- 1Antarctic Climate and Ecosystems Cooperative Research Centre, University of Tasmania, Hobart, Australia
- 2Institute for Marine and Antarctic Studies, University of Tasmania, Hobart, Australia
The availability of iron controls primary productivity in large areas of the Southern Ocean. Iron is largely supplied via atmospheric dust deposition, melting ice, the weathering of shelf sediments, upwelling, sediment resuspension, mixing (deep water, biogenic, and vertical mixing) and hydrothermal vents with varying degrees of temporal and spatial importance. However, large areas of the Southern Ocean are remote from these sources, leading to regions of low primary productivity. Recent studies suggest that recycling of iron by animals in the surface layer could enhance primary productivity in the Southern Ocean. The aim of this review is to provide a quantitative and qualitative assessment of the current literature on pelagic iron recycling by marine animals in the Southern Ocean and highlight the next steps forward in quantifying the retention and recycling of iron by higher trophic levels in the Southern Ocean. Phytoplankton utilize the iron in seawater to meet their metabolic demand. Through grazing, pelagic herbivores transfer the iron in phytoplankton cells into their body tissues and organs. Herbivores can recycle iron through inefficient feeding behavior that release iron into the water before ingestion, and through the release of fecal pellets. The iron stored within herbivores is transferred to higher trophic levels when they are consumed. When predators consume iron beyond their metabolic demand it is either excreted or defecated. Waste products from pelagic vertebrates can thus contain high concentrations of iron which may be in a form that is available to phytoplankton. Bioavailability of fecal iron for phytoplankton growth is influenced by a combination of the size of the fecal particle, presence of organic ligands, the oxidation state of the iron, as well as biological (e.g., remineralization, coprochaly, coprorhexy, and coprophagy) and physical (e.g., dissolution, fragmentation) processes that lead to the degradation and release of fecal iron. The flux of dissolved iron from pelagic recycling is comparable to other sources in the region such as atmospheric dust, vertical diffusivity, vertical flux, lateral flux and upwelling, but lower than sea ice, icebergs, sediment resuspension, and deep winter mixing. The temporal and seasonal importance of these various factors requires further examination.
Introduction
The ocean is a major sink of anthropogenic carbon dioxide (CO2) which occurs through a combination of the physical and the biological carbon pumps. Cold surface seawaters enhance the solubility of atmospheric CO2 into the ocean. In the Southern Ocean, the cold seawater south of the Polar Front mixes with the warmer waters in the sub-Antarctic region and sink, transporting the CO2 to the deep ocean and thereby removing it from the atmosphere (Khatiwala et al., 2009, 2013). The fixation of CO2 into deep-sinking particulate organic carbon (POC), known as the biological pump, can globally remove an estimated 10 × 1012 kg of carbon from the pelagic zone each year (Falkowski et al., 1998; Buesseler and Boyd, 2009; Passow and Carlson, 2012).
Our understanding of the processes that regulate the solubility of CO2 in seawater is relatively well constrained. In contrast, the biological carbon pump is less well understood. This is because many of the mechanisms underpinning key process such as the retention, uptake, recycling, remineralization and export of nutrients such as iron (Fe) and carbon are spatially and temporally variable (Boyd et al., 2017). Primary production depends on the availability of light, CO2 and the presence of a wide range of nutrients such as: Fe and manganese (Mn) for carbon fixation, zinc (Zn), cadmium (Cd), and cobalt (Co) for CO2 acquisition, Zn and Cd for silica uptake by large diatoms' Co and Zn as calcifiers, Fe for nitrogen (N2) fixation, copper (Cu) and Fe for nitrification, denitrification and organic N utilization, Zn for organic phosphorus (P) utilization, Fe for synthesis of photopigments, and Cu for methane oxidation (Morel and Price, 2003; Morel et al., 2003). However, the scarcity of some micronutrients such as Fe can limit primary productivity across large areas of the ocean, thereby reducing the efficiency of the biological carbon pump (Martin, 1990; Martin et al., 1990; Bowie et al., 2001; Blain et al., 2007; Boyd et al., 2007, 2017). The aim of this review is to qualitatively and quantitatively assess the role of marine animals such as herbivores and their predators in recycling Fe in the Fe-limited Southern Ocean and propose key future steps in examining the role of higher trophic levels on the marine Fe and carbon cycle.
Sources of Iron to Southern Ocean Surface Waters
The predominant supply of Fe into the ocean has been considered to be from the deposition of atmospheric dust from the surrounding continents (Boyd et al., 2004; Jickells et al., 2005; Cassar et al., 2007). However, the importance of atmospheric Fe deposition is regionally variable. For example, atmospheric Fe blown from the Sahara Desert is a major source of Fe in the Atlantic Ocean and Mediterranean Sea (Guieu et al., 2002; Sarthou et al., 2003). In contrast, atmospheric dust is a less important source of Fe in the Southern Ocean, with an estimated dissolved Fe contribution of 0.00027–0.05 μmol m−2 day−1 (Table 1). This is because Antarctica is ice-covered, which greatly reduces access to sources of dust, and circumpolar winds and currents isolate much of the Southern Ocean from the other continents further north.
More than 40% of the Southern Ocean is seasonally covered by sea ice, which is a significant source of Fe during spring melt, stimulating much of the productivity observed from satellites. Sea ice forms as the seawater temperature drops below −1.86°C, whilst incorporating the Fe from seawater into the ice. The spring-time melting of sea ice (Sedwick and Di Tullio, 1997; Lannuzel et al., 2007), ice shelves (Herraiz-Borreguero et al., 2016), and icebergs (Smith et al., 2007; Lancelot et al., 2009; Lin et al., 2011; Duprat et al., 2016) release the trapped Fe into the surface waters. Other sources of Fe can include: hydrothermal vents (Tagliabue et al., 2010; Klunder et al., 2011), upwelling (de Baar et al., 1995; Watson, 2001), deep winter mixing (Tagliabue et al., 2014), biogenic mixing (Katija and Dabiri, 2009; Katija, 2012), vertical mixing (Webb and Suginohara, 2001; Cisewski et al., 2005; Frants et al., 2013), the weathering of shelf sediments (Sedwick et al., 2008; Bowie et al., 2009), sediment resuspension (Moore et al., 2004; Blain et al., 2007; Dulaiova et al., 2009) and pelagic recycling (Tovar-Sanchez et al., 2007; Ortega-Retuerta et al., 2009; Schmidt et al., 2011, 2016; Lehette et al., 2012; Ratnarajah et al., 2014, 2016a,b, 2017; Wing et al., 2014, 2017; Shatova et al., 2016; Laglera et al., 2017).
Using a combination of models and point-source observations, studies have attempted to estimate the flux of dissolved Fe from these various sources into the Southern Ocean (Table 1). These flux estimates are highly localized, do not represent a constant daily flux, and the calculated upper limits will only be realized close to their respective sources. Therefore, localized flux estimates are not easily extrapolated to basin scales. However, as a localized source, the flux of fecal derived dissolved Fe from baleen whales and Antarctic krill are comparable to other sources in the region such as atmospheric dust, vertical diffusivity, vertical flux, lateral flux and upwelling, but lower than sea ice, icebergs, sediment resuspension, and deep winter mixing (Table 1).
Overview of Pelagic Iron Recycling by Marine Animals
Uptake of Fe by phytoplankton occurs within the euphotic zone over spring and summer (Figure 1). Microzooplankton such as flagellates and ciliates graze on phytoplankton in the euphotic zone (Safi et al., 2007; Pearce et al., 2011; Yang E. J. et al., 2016), whilst invertebrate herbivores such as krill, salps, copepods actively graze on phytoplankton and microzooplankton (Schmidt et al., 2014; Dupuy et al., 2016; Schmidt and Atkinson, 2016; Yang G. et al., 2016). Some of the nutrients ingested by herbivores is retained to satisfy their metabolic demands (e.g., Cu for respiratory pigment in crustaceans; Spicer and Saborowski, 2010, ammonia/ammonium for neutral buoyancy in diapausing calanoid copepods; Sartoris et al., 2010). Herbivores recycle nutrients during feeding through inefficiencies in the feeding behavior (sloppy feeding) and via the excretion/defecation of excess nutrients.
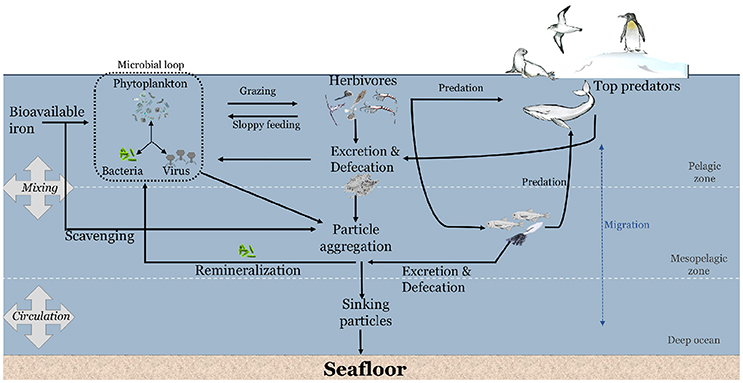
Figure 1. Conceptual diagram on the potential contribution of marine animals to the cycling of iron in the Southern Ocean.
The sloppy feeding during grazing by copepods and microzooplankton have been shown to release Fe and Fe-binding ligands into the water column (Sato et al., 2007; Sarthou et al., 2008; Laglera et al., 2017). This Fe might be available to primary producers (Figure 1). Excess nutrients beyond metabolic demand of zooplankton is ejected from their body by excretion or defecation (Tovar-Sanchez et al., 2007; Ortega-Retuerta et al., 2009; Ruiz-Halpern et al., 2011; Schmidt et al., 2011, 2016; Lehette et al., 2012; Ratnarajah et al., 2016b). For instance, Antarctic krill consume phytoplankton and process the ingested Fe within their digestive organs and muscle (Nicol et al., 2010; Schmidt et al., 2011, 2016; Ratnarajah et al., 2016b). Only two studies have measured the Fe concentrations in krill digestive organs, reporting a range from 13 to 2,783 mg kg−1 dry weight, with the variability likely driven by a combination of seasonal and regional differences in sampling, reflecting differences in the quantity and quality of their diet (Ratnarajah et al., 2016b; Schmidt et al., 2016).
A fraction of the Fe ingested by krill is retained in their muscle, with excess Fe being released in their fecal pellets (Nicol et al., 2010; Schmidt et al., 2011, 2016; Ratnarajah et al., 2016b). Additionally, excretion by Antarctic krill can release a portion of their ingested Fe. Experiments have suggested that krill excretion could increase total Fe concentrations in the seawater by 0.2–4.3 nM Fe L−1 d−1 (Tovar-Sanchez et al., 2007). Other Southern Ocean herbivores will have different effects on Fe cycling. For example, salp (Salpa thompsoni) fecal pellets appear to be retained in the upper 300 m of the water. Although these pellets contain high levels of Fe, they are highly refractory and do not release dissolved Fe via physicochemical pathways (Cabanes et al., 2017). Therefore, grazing by salps may lead to the depletion of Fe from surface seawater, with an estimated dissolved Fe export flux of 11.3 nmol Fe m−2 d−1 at 300 m (Cabanes et al., 2017). In addition, the large populations of pelagic herbivores in the Southern Ocean contain a significant amount of Fe in their bodies (Nicol et al., 2010). This Fe is unavailable to phytoplankton but can be released by excretion and defecation after the herbivores have been consumed by carnivores. Some herbivores are long-lived and can store Fe in their bodies between seasons and because they can swim, they retain this Fe in the pelagic zone.
The varying density and sinking rate of the fecal pellets of herbivores is a function of their size and shape and this results in species-specific differences in pellet retention in surface waters vs. export to deeper waters (Bruland and Silver, 1981; Turner, 2002, 2015). Although physicochemical processes may not degrade fecal pellets, biological processes such as coprorhexy (fragmentation of pellets), coprophagy (ingestion of pellets) and coprochaly (loosening of pellets) by other pelagic species, and bacterial degradation via the free-living in situ bacterial community or internal gut bacteria could remineralize the fecal pellets and may constitute an important component of nutrient recycling within the mixed layer (see reviews by Turner, 2002, 2015).
In addition, to their role in biological degradation processes, many herbivorous organisms undertake seasonal and diurnal vertical migration from the euphotic zone into deep waters which may redistribute nutrients. The deep ocean is richer in Fe than the surface waters (Bucciarelli et al., 2001; Boyd and Ellwood, 2010; Tagliabue et al., 2014). Such migratory movements have the potential to influence the transport of nutrients from the surface to the deep ocean, and vice versa (Smetacek and Nicol, 2005; Smetacek, 2008; Schmidt et al., 2011). For example, although most of the biomass of Antarctic krill is located within the upper 150 m of the water column (Demer and Hewitt, 1995; Lascara et al., 1999), Antarctic krill have been shown to actively feed at the seabed (Clarke and Tyler, 2008), thus influencing the vertical transfer of “new” Fe from the seafloor (Schmidt et al., 2011). However, vertically migrating herbivores would only influence the transfer of Fe if they ingest Fe at depth and release excretory products within the euphotic zone. Conversely, vertical migration by animals transfer carbon, Fe and other nutrients to the deep ocean and this may remain there if the animals are consumed or if the nutrients are released at depth (Schnetzer and Steinberg, 2002; Street and Payton, 2005). Vertical migration by animals can therefore either contribute to the enhancement or depletion of nutrient concentrations in the surface layer, depending on a range of temporal and spatial variables as well as the mix of species present.
The larger carnivores (e.g., seabirds, seals, whales etc.) consume the pelagic herbivores and some of the smaller carnivores, thereby transferring the nutrients from one trophic level onto the next. What happens next is dependent on the nature of the larger carnivore. Air breathing carnivores are linked to the surface so they release nutrients in the euphotic zone, or to their land-based colonies in the case of seals and seabirds. Some vertebrates and squid feed at great depth and can release their accumulated nutrients near the surface. This group of animals inexorably draws nutrients closer to the surface. Such recycling of excess nutrients that were ingested but not assimilated has been demonstrated in seabirds (Wing et al., 2014, 2017; Shatova et al., 2016), as well as land and pelagic dwelling marine mammals (Smetacek and Nicol, 2005; Nicol et al., 2010; Ratnarajah et al., 2014, 2017; Wing et al., 2014). Vertebrate fecal material has been linked to Fe enrichments within their local environment and this can stimulate phytoplankton growth (Shatova et al., 2016). Mesopelagic predators, such as squid and some fish species, on the other hand, spend most of their time at depth and only approach the euphotic zone sporadically. This group of animals may absorb their nutrients near the surface and draw them down to depth, however, no published studies have examined the relative contribution of mesopelagic predators to the cycling and/or export of Fe in the water column. Once again, the nature of the animal populations, the time of year and the location will all affect the magnitude and direction of nutrient cycling.
Iron Bioavailability
Not all Fe present in seawater is considered bioavailable. Bioavailability of Fe is influenced by the size and nature of the Fe particles, the complexation of certain forms of dissolved Fe by organic ligands, and by the oxidation state, with different phytoplankton classes able to access different chelated Fe species. The size fractionation between dissolved and particulate (operational cut-off of 0.2 or 0.4 μm) phases is the most commonly used as an approximation of element bioavailability, with dissolved Fe being considered as the most accessible form for biological uptake despite the particulate Fe fraction being the dominant pool of total Fe in the water column (de Baar and de Jong, 2001; Boyd and Ellwood, 2010; Lannuzel et al., 2011). The dissolved Fe pool can be further partitioned into two smaller fractions, soluble Fe (< 0.02 μm) and colloidal Fe (between 0.02 μm and 0.2 or 0.4 μm). The relative importance of these fractions is uncertain. Wu et al. (2001) demonstrated that the soluble fraction may be more bioavailable than the more chemically dynamic colloidal fraction, while Honeyman and Santschi (1989) demonstrated that the colloidal fraction could aggregate into larger particles and settle from the water column.
Fecal material released from marine animals span the dissolved to particulate size continuum (Figure 2). Some animals, such as copepods and krill, release fecal pellets bound within a peritrophic membrane, others defecate fecal material with a pulp-like consistency (e.g., seabird guano), or a liquid slurry at/close to the sea surface (e.g., whales) (Figure 2). Laboratory dissolution experiments of whale fecal material using natural seawater suggests that Fe continues to leach from the fecal particles over a 12 h time period (Ratnarajah et al., 2017). Despite the high Fe concentrations measured in krill (Schmidt et al., 2011, 2016; Ratnarajah et al., 2016b), seabird (Wing et al., 2014, 2017; Shatova et al., 2016) and whale (Nicol et al., 2010; Ratnarajah et al., 2014, 2017; Wing et al., 2014), fecal material bioavailability of the fecal Fe will depend on the size of the fecal particle, and/or the dissolution of fecal particles over time. In comparison, salp fecal pellets contain high concentrations of Fe, but the pellets are highly refractory (Cabanes et al., 2017). The peritrophic membrane may prevent the degradation of fecal pellets (Turner, 2002). Consequently, the consistency of fecal material will influence the potential bioavailability, where dissolved material may be favored over particulate material, however the dissolution of the particulate fraction in seawater, and microbial degradation may render this fraction bioavailable for uptake.
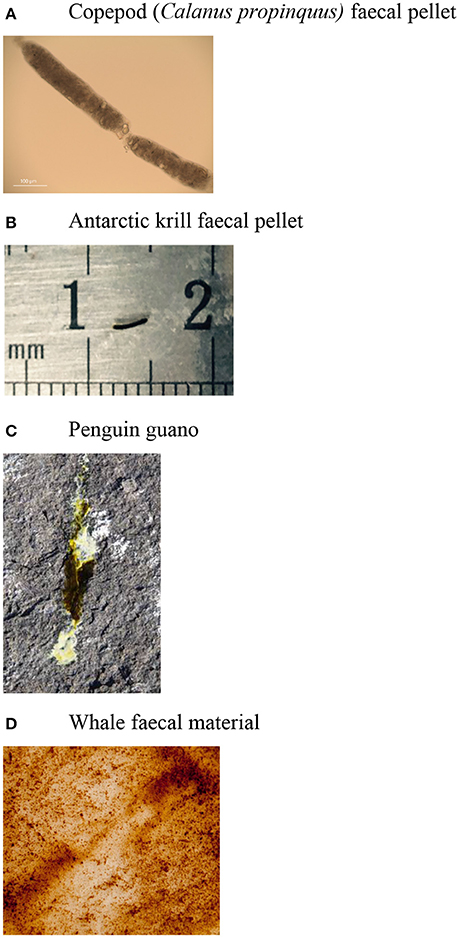
Figure 2. Images demonstrating the consistency of different types of fecal material: (A) copepod (Calanus propinquus) fecal pellet (credit: Jake Wallis), (B) Antarctic krill fecal pellet, (C) penguin guano (credit: Louise Emmerson), and (D) whale fecal material. No copyright permissions were required.
Complexation by some organic ligands also enhances the bioavailability of Fe for phytoplankton uptake (Gledhill and van den Berg, 1994; Boye et al., 2001, 2010; Hunter and Boyd, 2007; Hassler and Schoemann, 2009; Hassler et al., 2011a,b, 2012; Strzepek et al., 2011; Lannuzel et al., 2015). Organic ligands are molecules that can be excreted by the biota (e.g., siderophores are produced by bacteria as a response to Fe stress, Gledhill et al., 2004; Mawji et al., 2011), or during zooplankton grazing (Sato et al., 2007; Sarthou et al., 2008), that bind with trace metals forming stable complexes. More than 99% of the dissolved Fe in seawater is now known to be associated with strong organic ligands (Hassler et al., 2012). It remains unclear if marine animals can also release organic ligands in their fecal material.
Lastly, the the different oxidation states [Fe(II) or Fe(III)] influences the solubility of Fe. Fe(II) is considered to be the more bioavailable fraction because the thermodynamically stable Fe(III) redox species is less soluble (solubility of 0.07 nM in seawater of 4°C, pH 9 and salinity 36; Liu and Millero, 2002). In oxygen-rich waters, Fe(II) is rapidly oxidized into Fe(III) by oxygen and hydrogen peroxide. However, Fe(III) can be reduced to Fe(II) through photochemical reduction of colloidal Fe (Wells and Mayer, 1991; Rijkenberg et al., 2005) or reduction of organically bound Fe(III) (Barbeau, 2006; Rijkenberg et al., 2006). The reoxidation of Fe(II) can occur quite rapidly in seawater (t1/2 of 93.6 min at 4°C and pH 8; Croot and Laan, 2002). However, in the Southern Ocean, the cold temperatures (Millero et al., 1987), the presence of organic ligands (Gledhill and van den Berg, 1994; Boye et al., 2001; Maldonado et al., 2005; Barbeau, 2006; Hassler et al., 2012; Lannuzel et al., 2015), and generally low hydrogen peroxide concentrations (Sarthou et al., 1997) may slow the oxidation rates. At present, there is no information on the oxidation state of fecal Fe. The acidic digestion processes within the gut would favor the reduction of Fe(III) to Fe(II), however as the fecal material is expelled into an oxygenated environment, Fe(II) could return to an oxidized Fe(III). This is an area of further study.
Summary and Directions for Future Research
As discussed above, there is a suite of processes (e.g., grazing, predation, excretion/defecation, remineralization) involved in the transfer of Fe from herbivores to large carnivores, and the subsequent recycling and export of Fe (Figure 1). Pelagic herbivores appear to have two opposing effects on oceanic primary productivity. On the one hand, the high biomass of herbivores in marine ecosystems (Pakhomov et al., 2000; Atkinson et al., 2009), high grazing pressure on primary production (Perissinotto, 1997; Walsh et al., 2001; Smetacek et al., 2004; Sato et al., 2007), rapid gut passage time (Schnetzer and Steinberg, 2002; Tirelli, 2005; Schmidt and Atkinson, 2016), coupled with their fast sinking fecal pellets (Turner, 2015), suggests that herbivores play an important role in the export of Fe to below the mixed layer. On the other hand, the storage and release of Fe, and other essential nutrients, within the mixed layer (Tovar-Sanchez et al., 2007; Ortega-Retuerta et al., 2009) or through vertical migration (Schmidt et al., 2011) increases microbial productivity (Arístegui et al., 2014), and contributes substantially to Fe recycling (Maldonado et al., 2016). These different findings are likely due to the condition of the peritrophic membrane in zooplankton fecal pellets that acts as a protective mechanism, impeding the release of nutrients. Biological processes such as microbial degradation (Morata and Seuthe, 2014), and the loosening, fragmentation and ingestion of fecal pellets by other detritiverous animals (coprochaly, coprorhexy and coprophagy respectively) (Noji et al., 1991) can disrupt the membrane and release the incorporated nutrients into the water column.
The fate of any element ingested by herbivores is determined by the balance of predation behavior (i.e., surface feeding and defecating, vs. surface feeding but defecation at depth). As homeostatic regulation releases elements which are in excess to requirements, the transfer of Fe between successive trophic levels will differ from species to species based on their elemental requirements. Although we have focussed on Fe in this review, marine animals also recycle other nutrients through their diet and subsequent defecation such as labile dissolved organic carbon (DOC) which stimulates the DOC-limited heterotrophic bacterial community (Ruiz-Halpern et al., 2011; Arístegui et al., 2014), macronutrients such as ammonium (Atkinson and Whitehouse, 2000; Tovar-Sanchez et al., 2009; Whitehouse et al., 2011; Lehette et al., 2012), and micronutrients such as Cd, Co, Cu, Zn, and Mn (Tovar-Sanchez et al., 2009; Ratnarajah et al., 2014), which might be important for marine primary production. In addition, some large predators defecate solely in the water column (e.g., whales), whilst others may defecate both in the water during periods of intensive feeding as well as on land and ice (e.g., seals, penguins etc.). The material defecated on land and ice could wash into the seawater during periods of sea ice melt and through strong winds that fractionate and disperse fecal material into the ocean.
A growing body of literature suggests that biological recycling by multi-cellular animals is clearly important to primary production, trophodynamics, and phenology. However, the main contributors remain to be distinguished and quantified. To date, multicellular animals have been largely implicated in downward nutrient transfer but there is increasing evidence that such animals can also play a role in retaining nutrients in the surface layer, returning nutrients to the surface waters and exporting nutrients to different areas of the ocean. Biological recycling influences the biological carbon pump, but there are some important questions that need to be answered to determine the degree to which it does.
The consistency of fecal material, and the length of time that the fecal particles remain in the mixed layer will influence its bioavailability for phytoplankton growth. Critical research will require: determining the fraction of dissolved to particulate Fe in fecal material, looking for the presence of organic ligands released through grazing practices or defecation, examining the response of organic ligands in seawater to fecal derived Fe, and to the oxidation state of fecal material. It is also essential to determine the timeframe that fecal pellets or particles remain in the mixed layer before being exported, and what are the biological (e.g., remineralization, coprochaly, coprorhexy, and coprophagy) and physical (e.g., dissolution, fragmentation) processes involved in the degradation and release of fecal Fe during its transit. Answers to these questions will bring us closer to understanding if, and how, Fe is retained in the surface layer by marine animals. Ultimately, it will also be essential to quantify how much of the carbon taken in by animals is respired back into the atmosphere.
Author Contributions
LR, SN, and AB: equally contributed to the analysis, interpretation, and drafting of the manuscript.
Conflict of Interest Statement
The authors declare that the research was conducted in the absence of any commercial or financial relationships that could be construed as a potential conflict of interest.
Acknowledgments
We would like to thank Prof. Tom Trull, and Dr. Jessica Melbourne-Thomas for their comments and suggestions during the development of this manuscript. This work was supported by the Australian Government's Cooperative Research Centres Programme through the Antarctic Climate and Ecosystem Cooperative Research Centre, the Institute for Marine and Antarctic Studies, the Holsworth Wildlife Research Endowment and the Scientific Committee on Antarctic Research (SCAR) Fellowship.
References
Arístegui, J., Duarte, C. M., Reche, I., and Gómes-Pinchetti, J. L. (2014). Krill excretion boosts microbial activity in the Southern Ocean. PLoS ONE 9:e89391. doi: 10.1371/journal.pone.0089391
Atkinson, A., and Whitehouse, M. J. (2000). Ammonium excretion by antarctic krill euphausia superba at South Georgia. Limnol. Oceanogr. 45, 55–63. doi: 10.4319/lo.2000.45.1.0055
Atkinson, A., Siegel, V., Pakhomov, E. A., Jessopp, M. J., and Loeb, V. (2009). A re-appraisal of the total biomass and annual production of Antarctic krill. Deep Sea Res. I 56, 727–740. doi: 10.1016/j.dsr.2008.12.007
Barbeau, K. (2006). Photochemistry of organic iron(III) complexing ligands in oceanic systems. Photochem. Photobiol. 82, 1505–1516. doi: 10.1562/2006-06-16-IR-935
Blain, S., Queguiner, B., Armand, L., Belviso, S., Bombled, B., Wagener, L., et al. (2007). Effect of natural iron fertilization on carbon sequestration in the Southern Ocean. Nature 446, 1070–1074. doi: 10.1038/nature05700
Bowie, A. R., Lannuzel, D., Remenyi, T., Wagener, T., Lam, P. J., Trull, P. W., et al. (2009). Biogeochemical iron budgets of the Southern Ocean south of Australia: decoupling of iron and nutrient cycles in the subantarctic zone by the summertime supply. Global Biogeochem. Cycles 23, 1–14. doi: 10.1029/2009GB003500
Bowie, A. R., Maldonado, M. T., Frew, R., Croot, P. L., Achterberg, E. P., Boyd, R., et al. (2001). The fate of added iron during a mesoscale fertilisation experiment in the Southern Ocean. Deep Sea Res. II 48, 2703–2743. doi: 10.1016/S0967-0645(01)00015-7
Bowie, A. R., van der Merwe, P., Quéroué, F., Trull, T., Fourquez, M., Planchon, F., et al. (2015). Iron budgets for three distinct biogeochemical sites around the Kerguelen archipelago (Southern Ocean) during the natural fertilisation study, KEOPS-2. Biogeoscience 12, 4421–4445. doi: 10.5194/bg-12-4421-2015
Boyd, P. W., Law, C. S., Hutchins, D. A., Abraham, E. R., Croot, P., Ellwood, M., et al. (2005). FeCycle: attempting an iron biogeochemical budget from a mesosclate SF6 tracer experiment in an unperturbed low iron waters. Global Biogeochem. Cycles 19:GB4S20. doi: 10.1029/2005GB002494
Boyd, P. W., McTainsh, G., Sherlock, V., Richardson, K., Nichol, S., Ellwood, M., et al. (2004). Episodic enhancement of phytoplankton stocks in New Zealand subantarctic waters: contribution of atmospheric and oceanic iron supply. Global Biogeochem. Cycles 18:GB1029. doi: 10.1029/2002GB002020
Boyd, P. W., and Ellwood, M. J. (2010). The biogeochemical cycle of iron in the ocean. Nat. Geosci. 3, 675–682. doi: 10.1038/ngeo964
Boyd, P. W., Ellwood, M. J., Tagliabue, A., and Twining, B. S. (2017). Biotic and abiotic retention, recycling and remineralization of metals in the ocean. Nat. Geosci. 10, 167–173. doi: 10.1038/ngeo2876
Boyd, P. W., Jickells, T., Law, C. S., Blain, S., Boyle, E. A., Watson, K. O., et al. (2007). Mesoscale iron enrichment experiments 1993-2005: synthesis and future directions. Science 315, 612–617. doi: 10.1126/science.1131669
Boye, M., van den Berg, C. M. G., de Jong, J. T. M., Leach, H., Croot, P., and de Baar, H. J. W. (2001). Organic complexation of iron in the Southern Ocean. Deep Sea Res. I Oceanogr. Res. Papers 48, 1477–1497. doi: 10.1016/S0967-0637(00)00099-6
Boye, M., Nishioka, J., Croot, P., Laan, P., Timmermans, K. R., de Baar, V. H., et al. (2010). Significant portion of dissolved organic Fe complexes in fact is Fe colloids. Mar. Chem. 122, 20–27. doi: 10.1016/j.marchem.2010.09.001
Bruland, K. W., and Silver, M. W. (1981). Sinking rate of fecal pellets from gelatinous zooplankton (Salps, Pteropods, Doliolids). Mar. Biol. 63, 295–300.
Bucciarelli, E., Blain, S., and Tre guer, P. (2001). Iron and manganese in the wake of the Kerguelen Islands (Southern Ocean). Mar. Chem. 73, 21–36. doi: 10.1016/S0304-4203(00)00070-0
Buesseler, K. O., and Boyd, P. W. (2009). Shedding light on processes that control particle export and flux attenuation in the twilight zone of the open ocea. Limnol. Oceanogr. 54, 1210–1232. doi: 10.4319/lo.2009.54.4.1210
Cabanes, D. J. E., Norman, L., Santos-Echeandía, J., Iversen, M. H., Trimborn, S., Hassler, L. M., et al. (2017). First evaluation of the role of salp fecal pellets on iron biogeochemistry. Front. Mar. Sci. 3:289. doi: 10.3389/fmars.2016.00289
Cassar, N., Bender, M. L., Barnett, B. A., Fan, S., Moxim, W. J., Tilbrook, B., et al. (2007). The Southern Ocean biological response to aeolian iron deposition. Science 317, 1067–1070. doi: 10.1126/science.1144602
Charette, M. A., Gonneea, M. E., Morris, P., Statham, P., Fones, G., Garabato, A. N., et al. (2007). Radium isotopes as tracers of iron sources fueling a Southern Ocean phytoplankton bloom. Deep Sea Res. II 54, 1989–1998. doi: 10.1016/j.dsr2.2007.06.003
Cisewski, B., Strass, V. H., and Prandke, H. (2005). Upper-ocean vertical mixing in the Antarctic Polar Front Zone. Deep Sea Res. II Top. Stud. Oceanogr. 52, 1087–1108.
Clarke, A., and Tyler, P. A. (2008). Adult antarctic krill feeding at abyssal depths. Curr. Biol. 18, 282–285. doi: 10.1016/j.cub.2008.01.059
Croot, P. L., and Laan, P. (2002). Continuous shipboard determination of Fe(II) in polar waters using flow injection analysis with chemiluminescence detection. Anal. Chim. Acta 466, 261–273, doi: 10.1016/S0003-2670(02)00596-2
de Baar, H. J. W., and de Jong, J. T. M. (2001). Distributions, Sources and Sinks of Iron in Seawater, Biogeochemistry of Fe in Seawater, Chapter 5. Baltimore, MD: John Wiley.
de Baar, H. J. W., de Jong, J. T. M., Bakker, D. C. E., Loscher, B. M., Veth, C., Smetacek, V., et al. (1995). Importance of iron for plankton blooms and carbon dioxide drawdown in the Southern Ocean. Nature 373, 412–415. doi: 10.1038/373412a0
Demer, D. A., and Hewitt, R. P. (1995). Bias in acoustic biomass estimates of Euphausia superba due to diel vertical migration. Deep Sea Res. I Oceanogr. Res. Pap. 42, 455–475. doi: 10.1016/0967-0637(94)E0005-C
Duce, R. A., and Tindale, N. W. (1991). Atmospheric transport of iron and its deposition in the ocean. Limnol. Oceanogr. 36, 1715–1726. doi: 10.4319/lo.1991.36.8.1715
Dulaiova, H., Ardelan, M. V., Henderson, P. B., and Charette, M. A. (2009). Shelf-derived iron inputs drive biological productivity in the southern Drake Passage. Global Biogeochem. Cycles 23, 1–14. doi: 10.1029/2008GB003406
Duprat, L. P. A. M., Bigg, G. R., and Wilton, D. J. (2016). Enhanced Southern Ocean marine productivity due to fertilization by giant icebergs. Nat. Geosci. 9, 219–221. doi: 10.1038/ngeo2633
Dupuy, C., Pagano, M., Got, P., Domaizon, I., Chappuis, A., Marchessaux, G., et al. (2016). Trophic relationships between metazooplankton communities and their plankton food sources in the Iles Eparses (Western Indian Ocean). Mar. Environ. Res. 116, 18–31. doi: 10.1016/j.marenvres.2016.02.011
Falkowski, P. G., Barber, R. T., and Smetacek, V. (1998). Biogeochemical controls and feedbacks on ocean primary production. Science 281, 200–206. doi: 10.1126/science.281.5374.200
Frants, M., Gille, S. T., Hatta, M., Hiscock, M. R., Kahru, M., Measures, C. I., et al. (2013). Analysis of horizontal and vertical processes contributing to natural iron supply in the mixed layer in southern Drake Passage. Deep Sea Res. II Top. Stud. Oceanogr. 90, 68–76. doi: 10.1016/j.dsr2.2012.06.001
Fung, I. Y., Meyn, S. K., Tegen, I., Doney, S. C., John, J. G., and Bishop, J. K. B. (2000). Iron supply and demand in the upper ocean. Glob Biogeochem. Cycles 14, 281–295. doi: 10.1029/1999GB900059
Gledhill, M., and van den Berg, C. M. G. (1994). Determination of complexation of iron(III) with natural organic complexing ligands in seawater using cathodic stripping voltammetry. Mar. Chem. 47, 41–54. doi: 10.1016/0304-4203(94)90012-4
Gledhill, M., McCormack, P., Ussher, S. J., Achterberg, E. P., Mantoura, R. F. C., and Worsfold, P. J. (2004). Production of siderophore type chelate mixed bacterioplankton populations in nutrient enriched seawater incubations. Mar. Chem. 88, 75–83. doi: 10.1016/j.marchem.2004.03.003
Guieu, C., Bozec, Y., Blain, S., Ridame, C., Sarthou, G., and Leblond, N. (2002). Impact of high Saharan dust inputs on dissolved iron distributions in the Mediterranean Sea. Geophys. Res. Lett. 29, 17–1–17–4. doi: 10.1029/2001GL014454
Hassler, C. S., Alasonati, E., Mancuso Nichols, C. A., and Slaveykova, V. I. (2011a). Exopolysaccharides produced by bacteria isolated from the pelagic Southern Ocean—Role in Fe binding, chemical reactivity, and bioavailability. Mar. Chem. 123, 88–98. doi: 10.1016/j.marchem.2010.10.003
Hassler, C., and Schoemann, V. (2009). Bioavailability of organically bound Fe to model phytoplankton of the Southern Ocean. Biogeosciences 6, 2281–2296. doi: 10.5194/bg-6-2281-2009
Hassler, C. S. V., Boye, M., Tagliabue, A., Rozmarynowycs, M., and McKay, R. M. L. (2012). Iron Bioavailability in the Southern Ocean. Boca Raton, FL: CRC Press.
Hassler, C. S. V., Mancuso Nichols, C. A., Butler, E., and Boyd, P. W. (2011b). Saccharides enhance iron bioavailbility to Southern Ocean phytoplankton. Proc. Natl Acad. Sci. U.S.A. 108, 1076–1081. doi: 10.1073/pnas.1010963108
Herraiz-Borreguero, L., Church, J. A., Allison, I., Peña-Molino, B., Coleman, R., Craven, M., et al. (2016). Basal melt, seasonal water mass transformation, ocean current variability, and deep convection processes along the Amery Ice Shelf calving front, East Antarctica. J. Geophys. Res. 121, 4946–4965. doi: 10.1002/2016JC011858
Honeyman, B. D., and Santschi, P. H. (1989). A Brownian-pumping model for oceanic trace metal scavenging: evidence from Th isotopes. J. Mar. Res. 47, 951–992. doi: 10.1357/002224089785076091
Hunter, K. A., and Boyd, P. W. (2007). Iron-binding ligands and their role in the ocean bio-geochemistry of iron. Environ. Chem. 4, 221–232. doi: 10.1071/EN07012
Jickells, T. D., An, Z. S., Andersen, K. K., Baker, A. R., Bergametti, G., Brooks, N., et al. (2005). Global iron connections between desert dust, ocean biogeochemistry, and climate. Science 308, 67–71. doi: 10.1126/science.1105959
Katija, K. (2012). Biogenic inputs to ocean mixing. J. Exp. Biol. 215, 1040–1049. doi: 10.1242/jeb.059279
Katija, K., and Dabiri, J. O. (2009). A viscosity-enhanced mechanism for biogenic ocean mixing. Nat. Lett. 460, 624–627. doi: 10.1038/nature08207
Khatiwala, S., Primeau, F., and Hall, T. (2009). Reconstruction of the history of anthropogenic CO2 concentrations in the ocean. Nat. Lett. 462, 346–350. doi: 10.1038/nature08526
Khatiwala, S., Tanhua, T., Mikalof Fletcher, S., Gerber, M., Doney, S. C., Sabine, C. L., et al. (2013). Global ocean storage of anthropogenic carbon. Biogeosciences 10, 2169–2191. doi: 10.5194/bg-10-2169-2013
Klunder, M. B., Laan, P., Middag, R., De Baar, H. J. W., and van Ooijen, J. C. (2011). Dissolved iron in the Southern Ocean (Atlantic sector). Deep Sea Res. II Top. Stud. Oceanogr. 58, 2678–2694. doi: 10.1016/j.dsr2.2010.10.042
Laglera, L. M., Tovar-Sánchez, A., Iversen, M. H., González, H. E., Naik, H., Mangesh, G., et al. (2017). Iron partitioning during LOHAFEX: copepod grazing as a major driver for iron recycling in the Southern Ocean. Mar. Chem. 196, 148–161. doi: 10.1016/j.marchem.2017.08.011
Lancelot, C., deMontety, A., Goosse, H., Becquevort, S., Schoemann, V., Pasquer, B., et al. (2009). Spatial distribution of the iron supply to phytoplankton in the Southern Ocean: a model study. Biogeosciences 6, 2861–2878. doi: 10.5194/bg-6-2861-2009
Lannuzel, D., Bowie, A. R., Remenyi, T., Lam, P., Townsend, A., Schoemann, V., et al. (2011). Distributions of dissolved and particulate iron in the sub-Antarctic and Polar Frontal Southern Ocean (Australian sector). Deep Sea Res. II Top. Stud. Oceanogr. 58, 2094–2112. doi: 10.1016/j.dsr2.2011.05.027
Lannuzel, D., Grotti, M., Abelmoschi, M. L., and van der Merwe, P. (2015). Organic ligands control the concentrations of dissolved iron in Antarctic sea ice. Mar. Chem. 174, 120–130. doi: 10.1016/j.marchem.2015.05.005
Lannuzel, D., Schoemann, V., de Jong, J., Tison, J.-L., and Chou, L. (2007). Distribution and biogeochemical behaviour of iron in the East Antarctic sea ice. Mar. Chem. 106, 18–32. doi: 10.1016/j.marchem.2006.06.010
Lascara, C. M., Hofmann, E. E., Ross, R. M., and Quetin, L. B. (1999). Seasonal variability in the distribution of Antarctic krill, Euphausia superba, west of the Antarctic Peninsula. Deep Sea Res. I Oceanogr. Res. Pap. 46, 951–984. doi: 10.1016/S0967-0637(98)00099-5
Lefèvre, N., and Watson, A. J. (1999). Modeling the geochemical cycle of iron in the oceans and its impact on atmospheric CO2 concentrations. Glob. Biogeochem. Cycles 13, 727–736. doi: 10.1029/1999GB900034
Lehette, P., Tovar-Sanchez, A., Duarte, C., and Hernandez-Leon, S. (2012). Krill excretion and its effect on primary production. Mar. Ecol. Prog. Ser. 459, 29–38. doi: 10.3354/meps09746
Lin, H., Rauschenberg, S., Hexel, C. R., Shaw, T. J., and Twining, B. S. (2011). Free-drifting icebergs as sources of iron to the Weddell Sea. Deep Sea Rese. II Top. Stud. Oceanogr. 58, 1392–1406. doi: 10.1016/j.dsr2.2010.11.020
Liu, X., and Millero, F. J. (2002). The solubility of iron in seawater. Mar. Chem. 77, 43–54. doi: 10.1016/S0304-4203(01)00074-3
Mahowald, N. M., Baker, A. R., Bergametti, G., Brooks, N., Duce, R. A., Tegen, I., et al. (2005). Atmospheric global dust cycle and iron inputs to the ocean. Glob. Biogeochem. Cycles 19:GB4025. doi: 10.1029/2004GB002402
Maldonado, M. T., Strzepek, R. F., Sander, S., and Boyd, P. W. (2005). Acquisition of iron bound to strong organic complexes, with different Fe binding groups and photochemical reactivities, by plankton communities in Fe-limited subantarctic waters. Global Biogeochem. Cycles 19:GB4S23. doi: 10.1029/2005GB002481
Maldonado, M. T., Surma, S., and Pakhomov, E. A. (2016). Southern Ocean biological iron cycling in the pre-whaling and present ecosystems. Philos. Trans. R. Soc. A. 374:20150292. doi: 10.1098/rsta.2015.0292
Martin, J., Fitzwater, S., and Gordon, R. (1990). Iron deficiency limits phytoplankton growth in Antarctic waters. Global Biogeochem. Cycles 4, 5–12. doi: 10.1029/GB004i001p00005
Mawji, E., Gledhill, M., Zubkov, M. V., Thompson, A., and Wolff, G. (2011). Production of siderophore type chelates in Atlantic Ocean waters enriched with different carbon and nitrogen sources. Mar. Chem. 124, 90–99. doi: 10.1016/j.marchem.2010.12.005
Millero, F. J., Sotolongo, S., and Izaguirre, M. (1987). The oxidation kinetics of Fe(II) in seawater. Geochim. Cosmochim. Acta 51, 793–801. doi: 10.1016/0016-7037(87)90093-7
Moore, J. K., Doney, S. C., and Lindsay, K. (2004). Upper ocean ecosystem dynamics and iron cycling in a global three-dimensional model. Global Biogeochem. Cycles 18:GB4028. doi: 10.1029/2004GB002220
Morata, N., and Seuthe, L. (2014). Importance of bacteria and protozooplankton for faecal pellet degradation. Oceanologia 56, 565–581. doi: 10.5697/oc.56-3.565
Morel, F. M. M., Milligan, A. J., and Saito, M. A. (2003). Marine bioinorganic chemistry: the role of trace metals in the oceanic cycles of major nutrients. Oceans Mar. Geochem. Treatise Geochem. 6, 113–143. doi: 10.1016/B0-08-043751-6/06108-9
Morel, F. M. M., and Price, N. M. (2003). The biogeochemical cycles of trace metals in the oceans. Science 300, 944–947. doi: 10.1126/science.1083545
Nicol, S., Bowie, A., Jarman, S. N., Lannuzel, D., Meiners, K. M., and Van Der Merwe, P. (2010). Southern Ocean iron fertilization by baleen whales and Antarctic krill. Fish Fishe. 11, 203–209. doi: 10.1111/j.1467-2979.2010.00356.x
Noji, T. T., Estep, K. W., Macintyre, F., and Norrbin, F. (1991). Image analysis of faecal material grazed upon by three species of copepods: evidence for coprorhexy, coprophagy and coprochaly. J. Mar. Biol. Assoc. U.K. 71, 465–480. doi: 10.1017/S0025315400051717
Ortega-Retuerta, E., Frazer, T. K., Duarte, C. M., Ruiz-Halpern, S., Tovar-Sanchez, A., Rechea, I., et al. (2009). Biogeneration of chromophoric dissolved organic matter by bacteria and krill in the Southern Ocean. Limnol. Oceanogr. 54, 1941–1950. doi: 10.4319/lo.2009.54.6.1941
Pakhomov, E. A., Perissinotto, R., McQuaid, C. D., and Froneman, P. W. (2000). Zooplankton structure and grazing in the Atlantic sector of the Southern Ocean in late austral summer 1993: part 1: ecological zonation. Deep Sea Res. 1. Oceanogr. Res. Pap. 47, 1663–1686. doi: 10.1016/S0967-0637(99)00122-3
Passow, U., and Carlson, C. A. (2012). The biological pump in a high CO2 world. Mar. Ecol. Prog. Ser. 470, 249–271. doi: 10.3354/meps09985
Pearce, I., Davidson, A. T., Thomson, P. G., Wright, S., and, R., and van den Enden (2011). Marine microbial ecology in the sub-Antarctic Zone: rates of bacterial and phytoplankton growth and grazing by heterotrophic protists. Deep Sea Res. II 58, 2248–2259. doi: 10.1016/j.dsr2.2011.05.030
Perissinotto, R. (1997). In situ grazing rates and daily ration of Antarctic krill Euphausia superba feeding on phytoplankton at the antarctic polar front and the marginal ice zone. Mar. Ecol. Prog. Ser. 160:77. doi: 10.3354/meps160077
Ratnarajah, L., Bowie, A. R., Lannuzel, D., Meiners, K. M., and Nicol, S. (2014). Biogeochemical role of baleen whales and Antarctic krill in Southern Ocean nutrient cycling. PLoS ONE 9:e114067. doi: 10.1371/journal.pone.0114067
Ratnarajah, L., Lannuzel, D., Townsend, A. T., Meiners, K. M., Nicol, S., Bowie, A. R., et al. (2017). Physical speciation and solubility of iron from baleen whale faecal material. Mar. Chem. 194, 78–88. doi: 10.1016/j.marchem.2017.05.004
Ratnarajah, L., Melbourne-Thomas, J., Marzloff, M. P., Lannuzel, D., Meiners, K. M., Bowie, A. R., et al. (2016a). A preliminary model of iron fertilisation by baleen whales and Antarctic krill in the Southern Ocean: sensitivity of primary productivity estimates to parameter uncertainty. Ecol. Modell. 320, 203–212. doi: 10.1016/j.ecolmodel.2015.10.007
Ratnarajah, L., Nicol, S., Kawaguchi, S., Townsend, A. T., Lannuzel, D., Meiners, K. M., et al. (2016b). Understanding the variability in the iron concentration of Antarctic krill. Limnol. Oceanogr. 61, 1651–1650. doi: 10.1002/lno.10322
Rijkenberg, M. J. A., Fischer, A. C., Kroon, K., Gerringa, L. J. A., Timmermans, K. R., de Baar, H. J. W., et al. (2005). The influence of UV irradiation on the photoreduction of iron in the Southern Ocean. Mar. Chem. 93, 119–129. doi: 10.1016/j.marchem.2004.03.021
Rijkenberg, M. J. A., Gerringa, L. J. A., Carolus, V. E., Velzeboer, I., and de Baar, H. J. W. (2006). Enhancement and inhibition of iron photoreduction by individual ligands in open ocean seawater. Geochim. Cosmochim. Acta 70, 2790–2805. doi: 10.1016/j.gca.2006.03.004
Ruiz-Halpern, S., Duarte, C. M., Tovar-Sanchez, A., Pastor, M., Horstkotte, B., Lasternas, S., et al. (2011). Antarctic krill as a source of dissolved organic carbon to the Antarctic ecosystem. Limnol. Oceanogr. 56, 521–528. doi: 10.4319/lo.2011.56.2.0521
Safi, K. A., Griffiths, F. B., and Hall, J. A. (2007). Microzooplankton composition, biomass and grazing rates along the WOCE SR3 line between Tasmania and Antarctica. Deep Sea Res. I Oceanogr. Res. Pap. 54, 1025–1041. doi: 10.1016/j.dsr.2007.05.003
Sarthou, G., Baker, A. R., Blain, S., Achterberg, E. P., Boye, M., Bowie, A. R., et al. (2003). Atmospheric iron deposition and sea-surface dissolved iron concentrations in the eastern Atlantic Ocean. Deep Sea Res. I Oceanogr. Res. Pap. 50, 1339–1352. doi: 10.1016/S0967-0637(03)00126-2
Sarthou, G., Jeandel, C., Brisset, L., Amouroux, D., Besson, T., and Donard, O. F. X. (1997). Fe and H2O2 distributions in the upper water column in the Indian sector of the Southern Ocean. Earth Planet. Sci. Lett. 147, 83–92. doi: 10.1016/S0012-821X(97)00004-6
Sarthou, G., Vincent, D., Christaki, U., Obernosterer, I., Timmermans, K. R., and Brussaard, C. P. D. (2008). The fate of biogenic iron during a phytoplankton bloom induced by natural fertilisation: impact of copepod grazing. Deep Sea Res. II Top. Stud. Oceanogr. 55, 734–751. doi: 10.1016/j.dsr2.2007.12.033
Sartoris, F. J., Thomas, D. N., Cornils, A., and Schnack-Schiel, S. B. (2010). Buoyancy and diapause in Antarctic copepods: the role of ammonium accumulation. Limnol. Oceanogr. 55, 1860–1864. doi: 10.4319/lo.2010.55.5.1860
Sato, M., Takeda, S., and Furuya, K. (2007). Iron regeneration and organic iron(III)-binding lidang production during in situ zooplankton grazing experiment. Mar. Chem. 106, 471–488. doi: 10.1016/j.marchem.2007.05.001
Schmidt, K., and Atkinson, A. (2016). Feeding and food processing in Antarctic krill (Euphausia superba Dana). Adv. Polar Ecol. 175–224. doi: 10.1007/978-3-319-29279-3_50
Schmidt, K., Atkinson, A., Pond, D. W., and Ireland, L. C. (2014). Feeding and overwintering of Antarctic krill across its major habitats: the role of sea ice cover, water depth, and phytoplankton abundance. Limnol. Oceanogr. 59, 17–36. doi: 10.4319/lo.2014.59.1.0017
Schmidt, K., Atkinson, A., Steigenberger, S., Fielding, S., Lindsay, M. C. M., Achterberg, E. P., et al. (2011). Seabed foraging by Antarctic krill: implications for stock assessment, bentho-pelagic coupling, and the vertical transfer of iron. Limnol. Oceanogr. 56, 1411–1428. doi: 10.4319/lo.2011.56.4.1411
Schnetzer, A., and Steinberg, D. K. (2002). Active transport of particulate organic carbon and nitrogen by vertically migrating zooplankton in the Sargasso Sea. Mar. Ecol. Progr. Ser. 234, 71–84. doi: 10.3354/meps234071
Sedwick, P. N., Bowie, A. R., and Trull, T. W. (2008). Dissolved iron in the Australian sector of the Southern Ocean (CLIVAR SR3 section): meridional and seasonal trends. Deep Sea Res. I Oceanogr. Res. Pap. 55, 911–925. doi: 10.1016/j.dsr.2008.03.011
Sedwick, P. N., and Di Tullio, G. R. (1997). Regulation of algal blooms in Antarctic shelf waters by the release of iron from melting sea ice. Geophys. Res. Lett. 24, 2515–2518. doi: 10.1029/97GL02596
Shatova, O., Wing, S. R., Gault-Ringold, M., Wing, L., and Hoffmann, L. (2016). Seabird guano enhances phytoplankton production in the Southern Ocean. J. Exp. Mar. Biol. Ecol. 483, 74–87. doi: 10.1016/j.jembe.2016.07.004
Smetacek, V. (2008). Are Declining Antarctic Krill Stocks a Result of Global Warming or of the Decimation of the Whales. Fundación BBVA.
Smetacek, V., Assmy, P., and Henjes, J. (2004). The role of grazing in structuring Southern Ocean pelagic ecosystems and biogeochemical cycles. Antar. Sci. 16, 541–558. doi: 10.1017/S0954102004002317
Smetacek, V., and Nicol, S. (2005). Polar ocean ecosystems in a changing world. Nature 437, 362–368. doi: 10.1038/nature04161
Smith, K. L., Robinson, B. H., Helly, J. J., Kaufman, R. S., Ruhl, H. A., Shaw, T. J., et al. (2007). Free-drifting icebergs: hot spots of chemical and biological enrichment in the Weddell Sea. Science 317, 478–482. doi: 10.1126/science.1142834
Spicer, J. I., and Saborowski, R. (2010). Physiology and Metabolism of Northern Krill (Meganyctiphanes norvegica Sars). Adv. Mar. Biol. 57, 91–126. doi: 10.1016/B978-0-12-381308-4.00004-2
Street, J. H., and Payton, A. (2005). Iron, Phytoplankton Growth and the Carbon Cycle. Boca Raton, FL: Taylor and Francis.
Strzepek, R. F., Maldonado, M. T., Hunter, K. A., Frew, R. D., and Boyd, P. W. (2011). Adaptive strategies by Southern Ocean phytoplankton to lessen iron limitation: uptake of organically complexed iron and reduced cellular iron requirements. Limnol. Oceanogr. 56, 1983–2002. doi: 10.4319/lo.2011.56.6.1983
Tagliabue, A., Bopp, L., Dutay, J.-C., Bowie, A. R., Chever, F., Jean-Baptiste, P., et al. (2010). Hydrothermal contribution to the oceanic dissolved iron inventory. Nat. Geosci. 3, 252–256. doi: 10.1038/ngeo818
Tagliabue, A., Sallee, J.-B., Bowie, A. R., Levy, M., Swart, S., and Boyd, P. W. (2014). Surface-water iron supplies in the Southern Ocean sustained by deep winter mixing. Nat. Geosci. 7, 314–320. doi: 10.1038/ngeo2101
Tirelli, V. (2005). Relationship between functional response and gut transit time in the calanoid copepod Acartia clausi: role of food quantity and quality. J. Plankton Res. 57, 557–568. doi: 10.1093/plankt/fbi031
Tovar-Sanchez, A., Duarte, C. M., Hernández-León, S., and Sañudo-Wilhelmy, S. A. (2007). Krill as a central node for iron cycling in the Southern Ocean. Geophys. Res. Lett. 34:L11601. doi: 10.1029/2006GL029096
Tovar-Sanchez, A., Duarte, C. M., Hernández-León, S., and Sañudo-Wilhelmy, S. A. (2009). Impact of submarine hydrothermal vents on the metal composition of krill and its excretion products. Mar. Chem. 113, 129–136. doi: 10.1016/j.marchem.2009.01.010
Turner, J. T. (2002). Zooplankton fecal pellets, marine snow and sinking phytoplankton blooms. Aquat. Microb. Ecol. 27, 57–102. doi: 10.3354/ame027057
Turner, J. T. (2015). Zooplankton fecal pellets, marine snow, phytodetritus and the ocean's biological pump. Progr. Oceanogr. 130, 205–248. doi: 10.1016/j.pocean.2014.08.005
Wagener, T., Guieu, C., Losno, R., Bonnet, S., and Mahowald, N. (2008). Revisiting atmospheric dust export to the Southern Hemisphere ocean: biogeochemical implications. Glob. Biogeochem. Cycles 22:GB2006. doi: 10.1029/2007GB002984
Walsh, J. J., Dieterle, D. A., and Lenes, J. (2001). A numerical analysis of carbon dynamics of the Southern Ocean phytoplankton community: the roles of light and grazing in effecting both sequestration of atmospheric CO2 and food availability to larval krill. Deep Sea Res. I Oceanogr. Res. Pap. 48, 1–48. doi: 10.1016/S0967-0637(00)00032-7
Watson, A. J. (2001). “Iron limitation in the oceans,” in The Biogeochemistry of Iron in Seawater, IUPAC Series on Analytical and Physical Chemistry of Environmental Systems, eds D. R. Turner and K. H. Hunter (West Sussex: John Wiley and Sons Ltd.), 396.
Webb, D. J., and Suginohara, N. (2001). Oceanography: vertical mixing in the ocean. Nature 409:37. doi: 10.1038/35051171
Wells, M. L., and Mayer, L. M. (1991). The photoconversion of colloidal iron oxyhydroxides in seawater. Deep Sea Res. I 38, 1379–1395.
Whitehouse, M. J., Atkinson, A., and Rees, A. P. (2011). Close coupling between ammonium uptake by phytoplankton and excretion by Antarctic krill, Euphausia superba. Deep Sea Res. I Oceanogr. Res. Pap. 58, 725–732. doi: 10.1016/j.dsr.2011.03.006
Wing, S. R., Gault-Ringold, M., Stirling, C. H., Wing, L. C., Shatova, O. A., and Frew, R. D. (2017). δ56Fe in seabird guano reveals extensive recycling of iron in the Southern Ocean ecosystem. Limnol. Oceanogr. 62, 1671–1681. doi: 10.1002/lno.10524
Wing, S. R., Jack, L., Shatova, O., Leichter, J. J., Barr, D., Frew, R. D., et al. (2014). Seabirds and marine mammals redistribute bioavailable iron in the Southern Ocean. Mar. Ecol. Progr. Ser. 510, 1–13. doi: 10.3354/meps10923
Wu, J., Boyle, E., Sunda, W., and Wen, L.-S. (2001). Soluble and colloidal iron in the oligotrophic North Atlantic and North Pacific. Science 293, 847–849. doi: 10.1126/science.1059251
Yang, E. J., Jiang, Y., and Lee, S. H. (2016). Microzooplankton herbivory and community structure in the Amundsen Sea, Antarctica. Deep Sea Res. II Top. Stud. Oceanogr. 123, 58–68. doi: 10.1016/j.dsr2.2015.06.001
Yang, G., Li, C., Guilini, K., Peng, Q., Wang, Y., Zhang, Y., et al. (2016). Feeding strategies of four dominant copepod species in Prydz Bay, Antarctica: insights from a combined fatty acid biomarker and stable isotopic approach. Deep Sea Res. I Oceanogr. Res. Pap. 114, 55–63. doi: 10.1016/j.dsr.2016.04.016
Keywords: biological carbon pump, iron, carbon, Southern Ocean, biological recycling
Citation: Ratnarajah L, Nicol S and Bowie AR (2018) Pelagic Iron Recycling in the Southern Ocean: Exploring the Contribution of Marine Animals. Front. Mar. Sci. 5:109. doi: 10.3389/fmars.2018.00109
Received: 30 October 2017; Accepted: 15 March 2018;
Published: 29 March 2018.
Edited by:
Antonio Tovar-Sanchez, Consejo Superior de Investigaciones Científicas (CSIC), SpainReviewed by:
Elvira Pulido-Villena, UMR7294 Institut Méditerranéen d'océanographie (MIO), FranceEric 'Pieter Achterberg, GEOMAR Helmholtz Centre for Ocean Research Kiel, Germany
Copyright © 2018 Ratnarajah, Nicol and Bowie. This is an open-access article distributed under the terms of the Creative Commons Attribution License (CC BY). The use, distribution or reproduction in other forums is permitted, provided the original author(s) and the copyright owner are credited and that the original publication in this journal is cited, in accordance with accepted academic practice. No use, distribution or reproduction is permitted which does not comply with these terms.
*Correspondence: Lavenia Ratnarajah, bC5yYXRuYXJhamFoQGxpdmVycG9vbC5hYy51aw==