- 1Centre National de la Recherche Scientifique, Laboratoire d'Océanographie de Villefranche-sur-Mer (LOV), Sorbonne Universités, UPMC University Paris 06, Villefranche-sur-Mer, France
- 2Ocean Sciences, Rosenstiel School of Marine and Atmospheric Science, University of Miami, Miami, FL, United States
Most demersal fishes undergo a dispersal phase as larvae, which strongly influences the connectivity among adult populations and, consequently, their genetic structure and replenishment opportunities. Because this phase is difficult to observe directly, it is frequently simulated through numerical models, most of which consider larvae as passive or only vertically migrating. However, in several locations, including the Mediterranean Sea, many species have been shown to swim fast and orient. Here we use a Lagrangian model to study connectivity patterns among three Mediterranean Marine Protected Areas (MPAs) and compare simulations in which virtual larvae are passive to simulations in which oriented swimming is implemented. The parameterization of behavior is based on observations for two groups of species of the family Sparidae: species with small larvae (i.e., 9–11 mm), displaying a maximum swimming speed of 6 cm s−1 and a pelagic larval duration of 13–19 days (e.g., Diplodus annularis L., Oblada melanura L.) and species with large larvae (i.e., 14–16 mm), displaying a maximum swimming speed of 10 cm s−1 and a PLD of 28–38 days (e.g., Spondyliosoma cantharus L.). Including larval behavior in the model (i) increased the overall proportion of successful settlers, (ii) enhanced self-recruitment within the MPAs, but also (iii) increased the intensity, and (iv) widened the export of eggs and larvae (recruitment subsidy) from the MPAs; overall, it significantly changed connectivity patterns. These results highlight the need to gather the observational data that are required to correctly parameterize connectivity models.
Introduction
Many coastal fish species undergo a pelagic larval phase while adults generally have a restricted home range, leading most dispersal to occur during the larval phase (Gaines et al., 2007), even if it is not limited to this stage (e.g., Di Franco et al., 2015). Predicting recruitment in adult populations after this larval phase is a major challenge in marine ecology and for the sustainable management of marine resources. But it remains difficult because the factors determining larval dispersal and survival are not all identified (Cowen et al., 2007; Botsford et al., 2009). Various methods have been developed to study larval fish dispersal (Cowen et al., 2007) and showed that dispersal distances may reach hundreds of kilometers in some regions (Kinlan and Gaines, 2003) or be very limited, with surprisingly high self-recruitment rates, in others (Jones et al., 2005; Cowen et al., 2006; Gerlach et al., 2007; Almany et al., 2017). Among all methods, modeling is the only approach that enables tracking (virtual) larvae throughout their pelagic journey (North et al., 2009). Biophysical models therefore contributed largely to the understanding of larval dispersal and population connectivity (e.g., Truelove et al., 2017) and to the testing of marine protected areas (MPAs) networks efficiency (Mouillot et al., 2011). Today, the use of such models have generalized and some even succeeded in predicting the intensity of larval supply to coastal areas (Sponaugle et al., 2012).
Robust connectivity models are particularly required in the Mediterranean Sea because European Union regulations require to expand the network of Mediterranean MPAs by 2020. This network is currently small and not very effective (Mouillot et al., 2011). Throughout the French Riviera, in the North Western Mediterranean basin, fisheries are mostly traditional, coastal, and managed at a small scale (on the order of kilometers). Fishermen are actively implicated in the management of marine resources and the establishment of a no-take zone in Cap Roux (43.5°E, 6.9°N) on their demand proves their willingness to sustainably manage the marine resources they exploit. While this local management is laudable, it also stresses the need for using adequate tools to assess the efficiency of MPA networks at regional scale or to estimate the export of individuals toward exploited areas.
While biophysical models improved, the quantification of larval fish behavior also underwent major advances over the last two decades and it is now recognized that most settlement-stage larvae of tropical and temperate perciform fishes have strong swimming abilities, both in terms of speed and orientation (reviewed in Leis, 2006; see Faillettaz et al., 2017 for data on Mediterranean species). Oriented swimming was initially thought to be limited to coastal areas, where larvae may use odor (Paris et al., 2013), sound (Simpson et al., 2005) or both to detect favorable settlement habitats (Teodósio et al., 2016). Increasing observations now suggest that fish larvae may also orient in the open ocean using coast-independent cues such as the position of the sun (Mouritsen et al., 2013; Berenshtein et al., 2014; Faillettaz et al., 2015) or even the magnetic field (Bottesch et al., 2016; O'Connor and Muheim, 2017). These empirical observations were carried out in several regions and environments: Australia (Leis et al., 2014), Taiwan (Clark et al., 2005), North Sea (Cresci et al., 2017), Mediterranean Sea (Faillettaz et al., 2015) etc. They suggested that most perciform fish larvae have the means to orient at any spatial scale in the oceans using different mechanisms, which may have evolved to help them reach favorable settlement habitats (Paris et al., 2013; Leis et al., 2014, 2015; Bottesch et al., 2016).
It is noteworthy that nearly two decades ago, Jones et al. (1999) developed a numerical approach that suggested that active swimming might be necessary to explain the high self-recruitment rates they observed. Since then, several studies that included larval behavior in biophysical models found striking differences compared to simulations of passively drifting larvae (e.g., Paris and Cowen, 2004; Fiksen et al., 2007; Staaterman et al., 2012; Wolanski and Kingsford, 2014). Several reviews also stressed the importance of considering realistic larval fish behavior in biophysical models (Leis, 2007; Miller, 2007; North et al., 2009; Catalán et al., 2013; Staaterman and Paris, 2014). The reviews' main recommendations are to implement empirically observed behaviors, measured on the species that is modeled (not on a related one, unless if they do not differ), and to include behavior changes that occur throughout ontogeny. Given this amount of evidence and recommendations, today, it would clearly be wrong to apply the “simplifying assumption” (sensu Leis, 2006) and consider fish larvae as passively drifting particles advected by the currents.
However, mainly because of the historical lack of data on larval fish behavior, most models made this assumption, or only considered simple vertical migrations behavior (e.g., Johnson et al., 2013; Nanninga et al., 2015). This is particularly true in the Mediterranean Sea. For example, Di Franco et al. (2012) and Koeck et al. (2015) did not include any behavior in their biophysical model. Yet, they used these models, among other approaches, to test the maximum potential dispersal of Epinephelus marginatus (Lowe, 1834; dusky grouper) larvae (Koeck et al., 2015) or of white seabream Diplodus sargus L. (Di Franco et al., 2012; Pujolar et al., 2013) larvae from a network of MPAs. The youngest larval stages may indeed be considered as passive and, providing the physical model is adequate, the initial export of propagules should therefore be correctly represented (Leis, 2007). However, this is not true for later stages and omitting larval behavior might induce large errors in the estimation of connectivity among MPAs, especially a species with such a long pelagic larval duration (PLD) (about 25 days; Macpherson and Raventos, 2006).
To overcome the lack of behavioral data for this species, Andrello et al. (2013, 2015) ran two sets of simulations, the main one with passively drifting particles and a second one with diel vertical migrations between 0.2 and 50 m, throughout ontogeny. They found the connection distances to be >100 km on average and concluded that diel vertical migration had little influence on the connectivity patterns.
Some models focused on the Mediterranean Sea did implement directional swimming. Basterretxea et al. (2012) first assessed the connectivity in a network of MPA and found that passive retention reaches >30% within 10 km of the seeding MPA. Then, Basterretxea et al. (2013) tested the influence of swimming at 5 cm s−1 toward the coast, while advection transported the larvae offshore. It resulted in slightly higher retention rates (41%), yet still of the same order of magnitude. Faillettaz et al. (2017) implemented shoreward swimming at various speeds, based on empirical data, in a model of the competency period. In that case, the model suggested that swimming abilities of just few cm s−1, over only 4 days at the end of the pelagic phase, can strongly influence the rate of recruitment.
The contrast among these results, both among studies in the Mediterranean Sea and with studies elsewhere (which, almost systematically concluded that behavior strongly influenced dispersal patterns) makes it difficult to reach a conclusion. In addition, the only models that explicitly studied connectivity within the network of Mediterranean MPAs did not include swimming behavior. The present work is a case-study for the inclusion of larval behavior when assessing connectivity within a network of MPAs, with an application to the dispersal of Sparidae (sea breams) larvae in the Ligurian Sea (N-W Mediterranean Sea). We track virtual larvae throughout their pelagic phase and compare a passive scenario with an active one, in which we implement an oriented swimming behavior based on all data available for the species considered.
Methods
Study Area
This study was conducted in the Ligurian Sea, in the Northwestern Mediterranean Sea. In the region, the main hydrological structure is the Liguro-Provençal current, positioned on average 28 km from the shore (Figure 1), between the surface and 150–200 m depth, 25 km wide and flowing at 25–35 cm s−1 toward the south-west (Béthoux and Prieur, 1983; Stemmann et al., 2008). The current is present throughout the year and creates a marked hydrological front that may act as a barrier to offshore dispersal of coastal organisms (Pedrotti and Fenaux, 1992) comparable to other Mediterranean fronts (Galarza et al., 2009).
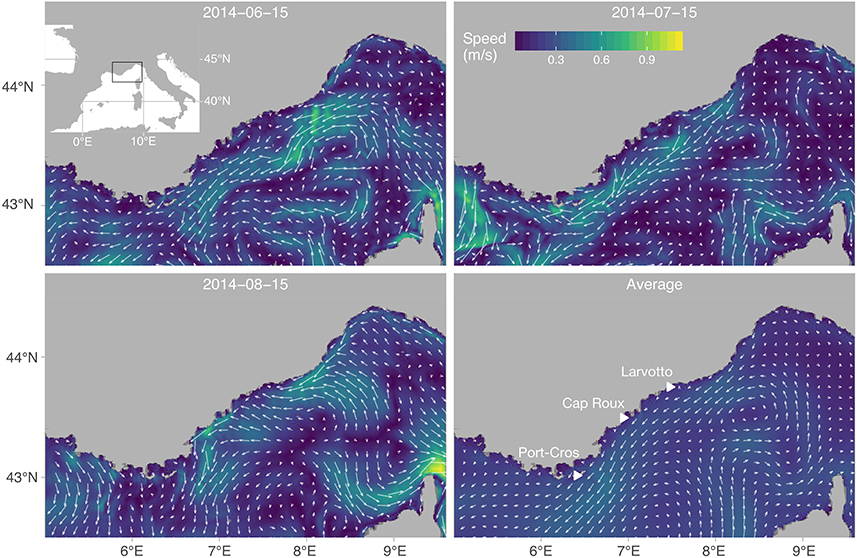
Figure 1. Map of the study region, with simulated current field snapshots at 5 m depth and averaged over the study period. Flow vectors are overlaid on top of a linearly interpolated map of average current intensity. The typical strong jet along the northern coast is highlighted, as well as two stable mesoscale eddies: a well defined cyclone (counterclockwise) centered on 8.5°W, 43.5°N and a weaker anticyclone below. Triangles indicate the three MPAs from where virtual larvae were released.
We focused on three MPAs located in the northern Ligurian Sea, where most behavioral data available for the species of this region has been collected (in and around the Bay of Villefranche-sur-Mer; 43.69°N, 7.31°E; Figure 1): The northernmost is the Larvotto MPA (Monaco, 43.7°N, 7.4°E; Figure 1) with 0.349 km2 closed to fishing activities since 1976. The second MPA is located in Cap Roux (France, 43.5°N, 6.9°E; Figure 1) and comprises an area of 4.45 km2 closed to fishing established in 2003. The third is the National Park of Port-Cros, a 29 km2 MPA established in 1963, in and around the islands of Port-Cros and Porquerolles (France, 6.4°E, 42.9°N).
Hydrodynamic and Lagrangian Models
Ocean current fields were provided by MARS3DMed (Ifremer). This model is described in detail in Lazure and Dumas (2008) and has been validated with observations (Pairaud et al., 2011). Current fields are available every 3 h, on a 1/64° grid (ca. 1.2 km), over 60 sigma layers, and cover the most of the NW Mediterranean Sea, from 0° to 15°E and 39.5° to 44.5°N. We used currents from June 1st to August 29th 2014, the main settlement period for larval fishes in the region (Faillettaz, 2015) and when behavioral data on swimming speed and orientation has been collected (Faillettaz et al., 2015, 2017).
Virtual larvae were simulated using the open-source Connectivity Modeling System (CMS; Paris et al., 2013). At each time-step, this Lagrangian model couples deterministic physical variables from a hydrodynamic model with probabilistic, individual-based biological characteristics such as spawning (location and date), mortality and vertical migration (diel and ontogenic). Of particular interest here, the CMS is the only full-fledged Lagrangian model that also comprises a biased and correlated random walk sub-model that can be used to simulate the oriented swimming behavior of virtual larvae (Staaterman et al., 2012). Swimming speed is set in cm s−1. The direction of orientation can be set to follow a bearing or a target. The precision of orientation is set by the k parameter of a Von Mises distribution (the circular equivalent of a Normal distribution), which ranges from 0 (no orientation) to 5 (high accuracy; Codling et al., 2004). Settlement zones are defined as polygons, drawn by adding a spatial buffer around actual settlement habitat. When a competent larva enters a polygon, it is considered as settled and stops moving, assuming that it will successfully reach the closest favorable settlement habitat within the polygon.
Horizontal diffusivity was set to 105 cm2 s−1 based on diffusion diagrams from Okubo (1971) and the time-step was set to 300 s (1/36th of the hydrodynamic model output time-step). Maximum current speeds were around 0.5 m s−1, which resulted in a maximum passive displacement of 150 m within one time step, well below the 1.2 km grid size, hence avoiding numerical issues during trajectory computation.
Simulations Scenarios
Modeled Species
We focused on two groups of sea bream species, identified as “small Sparidae” (i.e., 9–11 mm in standard length) and “large Sparidae” (i.e., 14–16 mm in standard length), because their morphology and behavioral abilities were found to be similar within group but different between groups (Faillettaz et al., 2017). Small Sparidae comprise, at least, Boops boops Linnaeus, 1758, Oblada melanura L., Spicara smaris L. and Diplodus annularis L. Large Sparidae comprise species such as Dentex dentex L., Pagellus acarne Risso, 1827, Pagrus pagrus L. and Spondyliosoma cantharus L. Larvae of small Sparidae are slenderer, less pigmented, and seemingly less developed at settlement than those in the large Sparidae group. Small Sparidae also swim slower than large Sparidae (Ucrit = 11.1 vs. 19.2 cm s−1; Faillettaz et al., 2017) and their pelagic larval duration is shorter (14–18 d on average vs. 30–38 d; Raventós and Macpherson, 2001; Macpherson and Raventos, 2006).
Particle Seeding
In each of the three MPA (Larvotto, Cap Roux, and Port-Cros), 1500 virtual larvae were seeded at 1 and 15 m depths, twice per day, at 10:00 p.m. and 2:00 a.m., every day from June 1st to 15th and from July 1st to 15th, to match the patterns of larval supply measured in the Bay of Villefranche-sur-Mer: presence of Sparidae between the last and the first quarters of the moon, in June and July 2014 (Faillettaz, 2015). In total, 540,000 larvae were tracked per simulation.
Biological Parameters
The species of the two modeled groups settle in relatively similar coastal habitats (i.e., in seagrass and rocky or soft bottoms; Nelson et al., 2016) and the geomorphology of the study region is homogeneous, with rocky capes and sheltered bays every few kilometers from Genova (Italy) to Toulon (France) and in Corsica. The whole coastline was therefore assumed to be a potential settlement habitat and divided in ~8 km2 polygons (~4 km of coastline × 2 km offshore, yielding 265 polygons). Given the scale of the study (~500 km of coastline) relative to the size of the settlement polygons (4 km of coastline) and of the grid (1.2 km), the possible small deviations from this assumption of uniform habitat distribution would have little influence on the results. Furthermore, most of the region is characterized by a narrow continental shelf, with bottom depth >300 m within a kilometer from the coastline. The offshore expansion of coastal habitats, and of the coastal fish populations they support, is therefore limited to nearshore areas (≤1 km). To avoid boundary issues in the interpolation scheme, we considered that virtual fish larvae could detect a coastal settlement habitat from as far as 2 km (1.5 grid point) from the shore and reach it. Such an assumption is common in Lagrangian models (Cowen et al., 2006; Wolanski and Kingsford, 2014) because settlement habitats and processes cannot be described at the appropriate scale given the coarseness of the models' grids (~1.2 km here).
Mediterranean larvae of Sparidae are mostly concentrated in the upper 10 m of the water column, with very limited to negligible diel vertical migration (Sabatés and Olivar, 1996). Larvae were therefore simulated on fixed, near-surface sigma levels.
Mortality would decrease the settlement rate but was not considered in the model because only constant mortality rates could have been implemented, which would not have altered the relative settlement rates predicted.
Two simulations were run for each of the two groups of Sparidae: one with passively drifting virtual larvae and the other with oriented swimming. For “passive” simulations, the only parameter that differed between Sparidae groups was the pelagic larval duration (PLD). To account for the flexibility in the competency phase, which can span up to several days in Mediterranean species of sea breams (Calò et al., 2016), small Sparidae were considered competent from 13 to 19 days and large ones from 28 to 38 days. Larvae that reached a settlement polygon within their competency period were considered as settled. Larvae that did not reach a polygon by the end of their PLD were considered as dead. For “active” simulations, swimming speed and orientation abilities were implemented based on empirical observations carried out within the simulated region and period, for the two groups of Sparidae considered (Faillettaz et al., 2015, 2017).
During the competency phase, swimming speed was set to half the critical speed measured by Faillettaz et al. (2017). This is an estimate for the in situ speed, a speed that accounts for feeding-related speed changes, is assumed to be sustainable over long periods of time, and is therefore a conservative estimate for the swimming abilities of wild larvae (Leis and Carson-Ewart, 1998; Leis and Clark, 2005; Leis and Fisher, 2006). The ontogeny of swimming abilities was based on observations for other temperate and tropical fish larvae (Fisher et al., 2000; Clark et al., 2005; Leis et al., 2007; Faria et al., 2009): swimming speed was negligible before flexion, after the flexion (8 days; Leis, 2010) it was set to 1 cm s−1 and then increased linearly to 6 cm s−1 at 13 d for small Sparidae and to 10 cm s−1 at 28 d for large Sparidae.
Similarly, the orientation abilities of species belonging to the two groups of Sparidae were investigated in the same location and time of the year (Faillettaz et al., 2015). The vast majority of individuals (>85%) followed a cardinal bearing while in blue waters and most used the sun's azimuth as a compass, providing a potential mechanism for large-scale orientation in the open ocean. Shoreward swimming during the larval phase is a relatively simple behavior that the existence of such a compass would enable. By limiting the loss of individuals in the open sea at the end of the larval phase, it would systematically increase survival and should therefore be strongly selected for, given the intense mortality experienced by fish larvae (Houde, 2008). More complex behaviors, such as orientation corrected for the drift due to currents or exploiting current structures (eddies, shears, etc.), would be even more favorable, but the sensory mechanisms and cognitive requirements involved are still unexplored. To compute the plausible influence of directional swimming on advection trajectories based on the current state of knowledge, swimming was aimed at the coastal polygon closest to the larva at each time step (i.e., without course correction). Orientation precision was set higher for large Sparidae (k = 4) compared to small Sparidae (k = 3.5). The values of k were estimated by fitting Von Mises distributions to the orientation bearings recorded for the two groups in Faillettaz et al., 2015).
Data Analysis
For each simulation, the proportion of successful settlers was computed as the number of virtual larvae that reached a coastal polygon during their competency phase divided by 540,000 (the number of larvae released). The proportion of settlement per MPA was computed the same way but considering only larvae reaching MPA polygons, i.e., polygons within 3 km of an MPA, to account for the fact that the resolution of the hydrodynamic model (~1.2 km) is not sufficient to describe local scale fluid dynamics.
The retention rate was defined as the number of larvae that were released from an MPA and settled in that same MPA's polygons, divided by the number of larvae released from that MPA. The self-recruitment rate was computed with the same numerator (number of particles that originated from and settled in the MPA) but divided by the total number of larvae that settled in the MPA, regardless their origin.
Dispersal kernels were computed as the probability density function of the great circle distance between the release MPA and the settlement location of successful settlers. The probability density function was estimated with a Gaussian smoothing kernel.
Results
Most virtual larvae as advected outside the domain, although small Sparidae do not move as far from their release sites as large Sparidae (Figure 2), simply because their pelagic phase is shorter. The overall proportion of successful settlers was strongly increased by the inclusion of larval behavior, for both groups of Sparidae (Figure 3): 16.3% (passive) vs. 28.4% (active) for small Sparidae and 1.2% (passive) vs. 10.2% (active) for large Sparidae. It was also always higher for small Sparidae compared to large Sparidae. However, the relative change induced by the inclusion of behavior was larger for large Sparidae: × 1.7 for small Sparidae vs. × 8.7 for large ones.
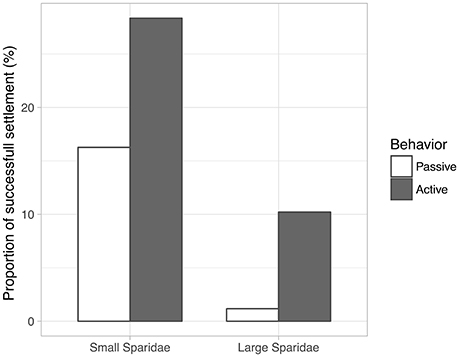
Figure 3. Proportion of virtual larvae that successfully reached a coastal habitat during their competency phase.
The proportion of virtual larvae that settled within any of the three MPA was low, with a maximum of 1.18% for passive small Sparidae in Larvotto and a minimum of 0.01% for passive large Sparidae in Port-Cros (Figure 4). For small Sparidae, the inclusion of larval behavior decreased the proportion of settlement by about half in Larvotto and Cap Roux, while it tripled it in Port-Cros. For large Sparidae, active swimming yielded at least a five-fold increase in the proportion of settlement in any of the three MPAs (Figure 4).
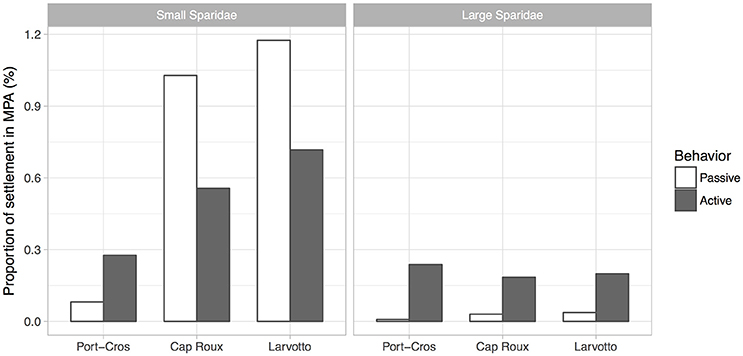
Figure 4. Proportion of virtual larvae that successfully reached a coastal habitat within an MPA during their competency phase.
Since the overall recruitment rates in MPAs were low, retention rates within each MPA were also low (<1%; Figure 5). However, including larval behavior markedly increased retention, except for small Sparidae in Cap Roux. Unsurprisingly, this higher retention of active larvae translated into higher self-recruitment rates, while the lower retention of small Sparidae in Cap Roux translated into a lower self-recruitment rate. However, an interesting pattern occurs for large Sparidae in Larvotto: active swimming yields higher retention but lower self-recruitment proportions. This means that the settlement of larvae from both Larvotto itself and the other MPAs increased when larvae were swimming, but the import of larvae from Cap Roux and Port-Cros increased more than the retention from Larvotto.
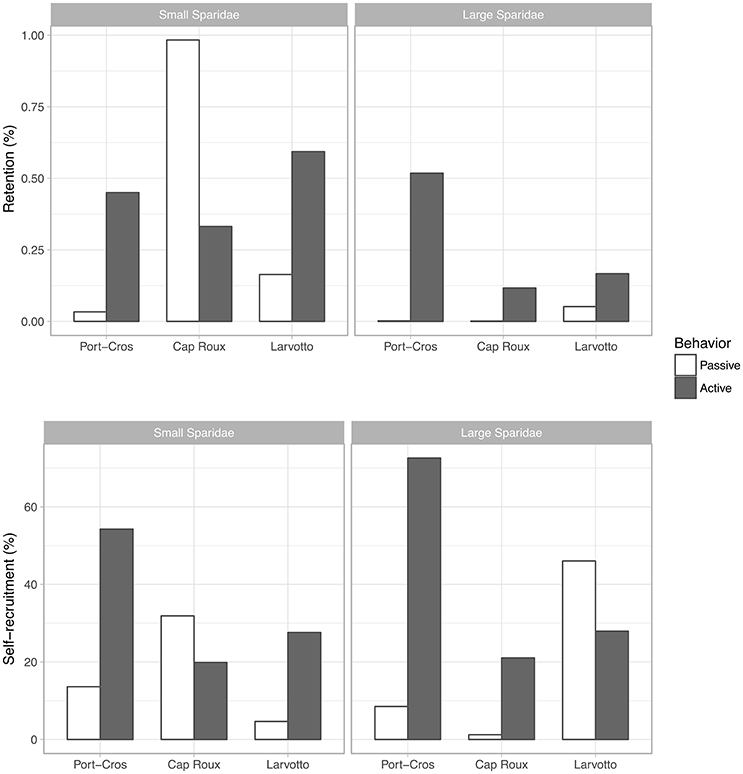
Figure 5. Retention rate (i.e., number of virtual larvae that originated from and settled in a given MPA divided by the number of virtual larvae that were released from that MPA) and self-recruitment rate (i.e., same as self-retention but divided by the number of virtual larvae that successfully settled in the MPA) for each release MPA and group of Sparidae.
Dispersal kernels show relatively few differences between simulations (Figure 6), with a consistent peak in larval supply to coastal habitat centered around 180–200 km away from the release MPA. Sill, passive small Sparidae displayed the lowest mean dispersal distance (141.1 ± 64.2 km; median = 146.2 km), followed by active small Sparidae (147.2 ± 71.4; median = 157.1 km), then active large Sparidae (149.5 ± 76.3; median = 167.2 km), and finally passive large Sparidae dispersed furthest (156.9 ± 67.2 km; median = 176.1 km). All kernels were statistically different from each other (Kolmogorov-Smirnoff tests, all D = [0.04–0.13], all p < 0.001). In the passive case, the shorter distances (to the left of the peak) are observed more often for small Sparidae compared to large ones. Conversely, larger dispersal distances are more often reached by large Sparidae than by small ones. The inclusion of larval behavior slightly increased larval supply within the closest 100 km for large Sparidae and circa 230 km for small Sparidae (Figure 6).
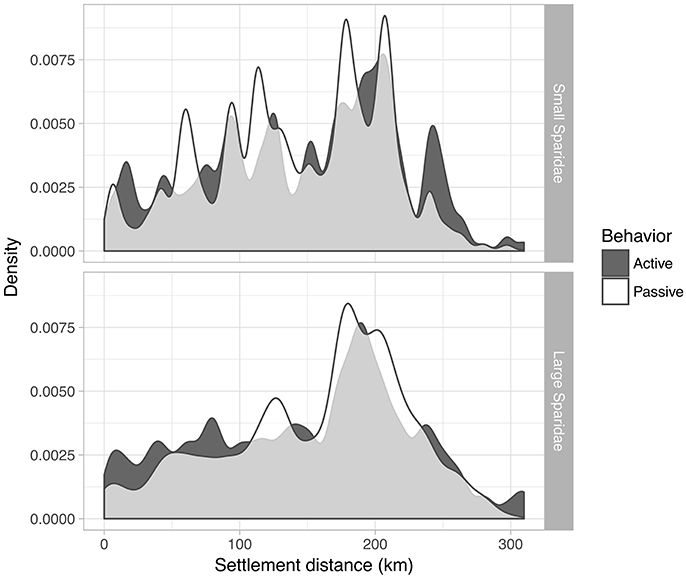
Figure 6. Dispersal kernels for the two groups of Sparidae and the two simulation configurations, i.e., kernel density smoothing of distances from the release site to the settlement site.
Beyond those differences in terms of dispersal kernels, including larval behavior substantially increased the number and intensity of connections throughout the Ligurian Sea (Figure 7). It is noteworthy that the three MPAs have the potential to export larvae of large Sparidae throughout the region. Fewer connections occur for small Sparidae compared to large Sparidae, but the intensity of those connections is generally higher, echoing the overall higher proportion of settlement of small Sparidae. In the passive simulations, the eastern region (Italian coast) received fewer larvae from the three MPAs considered than the rest of the domain (Figure 7). This pattern that was not as pronounced when behavior was added.
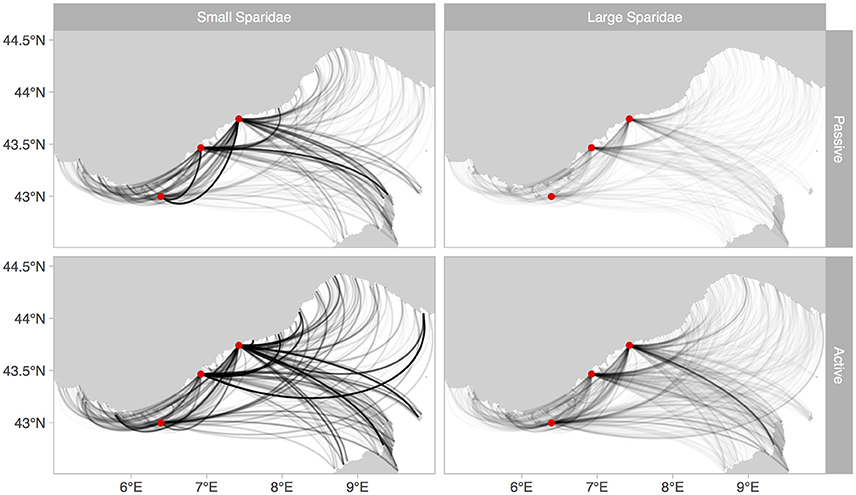
Figure 7. Connectivity patterns throughout the Ligurian Sea, from the three release MPA (red dots). Lines represent connections, not dispersal trajectories. Their intensity is proportional to the intensity of the connections.
Discussion
Generalization of the Present Results
We used a relatively simple example, based on a network of three French MPAs located in the Ligurian Sea, a part of the northwestern Mediterranean Sea, to reveal the potential differences in dispersal outcome that may be expected when accounting for oriented swimming by fish larvae.
We observed an increase in the overall proportion of settlement (Figure 3). This is intuitive since virtual larvae were parameterized to swim toward coastal habitats. All other things being equal, one would expect an increase in settlement rate proportional to the swimming speed (Faillettaz et al., 2017). More surprising however, is the lower proportion of large Sparidae that reached coastal habitats compared to small Sparidae, while they swam about twice as fast (10 cm s−1 at settlement vs. 6 cm s−1 for small Sparidae). The settlement rate of large Sparidae was even smaller compared to that of small Sparidae when larvae drifted passively. This difference is therefore probably attributable to the difference in PLD (13–19 d for small Sparidae vs. 29–38 d for large ones). Indeed, virtual larvae with a longer PLD may drift further away from their hatching site, which reduces the probability of reaching a coastal settlement habitat before the end of their competency phase. This highlights the foremost importance of the PLD in controlling larval supply rates, as suggested by several studies (e.g., O'Connor et al., 2007; Andrello et al., 2013).
Species with short PLDs, like small Sparidae, should therefore not drift as far as species with long PLDs, such as large Sparidae. In passive simulations, this is confirmed by the shape of the dispersal kernels, which highlight more short dispersal distances for small Sparidae (Figure 6), and the higher retention rates of small Sparidae (Figure 5). However, including behavior completely changed these retention patterns, indicating that retention is mostly determined by larval behavior rather than advection.
The fact that even the fastest-swimming larvae settled in relatively low numbers suggest that their swimming abilities are not sufficient to fully counteract the advection by oceans currents, when they drift too far offshore. Yet, considering their behavior still lead to a 9-fold increase in the overall proportion of settlement, for large Sparidae. This suggests that the relatively simple swimming behavior implemented in this model would be a much more favorable strategy in the face of natural selection, than randomly drifting with the flow.
In terms of connectivity patterns, active swimming increased the number and intensity of connections throughout the region, and in particular toward Corsica and the Italian coast (Figure 7). The lower intensity of connections to these regions in the passive case can be explained by the persistent Ligurian current, which transports particles mostly westward, at a fast pace, limiting eastward and southward connections. Our results suggest that, in this configuration of the coastline, larval behavior would favor connectivity at regional scale, in all directions. Accounting only for the general flow direction may thus not properly represent the main patterns of spillover.
We can compare the dispersal patterns of passively drifting larvae simulated here to the results of Andrello et al. (2013), who modeled the dispersal of Epinephelus marginatus (dusky grouper) among MPAs located throughout the Mediterranean Sea. They included the MPA of Port-Cros and set the PLD of E. marginatus to 30 d, which is in the range of PLDs considered for the large Sparidae we modeled (29–38 d). In the study by Andrello et al., self-recruitment accounted for most of the settlement, across all MPAs, but the MPA of Port-Cros was one of the six MPAs (over 99) that presented zero self-recruitment. Based on our passive simulation, we might also have concluded that Port-Cros may acts exclusively as a source MPA (Figure 5), but we actually showed that it becomes the MPA with the highest potential of self-recruitment rate when larval behavior is added, hence reversing the initial conclusion.
In summary, we found that including larval behavior in the model has (i) increased the overall proportion of successful settlers, (ii) enhanced self-recruitment within the MPAs, but also (iii) increased the intensity and (iv) widened the potential export of propagules from the MPAs. In line with previous modeling studies that focused on behavior (e.g., Paris et al., 2007; Staaterman et al., 2012; Wolanski and Kingsford, 2014), our results show the importance of exploring the potential effect of larval behavior when predicting connectivity patterns. Given the diversity of patterns detected here, which echoes that of other studies (e.g., Paris et al., 2007), the influence of larval behavior should definitely not be generalized as solely reducing the scales of connectivity, as it has been regularly suggested (Sponaugle et al., 2002; Andrello et al., 2013).
Toward More Accurate Connectivity Models in the Mediterranean Sea
Biophysical dispersal models have become a common tool for management and planning purposes. Yet, most still omit larval behavior, while this study adds to the evidence that larvae swimming at even just a few cm s−1 may substantially influence the outcomes of the pelagic phase (Werner et al., 1993; Leis, 2007; Vikebø et al., 2007; Staaterman et al., 2012; Wolanski and Kingsford, 2014; Faillettaz et al., 2017). But, to implement larval behaviors in a meaningful way, empirical data describing them is necessary.
Diel vertical migration behavior is well documented in fish larvae, including in the Mediterranean (Olivar and Sabatés, 1997; Sabates, 2004; Sabatés et al., 2010). Mediterranean coastal fish assemblages are dominated by perciformes and all perciformes larvae studied so far have been shown to swim at high, sustained speeds (Leis, 2006). In addition, it seems that their morphology could provide information on their swimming abilities in the absence of data on the species of interest (Fisher and Hogan, 2007; Faillettaz et al., 2017). Finally, swimming speeds achievable early during ontogeny can be somewhat inferred from swimming abilities during the installation phase, which is easier to study (Leis, 2010). Information on the swimming abilities of Mediterranean species is scarce (see Faillettaz et al., 2017 for a complete account) but estimations of their orientation abilities are even more so (Faillettaz et al., 2015 is the only study so far), and this is actually true all over the world (Leis, 2006). Yet, oriented swimming is likely to have a major impact on dispersal, especially if it occurs early in the larval phase (Staaterman et al., 2012).
Additional data on swimming abilities of young larvae and orientation abilities throughout ontogeny would therefore be the most critical to collect currently. When no data is available, the bias associated with their absence could be estimated by comparing dispersal patterns obtained from passive simulations and from simulations of caricatured behaviors (e.g., swimming at high speed and perfectly oriented), as part of a sensitivity study. North et al. (2009) provide suggestions for the implementation of such sensitivity approaches and Basterretxea et al. (2013) is an example of such a study in the Mediterranean.
Another essential criterion for the correct use of Lagrangian models is whether the spatial resolution of the underlying oceanographic model and the time step for the integration of the Lagrangian trajectories are adapted to the questions asked. In the present study, the grid of the hydrodynamic model (~1.2 km) could not resolve coastal patterns. Therefore, we did not attempt to represent them in the biological model and considered that larvae were able to reach the coast when they arrived within two kilometers of it (i.e., ~1.5 grid points). Overall, we interpret the predicted patterns of connectivity at scales of tens to hundreds of kilometers, for which the resolution of 1.2 km is not limiting. In contrast, to answer similar questions (regional connectivity and rates of larval retention), other studies used much lower resolutions (e.g., >12 km; Andrello et al., 2013, 2015) or integration time steps allowing larvae to cross more than one grid cell in a single step (Basterretxea et al., 2013). Such use of unsuitable configurations could skew the dispersal patterns obtained (North et al., 2009). To avoid these biases and better address near-shore, small-scale circulation processes, which are essential for egg dispersal and, perhaps, the installation of late larval stages, hydrodynamic models with higher resolution, especially near the coasts, are essential. Near-shore environment (e.g., bathymetry, habitat, etc.) may also affect larval fish sensitivity to coastal cues and considering these processes may help in further improving biophysical models.
Patterns of dispersal are also strongly influenced by the precise location and date of spawning (e.g., Guizien et al., 2012). However, in the North-Western Mediterranean Sea, the spawning areas of Sparidae, for example, are poorly known. We had to assume that they spawned along the coast, where they live. Their settlement and preferential recruitment habitats are better documented but often very restricted spatially (Vigliola et al., 1998; Cheminee et al., 2011). For example, around Marseille (France), the settlement habitat of the genus Diplodus could be limited to as little as 9% of the coastline. Nevertheless, this level of detail in the definition of settlement locations is not usually represented in dispersal models because accurate habitat mapping at the scale relevant to the models is not available (but see Holstein et al., 2014 for an example in the Caribbean Sea). Here, we again had to assume that suitable habitat, although possibly scarce, was uniformly distributed along the coast. Mapping of coastal habitats is currently being conducted in the Ligurian Sea (Meidinger et al., 2013) and may allow to refine future connectivity models in the region.
Finally, the results presented in this study show that the success of the pelagic larval phase is governed by both oceanographic processes and suite of biological characteristics (swimming speed, orientation accuracy, etc.). However, the unitary effect of each behavior on larval dispersal has rarely been explicitly evaluated (Werner et al., 1993; Paris et al., 2007 are two, rare, examples). It would therefore be interesting to compare a model simulating all observed larval behaviors with degraded versions in which behaviors are removed one by one, in order to quantify their respective effects and determine the importance of including them in connectivity models. Such a sensitivity analysis could effectively guide the collection of empirical data on the behavior of fish larvae in the future and, ultimately, improve the reliability of biophysical models. It would be particularly relevant in the context of the necessary improvement of the network of marine protected areas required by the European Union. Indeed, describing connectivity through the simulated dispersal of propagules is becoming a usual tool for the management and conservation of marine resources, particularly for the establishment of new protected (or urbanized) areas. Nevertheless, such approach may lead to the wrong conclusions if the biological components are not accurately parameterized because empirical data is lacking.
Author Contributions
RF, CP, and J-OI designed the modeling experiment. RF and J-OI run the simulations. RF analyzed the data and wrote the manuscript. CP and J-OI revised the manuscript.
Conflict of Interest Statement
The authors declare that the research was conducted in the absence of any commercial or financial relationships that could be construed as a potential conflict of interest.
Acknowledgments
This work was supported by a grant from the Partner University Fund to J-OI and CP. The CMS code development and maintenance is possible with a National Science Foundation award to CP (n°1260424). RF's doctoral fellowship was provided by the French Ministry of Education and Research (n°247/2012).
References
Almany, G. R., Planes, S., Thorrold, S. R., Berumen, M. L., Bode, M., Saenz-Agudelo, P., et al. (2017). Larval fish dispersal in a coral-reef seascape. Nat. Ecol. Evol. 1:148. doi: 10.1038/s41559-017-0148
Andrello, M., Mouillot, D., Beuvier, J., Albouy, C., Thuiller, W., and Manel, S. (2013). Low connectivity between Mediterranean marine protected areas: a biophysical modeling approach for the dusky grouper Epinephelus marginatus. PLoS ONE 8:e68564. doi: 10.1371/journal.pone.0068564
Andrello, M., Mouillot, D., Somot, S., Thuiller, W., and Manel, S. (2015). Additive effects of climate change on connectivity between marine protected areas and larval supply to fished areas. Divers. Distrib. 21, 139–150. doi: 10.1111/ddi.12250
Basterretxea, G., Catalán, I. A., Jordi, A., Álvarez, I., Palmer, M., and Sabatés, A. (2013). Dynamic regulation of larval fish self-recruitment in a marine protected area. Fish. Oceanogr. 22, 477–495. doi: 10.1111/fog.12035
Basterretxea, G., Jordi, A., Catalán, I. A., and Sabatés, A. N. A. (2012). Model-based assessment of local-scale fish larval connectivity in a network of marine protected areas. Fish. Oceanogr. 21, 291–306. doi: 10.1111/j.1365-2419.2012.00625.x
Berenshtein, I., Kiflawi, M., Shashar, N., Wieler, U., Agiv, H., and Paris, C. B. (2014). Polarized light sensitivity and orientation in coral reef fish post-larvae. PLoS ONE 9:e88468. doi: 10.1371/journal.pone.0088468
Béthoux, J. P., and Prieur, L. (1983). Hydrologie et circulation en méditerranée nord-occidentale. Pétroles et Techniques 299, 25–34.
Botsford, L. W., White, J. W., Coffroth, M. A., Paris, C. B., Planes, S., Shearer, T. L., et al. (2009). Connectivity and resilience of coral reef metapopulations in marine protected areas: matching empirical efforts to predictive needs. Coral Reefs 28, 327–337. doi: 10.1007/s00338-009-0466-z
Bottesch, M., Gerlach, G., Halbach, M., Bally, A., Kingsford, M. J., and Mouritsen, H. (2016). A magnetic compass that might help coral reef fish larvae return to their natal reef. Curr. Biol. 26, R1266–R1267. doi: 10.1016/j.cub.2016.10.051
Calò, A., Di Franco, A., De Benedetto, G. E., Pennetta, A., Pérez-Ruzafa, Á., and García-Charton, J. A. (2016). Propagule dispersal and larval patch cohesiveness in a Mediterranean coastal fish. Mar. Ecol. Prog. Ser. 544, 213–224. doi: 10.3354/meps11609
Catalán, I. A., Macías, D., Solé, J., Ospina-Álvarez, A., and Ruiz, J. (2013). Stay off the motorway: resolving the pre-recruitment life history dynamics of the European anchovy in the SW Mediterranean through a spatially-explicit individual-based model (SEIBM). Prog. Oceanogr. 111, 140–153. doi: 10.1016/j.pocean.2013.02.001
Cheminee, A., Francour, P., and Harmelin-Vivien, M. (2011). Assessment of Diplodus spp. (Sparidae) nursery grounds along the rocky shore of Marseilles (France, NW Mediterranean). Sci. Mar. 75, 181–188. doi: 10.3989/scimar.2011.75n1181
Clark, D. L., Leis, J. M., Hay, A. C., and Trnski, T. (2005). Swimming ontogeny of larvae of four temperate marine fishes. Mar. Ecol. Prog. Ser. 292, 287–300. doi: 10.3354/meps292287
Codling, E. A., Hill, N. A., Pitchford, J. W., and Simpson, S. D. (2004). Random walk models for the movement and recruitment of reef fish larvae. Mar. Ecol. Prog. Ser. 279, 215–224. doi: 10.3354/meps279215
Cowen, R., Gawarkiewicz, G., Pineda, J., Thorrold, S., and Werner, F. (2007). Population connectivity in marine systems: an overview. Oceanog 20, 14–21. doi: 10.5670/oceanog.2007.26
Cowen, R. K., Paris, C. B., and Srinivasan, A. (2006). Scaling of connectivity in marine populations. Science 311, 522–527. doi: 10.1126/science.1122039
Cresci, A., Paris, C. B., Durif, C. M. F., Shema, S., Bjelland, R. M., Skiftesvik, A. B., et al. (2017). Glass eels (Anguilla anguilla) have a magnetic compass linked to the tidal cycle. Sci. Adv. 3:e1602007. doi: 10.1126/sciadv.1602007
Di Franco, A., Calò, A., Pennetta, A., De Benedetto, G., Planes, S., and Guidetti, P. (2015). Dispersal of larval and juvenile seabream: implications for Mediterranean marine protected areas. Biol. Conserv. 192, 361–368. doi: 10.1016/j.biocon.2015.10.015
Di Franco, A., Coppini, G., Pujolar, J. M., De Leo, G. A., Gatto, M., Lyubartsev, V., et al. (2012). Assessing dispersal patterns of fish propagules from an effective mediterranean marine protected area. PLoS ONE 7:e52108. doi: 10.1371/journal.pone.0052108
Faillettaz, R. (2015). Estimation des Capacités Comportementales des Larves de Poissons et Leurs Implications Pour la Phase Larvaire?: Un cas d'étude d'espèces Démersales de Méditerranée Nord-Occidentale. Ph.D. thesis, Biodiversité et Ecologie, Université Pierre et Marie Curie - Paris VI, Paris.
Faillettaz, R., Blandin, A., Paris, C. B., Koubbi, P., and Irisson, J.-O. (2015). Sun-compass orientation in mediterranean fish larvae. PLoS ONE 10:e0135213. doi: 10.1371/journal.pone.0135213
Faillettaz, R., Durand, E., Paris, C. B., Koubbi, P., and Irisson, J.-O. (2017). Swimming speeds of Mediterranean settlement-stage fish larvae nuance Hjort's aberrant drift hypothesis. Limnol. Oceanogr.63, 509–523. doi: 10.1002/lno.10643
Faria, A. M., Ojanguren, A. F., Fuiman, L. A., and Gonçalves, E. J. (2009). Ontogeny of critical swimming speed of wild-caught and laboratory-reared red drum Sciaenops ocellatus larvae. Mar. Ecol. Prog. Ser. 384, 221–230. doi: 10.3354/meps08018
Fiksen, Ø., Jørgensen, C., Kristiansen, T., Vikeb, Ø, F., and Huse, G. (2007). Linking behavioural ecology and oceanography: larval behaviour determines growth, mortality and dispersal. Mar. Ecol. Prog. Ser. 347, 195–205. doi: 10.3354/meps06978
Fisher, R., Bellwood, D. R., and Job, S. D. (2000). Development of swimming abilities in reef fish larvae. Mar. Ecol. Prog. Ser. 202, 163–173. doi: 10.3354/meps202163
Fisher, R., and Hogan, J. D. (2007). Morphological predictors of swimming speed: a case study of pre-settlement juvenile coral reef fishes. J. Exp. Biol. 210, 2436–2443. doi: 10.1242/jeb.004275
Gaines, S. D., Gaylord, B., Gerber, L. R., Hastings, A., and Kinlan, B. (2007). Connecting places: the ecological consequences of dispersal in the sea. Oceanography 20, 90–99. doi: 10.5670/oceanog.2007.32
Galarza, J. A., Carreras-Carbonell, J., Macpherson, E., Pascual, M., Roques, S., Turner, G. F., et al. (2009). The influence of oceanographic fronts and early-life-history traits on connectivity among littoral fish species. Proc. Natl. Acad. Sci. U.S.A. 106, 1473–1478. doi: 10.1073/pnas.0806804106
Gerlach, G., Atema, J., Kingsford, M. J., Black, K. P., and Miller-Sims, V. (2007). Smelling home can prevent dispersal of reef fish larvae. Proc. Natl. Acad. Sci. U.S.A. 104, 858–863. doi: 10.1073/pnas.0606777104
Guizien, K., Belharet, M., Marsaleix, P., and Guarini, J. M. (2012). Using larval dispersal simulations for marine protected area design: application to the Gulf of Lions (Northwest Mediterranean). Limnol. Oceanogr. 57, 1099–1112. doi: 10.4319/lo.2012.57.4.1099
Holstein, D. M., Paris, C. B., and Mumby, P. J. (2014). Consistency and inconsistency in multispecies population network dynamics of coral reef ecosystems. Mar. Ecol. Prog. Ser. 499, 1–18. doi: 10.3354/meps10647
Houde, E. D. (2008). Emerging from Hjort's Shadow. J. Northw. Atl. Fish. Sci. 41, 53–70. doi: 10.2960/J.v41.m634
Johnson, D. R., Perry, H. M., and Lyczkowski-Shultz, J. (2013). Connections between campeche bank and red snapper populations in the Gulf of Mexico via modeled larval transport. Trans. Am. Fish. Soc. 142, 50–58. doi: 10.1080/00028487.2012.720630
Jones, G. P., Milicich, M. J., Emslie, M. J., and Lunow, C. (1999). Self-recruitment in a coral reef fish population. Nature 402, 802–804. doi: 10.1038/45538
Jones, G. P., Planes, S., and Thorrold, S. R. (2005). Coral reef fish larvae settle close to home. Curr. Biol. 15, 1314–1318. doi: 10.1016/j.cub.2005.06.061
Kinlan, B. P., and Gaines, S. D. (2003). Propagule dispersal in marine and terrestrial environments: a community perspective. Ecology 84, 2007–2020. doi: 10.1890/01-0622
Koeck, B., Gérigny, O., Durieux, E. D. H., Coudray, S., Garsi, L.-H., Bisgambiglia, P.-A., et al. (2015). Connectivity patterns of coastal fishes following different dispersal scenarios across a transboundary marine protected area (Bonifacio strait, NW Mediterranean). Estuar. Coast. Shelf Sci. 154, 234–247. doi: 10.1016/j.ecss.2015.01.010
Lazure, P., and Dumas, F. (2008). An external–internal mode coupling for a 3D hydrodynamical model for applications at regional scale (MARS). Adv. Water Resour. 31, 233–250. doi: 10.1016/j.advwatres.2007.06.010
Leis, J. M. (2006). Are larvae of demersal fishes plankton or nekton? Adv. Mar. Biol. 51, 57–141. doi: 10.1016/S0065-2881(06)51002-8
Leis, J. M. (2007). Behaviour as input for modelling dispersal of fish larvae: behaviour, biogeography, hydrodynamics, ontogeny, physiology and phylogeny meet hydrography. Mar. Ecol. Prog. Ser. 347, 185–193. doi: 10.3354/meps06977
Leis, J. M. (2010). Ontogeny of behaviour in larvae of marine demersal fishes. Ichthyol. Res. 57, 325–342. doi: 10.1007/s10228-010-0177-z
Leis, J. M., and Carson-Ewart, B. M. (1998). Complex behaviour by coral-reef fish larvae in open-water and near-reef pelagic environments. Environ. Biol. Fishes 53, 259–266. doi: 10.1023/A:1007424719764
Leis, J. M., and Clark, D. L. (2005). Feeding greatly enhances swimming endurance of settlement-stage reef-fish larvae of damselfishes (Pomacentridae). Ichthyol. Res. 52, 185–188. doi: 10.1007/s10228-004-0265-z
Leis, J. M., and Fisher, R. (2006). “Swimming speed of settlement-stage reef-fish larvae measured in the laboratory and in the field: a comparison of critical speed and in situ speed,” in Proceedings of the 10th International Coral Reef Symposium (Okinawa: Coral Reef Society of Japan Tokyo), 438–445.
Leis, J. M., Hay, A. C., Lockett, M. M., Chen, J., and Fang, L. (2007). Ontogeny of swimming speed in larvae of pelagic-spawning, tropical, marine fishes. Mar. Ecol. Prog. Ser. 349, 255–267. doi: 10.3354/meps07107
Leis, J. M., Paris, C. B., Irisson, J. O., Yerman, M. N., and Siebeck, U. E. (2014). Orientation of fish larvae in situ is consistent among locations, years and methods, but varies with time of day. Mar. Ecol. Prog. Ser. 505, 193–208. doi: 10.3354/meps10792
Leis, J. M., Siebeck, U. E., Hay, A. C., Paris, C. B., Chateau, O., and Wantiez, L. (2015). In situ orientation of fish larvae can vary among regions. Mar. Ecol. Prog. Ser. 537, 191–203. doi: 10.3354/meps11446
Macpherson, E., and Raventos, N. (2006). Relationship between pelagic larval duration and geographic distribution of Mediterranean littoral fishes. Mar. Ecol. Prog. Ser. 327, 257–265. doi: 10.3354/meps327257
Meidinger, M., Vasiliki, M., Sano, M., Palma, M., and Ponti, M. (2013). Seafloor mapping and cartography for the management of marine protected areas. Adv. Oceanogr. Limnol. 4, 120–137. doi: 10.1080/19475721.2013.848529
Miller, T. J. (2007). Contribution of individual-based coupled physical–biological models to understanding recruitment in marine fish populations. Mar. Ecol. Prog. Ser. 347, 127–138. doi: 10.3354/meps06973
Mouillot, D., Albouy, C., Guilhaumon, F., Ben Rais Lasram, F., Coll, M., Devictor, V., et al. (2011). Protected and threatened components of fish biodiversity in the Mediterranean sea. Curr. Biol. 21, 1044–1050. doi: 10.1016/j.cub.2011.05.005
Mouritsen, H., Atema, J., Kingsford, M. J., and Gerlach, G. (2013). Sun compass orientation helps coral reef fish larvae return to their natal reef. PLoS ONE 8:e66039. doi: 10.1371/journal.pone.0066039
Nanninga, G. B., Saenz-Agudelo, P., Zhan, P., Hoteit, I., and Berumen, M. L. (2015). Not finding Nemo: limited reef-scale retention in a coral reef fish. Coral Reefs 34, 383–392. doi: 10.1007/s00338-015-1266-2
Nelson, J. S., Grande, T. C., and Wilson, M. V. H. (2016). Fishes of the World. New York, NY: John Wiley & Sons.
North, E. W., Gallego, A., Petitgas, P., Ådlandsvik, B., Bartsch, J., Brickman, D., et al. (2009). Manual of Recommended Practices for Modelling Physical - Biological Interactions During Fish Early Life. ICES Cooperative Research Report.
O'Connor, J., and Muheim, R. (2017). Pre-settlement coral-reef fish larvae respond to magnetic field changes during the day. J. Exp. Biol. 220, 2874–2877. doi: 10.1242/jeb.159491
O'Connor, M. I., Bruno, J. F., Gaines, S. D., Halpern, B. S., Lester, S. E., Kinlan, B. P., et al. (2007). Temperature control of larval dispersal and the implications for marine ecology, evolution, and conservation. Proc. Natl. Acad. Sci. U.S.A. 104, 1266–1271. doi: 10.1073/pnas.0603422104
Okubo, A. (1971). Oceanic diffusion diagrams. Deep Sea Res. Oceanogr. Abstr. 18, 789–802. doi: 10.1016/0011-7471(71)90046-5
Olivar, M. P., and Sabatés, A. (1997). Vertical distribution of fish larvae in the north-west Mediterranean Sea in spring. Mar. Biol. 129, 289–300. doi: 10.1007/s002270050169
Pairaud, I. L., Gatti, J., Bensoussan, N., Verney, R., and Garreau, P. (2011). Hydrology and circulation in a coastal area off Marseille: validation of a nested 3D model with observations. J. Mar. Syst. 88, 20–33. doi: 10.1016/j.jmarsys.2011.02.010
Paris, C. B., Atema, J., Irisson, J.-O., Kingsford, M., Gerlach, G., and Guigand, C. M. (2013). Reef odor: a wake up call for navigation in reef fish larvae. PLoS ONE 8:e72808. doi: 10.1371/journal.pone.0072808
Paris, C. B., Chérubin, L. M., and Cowen, R. K. (2007). Surfing, spinning, or diving from reef to reef: effects on population connectivity. Mar. Ecol. Prog. Ser. 347, 285–300. doi: 10.3354/meps06985
Paris, C. B., and Cowen, R. K. (2004). Direct evidence of a biophysical retention mechanism for coral reef fish larvae. Limnol. Oceanogr. 49, 1964–1979. doi: 10.4319/lo.2004.49.6.1964
Pedrotti, M. L., and Fenaux, L. (1992). Dispersal of echinoderm larvae in a geographical area marked by upwelling (Ligurian Sea, NW Mediterranean). Mar. Ecol. Prog. Ser. 87, 217–227. doi: 10.3354/meps087217
Pujolar, J. M., Schiavina, M., Di Franco, A., Melià, P., Guidetti, P., Gatto, M., et al. (2013). Understanding the effectiveness of marine protected areas using genetic connectivity patterns and Lagrangian simulations. Divers. Distrib. 19, 1531–1542. doi: 10.1111/ddi.12114
Raventós, N., and Macpherson, E. (2001). Planktonic larval duration and settlement marks on the otoliths of Mediterranean littoral fishes. Mar. Biol. 138, 1115–1120. doi: 10.1007/s002270000535
Sabates, A. (2004). Diel vertical distribution of fish larvae during the winter-mixing period in the Northwestern Mediterranean. ICES J. Mar. Sci. 61, 1243–1252. doi: 10.1016/j.icesjms.2004.07.022
Sabatés, A., and Olivar, M. P. (1996). Variation of larval fish distributions associated with variability in the location of a shelf-slope front. Mar. Ecol. Prog. Ser. 135, 11–20. doi: 10.3354/meps135011
Sabatés, A., Pagès, F., Atienza, D., Fuentes, V., Purcell, J. E., and Gili, J.-M. (2010). Planktonic cnidarian distribution and feeding of Pelagia noctiluca in the NW Mediterranean Sea. Hydrobiologia 645, 153–165. doi: 10.1007/s10750-010-0221-z
Simpson, S. D., Meekan, M., Montgomery, J., McCauley, R., and Jeffs, A. (2005). Homeward sound. Science 308:221. doi: 10.1126/science.1107406
Sponaugle, S., Cowen, R. K., Shanks, A., Morgan, S. G., Leis, J. M., Pineda, J., et al. (2002). Predicting self-recruitment in marine populations : biophysical correlates and mechanisms. Bull. Mar. Sci. 70, 341–375.
Sponaugle, S., Paris, C., Walter, K. D., Kourafalou, V., and D'Alessandro, E. (2012). Observed and modeled larval settlement of a reef fish to the Florida Keys. Mar. Ecol. Prog. Ser. 453, 201–212. doi: 10.3354/meps09641
Staaterman, E., and Paris, C. B. (2014). Modelling larval fish navigation: the way forward. ICES J. Mar. Sci. 71, 918–924. doi: 10.1093/icesjms/fst103
Staaterman, E., Paris, C. B., and Helgers, J. (2012). Orientation behavior in fish larvae: a missing piece to Hjort's critical period hypothesis. J. Theor. Biol. 304, 188–196. doi: 10.1016/j.jtbi.2012.03.016
Stemmann, L., Prieur, L., Legendre, L., Taupier-Letage, I., Picheral, M., Guidi, L., et al. (2008). Effects of frontal processes on marine aggregate dynamics and fluxes: an interannual study in a permanent geostrophic front (NW Mediterranean). J. Mar. Syst 70, 1–20. doi: 10.1016/j.jmarsys.2007.02.014
Teodósio, M. A., Paris, C. B., Wolanski, E., and Morais, P. (2016). Biophysical processes leading to the ingress of temperate fish larvae into estuarine nursery areas: a review. Estuar. Coast. Shelf Sci. 183, 187–202. doi: 10.1016/j.ecss.2016.10.022
Truelove, N. K., Kough, A. S., Behringer, D. C., Paris, C. B., Box, S. J., Preziosi, R. F., et al. (2017). Biophysical connectivity explains population genetic structure in a highly dispersive marine species. Coral Reefs 36, 233–244. doi: 10.1007/s00338-016-1516-y
Vigliola, L., Harmelin-vivien, M. L., Biagi, F., Galzin, R., Garcia-Rubies, A., Harmelin, J.-G., et al. (1998). Spatial and temporal patterns of settlement among sparid fishes of the genus Diplodus in the northwestern Mediterranean. Mar. Ecol. Prog. Ser. 168, 45–56. doi: 10.3354/meps168045
Vikebø, F., Jørgensen, C., Kristiansen, T., and Fiksen, Ø. (2007). Drift, growth, and survival of larval Northeast Arctic cod with simple rules of behaviour. Mar. Ecol. Prog. Ser. 347, 207–219. doi: 10.3354/meps06979
Werner, F. E., Page, F. H., Lynch, D. R., Loder, J. W., Lough, R. G., Perry, R. I., et al. (1993). Influences of mean advection and simple behavior on the distribution of cod and haddock early life stages on Georges Bank. Fish. Oceanogr. 2, 43–64. doi: 10.1111/j.1365-2419.1993.tb00120.x
Keywords: fish larvae, behavior, swimming, connectivity, dispersal, mediterranean sea, marine protected areas, modeling
Citation: Faillettaz R, Paris CB and Irisson J-O (2018) Larval Fish Swimming Behavior Alters Dispersal Patterns From Marine Protected Areas in the North-Western Mediterranean Sea. Front. Mar. Sci. 5:97. doi: 10.3389/fmars.2018.00097
Received: 21 December 2017; Accepted: 09 March 2018;
Published: 26 March 2018.
Edited by:
Stelios Katsanevakis, University of the Aegean, GreeceReviewed by:
Enrico Zambianchi, Consorzio Nazionale Interuniversitario per le Scienze del Mare (CoNISMa), ItalyPablo Saenz-Agudelo, Universidad Austral de Chile, Chile
Antonio Di Franco, University of Nice Sophia Antipolis, France
Copyright © 2018 Faillettaz, Paris and Irisson. This is an open-access article distributed under the terms of the Creative Commons Attribution License (CC BY). The use, distribution or reproduction in other forums is permitted, provided the original author(s) and the copyright owner are credited and that the original publication in this journal is cited, in accordance with accepted academic practice. No use, distribution or reproduction is permitted which does not comply with these terms.
*Correspondence: Robin Faillettaz, cm9iaW4uZmFpbGxldHRhekBnbWFpbC5jb20=