- 1Red Sea Research Center, King Abdullah University of Science and Technology, Thuwal, Saudi Arabia
- 2Global Change Research Group, Mediterranean Institute for Advanced Studies (IMEDEA, CSIC-UIB), Esporles, Spain
- 3Department of Bioscience, Aarhus University, Silkeborg, Denmark
Iron can limit primary production in shallow marine systems, especially in tropical waters characterized by carbonated sediments, where iron is largely trapped in a non-available form. The Red Sea, an oligotrophic ecosystem characterized by a strong N-S latitudinal nutrient gradient, is a suitable setting to explore patterns in situ of iron limitation in macrophytes and their physiological performance under different iron regimes. We assessed the interactions between environmental gradients and physiological parameters of poorly-studied Red Sea macrophytes. Iron concentration, chlorophyll a concentration, blade thickness, and productivity of 17 species of macrophytes, including seven species of seagrasses and 10 species of macroalgae, were measured at 21 locations, spanning 10 latitude degrees, along the Saudi Arabian coast. Almost 90% of macrophyte species had iron concentrations below the levels indicative of iron sufficiency and more than 40% had critically low iron concentrations, suggesting that iron is a limiting factor of primary production throughout the Red Sea. We did not identify relationships between tissue iron concentration, chlorophyll a concentration and physiological performance of the 17 species of seagrass and macroalgae. There was also no latitudinal pattern in any of the parameters studied, indicating that the South to North oligotrophication of the Red Sea is not reflected in iron concentration, chlorophyll a concentration or productivity of Red Sea macrophytes.
Introduction
Iron (Fe) is an essential nutrient that can act as a limiting factor controlling primary production in freshwater and marine systems (Coale et al., 1996; Sterner et al., 2004). In particular, iron limits phytoplankton production across vast spans of the open ocean (Martin et al., 1994; Welch and Shuman, 1995). Iron can also limit primary production in shallow marine systems, especially in carbonated sediments characterized by very low iron pools (Pestana et al., 2003). Observations and field experiments provide evidence of iron deficiency in seagrasses growing on carbonate sediments (Marbà et al., 2008), where iron, addition to sediments stimulates seagrass growth in the Yucatan Peninsula (Duarte et al., 1995), Southern Florida (Chambers et al., 2001), and the Balearic Islands (Marbà et al., 2007). Field observations on coral reefs, which are also carbonate-rich habitats, also suggest potential iron limitation in the growth of macroalgae, as indicated by higher growth rates of these macrophytes near ship wrecks, which leak iron in micronutrient-poor islands in the Central Pacific (Kelly et al., 2012).
Iron limits the growth of primary producers because it is required for the synthesis of photosynthetic pigments and the functioning of the key enzyme in photosynthesis, Rubisco (Winder and Nishio, 1995). As such, iron concentration has been positively linked to pigment concentration across many groups of primary producers (Geider et al., 1993; Abadía, 2008). In marine benthic habitats, experimental iron additions in plots of seagrasses growing on coralline carbonate sediments in the Caribbean increased the chlorophyll a (Chl a) concentration in seagrass leaves and enhanced seagrass growth (Duarte et al., 1995). Moreover, iron addition experiments show that the survival of seedlings and growth of young plants of the seagrass Zostera marina can be limited by iron availability (Wang et al., 2017). However, there is a paucity of studies in situ across a broad range of iron inputs to assess effects on primary production in marine benthic ecosystems (Marbà et al., 2008).
The Red Sea is a suitable location to describe patterns of primary production under different iron regimes. The Red Sea is an oligotrophic ecosystem characterized by a strong nutrient gradient, including potentially trace metals like iron, driven by the depletion of nutrients as the water entering the southern Red Sea from the Gulf of Aden flows north (Raitsos et al., 2015). This nutrient gradient is associated with a gradual decrease of Chl a concentration in phytoplankton from south to north of the Red Sea (Raitsos et al., 2013; Kürten et al., 2015). This gradient is especially prominent during the summer months and more diffuse during the winter, when wind-driven water-mixing replenishes the nutrients in the sea surface in the northern Red Sea (Sofianos and Johns, 2003). This gradient also affects primary production in the ubiquitous coral Pocillopora verrucosa (Sawall et al., 2015) and to a certain extent phytoplankton growth (Qurban et al., 2017). Thus, the Red Sea presents a unique environment to study the effect of iron concentration regimes in situ. Indeed, Almahasheer et al. (2016a) reported the dominant mangrove species Avicennia marina to be iron-limited in the Central Red Sea.
The Red Sea, with the exception of the Gulf of Aqaba, is grossly understudied when compared to other vast coral reef regions such as the Great Barrier Reef, the Coral Reef Triangle, the Caribbean Basin or the Hawaiian Archipelago. With a few exceptions (Almahasheer et al., 2016a,b,c), corals and phytoplankton are the main focus of research on primary producers in the Red Sea (Cantin et al., 2010; Ziegler et al., 2014; Kürten et al., 2015; Sawall et al., 2015; Roik et al., 2016; Qurban et al., 2017, among others). However, other important and uncharismatic habitat-forming macrophytes, such as seagrasses and macroalgae within coral reefs (Duarte et al., 2008), are common but poorly studied in the Red Sea.
Here we assessed patterns of iron concentration in macrophyte tissue and its association with macrophyte productivity, as a proxy of iron availability, along a latitudinal gradient in the Saudi Arabian Red Sea. Specifically, we sampled macrophytes from 12 seagrass meadows and 9 shallow corals reefs spanning nearly 10 latitudinal degrees (Figure 1). Our first goal was to describe tissue iron concentration, Chl a concentration, blade thickness, and physiological performance of seagrass and macroalgae species in the Red Sea and identify potential relationships between these variables. Our second goal was to test for a latitudinal pattern of physiological parameters in the Red Sea associated with tissue iron concentration regimes in macrophytes in general and, in particular, for two ubiquitous species, the seagrass Halophila stipulacea and the macroalgae Turbinaria ornata. We tested whether productivity was related with the blade thickness and Chl a concentration of the photosynthetic tissues, as has been reported for marine plants in general (Enriquez et al., 1995; Enríquez et al., 1996), and whether such potential relationships depended on iron concentration.
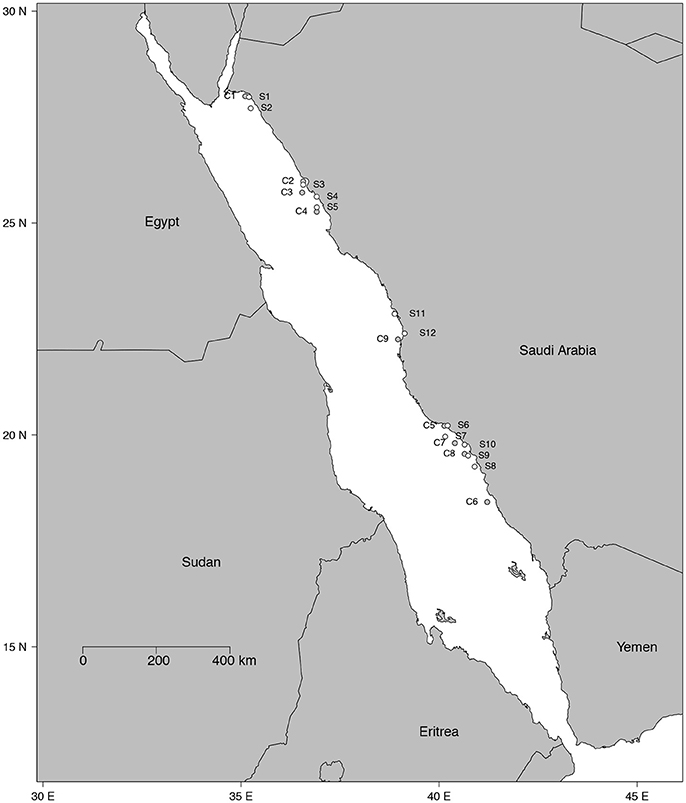
Figure 1. Map of the 21 study sites. White dots denote the 12 seagrass meadows (from S1 to S12) and gray dots indicate the 9 shallow coral reefs (from C1 to C9).
Materials and Methods
Study Sites and Species Information
Twenty-one study sites were surveyed along the Saudi Arabian coast of the Red Sea (Figure 1) between February 19th and April 10th 2016 from 18.4 to 27.9° latitude, covering a large range of environmental conditions. In situ temperature ranged from 22.6 to 29.5°C and salinity varied from 37.5 to 41.4 ppt (Table 1). Sites included 9 shallow coral reefs and 12 seagrass meadows growing at depths ranging between 1 and 8 m (Table 1). Specimens of the most common conspicuous species of macroalgae and seagrasses were collected at each study site snorkeling or diving. In total, we evaluated 10 species of macroalgae in coral reefs and seagrass meadows (Caulerpa racemosa, Caulerpa serrulata, Dictyosphaeria cavernosa, Halimeda tuna, Halymenia sp. Padina pavonica, Sargassum ilicifolium, Tydemania expeditionis, T. ornata, and Udotea flabellum) and seven species of seagrasses (Enhalus acoroides, Halodule uninervis, Halophila decipiens, Halophila ovalis, H. stipulacea, Thalassodendron ciliatum, and Thalassia hemprichii). Chl a concentration, thickness, and the maximum electron transport rate of photosystem II, ETRmax, a proxy of productivity, were assessed for most of the specimens collected (see Table 2 for specific number of replicates), after carefully rinsing them with seawater and removing the epiphytes. Thickness was measured on the blades of seagrasses and macroalgae using a Fisherbrand Traceable digital caliper. For irregular macroalgae, blade thickness was assessed on the photosynthetic unit (e.g., the diameter of the spheroids on C. racemosa or the height of the pyramidoid blades on T. ornata), as reported previously (Agustí et al., 1994). Iron concentration was measured in the tissues of the macrophytes (see Table 2 for specific number of replicates). Additional measurements of iron concentration were performed for T. ornata and H. stipulacea at five sites (C1, C3, C5, C7, C9, and S1, S3, S7, S10, S11 for T. ornata and H. stipulacea respectively, see Tables 1, 2) to perform more robust species-specific latitudinal analyses.
Chl a and Iron Concentration of Macrophytes
Chl a content and iron concentration of seagrass and macroalgae blades were quantified in the fragment of macrophyte where the ETRmax of photosystem II activity was performed (see Physiological Performance section below). Chl a concentration was obtained from a known amount of frozen tissue using a QIAGEN TissueLyser II and extracted overnight in 10 ml of acetone 80%. Pigment concentration was assessed spectrophotometrically by measuring absorbance at 646, 663, and 710 nm. First, absorbance (A) at each wavelength was subtracted from A of the blank following Wellburn (1994):
Then, the concentration of the Chl a was determined as follows (Wellburn, 1994):
We analyzed the iron concentration on the blades of seagrass and macroalgae to assess potential iron limitation in macrophytes since iron content is a robust indicator of the trace metal status of seagrasses (Duarte et al., 1995; Chambers et al., 2001). For small species of seagrasses (H. uninervis, H. decipiens, H. ovalis, and H. stipulacea) we collected four shoots per replicate and for large species (E. acoroides, T. ciliatum, and T. hemprichii) we collected the second-youngest leaf of four shoots for each replicate. Iron concentration in macroalgae was analyzed within a piece of blade.
Leaves and blades were dried at 60°C and ground using an agate mortar and pestle until powderized. Iron concentrations were determined after digesting 50–100 mg of the powderized tissue material with 5 ml of HNO3 (69%) and 1 ml of H2O2 (30%), using a 2 step temperature microwave heating protocol for 5 min and 10 min at 180°C. The samples were allowed to cool, then diluted with Mili-Q water and analyzed by Inductively Coupled Plasma-Optical Emission Spectrometry (Varian Inc. model 720-ES).
Physiological Performance
The measurement of the Chl a fluorescence is a powerful method of assessing the properties of the photosynthetic apparatus (Ralph and Gademann, 2005) and has been shown to be a good proxy for the productivity of macrophytes (Beer, 1998; Beer and Björk, 2000; Silva et al., 2005). Chl a fluorescence was assessed using an underwater Pulse Amplitude Modulated (PAM) shutter fluorometer (Aquation, Australia). Fluorescence measurements were performed in situ and soon after collection in intact specimens of macroalgae and seagrasses that were transferred to flow-through aquaria (122 × 50 × 40 cm) filled with water from the nearby study site and therefore kept at in situ salinity and temperature and under natural light conditions. The majority of the fluorescence measurements were performed in the aquaria (140 out of 196), due to time constrains in the field, and the rest (56 out of 196) were performed in situ. Statistical analyses (see Statistical Analysis section below) indicated no significant differences between the fluorescence measurements performed in the field and at the aquariums for several species of seagrasses at 6 locations (Table 3). For large seagrass species (E. acoroides, T. ciliatum, and T. hemprichii) all measurements took place above the meristem of the second leaf to reduce the effect of variability within leaves. Rapid light curves (RLCs; Ralph and Gademann, 2005) were quantified by applying a saturating pulse to specimens after dark-adaptation for 5 min. Samples were illuminated with a series of 12 increasing actinic light intensities (from 0 to 990 μmol photons m−2 s−1) at intervals of 10 s. At the end of each interval a saturating light pulse was applied (~2,800 μmol photons m−2 s−1) and the effective yield recorded. The absorbance (AF) of each species was measured by placing specimens in front of a PAR sensor and recording the percentage of light absorbed by the sample (Beer, 1998). Irradiance through 1 to 4 layers of leaves or blades was recorded in 3–5 replicates for each species and the ln of the recorded irradiance was then plotted against the number of layers. Using a linear correlation, the slope (α) of the resulting line was determined and AF calculated as 1–exp(–α).
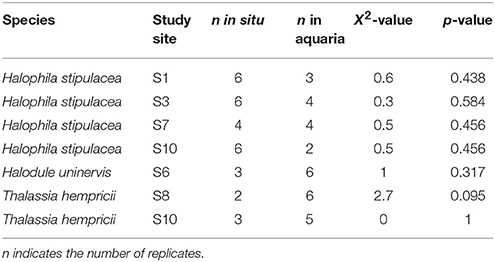
Table 3. Results of the Kruskal–Wallis rank test comparing ETRmax measurements in situ in the field and in aquaria.
The relative Electron Transport Rates (ETRr; μmol electrons m−2 s−1) from each RLC was calculated following Beer et al. (2001):
The maximum ETRr (ETRmax) was derived by fitting the RLCs to the equations defined by Eilers and Peeters (1988).
Statistical Analyses
We performed a Kruskal–Wallis rank test to determine differences between the fluorescence measures performed in situ in the field and in the aquaria, because sample sizes were unbalanced (Table 3). Because no significant differences were detected within species and locations (Table 3), we used indistinctly the ETRmax measurements from the field and the aquaria.
The relationships between ETRmax and iron concentration, Chl a concentration and blade thickness were tested using linear regression analysis for all species of seagrasses and macroalgae separately, using the mean of each species per site for the three variables. To assess the relationship between iron concentration and Chl a concentration in the macrophyte tissue, exponential regressions and linear regressions were fitted to describe the relationship between Chl a and blade thickness. We also performed linear regressions to describe the relationships between latitude and iron concentration, Chl a concentration and ETRmax for seagrasses or macroalgae species separately. The latitudinal patterns of H. stipulacea and T. ornata were analyzed using mixed-effects models with site as random factor using the nlme R package (Pinheiro et al., 2015), on which the information for each specimen at each location was included to strengthen the power of the analysis. All calculations, graphs and statistical analyses were performed using RStudio 0.99.491 (1.0.143) (©RStudio, Inc.).
Results
We found that seven species of macrophytes in the Red Sea, two seagrasses (T. hemprichii and T. ciliatum) and five macroalgae (C. serrulata, D. cavernosa, Halymenia sp. S. ilicifolium, and T. ornata), had average iron concentrations below 100 mg Fe kg DW−1 (Figure 2A, Table 4), considered to be the critical iron concentration for marine macrophytes (Duarte et al., 1995). Additionally, eight species in the Red Sea, including four seagrasses (E. acoroides, H. uninervis, H. ovalis, and H. stipulacea) and four macroalgae (C. racemosa, H. tuna, P. pavonica, and T. expeditionis), had average iron concentrations below 600 mg Fe kg DW−1 (Figure 2A, Table 4), which is still below iron sufficiency (Duarte et al., 1995). Only two species of macrophytes, the seagrass H. decipiens and the macroalgae U. flabellum, were iron sufficient with an average concentration of 621 and 906 mg Fe kg DW−1, respectively (Figure 2A, Table 4). In general, Chl a content was relatively homogeneous within different macrophyte taxa (Figure 2B), with seagrasses having consistently higher concentration of Chl a than macroalgae. Five species of seagrasses, H. decipiens, H. ovalis, H. stipulacea, H. uninervis, and T. ciliatum, had the highest Chl a concentrations while six species of macroalgae, C. racemosa, D. cavernosa, Halymenia sp. P. pavonica, T. ornata, and T. expeditionis, had the lowest Chl a concentrations (Figure 2B). ETRmax, a proxy for productivity, was intermediate for seagrasses, while the photophilic species of brown macroalgae (S. ilicifolium and T. ornata) exhibited high levels of ETRmax and the species of macroalgae that inhabit crevices (e.g., T. expeditionis and Halymenia sp.) had low ETRmax values (Figure 3A). Macrophyte thickness is also an important plant trait and we found it was primarily associated to taxonomic affiliation: Seagrass leaves were thinner than macroalgal blades with two species exceptions (e.g., P. pavonica and E. acoroides; Figure 3B).
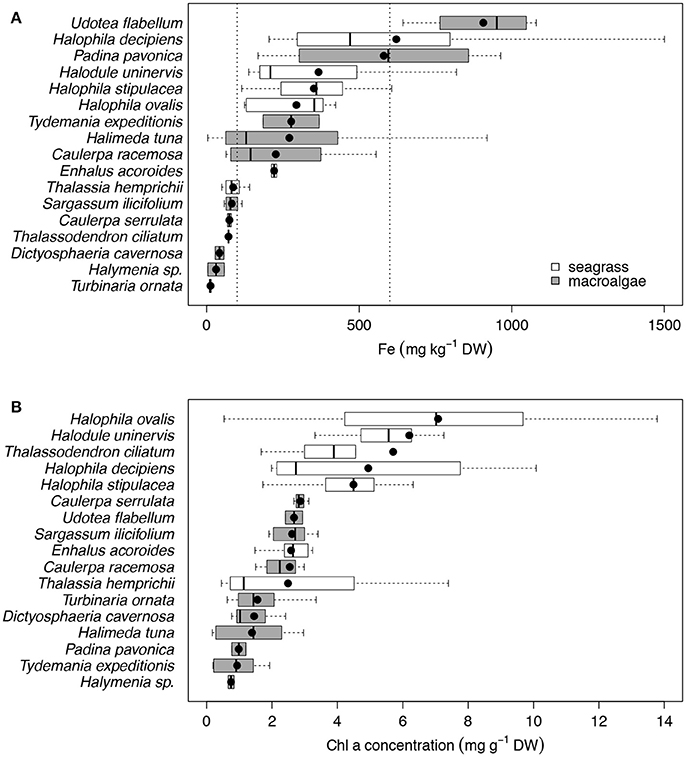
Figure 2. Box plots of the iron concentration (mg kg−1 DW; A) and the Chl a concentration (mg g−1 DW; B) for the 17 species of primary producers ordered by increasing mean values for the Red Sea, which are represented with black dots. Seagrass species are represented in white and macroalgae species in gray. Vertical dash lines indicate critical and sufficient iron concentrations at 100 and 600 mg Fe kg DW−1, respectively. The number of replicates is indicated in Table 2.
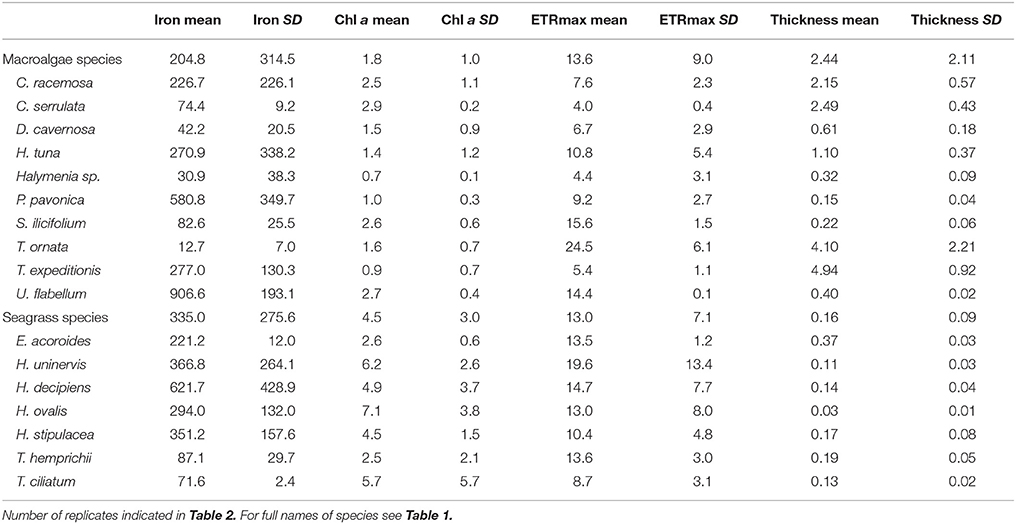
Table 4. Mean and SD of iron concentration (mg kg−1 DW), Chl a concentration (mg g−1 DW), ETRmax (μmol electrons m−2 s−1), and blade thickness (mm) by species.
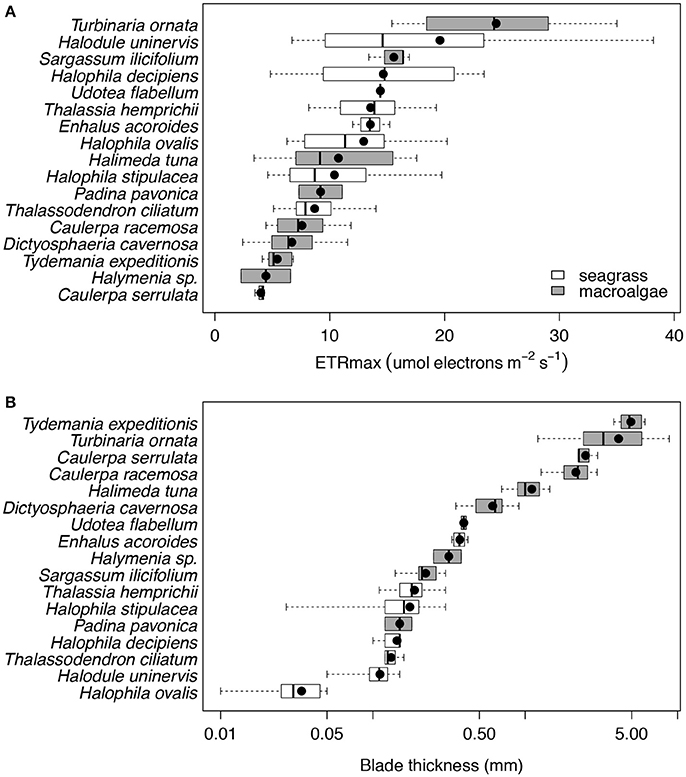
Figure 3. Box plots of the ETRmax (μmol electrons m−2 s−1; (A) and the blade thickness (mm; B) for the 17 species of primary producers ordered by increasing mean values for the Red Sea, which are represented with black dots. Seagrass species are represented in white and macroalgae species in gray. The number of replicates is indicated in Table 2.
None of the three plant traits examined, iron concentration, Chl a abundance and blade thickness, were related to macrophyte productivity (ETRmax) for seagrasses (linear regressions, p = 0.316, R2 = 0.056, p = 0.624, R2 = 0.014, p = 0.742, R2 = 0.006, respectively; Figure 4) or macroalgae (linear regressions, p = 0.286, R2 = 0.086, p = 0.765, R2 = 0.007, p = 0.189, R2 = 0.128, respectively; Figure 4). The concentration of iron in the photosynthetic tissues of the seagrass and macroalgae blades was unrelated to the concentration of Chl a when compared across all species (exponential regressions, p = 0.070, R2 = 0.170 for seagrasses, and p = 0.663, R2 = 0.015 for macroalgae; Figures 5A,B). The thickness of the seagrass leaves was negatively associated with the concentration of Chl a (linear regression, p = 0.017, R2 = 0.277; Figure 5B), but this relationship was not found in macroalgae blades (linear regression, p = 0.777, R2 = 0.006; Figure 5B).
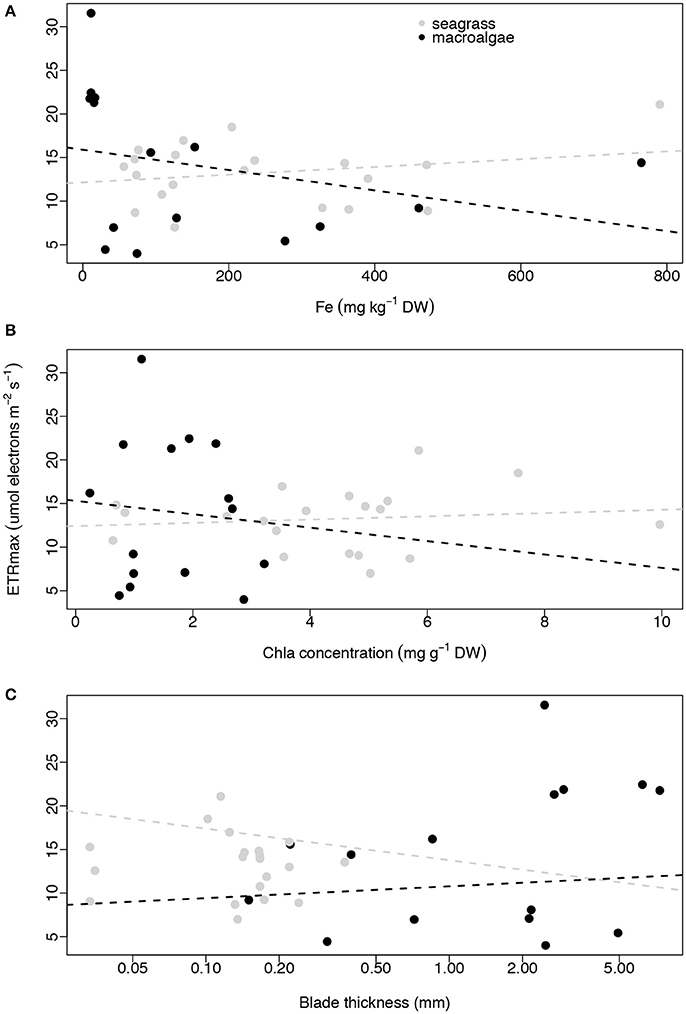
Figure 4. Relationships between average ETRmax (μmol electrons m−2 s−1) and average iron concentration (mg kg−1 DW; A), average Chl a concentration (mg g−1 DW; B) and average blade thickness (mm; C) per species per site. Seagrasses are depicted with gray dots and macroalgae with black dots. Non-significant regressions are indicated with a dash line. The number of replicates is indicated in Table 2.
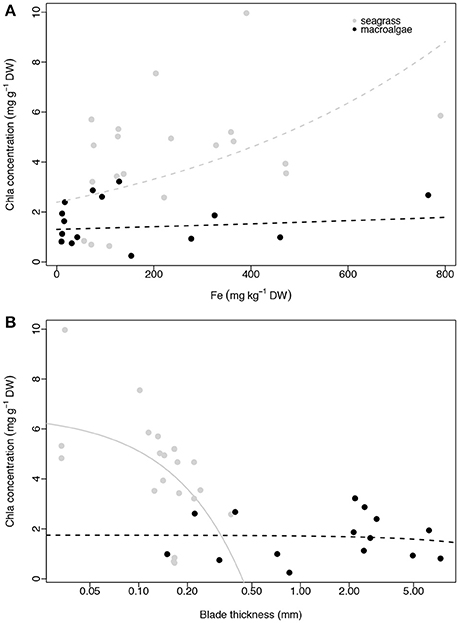
Figure 5. Relationship between average Chl a concentration (mg g−1 DW) and average iron concentration (mg kg−1 DW; A) and average blade thickness (mm; B) per species per site. Seagrasses are depicted with gray dots and macroalgae with black dots. Significant regressions (p < 0.05) are represented with a solid line and non-significant regressions are indicated with a dash line. The number of replicates is indicated in Table 2.
Contrary to our expectations, we found no relationship between latitude and iron concentration in the leaves of the seagrasses and macroalgae (linear regression, p = 0.958, R2 ≤ 0.001, and p = 0.627, R2 = 0.018, respectively; Figure 6A). Similar results were found for Chl a concentration and ETRmax for seagrasses and macroalgae along the latitudinal gradient (linear regression, p = 0.520, R2 = 0.023, and p = 0.387, R2 = 0.041 for seagrasses and p = 0.882, R2 = 0.002, and p = 0.856, R2 = 0.002 for macroalgae; Figures 6B,C).
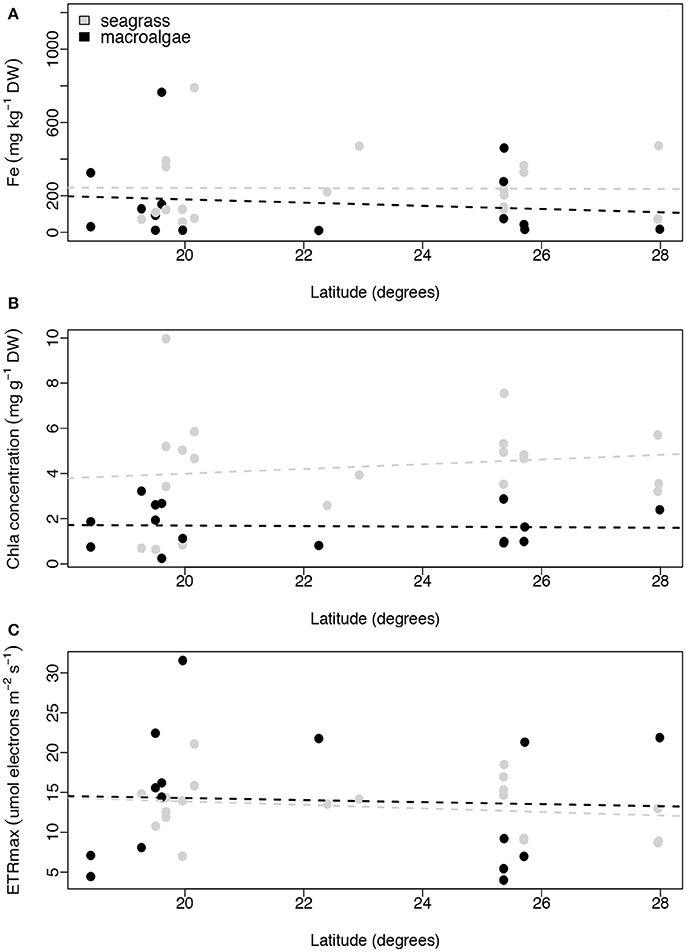
Figure 6. Relationships between latitude (degrees) and average iron concentration (mg kg−1 DW; A), average Chl a concentration (mg g−1 DW, B), and average ETRmax (μmol electrons m−2 s−1; C) per species per site. Non-significant regressions are indicated with a dash line. The number of replicates is indicated in Table 2.
We performed additional latitudinal analyses for the two most common species of macrophytes, the seagrass H. stipulacea and the macroalgae T. ornata. For both species we found no relationship between latitude and leaf iron concentration (linear regressions with site as random factor, p = 0.224 and p = 0.243, respectively, for the seagrass and macroalgae; Figures 7A,B), between latitude and leaf Chl a concentration (linear regressions with site as random factor, p = 0.114 and p = 0.296, respectively, for the seagrass and macroalgae; Figures 7C,D), or between latitude and ETRmax (linear regressions with site as random factor, p = 0.536 and p = 0.283 respectively for the seagrass and macroalgae; Figures 7E,F).
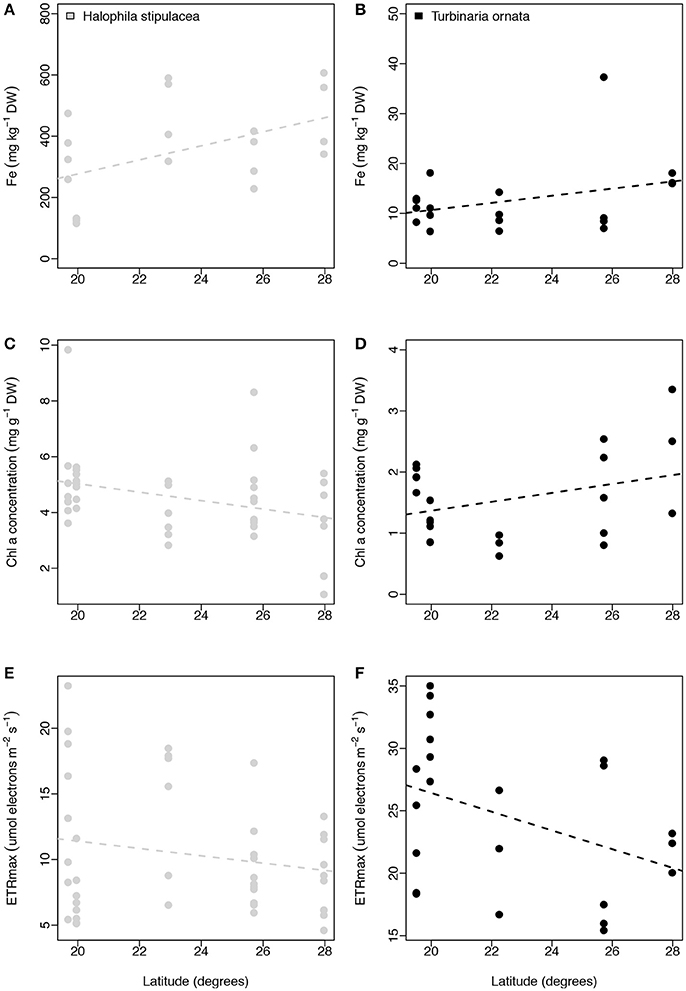
Figure 7. Relationships between latitude (degrees) and iron concentration (mg kg−1 DW, A,B), chl a concentration (mg g−1 DW, C,D), and ETRmax (μmol electrons m−2 s−1; E,F) for Halophila stipulacea (depicted with gray dots) and Turbinaria ornata (represented with black dots). Non-significant regressions are indicated with a dash line. The number of replicates is indicated in Table 2.
Discussion
Benthic macrophytes in the oligotrophic Red Sea were characterized by low iron concentration, with 86% of our seven species of seagrasses and 90% of our ten species of macroalgae having iron concentration below the levels indicative of iron sufficiency (600 mg Fe kg DW−1, Duarte et al., 1995) in their blades (Figure 2, Table 4). Furthermore, Fe levels indicated that iron limitation was critical for more than 40% of the species of macrophytes, as indicated by Fe concentrations in the blades below 100 mg Fe kg DW−1 (Duarte et al., 1995). For instance, the iron concentration for two species of macroalgae, T. ornata and S. ilicifolium was 12.7 and 82.6 mg Fe kg DW−1 respectively, which is 69 and 10 times lower than the concentrations reported for two T. ornata and Sargassum mangarevense on coral reefs in the island of Tahiti (Zubia et al., 2003), illustrating severe iron limitation. Overall, the seagrasses and macroalgae sampled along the Red Sea had average Fe concentrations of 335 (±275 SD) and 204 (±314 SD) mg Fe kg DW−1, respectively, which are well below the average global values reported for seagrasses (412 mg Fe kg DW−1) and macroalgae (426 mg Fe kg DW−1; Sánchez-Quiles et al., 2017). Iron limitation was recently reported for the mangrove species A. marina in the Central Red Sea (Almahasheer et al., 2016a) but, to our knowledge, this is the first documentation of iron depletion down to limiting levels in seagrasses and macroalgae in the Red Sea, which suggests a potential generalized iron limitation for growth and productivity of benthic macrophytes at the basin level. Trace metal concentration in macrophytes in the Red Sea has only previously been assessed in the Gulf of Aqaba (Sánchez-Quiles et al., 2017), the northern most region in the Red Sea, where Fe concentrations in macrophyte blades are orders of magnitude higher than those in our study (e.g., 10,689 and 7,872 mg Fe kg DW−1 for H. stipulacea and H. uninervis respectively; Wahbeh, 1984; Abu-Kharma, 2006). The Gulf of Aqaba has undergone massive commercial and industrial development in the past five decades (Al-Rousan et al., 2007) compared to the main body of the Red Sea. This development has been reported to lead to the pollution of sediments, corals, and fishes of the Gulf with heavy metals (Abu-Hilal, 1987; Al-Rousan et al., 2007, 2012; Abu-Hilal and Ismail, 2008), likely explaining the elevated concentrations of Fe in Gulf of Aqaba macrophytes as well.
We found no correlation between the iron concentration in the blades of macrophytes and their productivity, as measured by ETRmax (Figure 4A). These results are surprising for seagrasses, since it has been shown that Fe inputs can stimulate seagrass growth in iron-deficient carbonate systems in tropical and temperate regions (Duarte et al., 1995; Chambers et al., 2001; Marbà et al., 2007, 2008). Little is known about iron-limitation and growth of macroalgae on coral reefs, with few observations in the Line Islands (Kelly et al., 2012) and Guam (Kuffner and Paul, 2001) indicating contrasting results. Kelly et al. (2012) found that iron concentrations in macroalgae tissue from coral reefs near shipwrecks (which release iron) were six times higher than in macroalgae collected from reference sites. Kuffner and Paul (2001), on the other hand, reported a lack of iron-limitation in the growth of three species of macroalgae (Padina tenuis, Dictyota bartayresiana, and Halimeda incrassata) in experimental mesocosms in the Cocos Lagoon in Guam. Our results show a lack of relationship between Fe concentration in the tissue of macroalgae and the associated ETRmax, which could indicate a generalized iron limitation for the growth of macroalgae in the main body of the Rea Sea. Nonetheless, ETRmax provides a measure of the capacity of the photosystems to utilize the absorbed light energy (Marshall et al., 2000) but this does not necessarily translate into plant growth (e.g., Seboek et al., 2017), and further experimental investigation manipulating Fe inputs while assessing gross primary production is necessary.
We did not find a relationship between Fe concentration and Chl a abundance in seagrasses leaves (Figure 5A). This was unexpected since iron deficiency of angiosperms, including seagrasses, is often diagnosed from a deficiency of Chl a (Duarte et al., 1995; Belkhodja et al., 1998). Similarly, increasing Chl a in seagrasses was not related to increasing productivity, as denoted by the ETRmax values (Figure 4B). These results indicate that seagrasses may adjust their photosynthetic efficiency (e.g., α) based on the amount of light-harvesting pigments (Chl a), resulting in similar productivity rates across a gradient of Chl a abundance. We found no relationship between Fe and Chl a concentrations in the blades of ten species of macroalgae in the Red Sea (Figure 5A), with Chl a concentration being independent of productivity (Figure 4B). Our Chl a concentrations in the blades of macroalgae were low when compared to values reported in the literature: For instance, 1.4 mg Chl a g DW−1 were reported for H. tuna in this study (Table 4) vs. 2.36 mg Chl a g DW−1 reported by Beach et al. (2003) in the Florida Keys (we used a conversion factor of 8.18 from fresh weight to dry weight for H. tuna), which could indicate low Chl a concentration levels in macroalgae throughout the Red Sea. Plants have developed a remarkably uniform strategy for harvesting light using mainly Chl a and Chl b, while macroalgae have evolved a greater diversity of pigments for capturing light, including, for instance, Chl c and caroteniod fucoxanthin (Larkum and Barrett, 1983). Hence, the absence of a relationship between Chl a concentration and Fe concentration or productivity of macroalgae might be also due to a limitation in our study to assess of the abundance of all light-harvesting pigments, rather than an actual lack of a relationship with Fe concentration and productivity.
In photosynthetic organisms, the amount of Chl a per unit of tissue weight decreases with leaf thickness (Agustí et al., 1994). Our results on seven species of seagrasses in the Red Sea support this general trend, but we did not find such correlation for the 10 species of macroalgae studied here (Figure 5B). Similarly, we did not find a correlation between leaf blade thickness and productivity (Figure 4C), suggesting that this morphological trait might be unrelated to photosynthetic performance in these two types of Red Sea benthic macrophytes.
Our results showed a lack of latitudinal gradients in all studied parameters. We did not find evidence of the expected North to South gradients for Fe concentration, Chl a concentration or productivity when looking at all species of seagrasses or macroalgae together (Figure 6). The same was true for the two species that were collected throughout the Red Sea, the seagrass H. stipulacea and the macroalgae T. ornata (Figure 7), since we did not find a latitudinal gradient of Fe concentration or Chl a concentration in either of the two species (Figure 7). A similar pattern was described for the photocollecting-pigments in the zooxanthellae of the ubiquitous coral P. verrucosa, which do not increase toward southern latitudes (Sawall et al., 2014, 2015). Productivity, as indicated by ETRmax, did not show a latitudinal pattern neither when all species of seagrasses or macroalgae were grouped together (Figure 6C) nor for H. stipulacea or T. ornata alone (Figures 7E,F, respectively). This lack of a gradient in productivity for macrophytes contrasts with the results reported for other species found on coral reefs in the Red Sea, such as the coral P. verrucosa (Sawall et al., 2015), which productivity decreased from South to North. On the other hand, the lack of a latitudinal pattern described here for seagrasses and macroalgae aligns with the results for productivity of phytoplankton obtained in several research cruises in the Red Sea (Qurban et al., 2017), which showed no clear latitudinal patterns. Hence, whereas the South to North oligotrophication of the Red Sea affects some species of benthic invertebrates on corals reefs (Sawall et al., 2015; Al-Aidaroos et al., 2016) and to a certain extent pelagic phytoplankton Chl a concentrations (Raitsos et al., 2013; Kürten et al., 2015), it is not reflected in iron concentration, Chl a concentration or physiological performance of Red Sea macrophytes.
The Red Sea is characterized by scarce rainfall and an absence of rivers. Similar to our results, the seagrasses Syringodium filiforme and Thalassia testudinum were described to be iron-limited in a karstic region in the Mexican Caribbean also lacking riverine inputs (Duarte et al., 1995). In the Red Sea Basin inputs of iron are likely coming from three sources: The Indian Ocean via the Strait of Bab al Mandab, wind-induced upwelling of deep water (Triantafyllou et al., 2014; Al-Aidaroos et al., 2016), and dust deposition from the Sahara and Arabian Deserts (Jish Prakash et al., 2015). Although the quantitative sources and inputs of Fe in the Red Sea are barely known (Jish Prakash et al., 2015), our results on Fe concentration in the tissue of 17 species of macrophytes suggest that Fe is scarce and limiting in most benthic shallow ecosystems in the Arabian Red Sea, regardless of their latitudinal location. This is characteristic of carbonate rich sediments, such as those in the Red Sea, where iron is often bound in unavailable forms (e.g., Ruiz-Halpern et al., 2008). In addition, the other two potential sources of iron, dust deposition and upwelling, may play a role at homogenizing iron inputs throughout the Red Sea.
Understanding what factors limit benthic macrophytes is important given their role sequestering carbon both within blue carbon habitats and in the deep ocean (Fourqurean et al., 2012; Krause-Jensen and Duarte, 2016; Duarte and Krause-Jensen, 2017), as well as their role in supporting food webs (Antón et al., 2011). The results reported in this study contribute to unravel the complex interactions between environmental gradients and poorly-studied Red Sea macrophytes at large spatial scales. The next step forward in this research would be to experimentally investigate potential iron limitation in benthic macrophytes and evaluate more complex interactions using factorial studies examining possible synergistic effects of temperature, nitrate, phosphate, and iron.
Author Contributions
AA and CD: Conceived the project; AA, IH, NM, and DK-J: Collected the samples; AA: Performed the research and processed the samples; AA: Analyzed the data and wrote the first draft of the paper; all authors interpreted the data, made a substantial contribution to final drafts of the paper, and approved it for publication.
Funding
This research was supported by the King Abdullah University of Science and Technology through baseline funding and funds by the Tarek Ahmed Juffali Research Chair in Red Sea Ecology to CD.
Conflict of Interest Statement
The authors declare that the research was conducted in the absence of any commercial or financial relationships that could be construed as a potential conflict of interest.
Acknowledgments
We thank Vijayalaxmi Dasari, Isidora Mendia Saez de Zuazola, Sabrina Roth, Yi Zhang, Janna Randle, and Mongi Ennasri for support in the laboratory analyses, Brian Hession, CMOR and the RV Thuwal crew for logistical support, Nathan Geraldi for helpful comments on early drafts of the manuscript, John Runcie for support with the Shutter Fluorometer and two reviewers for constructive comments.
References
Abadía, J. (2008). Leaf responses to Fe deficiency: a review. J. Plant Nutr. 15, 1699–1713. doi: 10.1080/01904169209364432
Abu-Hilal, A. H. (1987). Distribution of trace elements in nearshore surface sediments from the Jordan Gulf of Aqaba (Red Sea). Mar. Pollut. Bull. 18, 190–193. doi: 10.1016/0025-326X(87)90245-1
Abu-Hilal, A. H., and Ismail, N. (2008). Heavy metals in eleven common species of fish from the gulf of aqaba, Red Sea. Jordan J. Biol. Sci. 1, 13–18. Available online at: http://jjbs.hu.edu.jo/files/vol1n1/003.pdf
Abu-Kharma, Y. M. (2006). Algae and Seagrass as Bioindicators for Trace Metal Pollution along the Jordanian Coast of the Gulf of Aqaba. Master thesis, Yarmouk University, Irbid.
Agustí, S., Enríquez, S., Frost-Christensen, H., Sand-Jensen, K., and Duarte, C. M. (1994). Light harvesting among photosynthetic organisms. Funct. Ecol. 8, 273–279. doi: 10.2307/2389911
Al-Aidaroos, A. M., Karati, K. K., El-sherbiny, M. M., Devassy, R. P., and Kürten, B. (2016). Latitudinal environmental gradients and diel variability influence abundance and community structure of Chaetognatha in Red Sea coral reefs. System. Biodivers. 15, 35–48. doi: 10.1080/14772000.2016.1211200
Almahasheer, H., Aljowair, A., Duarte, C. M., and Irigoien, X. (2016a). Decadal stability of Red Sea mangroves. Estuar. Coast. Shelf Sci. 169, 164–172. doi: 10.1016/j.ecss.2015.11.027
Almahasheer, H., Duarte, C. M., and Irigoien, X. (2016b). Nutrient limitation in central red sea mangroves. Front. Mar. Sci. 3:271. doi: 10.3389/fmars.2016.00271
Almahasheer, H., Duarte, C. M., and Irigoien, X. (2016c). Phenology and growth dynamics of Avicennia marina in the central red sea. Sci. Rep. 6:37785. doi: 10.1038/srep37785
Al-Rousan, S. A., Al-Shloul, R. N., Al-Horani, F. A., and Abu-Hilal, A. H. (2007). Heavy metal contents in growth bands of Porites corals: record of anthropogenic and human developments from the Jordanian Gulf of Aqaba. Mar. Pollut. Bull. 54, 1912–1922. doi: 10.1016/j.marpolbul.2007.08.014
Al-Rousan, S., Al-Shloul, R., Al-Horani, F., and Abu-Hilal, A. (2012). Heavy metals signature of human activities recorded in coral skeletons along the Jordanian coast of the Gulf of Aqaba, Red Sea. Environ. Earth Sci. 67, 2003–2013. doi: 10.1007/s12665-012-1640-0
Antón, A., Cebrian, J., Heck, K. L., Duarte, C. M., Sheehan, K. L., Miller, M. E., et al. (2011). Decoupled effects (positive to negative) of nutrient enrichment on ecosystem services. Ecol. Appl. 21, 991–1009. doi: 10.1890/09-0841.1
Beach, K., Walters, L., Borgeas, H., Smith, C., Coyer, J., and Vroom, P. (2003). The impact of Dictyota spp. on halimeda populations of conch reef, florida keys. J. Exp. Mar. Biol. Ecol. 297, 141–159. doi: 10.1016/j.jembe.2003.07.003
Beer, S. (1998). Measuring photosynthetic rates in seagrasses by pulse amplitude modulated (PAM) fluorometry. Mar. Ecol. Prog. Ser. 174, 293–300. doi: 10.3354/meps174293
Beer, S., and Björk, M. (2000). Measuring rates of photosynthesis of two tropical seagrasses by pulse amplitude modulated (PAM) fluorometry. Aquat. Bot. 66, 69–76. doi: 10.1016/S0304-3770(99)00020-0
Beer, S., Bjork, M., Gademann, R., and Ralph, P. J. (2001). “Measurements of photosynthetic rates in seagrasses,” in Global Seagrass Research Methods, eds F. Short and R. G. Coles (Amsterdam: Elsevier Publishing), 183–198.
Belkhodja, R., Morales, F., Sanz, M., Abadía, A., and Abadía, J. (1998). Iron deficiency in peach trees: effects on leaf chlorophyll and nutrient concentrations in flowers and leaves. Plant. Soil. 203, 257–268. doi: 10.1023/A:1004373202458
Cantin, N. E., Cohen, A. L., Karnauskas, K. B., Tarrant, A. M., and McCorkle, D. C. (2010). Ocean warming slows coral growth in the Central Red Sea. Science 329, 322–325. doi: 10.1126/science.1190182
Chambers, R. M., Fourqurean, J. W., Macko, S. A., and Hoppenot, R. (2001). Biogeochemical effects of iron availability on primary producers in a shallow marine carbonate environment. Limnol. Oceanogr. 46, 1278–1286. doi: 10.4319/lo.2001.46.6.1278
Coale, K. H., Johnson, K. S., Fitzwater, S. E., Gordon, R. M., Tanner, S., Chavez, F. P., et al. (1996). A massive phytoplankton bloom induced by an ecosystem-scale iron fertilization experiment in the equatorial Pacific Ocean. Nature 383, 495–501. doi: 10.1038/383495a0
Duarte, C. M., and Krause-Jensen, D. (2017). Export from seagrass meadows contributes to marine carbon sequestration. Front. Mar. Sci. 4:13. doi: 10.3389/fmars.2017.00013
Duarte, C. M., Merino, M., and Gallegos, M. (1995). Evidence of iron deficiency in seagrasses growing above carbonate sediments. Limnol. Oceanogr. 40, 1153–1158. doi: 10.4319/lo.1995.40.6.1153
Duarte, C. M., Dennison, W. C., Orth, R. J. W., and Carruthers, T. J. B. (2008). The charisma of coastal ecosystems: addressing the imbalance. Estuaries Coasts 31, 233–238. doi: 10.1007/s12237-008-9038-7
Eilers, P. H. C., and Peeters, J. C. H. (1988). A model for the relationship between light intensity and the rate of photosynthesis in phytoplankton. Ecol. Modell. 42, 199–215. doi: 10.1016/0304-3800(88)90057-9
Enriquez, S., Duarte, C. M., and Sand-Jensen, K. (1995). Patterns in the photosynthetic metabolism of mediterranean macrophytes. Mar. Ecol. Prog. Ser. 119, 243–252. doi: 10.3354/meps119243
Enríquez, S., Duarte, C. M., Sand-Jensen, K., and Nielsen, S. L. (1996). Broad-scale comparison of photosynthetic rates across phototrophic organisms. Oecologia 108, 197–206. doi: 10.1007/BF00334642
Fourqurean, J. W., Duarte, C. M., Kennedy, H., Marbà, N., Holmer, M., Serrano, M. A., et al. (2012). Seagrass ecosystems as a globally significant carbon stock. Nat. Geosci. 5, 505–509. doi: 10.1038/ngeo1477
Geider, R. J., La Roche, J., Greene, R. M., and Olaizola, M. (1993). Response of the photosynthetic apparatus of Phaeodactylum tricornutum (Bacillariophyceae) to nitrate, phosphate, or iron starvation. J. Phycol. 29, 755–766. doi: 10.1111/j.0022-3646.1993.00755.x
Jish Prakash, P., Stenchikov, G., Kalenderski, S., Osipov, S., and Bangalath, H. (2015). The impact of dust storms on the arabian peninsula and the red sea. Atmos. Chem. Phys. 15, 199–222. doi: 10.5194/acp-15-199-2015
Kelly, L. W., Barott, K. L., Dinsdale, E., Friedlander, A. M., Nosrat, B., Rohwer, D., et al. (2012). Black reefs: iron-induced phase shifts on coral reefs. ISME J. 6, 708–708. doi: 10.1038/ismej.2011.148
Krause-Jensen, D., and Duarte, C. M. (2016). Substantial role of macroalgae in marine carbon sequestration. Nat. Geosci. 9, 737–742. doi: 10.1038/ngeo2790
Kuffner, I. B., and Paul, V. J. (2001). Effects of nitrate, phosphate and iron on the growth of macroalgae and benthic cyanobacteria from Cocos Lagoon, Guam. Mar. Ecol. Prog. Ser. 222, 63–72. doi: 10.3354/meps222063
Kürten, B., Khomayis, H. S., Devassy, R., Audritz, S., Sommer, U., Al-Aidaroos, U., et al. (2015). Ecohydrographic constraints on biodiversity and distribution of phytoplankton and zooplankton in coral reefs of the Red Sea, Saudi Arabia. Mar. Ecol. 36, 1195–1214. doi: 10.1111/maec.12224
Larkum, A. W. D., and Barrett, J. (1983). Light-harvesting processes in algae. Adv. Bot. Res. 10, 1–219. doi: 10.1016/S0065-2296(08)60260-8
Marbà, N., Duarte, C. M., Holmer, M., Calleja, M. L., Álvarez, E., Díaz-Almela, E., and Garcias-Bonet, N. (2008). Sedimentary iron inputs stimulate seagrass (Posidonia oceanica) population growth in carbonate sediments. Estuar. Coast. Shelf Sci. 76, 710–713. doi: 10.1016/j.ecss.2007.07.021
Marbà, N., Calleja, M. L., Duarte, C. M., Álvarez, E., Díaz-Almela, E., and Holmer, M. (2007). Iron additions reduce sulfide intrusion and reverse seagrass (posidonia oceanica) decline in carbonate sediments. Ecosystems 10, 745–756. doi: 10.1007/s10021-007-9053-8
Marshall, H. L., Geider, R. J., and Flynn, K. J. (2000). A mechanistic model of photoinhibition. New Phytol. 145, 347–359. doi: 10.1046/j.1469-8137.2000.00575.x
Martin, J. H., Coale, K. H., Johnson, K. S., Fitzwater, S. E., Gordon, R. M., Tindale, S. J., et al. (1994). Testing the iron hypothesis in ecosystems of the equatorial Pacific Ocean. Nature 371, 123–129. doi: 10.1038/371123a0
Pestana, M., Varennes, D., and Faria, E. A. (2003). Diagnosis and correction of iron chlorosis in fruit trees: a review. J. Food Agric. Environ. 1, 46–51. Available online at: http://www.nutricaodeplantas.agr.br/site/downloads/qualidade_frutas_pestanaFe.pdf
Pinheiro, J., Bates, D., DebRoy, S., Sarkar, D., and Core Team, R. (2015). nlme: Linear and Nonlinear Mixed Effects Models.
Qurban, M. A., Wafar, M., Jyothibabu, R., and Manikandan, K. P. (2017). Patterns of primary production in the Red Sea. J. Mar. Syst. 169, 87–98. doi: 10.1016/j.jmarsys.2016.12.008
Raitsos, D. E., Yi, X., Platt, T., Racault, M. F., Brewin, R. J. W., Hoteit, Y., et al. (2015). Monsoon oscillations regulate fertility of the Red Sea. Geophys. Res. Lett. 42, 855–862. doi: 10.1002/2014GL062882
Raitsos, D. E., Pradhan, Y., Brewin, R. J. W., Stenchikov, G., and Hoteit, I. (2013). Remote sensing the phytoplankton seasonal succession of the red sea. PLoS ONE 8:e64909. doi: 10.1371/journal.pone.0064909
Ralph, P. J., and Gademann, R. (2005). Rapid light curves: a powerful tool to assess photosynthetic activity. Aquat. Bot. 82, 222–237. doi: 10.1016/j.aquabot.2005.02.006
Roik, A., Roder, C., Röthig, T., and Voolstra, C. R. (2016). Spatial and seasonal reef calcification in corals and calcareous crusts in the central Red Sea. Coral Reefs 35, 681–693. doi: 10.1007/s00338-015-1383-y
Ruiz-Halpern, S., Macko, S. A., and Fourqurean, J. W. (2008). The effects of manipulation of sedimentary iron and organic matter on sediment biogeochemistry and seagrasses in a subtropical carbonate environment. Biogeochemistry 87, 113–126. doi: 10.1007/s10533-007-9162-7
Sánchez-Quiles, D., Marba, N., and Tovar-Santhez, A. (2017). Trace metal accumulation in marine macrophytes: hotspots of coastal contamination worldwide. Sci. Tot. Environ. 576, 520–527. doi: 10.1016/j.scitotenv.2016.10.144
Sawall, Y., Al-Sofyani, A., Banguera-Hinestroza, E., and Voolstra, C. R. (2014). Spatio-temporal analyses of Symbiodinium physiology of the coral Pocillopora verrucosa along large-scale nutrient and temperature gradients in the Red Sea. PLoS ONE 9:e103179. doi: 10.1371/journal.pone.0103179
Sawall, Y., Al-Sofyani, A., Hohn, S., Banguera-Hinestroza, E., Voolstra, C. R., and Wahl, M. (2015). Extensive phenotypic plasticity of a Red Sea coral over a strong latitudinal temperature gradient suggests limited acclimatization potential to warming. Sci. Rep. 5:8940. doi: 10.1038/srep08940
Seboek, S., Herppich, W. B., and Hanelt, D. (2017). Red alga Palmaria palmata growth rate and photosynthetic performance under elevated CO2 treatment. J. Appl. Phycol. 29, 381–393. doi: 10.1007/s10811-016-0939-8
Silva, J., Santos, R., Calleja, M. L., and Duarte, C. M. (2005). Submerged versus air-exposed intertidal macrophyte productivity: from physiological to community-level assessments. J. Exp. Mar. Biol. Ecol. 317, 87–95. doi: 10.1016/j.jembe.2004.11.010
Sofianos, S. S., and Johns, W. E. (2003). An Oceanic General Circulation Model (OGCM) investigation of the Red Sea circulation: 2. Three-dimensional circulation in the Red Sea. J. Geophys. Res. Oceans 108, 3066. doi: 10.1029/2001JC001185
Sterner, R. W., Smutka, T. M., McKay, R. M. L., Qin, X. M., Brown, E. T., and Sherrell, R. M. (2004). Phosphorus and trace metal limitation of algae and bacteria in Lake Superior. Limnol. Oceanogr. 49, 495–507. doi: 10.4319/lo.2004.49.2.0495
Triantafyllou, G., Yao, F., Petihakis, G., Tsiaras, K. P., Raitsos, D. E., and Hoteit, I. (2014). Exploring the Red Sea seasonal ecosystem functioning using a three-dimensional biophysical model. J. Geophy. Res. 119, 1791–1811. doi: 10.1002/2013JC009641
Wahbeh, M. I. (1984). Levels of zinc, manganese, magnesium, iron and cadmium in three species of seagrass from Aqaba (Jordan). Aquat. Bot. 20, 179–183. doi: 10.1016/0304-3770(84)90034-2
Wang, X. T., Zhang, Q., Liu, Y. S., Zhang, P. D., and Li, W. T. (2017). The influence of increased iron concentration on survival and growth of seedlings and young plants of eelgrass Zostera marina. Mar. Ecol. Evol. Perspect. 38:e12425. doi: 10.1111/maec.12425
Welch, R. M., and Shuman, D. L. (1995). Micronutrient nutrition of plants. CRC Crit. Rev. Plant Sci. 14, 49–82. doi: 10.1080/07352689509701922
Wellburn, A. R. (1994). The spectral determination of chlorophylls a and b, as well as total carotenoids, using various solvents with spectrophotometers of different resolution. J. Plant Physiol. 144, 307–313. doi: 10.1016/S0176-1617(11)81192-2
Winder, T. L., and Nishio, J. N. (1995). Early iron deficiency stress response in leaves of sugar beet. Plant Physiol. 108, 1487–1494. doi: 10.1104/pp.108.4.1487
Ziegler, M., Roder, C. M., Büchel, C., and Voolstra, C. R. (2014). Limits to physiological plasticity of the coral Pocillopora verrucosa from the central Red Sea. Coral Reefs 33, 1115–1129. doi: 10.1007/s00338-014-1192-8
Keywords: coral reefs, fluorescence, latitudinal gradient, macroalgae, oligotrophic, primary production, seagrass, seaweed
Citation: Anton A, Hendriks IE, Marbà N, Krause-Jensen D, Garcias-Bonet N and Duarte CM (2018) Iron Deficiency in Seagrasses and Macroalgae in the Red Sea Is Unrelated to Latitude and Physiological Performance. Front. Mar. Sci. 5:74. doi: 10.3389/fmars.2018.00074
Received: 15 November 2017; Accepted: 16 February 2018;
Published: 06 March 2018.
Edited by:
Angel Borja, Centro Tecnológico Experto en Innovación Marina y Alimentaria (AZTI), SpainReviewed by:
Stein Fredriksen, University of Oslo, NorwayLech Kotwicki, Institute of Oceanology (PAN), Poland
Copyright © 2018 Anton, Hendriks, Marbà, Krause-Jensen, Garcias-Bonet and Duarte. This is an open-access article distributed under the terms of the Creative Commons Attribution License (CC BY). The use, distribution or reproduction in other forums is permitted, provided the original author(s) and the copyright owner are credited and that the original publication in this journal is cited, in accordance with accepted academic practice. No use, distribution or reproduction is permitted which does not comply with these terms.
*Correspondence: Andrea Anton, YW5kcmVhLmFudG9uZ2FtYXpvQGthdXN0LmVkdS5zYQ==