths and excessive light intensities. In other word, acting as optical filters. It is known that diatoms use blue and red wavelengths for photosynthesis, so excess supply of blue light might generate active oxygen molecules that are dangerous for the living cell. It was proposed that the ordered porous silica shell might protect from dangerous UV and excessive wavelength intensities (Ellegaard et al., 2016).
Using Melosira varians frustules it was shown that the transmission of incident light was principally dependent in the wavelength, with enhanced transmission of red light and absorption in the blue range (Yamanaka et al., 2008). Such filtering behavior was also shown for circular valve from Arachnoidiscus sp. for which green and red wavelengths were shown to be more concentrated than blue light (Ferrara et al., 2014). In addition, in the case of UVB light it was shown that the light spot was very weak, and far away from the valve. These results were consistent with simulations (Ferrara et al., 2014).
Photoluminescence
A distinctive optical feature, photoluminescence (PL), was also observed with frustules. When porous biosilica was irradiated by UV wavelengths it emitted one or more PL peaks in the visible spectral region (Yoon and Goorsky, 1995; Cullis et al., 1997). Scientists investigated such features and their potential applications, for example in gas detection (Table 2). Photoluminescence responses of Thalassiosira rotula valves were examined when exposed to several gases and volatiles substances. Frustules irradiated by a He-Cd laser (325 nm) showed a multiband PL (between 450 and 690 nm) that was attributed to oxidized silicon nanocrystals (533 nm), porous silicon (609 nm), and hydrogenated amorphous silicon (661 nm). As a result, valves PL were quenched with electrophilic gases while they were enhanced for nucleophilic ones (De Stefano et al., 2005; Bismuto et al., 2008). Furthermore, PL of T. rotula frustules was quenched when exposed to NO2(g) flux, showing a high sensitivity in the order of sub-ppm level that it is strongly dependent on the structure, porosity, and gas nature (Bismuto et al., 2008). PL properties of the frustules from other centric and pennate species (T. rotula, Cosinodiscus. Wailesii, and Cocconeis scutellum) were also demonstrated, and high gas detection sensitivity was shown at room temperature (Setaro et al., 2007).
Photoluminescence was also investigated for chemically modified diatoms frustules. Townley et al. report a study on nickel sulfate modified C. wailesii frustules. When irradiated with a He-Cd laser (442 nm), intact frustules exhibit a broad PL peak (500–650 nm) while those growing with NiSO4 quenched the PL (Townley et al., 2007). Photoluminescence of chemically modified frustules have been investigated, for medical purposes, by fixing antibodies on the silica shells. Antibodies doped frustules were irradiated by 325 nm laser's wavelength, which show a high sensitivity in the order of 1.2 nm.μm−1 and detection limit around 100 nmol.L−1 (De Stefano et al., 2009). Recently, frustules from the pennate diatom Psammodictyon panduriforme were investigated under 325 nm laser excitation. Frustules showed two emission peaks at 417 and 534 nm that were considered as radiative luminescence generated by oxygen-vacancy defects in the structure (Camargo et al., 2016). However, under pulse laser excitation, a single narrow emission peak was observed near 475 nm. This result was interpreted as the consequence of a putative quantum confinement effect due to the mesoporous silica and the quasi-regular pores in frustules structure (Camargo et al., 2016).
Potential Applications of Biosilica
Biosilica materials from marine organisms, and in particular diatom frustules have been proposed and used in several industrial applications from filtration, insulation, fine abrasion, mineral fillers, catalysts, pesticides, building materials, food additives, anti-caking agent, carriers, or coating (Harwood, 2010), and have been proposed to develop new bio-chemical sensors or nanotechnologies. Some of the present and future applications have to be based on the optical properties of the frustule (Figure 4).
The advances in the understanding of the biomineralization processes, have inspired the development of new materials. The identified biomineralization enzymes from sponges (i.e., silicateins) and diatoms, were used for applications in biomedicine such as prototyping and 3D bio-printing of customized structures for biomedical purposes (Lopez et al., 2005; Schröder et al., 2016). Several applications in vision such as bacterial sensors (by immobilization of the biosilica-encapsulated bacterial cells on a sensor chip); development of core-shell nanomaterials for nanomedicine applications and 3D cell printing as promising materials for regenerative medicine. Modified biosilica from diatoms was also reported for biomedical applications as for example in drug delivery (Yang et al., 2011; Jo et al., 2016).
Frustules from diatoms have been investigated for their potential in biosensing, by exploiting their photoluminescence properties for gas detection. Frustules from Aulacoseira or Thalassiosira have been showed to be useful to detect trace of pure gas, as for example NO(g), H2(g), and NO2(g) (Bismuto et al., 2008; Leonardo et al., 2016). Since intact biosilica is still not efficient to distinguish substances in very complex mixtures, to increase sensitivity and specific substance identification, frustules were chemically modified on their silanol groups which could increase PL intensity and peak (Medarevic et al., 2016). Biosilica materials might have also great potential in the energy field. For example, in solar cells technologies, the ability of light trapping by diatoms valves could enhances light harvest (Chen et al., 2015), and for Dye Sensitized Solar Cells (DSSC) technology, TiO2 modified frustules (Jeffryes et al., 2011). In these cited examples, the solar cells efficiency was increased by the mediation of natural silica. In lithium batteries, biosilica materials might also found application as anode material because of their Li storage capability. Silicon nanostructures, obtained by reducing silica by magnesiothermic process, increase Li storage capacity in battery. For supercapacitors application, metal oxide modified biosilica seemed to enhance capacitance and cyclic stability (Sun et al., 2017). Natural silica is also under investigation for its potential as photoelectrodes for solar water electrolysis, production of hydrogen from water electrolysis, an important renewable source of energy. Biosilica is reduced to obtain porous silicon, a semiconductor necessary to enable water electrolysis reaction (Chandrasekaran et al., 2015).
Finally, biosilica was also explored for photonics applications due to their remarkable micro and nano-scalable 3D structures. The integration of luminescent dyes in the periodicity of the diatoms frustules is of high interest for scientists to elaborate new bio-hybrid luminescent materials that would be of great potential for application in photonics and laser technology. Organic dyes modified frustules had been reported in the literature. For example, the use of fluorescein-5-isothiocyanate and thiophene-benzothiadiazole-thiophene molecules, where shown to present interest in photonics and optoelectronics due to their important charge transport and high PL quantum yields in solid state (Vona et al., 2016). Furthermore, diatoms have been explored for their potential as nano-plasmonic sensors. In-situ growth of silver nanoparticles in frustule photonic crystal structures, show a label-free chemical and biological sensing based on surface enhanced Raman scattering, which can be of great interest for medical and food sensing applications (Kong et al., 2016).
Conclusion
We have focused here on light interaction in air condition mainly from purified biosilica materials, and have tried to emphases the importance of the structural organization of the sophisticated structured encountered in glass sponges and diatoms. Indeed, beside key roles in mechanical toughness and protection against predators, we question if is there any need for light modulation? This question arises because light-guiding process was demonstrated for biomaterials (i.e., glass-sponge spicules) extracted from marine organisms at depth where no light can reach. However, remarkable optical properties of sponge spicules were shown to compete manmade optical fibers in light guiding, and enable us to learn from nature and to envision soft processes to design new materials.
As mentioned above, most of the studies concerning the diatoms, have explored the optical properties of isolated frustules with hopefully almost no organic material remaining; even if the presence of trace contaminants has not been systematically analyzed. It remains that optical measurements have mostly failed to properly address the putative interaction of the incident light with the organic material. According to our knowledge such question remains open because on one side the presence of organic materials in the bio-silica, could eventually generate scattering effects, and therefore affect the propagation of light over long distances, but on the other side the low concentration of organic materials, might makes effects practically negligible. In addition, measurements have been generally performed in air and not in water. Valve orientation has also to be further considered as well as the complete structural organization. Indeed, in the environment the light hits the diatom cells from different directions.
Recent evidences suggested that the interaction between light and silica-based materials also applied in living organism (Ellegaard et al., 2016). Therefore, there is hope that in the future we might have more evidences on the role of the silica frustule to perform selective wavelength filtering, for example to collect specific photosynthetic wavelengths or to protect from dangerous once. Indeed, the putative roles of biomaterials in UV protections is a key question that should attract more and more attention for both marine (Xu et al., 2016) and terrestrial organisms (Schaller et al., 2013).
Author Contributions
All authors listed have made a substantial, direct, and intellectual contribution to the work, and approved it for publication.
Funding
This work was supported by French state funds managed by the ANR within the Investissements d'Avenir Programme (ANR-11-IDEX-0004-02), and more specifically within the framework of the Cluster of Excellence MATISSE led by Sorbonne Université. Works in PL laboratory is also supported by the Labex DRIIHM, French programme Investissements d'Avenir (ANR-11-LABX-0010).
Conflict of Interest Statement
The authors declare that the research was conducted in the absence of any commercial or financial relationships that could be construed as a potential conflict of interest.
Acknowledgments
The authors are grateful to Dr. Julie Villanova and the ESRF for beam time allocation. We also wish to thank Dr. Jean-Philippe Buffet, Dr. Alain Bulou, Landry Kamtcheu Ouanssi and Dr. Oxana Cherkas for their contribution to diatoms culture and some images acquisition.
References
Aitken, Z. H., Luo, S., Reynolds, S. N., Thaulow, C., and Greer, J. R. (2016). Microstructure provides insights into evolutionary design and resilience of Coscinodiscus sp frustule. Proc. Natl. Acad. Sci. U.S.A. 113, 2017–2022. doi: 10.1073/pnas.1519790113
Aizenberg, J., Sundar, V. C., Yablon, A. D., Weaver, J. C., and Chen, G. (2004). Biological glass fibers: correlation between optical and structural properties. Proc. Natl. Acad. Sci. U.S.A. 101, 3358–3363. doi: 10.1073/pnas.0307843101
Aizenberg, J., Weaver, J. C., Thanawala, M. S., Sundar, V. C., Morse, D. E., and Fratzl, P. (2005). Skeleton of Euplectella sp.: structural hierarchy from the nanoscale to the macroscale. Science 309, 275–278. doi: 10.1126/science.1112255
Armbrust, E. V. (2009). The life of diatoms in the world's oceans. Nature 459, 185–192. doi: 10.1038/nature08057
Birkbak, M. E., Guizar-Sicairos, M., Holler, M., and Birkedal, H. (2016). Internal structure of sponge glass fiber revealed by ptychographic nanotomography. J. Struct. Biol. 194, 124–128. doi: 10.1016/j.jsb.2016.02.006
Bismuto, A., Setaro, A., Maddalena, P., De Stefano, L., and De Stefano, M. (2008). Marine diatoms as optical chemical sensors: a time-resolved study. Sens. Actuat. B Chem. 130, 396–399. doi: 10.1016/j.snb.2007.09.012
Burns, E., and Ilan, M. (2003). Comparison of anti-predatory defenses of Red Sea and Caribbean sponges. II. Physical defense. Mar. Ecol. Prog. Ser. 252, 115–123. doi: 10.3354/meps252115
Camargo, E., Jaime, P. C. J., Lin, C. F., Lin, M. S., Yu, T. Y., Wu, M. C., et al. (2016). Chemical and optical characterization of Psammodictyon panduriforme (Gregory) Mann comb. nov (Bacillariophyta) frustules. Opt. Mat. Express 6, 1436–1443. doi: 10.1364/OME.6.001436
Cattaneo-Vietti, R., Bavestrello, G., Cerrano, C., Sara, M., Benatti, U., Giovine, M., et al. (1996). Optical fibres in an Antarctic sponge. Nature 383, 397–398. doi: 10.1038/383397b0
Chandrasekaran, S., Nann, T., and Voelcker, N. H. (2015). Nanostructured silicon photoelectrodes for solar water electrolysis. Nano Energy 17, 308–322. doi: 10.1016/j.nanoen.2015.08.022
Chang, S., Feng, Q. L., Clausen, S., and Zhang, L. (2017). Sponge spicules from the lower Cambrian in the Yanjiahe formation, South China: the earliest biomineralizing sponge record. Palaeogeogr. Palaeoclimatol. Palaeoecol. 474, 36–44. doi: 10.1016/j.palaeo.2016.06.032
Chen, X. F., Wang, C., Baker, E., and Sun, C. (2015). Numerical and experimental investigation of light trapping effect of nanostructured diatom frustules. Sci. Rep. 5:11977. doi: 10.1038/srep11977
Cooke, J., and Leishman, M. R. (2016). Consistent alleviation of abiotic stress with silicon addition: a meta-analysis. Funct. Ecol. 30, 1340–1357. doi: 10.1111/1365-2435.12713
Cullis, A. G., Canham, L. T., and Calcott, P. D. J. (1997). The structural and luminescence properties of porous silicon. J. Appl. Phys. 82, 909–965. doi: 10.1063/1.366536
De Stefano, L., Rea, I., Rendina, I., De Stefano, M., and Moretti, L. (2007). Lensless light focusing with the centric marine diatom Coscinodiscus walesii. Opt. Express 15, 18082–18088. doi: 10.1364/OE.15.018082
De Stefano, L., Rendina, I., De Stefano, M., Bismuto, A., and Maddalena, P. (2005). Marine diatoms as optical chemical sensors. Appl. Phys. Lett. 87, 396–399. doi: 10.1063/1.2140087
De Stefano, L., Rotiroti, L., De Stefano, M., Lamberti, A., Lettieri, S., Setaro, A., et al. (2009). Marine diatoms as optical biosensors. Biosens. Bioelectron. 24, 1580–1584. doi: 10.1016/j.bios.2008.08.016
De Tommasi, E., Rea, I., Mocella, V., Moretti, L., De Stefano, M., Rendina, I., et al. (2010). Multi-wavelength study of light transmitted through a single marine centric diatom. Opt. Express 18, 12203–12212. doi: 10.1364/OE.18.012203
Ehrlich, H., Demadis, K. D., Pokrovsky, O. S., and Koutsoukos, P. G. (2010a). Modern views on desilicification: biosilica and abiotic silica dissolution in natural and artificial environments. Chem. Rev. 110, 4656–4689. doi: 10.1021/cr900334y
Ehrlich, H., Deutzmann, R., Brunner, E., Cappellini, E., Koon, H., Solazzo, C., et al. (2010b). Mineralization of the metre-long biosilica structures of glass sponges is templated on hydroxylated collagen. Nat. Chem. 2, 1084–1088. doi: 10.1038/nchem.899
Ehrlich, H., Maldonado, M., Parker, A. R., Kulchin, Y. N., Schilling, J., Kohler, B., et al. (2016). Supercontinuum generation in naturally occurring glass sponges spicules. Adv. Opt. Mater. 4, 1608–1613. doi: 10.1002/adom.201600454
Ehrlich, H., and Witkowski, A. (2015). “Biomineralization in diatoms: the organic templates,” in Evolution of Lightweight Structures: Analyses and Technical Applications, Vol 6, ed C. Hamm (Dordrecht: Springer), 39–58.
Ellegaard, M., Lenau, T., Lundholm, N., Maibohm, C., Friis, S. M. M., Rottwitt, K., et al. (2016). The fascinating diatom frustule-can it play a role for attenuation of UV radiation? J. Appl. Phycol. 28, 3295–3306. doi: 10.1007/s10811-016-0893-5
Ferrara, M. A., Dardano, P., De Stefano, L., Rea, I., Coppola, G., Rendina, I., et al. (2014). Optical properties of diatom nanostructured biosilica in Arachnoidiscus sp: micro-optics from mother nature. PLoS ONE 9:e103750. doi: 10.1371/journal.pone.0103750
Fuhrmann, T., Landwehr, S., El Rharbi-Kucki, M., and Sumper, M. (2004). Diatoms as living photonic crystals. Appl. Phys. B 78, 257–260. doi: 10.1007/s00340-004-1419-4
Gaino, E., and Sara, M. (1994). Siliceous spicules of tethya-seychellensis (Porifera) support the growth of a green-alga - a possible light conducting system. Mar. Ecol. Prog. Ser. 108, 147–151. doi: 10.3354/meps108147
Hamm, C. E., Merkel, R., Springer, O., Jurkojc, P., Maier, C., Prechtel, K., et al. (2003). Architecture and material properties of diatom shells provide effective mechanical protection. Nature 421, 841–843. doi: 10.1038/nature01416
Harwood, D. M. (2010). “Diatomite,” in The Diatoms: Applications for the Environmentals and Earth Sciences, 2nd Edn., eds J. P. Smol and E. F. Stoermer (Cambridge: Cambridge University Press), 570–574.
Hildebrand, M. (2008). Diatoms, biomineralization processes, and genomics. Chem. Rev. 108, 4855–4874. doi: 10.1021/cr078253z
Hildebrand, M., and Lerch, S. J. (2015). Diatom silica biomineralization: parallel development of approaches and understanding. Semin. Cell Dev. Biol. 46, 27–35. doi: 10.1016/j.semcdb.2015.06.007
Jeffryes, C., Campbell, J., Li, H. Y., Jiao, J., and Rorrer, G. (2011). The potential of diatom nanobiotechnology for applications in solar cells, batteries, and electroluminescent devices. Energy Environ. Sci. 4, 3930–3941. doi: 10.1039/c0ee00306a
Jo, B. H., Kim, C. S., Jo, Y. K., Cheong, H., and Cha, H. J. (2016). Recent developments and applications of bioinspired silicification. Korean J. Chem. Eng. 33, 1125–1133. doi: 10.1007/s11814-016-0003-z
Johnson, S. G., Fan, S. H., Villeneuve, P. R., Joannopoulos, J. D., and Kolodziejski, L. A. (1999). Guided modes in photonic crystal slabs. Phys. Rev. B 60, 5751–5758. doi: 10.1103/PhysRevB.60.5751
Johnson, S. G., and Joannopoulos, J. D. (2001). Block-iterative frequency-domain methods for Maxwell's equations in a planewave basis. Opt. Express 8, 173–190. doi: 10.1364/OE.8.000173
Kieu, K., Li, C., Fang, Y., Cohoon, G., Herrera, O. D., Hildebrand, M., et al. (2014). Structure-based optical filtering by the silica microshell of the centric marine diatom Coscinodiscus wailesii. Opt. Express 22, 15992–15999. doi: 10.1364/OE.22.015992
Kong, X. M., Squire, K., Li, E. W., LeDuff, P., Rorrer, G. L., Tang, S. N., et al. (2016). Chemical and biological sensing using diatom photonic crystal biosilica with in-situ growth plasmonic nanoparticles. IEEE Trans. Nanobiosci. 15, 828–834. doi: 10.1109/TNB.2016.2636869
Kroger, N., and Poulsen, N. (2008). Diatoms–from cell wall biogenesis to nanotechnology. Annu. Rev. Genet. 42, 83–107. doi: 10.1146/annurev.genet.41.110306.130109
Kul'chin, Y. N., Voznesenski, S. S., Bukin, O. A., Bezverbnyi, A. V., Drozdov, A. L., Nagorny, I. G., et al. (2009). Spicules of glass sponges as a new type of self-organizing natural photonic crystal. Optics Spectrosc. 107, 442–447. doi: 10.1134/S0030400X09090227
Leonardo, S., Prieto-Simon, B., and Campas, M. (2016). Past, present and future of diatoms in biosensing. Trends Anal. Chem. 79, 276–285. doi: 10.1016/j.trac.2015.11.022
Liu, H., Chen, M., Zhu, F., and Harrison, P. J. (2016). Effect of diatom silica content on copepod grazing, growth and reproduction. Front. Mar. Sci. 3:89. doi: 10.3389/fmars.2016.00089
Liu, J., Zhu, J. W., Zhang, P. J., Han, L. W., Reynolds, O. L., Zeng, R. S., et al. (2017). Silicon supplementation alters the composition of herbivore induced plant volatiles and enhances attraction of parasitoids to infested rice plants. Front. Plant Sci. 8:1265. doi: 10.3389/fpls.2017.01265
Lopez, P. J., Desclés, J., Allen, A. E., and Bowler, C. (2005). Prospects in diatom research. Curr. Opin. Biotechnol. 16, 180–186. doi: 10.1016/j.copbio.2005.02.002
Luyckx, M., Hausman, J. F., Lutts, S., and Guerriero, G. (2017). Silicon and plants: current knowledge and technological perspectives. Front. Plant Sci. 8:411. doi: 10.3389/fpls.2017.00411
Medarevic, D. P., Losic, D., and Ibric, S. R. (2016). Diatoms - nature materials with great potential for bioapplications. Hemijska Industrija 70, 613–627. doi: 10.2298/HEMIND150708069M
Monn, M. A., and Kesari, H. (2017). A new structure-property connection in the skeletal elements of the marine sponge Tethya aurantia that guards against buckling instability. Sci. Rep. 7:39547. doi: 10.1038/srep39547
Monn, M. A., Weaver, J. C., Zhang, T. Y., Aizenberg, J., and Kesari, H. (2015). New functional insights into the internal architecture of the laminated anchor spicules of Euplectella aspergillum. Proc. Natl. Acad. Sci. U.S.A. 112, 4976–4981. doi: 10.1073/pnas.1415502112
Müller, W. E. G., Wang, X. H., Cui, F. Z., Jochum, K. P., Tremel, W., Bill, J., et al. (2009). Sponge spicules as blueprints for the biofabrication of inorganic-organic composites and biomaterials. Appl. Microbiol. Biotechnol. 83, 397–413. doi: 10.1007/s00253-009-2014-8
Müller, W. E., Wendt, K., Geppert, C., Wiens, M., Reiber, A., and Schroder, H. C. (2006). Novel photoreception system in sponges? Unique transmission properties of the stalk spicules from the hexactinellid Hyalonemasieboldi. Biosens. Bioelectron. 21, 1149–1155. doi: 10.1016/j.bios.2005.04.017
Pierantoni, M., Tenne, R., Brumfeld, V., Kiss, V., Oron, D., Addadi, L., et al. (2017). Plants and light manipulation: the integrated mineral system in okra leaves. Adv. Sci. 4:1600416. doi: 10.1002/advs.201600416
Polini, A., Pagliara, S., Camposeo, A., Cingolani, R., Wang, X. H., Schroder, H. C., et al. (2012). Optical properties of in-vitro biomineralised silica. Sci. Rep. 2:607. doi: 10.1038/srep00607
Pondaven, P., Gallinari, M., Chollet, S., Bucciarelli, E., Sarthou, G., Schultes, S., et al. (2007). Grazing-induced changes in cell wall silicification in a marine diatom. Protist 158, 21–28. doi: 10.1016/j.protis.2006.09.002
Raven, J. A., and Giordano, M. (2009). Biomineralization by photosynthetic organisms: evidence of coevolution of the organisms and their environment? Geobiology 7, 140–154. doi: 10.1111/j.1472-4669.2008.00181.x
Raven, J. A., and Waite, A. M. (2004). The evolution of silicification in diatoms: inescapable sinking and sinking as escape? New Phytol. 162, 45–61. doi: 10.1111/j.1469-8137.2004.01022.x
Rohde, S., and Schupp, P. J. (2011). Allocation of chemical and structural defenses in the sponge Melophlus sarasinorum. J. Exp. Mar. Biol. Ecol. 399, 76–83. doi: 10.1016/j.jembe.2011.01.012
Romann, J., Valmalette, J. C., Chauton, M. S., Tranell, G., Einarsrud, M. A., and Vadstein, O. (2015a). Wavelength and orientation dependent capture of light by diatom frustule nanostructures. Sci. Rep. 5:17403. doi: 10.1038/srep17403
Romann, J., Valmalette, J. C., Røyset, A., and Einarsrud, M. A. (2015b). Optical properties of single diatom frustules revealed by confocal microspectroscopy. Opt. Lett. 40, 740–743. doi: 10.1364/OL.40.000740
Sar, E. A., Sunesen, I., and Hinz, F. (2008). Fine morphology of coscinodiscus jonesianus and coscinodiscus commutatus and their transfer to coscinodiscopsis gen. Nov. Diatom Res. 23, 401–421. doi: 10.1080/0269249X.2008.9705766
Schaller, J., Brackhage, C., Bäucker, E., and Dudel, E. G. (2013). UV-screening of grasses by plant silica layer? J. Biosci. 38, 413–416. doi: 10.1007/s12038-013-9303-1
Schröder, H. C., Grebenjuk, V. A., Wang, X. H., and Muller, W. E. G. (2016). Hierarchical architecture of sponge spicules: biocatalytic and structure-directing activity of silicatein proteins as model for bioinspired applications. Bioinspir. Biomim. 11:041002. doi: 10.1088/1748-3190/11/4/041002
Schultes, S., Lambert, C., Pondaven, P., Corvaisier, R., Jansen, S., and Ragueneau, O. (2010). Recycling and uptake of Si(OH)4 when protozoan grazers feed on diatoms. Protist 161, 288–303. doi: 10.1016/j.protis.2009.10.006
Setaro, A., Lettieri, S., Maddalena, P., and De Stefano, L. (2007). Highly sensitive optochemical gas detection by luminescent marine diatoms. Appl. Phys. Lett. 91:051921. doi: 10.1063/1.2768027
Stramski, D., Sciandra, A., and Claustre, H. (2002). Effects of temperature, nitrogen, and light limitation on the optical properties of the marine diatom Thalassiosira pseudonana. Limnol. Oceanogr. 47, 392–403. doi: 10.4319/lo.2002.47.2.0392
Sumper, M. (2002). A phase separation model for the nanopatterning of diatom biosilica. Science 295, 2430–2433. doi: 10.1126/science.1070026
Sun, X. W., Zhang, Y. X., and Losic, D. (2017). Diatom silica, an emerging biomaterial for energy conversion and storage. J. Mater. Chem. A 5, 8847–8859. doi: 10.1039/C7TA02045G
Townley, H. E., Woon, K. L., Payne, F. P., White-Cooper, H., and Parker, A. R. (2007). Modification of the physical and optical properties of the frustule of the diatom Coscinodiscus wailesii by nickel sulfate. Nanotechnology 18:295101. doi: 10.1088/0957-4484/18/29/295101
Van Soest, R. W. M., Boury-Esnault, N., Vacelet, J., Dohrmann, M., Erpenbeck, D., De Voogd, N. J., et al. (2012). Global diversity of sponges (Porifera). PLoS ONE 7:e35105. doi: 10.1371/journal.pone.0035105
Vona, D., Lo Presti, M., Cicco, S. R., Palumbo, F., Ragni, R., and Farinola, G. M. (2016). Light emitting silica nanostructures by surface functionalization of diatom algae shells with a triethoxysilane-functionalized pi-conjugated fluorophore. Mrs Adv. 1, 3817–3823. doi: 10.1557/adv.2015.21
Wang, M., Gao, L. M., Dong, S. Y., Sun, Y. M., Shen, Q. R., and Guo, S. W. (2017). Role of silicon on plant-pathogen interactions. Front. Plant Sci. 8:701. doi: 10.3389/fpls.2017.00701
Werner, P., Blumtritt, H., and Natalio, F. (2017). Organic crystal lattices in the axial filament of silica spicules of Demospongiae. J. Struct. Biol. 198, 186–195. doi: 10.1016/j.jsb.2017.03.005
Xing, Y., Yu, L. H., Wang, X. L., Jia, J. Q., Liu, Y. Y., He, J. Y., et al. (2017). Characterization and analysis of Coscinodiscus genus frustule based on FIB-SEM. Prog. Nat. Sci. Mater. Int. 27, 391–395. doi: 10.1016/j.pnsc.2017.04.019
Xu, J. T., Bach, L. T., Schulz, K. G., Zhao, W. Y., Gao, K. S., and Riebesell, U. (2016). The role of coccoliths in protecting Emiliania huxleyi against stressful light and UV radiation. Biogeosciences 13, 4637–4643. doi: 10.5194/bg-13-4637-2016
Yamanaka, S., Yano, R., Usami, H., Hayashida, N., Ohguchi, M., Takeda, H., et al. (2008). Optical properties of diatom silica frustule with special reference to blue light. J. Appl. Phys. 103:074701. doi: 10.1063/1.2903342
Yang, W. R., Lopez, P. J., and Rosengarten, G. (2011). Diatoms: self assembled silica nanostructures, and templates for bio/chemical sensors and biomimetic membranes. Analyst 136, 42–53. doi: 10.1039/C0AN00602E
Yoneda, S., Ito, F., Yamanaka, S., and Usami, H. (2016). Optical properties of nanoporous silica frustules of a diatom determined using a 10 μm microfiber probe. Jpn. J. Appl. Phys. 55:072001. doi: 10.7567/JJAP.55.072001
Yoon, H., and Goorsky, M. S. (1995). “Systematic study of the structural and luminescence properties of P-type porous silicon,” in Materials Research Society Symposium - Proceedings, Vol. 378, 893–898.
Keywords: diatoms, sponges, photonics materials, light-silica interaction, biosilica
Citation: Mcheik A, Cassaignon S, Livage J, Gibaud A, Berthier S and Lopez PJ (2018) Optical Properties of Nanostructured Silica Structures From Marine Organisms. Front. Mar. Sci. 5:123. doi: 10.3389/fmars.2018.00123
Received: 09 October 2017; Accepted: 22 March 2018;
Published: 10 April 2018.
Edited by:
Brivaela Moriceau, UMR6539 Laboratoire des Sciences de L'environnement Marin (LEMAR), FranceReviewed by:
Christian Maibohm, Laboratório Ibérico Internacional de Nanotecnologia (INL), PortugalHermann Ehrlich, Freiberg University of Mining and Technology, Germany
Copyright © 2018 Mcheik, Cassaignon, Livage, Gibaud, Berthier and Lopez. This is an open-access article distributed under the terms of the Creative Commons Attribution License (CC BY). The use, distribution or reproduction in other forums is permitted, provided the original author(s) and the copyright owner are credited and that the original publication in this journal is cited, in accordance with accepted academic practice. No use, distribution or reproduction is permitted which does not comply with these terms.
*Correspondence: Pascal J. Lopez, cGFzY2FsLWplYW4ubG9wZXpAbW5obi5mcg==