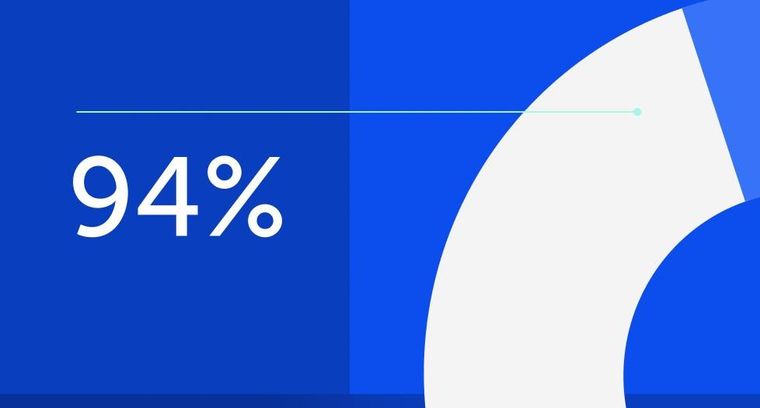
94% of researchers rate our articles as excellent or good
Learn more about the work of our research integrity team to safeguard the quality of each article we publish.
Find out more
ORIGINAL RESEARCH article
Front. Mar. Sci., 21 December 2017
Sec. Marine Biogeochemistry
Volume 4 - 2017 | https://doi.org/10.3389/fmars.2017.00390
This article is part of the Research TopicChanges in nutrient turnover in response to ocean deoxygenationView all 14 articles
Despite its potential to provide new nitrogen (N) to the environment, knowledge on benthic dinitrogen (N2) fixation remains relatively sparse, and its contribution to the marine N budget is regarded as minor. Benthic N2 fixation is often observed in organic-rich sediments coupled to heterotrophic metabolisms, such as sulfate reduction. In the present study, benthic N2 fixation together with sulfate reduction and other heterotrophic metabolisms were investigated at six station between 47 and 1,108 m water depth along the 18°N transect traversing the highly productive upwelling region known as Mauritanian oxygen minimum zone (OMZ). Bottom water oxygen concentrations ranged between 30 and 138 μM. Benthic N2 fixation determined by the acetylene reduction assay was detected at all stations with highest rates (0.15 mmol m−2 d−1) on the shelf (47 and 90 m water depth) and lowest rates (0.08 mmol m−2 d−1) below 412 m water depth. The biogeochemical data suggest that part of the N2 fixation could be linked to sulfate- and iron-reducing bacteria. Molecular analysis of the key functional marker gene for N2 fixation, nifH, confirmed the presence of sulfate- and iron-reducing diazotrophs. High N2 fixation further coincided with bioirrigation activity caused by burrowing macrofauna, both of which showed high rates at the shelf sites and low rates in deeper waters. However, statistical analyses proved that none of these processes and environmental variables were significantly correlated with benthic diazotrophy, which lead to the conclusion that either the key parameter controlling benthic N2 fixation in Mauritanian sediments remains unidentified or that a more complex interaction of control mechanisms exists. N2 fixation rates in Mauritanian sediments were 2.7 times lower than those from the anoxic Peruvian OMZ.
Dinitrogen (N2) fixation is the dominant source of new bioavailable nitrogen (N) in the marine environment (Brandes and Devol, 2002). Only N2 fixing prokaryotes (diazotrophs) have the capability to convert N2 to bioavailable N, i.e., ammonium, and make it available for non-diazotrophic organisms (Ward and Bronk, 2001; Gruber, 2008). Diazotrophs can be detected using molecular tools such as the nifH gene, the key functional marker encoding a subunit of the nitrogenase reductase enzyme (Sisler and ZoBell, 1951; Riederer-Henderson and Wilson, 1970; Zehr and Turner, 2001).
While most studies on marine N2 fixation have focused on pelagic environments (e.g., Zehr and Ward, 2002; Galloway et al., 2004; Riemann et al., 2010; Löscher et al., 2014 and references therein), benthic N2 fixation gained renewed attention only recently with a few studies demonstrating active N2 fixation in sediments and identifying diazotrophs by nifH gene analysis (Fulweiler et al., 2007; Bertics et al., 2010, 2013; Gier et al., 2016). However, there is uncertainty regarding the environmental factors ultimately controlling benthic N2 fixation. Previous studies identified the availability of organic matter as major control on benthic microbial processes (Jørgensen, 1983; Howarth et al., 1988; Fulweiler et al., 2007; Bertics et al., 2013). Further, benthic N2 fixation and organic matter have been found to correlate in different habitats, such as sediments within the high-productive Peruvian upwelling region (Gier et al., 2016) and coastal sediments inhabited by the bioturbating ghost shrimp Neotrypaea californiensis (Bertics et al., 2010).
Other studies have shown that the physical movement of animals through surface sediments can enhance N2 fixation (Bertics et al., 2010). Bioturbation and bioirrigation in sediments increase the rate of organic matter supply to subsurface sediment layers, leading to elevated microbial metabolic rates there (Aller and Aller, 1986; Bertics et al., 2010, 2012). While bioturbation describes the sediment mixing by benthic organisms, bioirrigation encompasses the exchange of seawater with sediment porewater due to the pumping action of burrow-dwelling organisms (Meysman et al., 2006; Kristensen et al., 2012). These processes were associated with increased rates of microbial sulfate reduction (Bertics and Ziebis, 2010) and N2 fixation (Bertics et al., 2012). Both microbial processes are often coupled in organic-rich sediments (Bertics and Ziebis, 2010; Bertics et al., 2013; Gier et al., 2016). Additionally, many sulfate reducers carry the nifH gene (Zehr and Turner, 2001; Muyzer and Stams, 2008; Fulweiler et al., 2013; Gier et al., 2016) and actively fix N2 in culture (Riederer-Henderson and Wilson, 1970), indicating that these bacteria may play a role in supplying bioavailable N to the benthic community (Bertics et al., 2010; Sohm et al., 2011; Fulweiler et al., 2013).
In the present study, the effect of organic matter availability and bioirrigation on benthic N2 fixation was determined in the highly productive upwelling region off Mauritania. The region is characterized by a weak oxygen minimum zone (OMZ) with dissolved oxygen (O2) concentrations of down to 27 μM (Löscher et al., 2016). The OMZ is predicted to lose more O2 in the future at a rate of ~0.5 μM y−1 (Stramma et al., 2008; Keeling et al., 2010). As a consequence of low O2, Mauritanian OMZ sediments are a net sink for dissolved inorganic N due to denitrification (Dale et al., 2014). N loss is highest on the shelf and decreases with increasing water depth, in line with particulate organic carbon flux to the seafloor. The N deficit resulting from this N loss could to a certain extent be replenished by N2 fixation. However, the relevance of benthic N2 fixation for N cycling in the Mauritanian OMZ sediments is yet unknown. Extensive bioirrigation and bioturbation by bottom dwelling macrofauna observed in this region may be a major promoting factor for benthic N2 fixation (Dale et al., 2014). Thus, subsurface microbial activities including N2 fixation could be stimulated here (Jørgensen, 1983; Fulweiler et al., 2007; Bertics et al., 2010). Due to the potential expansion of the OMZ, together with high input of labile organic matter to the seafloor, the Mauritanian OMZ is a key region to understand how benthic N2 fixation may change in the future.
We postulate that a coupling between N2 fixation and sulfate reduction, and potentially other heterotrophic bacteria, exists in Mauritanian OMZ sediments, which is stimulated by enhanced benthic organic matter availability due to high carbon export and bioirrigation. The overall goal of the present study was to (1) investigate the relation of benthic N2 fixation and heterotrophic bacteria (specifically sulfate reducers) along the Mauritanian margin, (2) explore benthic diazotrophic diversity, and (3) investigate the effect of bioirrigation on N2 fixation. Finally, we compared benthic N2 fixation in the weak OMZ off Mauritania with benthic N2 fixation in the anoxic Peruvian OMZ to better understand how marine N cycling may change as bottom water O2 levels diminish.
The region off Mauritania belongs to the extensive eastern tropical North Atlantic upwelling system, which represents a moderate OMZ with lowest O2 concentrations of ~30 μM (Karstensen et al., 2008; Chavez and Messié, 2009). The upwelling system extends between 43°N at the Iberian peninsula and 10°N south off Dakar (Schafstall et al., 2010). While upwelling is continuous between 20 and 25°N, upwelling north and south of this region is seasonal, induced by variations in wind forcing related to the migration of the Intertropical Convergence Zone (Barton et al., 1998). Along the continental slope highly non-linear internal waves export fine-grained sediment particles down slope (Schafstall et al., 2010). The upwelling intensity at 18°N (this study) is strongest between December and April (boreal winter). The 18°N area (50–1,100 m water depth) features a perennial high primary production (80–200 mmol C m−2 d−1) (Huntsman and Barber, 1977), probably enhanced by the iron-rich dust input from the Sahara (Baker et al., 2006). This makes the eastern tropical North Atlantic one of the most productive marine environments (Carr, 2001).
Sediments at 18°N are characterized by an increase of surface particulate organic carbon with water depth (0.6 wt% on the shelf and 2.7 wt% at 800 m) and a decrease of particulate organic carbon with depth in the sediments (Dale et al., 2014). While the shelf at the 18°N upwelling region is characterized by minor sediment accumulation rates, sedimentation rates between 0.1 and 0.35 cm yr−1 were found at the deeper stations. Dale et al. (2014) described the 18°N sediment as muddy sand down to 400 m water depth and as slightly sandy mud from 786 m. Surface porosity was low (0.56–0.62) at the shallow sites (<100 m) and high (0.83–0.85) at deeper sites (>786 m), with grain size observations (Sokoll, 2013) indicating permeable sediments down to 400 m water depth (Dale et al., 2014). Permeable sandy sediments were originally considered to be biogeochemically inert due to their low organic carbon content (Shum and Sundby, 1996; Boudreau et al., 2001). Yet, topography-driven advective solute transport due to pressure gradients and bottom currents has changed this view (Huettel and Rusch, 2000; Rusch and Huettel, 2000; Janssen et al., 2005). Sandy sediments are thus often regarded as potential sites for high metabolic activity (Boudreau and Westrich, 1984; Huettel et al., 2003).
Sampling was conducted in June 2014 at seven stations (47, 90, 169, 236, 412, 786, and 1,108 m) at 18°N (Figure 1) during an expedition on RV Meteor (M107). The station ID, corer ID, sampling date, and location, water depth, temperature, O2 concentration, and parameters determined for each station are listed in Table 1.
Figure 1. Contour plot of dissolved O2 (μM) concentrations along the continental margin of the Mauritanian OMZ at 18°N. The black dots represent stations for MUC sampling with corresponding water depth in meters. The inset shows the sampling area in a regional context (black box).
Table 1. Sampling stations along the depth transect at 18°N off Mauritania, with five replicate cores used for the determination of (1) porewater geochemistry, (2) sediment properties, (3) bioirrigation, (4) N2 fixation and molecular microbiology, and (5) sulfate reduction.
Temperature and dissolved O2 concentrations in the water column were obtained using a SeaBird CTD rosette system equipped with a Seabird SBE43 membrane O2 sensor. The sensors were calibrated by Winkler titration with a detection limit of 2 μmol L−1.
Sediment samples for biogeochemical investigations were taken by a TV-guided multiple corer (MUC) equipped with six core liners. Each core liner had a length of 60 cm and an inner diameter of 10 cm. All sediment cores were immediately transferred to a cold room (12°C) for further processing.
Measurements for the analysis of porewater geochemistry are described in detail by Dale et al. (2011), Dale et al. (2015). In short, one replicate core from each MUC sampling (Table 1) was subsampled at anoxic conditions using an argon-filled glove bag to preserve redox sensitive constituents. Concentrations of ammonium, nitrate, ferrous iron, and sulfide were determined on a Hitachi U2800 UV/VIS spectrophotometer using standard procedures (Grasshoff et al., 1999). Sediment properties (porosity, particulate organic carbon, and nitrogen) were determined on a second replicate MUC core (Table 1) as described by Dale et al. (2014). A third replicate MUC core (Table 1) was used for bioirrigation experiments. Bioirrigation experiments were performed following former procedures (Dale et al., 2013), involving the addition of bromide (Br−) as a dissolved conservative tracer. Cores were incubated for several days, after which the Br− depth distribution was determined in extracted porewater samples by ion chromatography (Metrohm 761). These data were used to calculate bioirrigation rates using a numerical model that considered Br− transport due to diffusion and bioirrigation. Details of the model are described fully by Dale et al. (2013). The flux due to irrigation was calculated as
In this equation, the bromide concentration is in mol l−1, αbi (d−1) is the depth-dependent bioirrigation coefficient describing solute pumping through animal burrows and Brolw (M) is the time-dependent Br− concentration in the well mixed, overlying water. The sediment porosity, ϕ, was defined using a depth-dependent empirical function (Dale et al., 2013). The depth-dependence of αbi was described using
where αbi1 (d−1) is approximately equal to the bioirrigation coefficient at the sediment surface and αbi2 (cm) is a parameter that controls the irrigation depth. We integrated αbi over the upper 30 cm for each site, j, and normalized this value to the integrated coefficient at the deepest site, to compare irrigation intensities between sites:
We acknowledge that several burrowing species perform bioirrigation and bioturbation simultaneously and may also transport and mix particulate organic matter into the sediment (Christensen et al., 2000; Griffen et al., 2004; Quintana et al., 2007; Kristensen et al., 2012). Bioturbation rates were not determined in this study, and bioirrigation alone was considered as a quantitative indicator for the activity of animals in the sediment.
The sampling procedure (Table 1) and core slicing details for N2 fixation have previously been described by Gier et al. (2016). In short, at a fourth replicate MUC core (Table 1) from the sampling stations was sliced in the cold room in 1-cm intervals from 0 to 6 cm, in 2-cm intervals from 6 to 10 cm, and in 5-cm intervals from 10 to 20 cm. In order to quantify the nitrogenase activity, the acetylene reduction assay was applied (Stewart et al., 1967; Capone, 1993). Serum vials (60 mL) were flushed with N2, and then filled with 10 cm3 sediment (in triplicate) from each depth horizon, flushed again with N2 and crimp sealed with a butyl stopper. Samples were injected with 5 mL pure compressed acetylene, which was bubbled through ultrapure water to remove impurities. Finally, samples were gently pivoted and stored in the dark at average in situ temperature found at the seafloor along the depth-transect (12°C, see Table 1). Two sets of triplicate controls were prepared for every station. One set of controls was not injected with acetylene to test for natural ethylene production. The second set of controls was killed with 1 mL formalin (37.5%) to quantify abiotic ethylene production.
The increase of ethylene in each sample was assayed on board for over 1 week (5 time points) by a gas chromatograph. To convert nitrogenase activity to N2 fixation, a conversion factor of 3 ethylene: 1 N2 (Patriquin and Knowles, 1972; Orcutt et al., 2001; Capone et al., 2005; Bertics et al., 2013) was applied. In the following sections, converted acetylene reduction will therefore be termed N2 fixation. Standard deviations of N2 fixation were calculated from three replicates per sediment depth. For integrated N2 fixation rates, standard deviations were calculated from the three integrated rates per station.
To determine sulfate reduction rates, one push core (length 30 cm, inner diameter 2.6 cm) was taken from a fifth replicate MUC core (Table 1). Six microliters of the carrier-free 35 radio tracer (dissolved in water, 150 kBq, specific activity 37 TBq mmol−1) were injected in 1-cm intervals according to the whole-core injection method (Jørgensen, 1978). Push cores varied in length between 21 and 25 cm. Each core was incubated in the dark at 12°C for ~12 h. The incubation was stopped by slicing each core in 1-cm intervals and transferring the sediment into 50 mL plastic centrifuge tubes filled with 20 mL zinc acetate (20% w/w). The controls (in triplicate) were fixed with zinc acetate (20% w/w) before adding the radiotracer. Samples were stored frozen at −20°C (Røy et al., 2014) until further processing in the home laboratory. Sulfate reduction rates were determined using the cold chromium distillation procedure according to Kallmeyer et al. (2004).
Samples for nifH gene analysis were collected from the N2 fixation MUC cores (Table 1). Sediment (~5 mL) from each sampling depth (except 0–1 cm for 47 m and 10–15 cm for 786 m) was transferred to plastic whirl-paks® (Nasco, Fort Atkinson, USA), frozen at −20°C and transported back to the home laboratory. To extract DNA, the FastDNA® SPIN Kit for Soil (MP Biomedicals, Carlsbad, CA, USA) was used according to the manufactures instructions, except that the sample homogenization that was done in a Mini-BeadbeaterTM (Biospec Products, Bartlesville, USA) for 15 s. The yield of DNA ranged from 22 to 65 ng/μl based on NanoDrop spectrophotometer (Nanodrop 1000, Thermo Fisher Scientific, Waltham, MA, USA) quantification.
Overall, 60 samples were used for nifH amplicon sequencing. Nested polymerase chain reactions (PCRs) for nifH were performed following established protocols (Zehr and Turner, 2001). Modifications of the protocol adjusted for Illumina sequencing preparation have previously been described by Bentzon-Tilia et al. (2015). Illumina indices were added to amplicons in the second PCR round. In addition to the nifH1 and nifH2 primer sequences, the primer contained a linker sequence, an 8-base barcode and the Illumina specific region P5 (forward primer) or P7 (reverse primer) (for details on the sequence of primers see Table S1 in Supplementary Material). Negative controls consisted of the reaction mixture of the addition of DNA. PCRs were performed in triplicate for each sample. Triplicates were then pooled, and purified using the MinElute Gel Extraction Kit (Qiagen, Hildesheim, Germany) and quantified on a spectrophotometer (Nanodrop 1000, Thermo Fisher Scientific, Waltham, MA, USA). Samples were pooled in equimolar ratios and sequencing took place on an Illumina MiSeq Instrument using the MiSeq reagent Kit with V3 chemistry (Illumina, San Diego, CA, USA). Sequences were submitted as a sequence read archive (SRA) to GenBank, submission ID SUB3036872.
Sequences were assembled using MOTHUR software version 1.32.1 (Kozich et al., 2013). Contigs containing ambiguous bases or homopolymers longer than eight bases were removed from the dataset. Redundant sequences were clustered using the command unique.seqs and aligned against the functional gene pipeline and repository database (http://fungene.cme.msu.edu/). Sequences not aligning with the seed nifH sequence pool were removed. Chimeric sequences were removed with the MOTHUR implemented software Uchime (Edgar et al., 2011). Remaining sequences were clustered at 97% nucleotide similarity and reference sequences for the 10 most abundant clusters were obtained using BLAST search on the NCBI database. Amplicons and reference nifH sequences were consecutively ClustalW aligned using MEGA version 6.0 (Tamura et al., 2013), and a maximum likelihood tree was constructed and visualized using iTOL (Letunic and Bork, 2011).
At 18°N, dissolved O2 was present in the bottom water across the entire transect (Figure 1). Bottom water O2 concentration on the shelf station at 47 m was 123 μM and decreased to 30 μM at 90 m, representing the lowest measured concentration along the transect. At 236 m and 412 m, the O2 concentration was 48 and 50 μM, respectively, and increased from 98 μM at 786 m to 138 μM at 1,108 m.
Figure 2 shows the geochemical porewater profiles of ammonium, nitrate, sulfide, organic carbon content, and the C/N ratio in the upper 20 cm at each station. In general, the profiles were very similar to those measured along the same transect in spring 2011 (Dale et al., 2011). Ammonium concentrations increased with sediment depth, with highest concentrations (111 μM) at 1108 m. The lowest ammonium concentration (30 μM) at 20 cm sediment depth of all cores was measured at 236 m. Concentrations of nitrate were highest at the sediment surface (0–1 cm) in all cores, except for the 236 m station, which showed no peak. Peaks ranged between 0.1 and 34 μM and rapidly decreased to zero below the surface layer. The accumulation of sulfide was detected only at the two shelf stations (47 and 90 m) with peaks of 88 μM at 14 cm and 45 μM at 13 cm, respectively. Sulfide accumulated below ca. 10 cm at these sites, with near-zero concentrations closer to the sediment surface. Organic carbon content was ~1 wt% throughout the cores from 47 to 412 m. The highest organic carbon (~3 wt%) was measured at 786 m and 1,108 m. The lowest value (~0.5 wt%) was measured at 90 m. The benthic molar C/N ratio scattered around 13 at the surface and 8 at the bottom of the core at the shallowest station (47 m) and remained relatively constant at around 9–10 throughout the cores at the stations between 90 and 1,108 m.
Figure 2. Biogeochemical porewater profiles in sediments cores from sampling stations along the 18°N transect. Plots show ammonium (, μM), nitrate (, μM), sulfide (μM), organic carbon content (Corg, wt%), and the C/N ratio (molar). Bottom water O2 concentrations (BW O2, μM) is provided at the right margin.
Sediment porosity (data not shown) at the surface was low (0.52) at the shelf stations (47 and 90 m), increased with water depth, and was highest (0.86) at the deepest station. Porosity gradually decreased with sediment depth, to a value of 0.45 at 20 cm sediment depth at 47 m and 0.74 at the deep 1,108 m site.
Bioirrigation was detected at all sites, with higher coefficients and irrigation depths at the shelf stations (47, 90, and 169 m) vs. the deep sites (412, 786, and 1,108 m) (Figure 3A). At 47 m the highest bioirrigation coefficient (αbi1 = 0.82 d−1) was measured along with a high bioirrigation depth parameter (αbi2 = 11.4 cm). The lowest bioirrigation depth parameter (0.23 cm) was determined at 1,108 m. The normalized irrigation coefficient (Figure 3B) at 47 m is 50 times greater than that at the deepest site, coincident with high bottom water O2 (123 μM) (Figures 1, 5) and low integrated organic carbon content (0.8 wt%, Figures 2, 5).
Figure 3. (A) Sediment depth profiles of measured (symbols) and modeled (curves) bromide (Br−) concentrations at the end of the bioirrigation experiments along the depth transect (47, 90, 169, 412, 786, and 1,108 m). The gray area indicates the transport of Br− as expected by molecular diffusion only. The coefficients αbi1 (d−1) and αbi2 (cm) represent the bioirrigation coefficient at the sediment surface and the parameter that controls the bioirrigation depth, respectively. (B) Depth-integrated bioirrigation coefficient (dimensionless) along the depth transect (m) normalized to the deepest site.
In total ~8,000 nifH gene sequences were obtained that grouped into 10 clusters (Figure 4). NifH sequences were detected at all sampling sites and clustered with Cluster I proteobacterial sequences and Cluster III sequences as defined by Zehr and Turner (2001). No Cluster I cyanobacterial nifH sequences were identified. At a first look, we followed the redox cascade to investigate the potential involvement of different anaerobic heterotrophic bacteria in N2-fixation. No sequences for denitrifying or manganese-reducing bacteria were detected. Sequences clustering with known organisms using iron and sulfur as electron acceptors, namely Pelobacter carbinolicus (Lovley et al., 1995) and the species Caldicellulosiruptor saccharolyticus, which hydrolyses a variety of polymeric carbohydrates (Rainey et al., 1994), were found in low (2%) abundance (at 46, 90, 412, and 1,108 m). Sequences closely related to sulfate-reducing bacteria of the genus Desulfovibrio, such as Desulfovibrio desulfuricans (Steenkamp and Peck, 1981; Lobo et al., 2007), Desulfovibrio vulgaris (Riederer-Henderson and Wilson, 1970; Muyzer and Stams, 2008), and Desulfovibrio salexigens (Postgate and Campbell, 1966; van Niel et al., 1996) were detected at all stations. Archaeal genes, which could potentially harbor diazotrophic methanogens, were not detected. One cluster was related to the facultative anaerobe Vibrio diazotrophicus, which was found at 236 m (up to 4% between 4 and 5 cm) and in low sequence abundances (1%) at 412 m. Several sequences were phylogenetically related to uncultured microorganisms and were found at all sites, e.g., a γ-proteobacterial clone (Langlois et al., 2015), which had its highest abundance in the sequence pool (>6%) at 46 m between 2 and 3 cm and an uncultured diazotroph (Ribes et al., 2015), that was found in highest sequence abundance (>6% of all sequences) at 90 m between 2 and 3 cm sediment depth.
Figure 4. Phylogenetic tree of expressed nifH genes based on the analysis of ~ 8000 sequences and respective abundance from the total data set (see legend of the color code on the right). The six sampling stations are shown with the corresponding sediment depth (cm). The scale bar represents 10% estimated sequence divergence.
Benthic N2 fixation was detected at all sampling sites and in all sediment depths (Figure 4). No activity was detected in killed controls. In general, N2 fixation had low activities at the sediment surface, increased in deeper layers and decreased to the bottom of the core. Highest surface N2 fixation was measured at the three shallow sites (47–236 m, between 0.52 ± 0.09 and 0.57 ± 0.03 nmol N2 cm−3 d−1), while the lowest surface activity was measured at the three deep sites (412–1,108 m, between 0.1 ± 0.04 and 0.25 ± 0.02 nmol N2 cm−3 d−1). Because molecular analyses of the nifH gene indicated the involvement of both iron-reducing and sulfate-reducing bacteria in benthic N2 fixation, we compared the distribution of N2 fixation with the concentration of ferrous iron (produced reductive iron dissolution) and the activity of sulfate reduction in more detail.
The ferrous iron porewater profiles showed peaks between 0 and 10 cm at all stations, except at 412 m, where ferrous iron increased with sediment depth (Figure 5). At 47 m and 90 m ferrous iron profiles had concentration peaks at 4 and 3 cm (22 and 17 μM), which were slightly below the N2 fixation peaks at 5–6 and 3–4 cm (1.17 ± 0.06 and 1.6 ± 0.04 nmol N2 cm−3 d−1), respectively. At 236 m ferrous iron followed the N2 fixation depth profile with overlapping peaks (31 μM, 0.85 ± 0.05 nmol N2 cm−3 d−1) at 3–5 cm. The highest ferrous iron concentration (49 μM) of all stations was measured at 412 m, which did not overlap with N2 fixation. At 786 m, ferrous iron concentrations peaked (30 μM) at 1–6 cm, which overlapped with a peak in N2 fixation (0.57 ± 0.02 nmol N2 cm−3 d−1) at 2–8 cm. A similar depth profile of both was also detected at the 1108 m site. Ferrous iron peaked (27 μM) at 3–9 cm, which coincided with an activity peak of N2 fixation (0.66 ± 0.05 nmol N2 cm−3 d−1) at 4–5 cm.
Figure 5. Depth profiles of N2 fixation (nmol N2 cm−3 d−1, gray triangles, average of three replicates plus standard deviation), sulfate reduction rates (SR, nmol cm−3 d−1, green diamonds, single measurements) and ferrous iron porewater concentrations (Fe2+, μM, brown dots) between 0 and 20 cm at the six stations in Table 1. N2 fixation scale is shown on the upper x-axis, while sulfate reduction and ferrous iron concentration are reported on the lower x-axis.
Sulfate reduction and N2 fixation rates were high at the shallow sites (46, 90, and 236 m), and low at the deep sites (412, 768, and 1,108 m). At most stations, N2 fixation and sulfate reduction rates were low at the top and at the bottom of the cores, with N2 fixation peaks between 3 and 8 cm and sulfate reduction maxima between 9 and 14 cm (Figure 5). At 47 m, N2 fixation and sulfate reduction showed non-conforming profiles in the sediment surface, but aligned toward the bottom of the core. A matching peak (0.71 ± 0.11 nmol N2 cm−3 d−1, 19.3 nmol cm−3 d−1) was observed at 12–14 cm. This site had the highest (88 μM, 15 cm) sulfide concentration. At 90 m, N2 fixation did not overlap with sulfate reduction activity. N2 fixation peaked (1.1 ± 0.03 nmol N2 cm−3 d−1) at 3–4 cm, while sulfate reduction peaked (37–43 nmol cm−3 d−1) at 7–12 cm. The 236 m site, showed overlapping peaks of N2 fixation and sulfate reduction (0.85 ± 0.05 nmol N2 cm−3 d−1, 12 nmol cm−3 d−1) at 3–4 cm. No overlap in activities was observed at the 412 m site. Highest N2 fixation was measured at 3–4 cm (0.68 ± 0.07 nmol N2 cm−3 d−1), while the highest sulfate reduction (17.5 nmol cm−3 d−1) was measured at 10–11 cm. Station 786 m had an unusually high sulfate reduction rate (295 nmol cm−3 d−1, 13–14 cm), which was not detected in N2 fixation. At 1,108 m, N2 fixation and sulfate reduction had corresponding depth profiles from the surface down to 8 cm. While sulfate reduction had a second, higher activity peak at 9–10 cm (70 nmol cm−3 d−1), N2 fixation showed a continuous decrease below the peak at 6 cm. No activity was detected in killed sulfate reduction controls.
Integrated (0–20 cm) N2 fixation rates did not track integrated (0–20 cm) ferrous iron concentrations along the depth transect (Figure 6). While N2 fixation was highest (0.15 ± 0.004 mmol N2 m−2 d−1) at 90 m, integrated ferrous iron was lowest (0.8 μmol m−2). Further, at 412 m integrated N2 fixation was lowest (0.08 ± 0.002 mmol N2 m−2 d−1) and integrated ferrous iron was highest (4.2 μmol m−2). The average (0–20 cm, n = 10–20) organic carbon content increased from the shelf (46 m, 0.8 wt%) down the continental margin with the highest value (2.9 wt%) at 1,108 m. At this site, N2 fixation and the ferrous iron concentration had low and medium values, respectively (0.08 ± 0.002 mmol N2 m−2 d−1, 2.0 μmol m−2).
Figure 6. Top: Bottom water O2 (μM) and average (0–20 cm, n = 10–20) particulate organic carbon content (Corg, wt%) along the depth transect. Bottom: Integrated nitrogen fixation (mmol N2 m−2 d−1, gray bars, average of three replicates plus standard deviation), sulfate reduction rate (mmol m−2 d−1, green bars, single measurements) and average ferrous iron concentration (Fe2+, μmol m−2, brown bars) between 0 and 20 cm along the depth transect (m).
Integrated N2 fixation roughly followed integrated (0–20 cm) sulfate reduction rates from 46 to 1,108 m (Figure 6). Both rates were highest (0.15 ± 0.004 mmol N2 m−2 d−1, 4.2 mmol m−2 d−1) at 90 m and lowest at 412 m (0.08 ± 0.002 mmol N2 m−2 d−1, 1.4 mmol m−2 d−1). Likewise, the averaged organic carbon content did not track the sulfate reduction activities along the depth transect.
The Pearson correlation coefficient of vertical depth profiles (Table 2A) detected a moderate positive relationship between N2 fixation and sulfate reduction (r = 0.43). A moderate negative correlation was detected between N2 fixation and the variables organic carbon (r = −0.45) and nitrate (r = −0.50). Low negative (r = −0.2) and low positive (r = 0.15) correlations were found between N2 fixation and the organic C/N ratio and sulfide, respectively. No correlation was identified between N2 fixation and ammonium and ferrous iron. The regression analysis (Table S2a) detected a significance between N2 fixation and sulfate reduction and organic carbon content (p = 0.0142 and p = 0.0001, respectively).
Table 2. Statistical analysis of Pearson correlation coefficients including r-values along (a) vertical depth profiles, and (b) integrated rates and means of environmental variables.
The Pearson correlation coefficient of integrated N2 fixation (Figure 3B) found high negative relationships between N2 fixation and organic carbon (r = −0.81) and ferrous iron (r = −0.77), while a high positive relationship was observed between N2 fixation and bioirrigation (r = 0.71). N2 fixation and sulfate reduction rates, as well as sulfide, indicated a moderate positive correlation (r = 0.43 and r = 0.54, respectively). Moderate negative correlations were identified between N2 fixation and variables C/N ratio (r = −0.52) and nitrate (r = −0.54). N2 fixation and ammonium (r = −0.31), as well as N2 fixation and bottom water oxygen (r = −0.37) had low negative correlations. The regression analysis (Table S2b) found a significant p-value (p = 0.0258) for the predictor integrated N2 fixation and the response variable ferrous iron.
For details on Pearson correlation coefficients of environmental variables among each other, see Table 2. For details on p-values see Table S2.
We explored the relationship between indicators of diazotrophic activity and heterotrophic metabolisms in sediments along the vertical sedimentary redox gradient. One working hypothesis is that benthic N2-fixation in the OMZ off Mauritania is linked to heterotrophic bacteria, whose activity is controlled by the availability of organic matter. Oxygen (aerobic respiration) was excluded from the following discussion, as it is a known inhibitor for N2 fixation (Postgate, 1998; Dixon and Kahn, 2004); it will be revisited in the bioirrigation section below.
At all stations, nitrate was present in the top 1–2 cm sediment depth (Figure 2) and N2 fixation was low close to the sediment-water interface (Figure 5), suggesting a negative correlation of nitrate with N2 fixation by the absence of an ecological niche for diazotrophs (Bertics et al., 2010). This negative relationship was identified statistically (Table 2) and phylogenetically, as denitrifying bacteria did not cluster with nifH gene sequences (Figure 4). Altogether, it seems that denitrification was not related to N2 fixation in the investigated sediments. Remarkably, nifH sequences also clustered with V. diazotrophicus, which reduces nitrate to nitrite and which has previously shown to be capable of N2 fixation (Guerinot et al., 1982). Similar sequences were found in the Peruvian OMZ sediment (Gier et al., 2016) and water column (Löscher et al., 2014). Furthermore, no manganese reducers were detected by the nifH analysis (Figure 4).
Ferrous iron accumulation in porewater, an indicator for the microbial iron reduction zone (Vandieken et al., 2006), mostly showed an overlapping distribution with N2 fixation activity at all stations (Figure 5). In accordance with this finding, some nifH gene sequences (2 %) clustered with P. carbinolicus (Figure 4), which uses iron and sulfur as electron acceptors (Lovley et al., 1995). This organism was previously shown to be involved in N2 fixation in subtidal sediments of Narragansett Bay (Rhode Island) (Fulweiler et al., 2013), as well as in bioturbated muddy sand sediments at Catalina Island (California) (Bertics et al., 2010). Most remarkably, the sequences related to P. carbinolicus coincided with a ferrous iron peak at the 412 m (Figure 4) and 1,108 m site (Figure 5), indicating a potential involvement of iron-reducing bacteria in N2 fixation. However, the Pearson correlation analysis did not identify a relationship between vertical profiles of N2 fixation and ferrous iron, and even detected a highly negative correlation for integrated N2 fixation and ferrous iron along with a p < 0.5, pointing to more influential environmental control mechanisms or to more complex interactions. In addition to the known heterotrophs discussed above, we recognized several new nifH clusters in the 18°N depth transect, that have not been identified, yet (Figure 4), highlighting the diversity of diazotrophs in marine sediments.
Vertical activity of sulfate reduction and benthic N2 fixation generally overlapped (Figure 5) and integrated rates of both processes revealed similar trends along the depth transect (Figure 6). Overall, the phylogenetic analysis of diazotrophs indicated a potential role of sulfate-reducing bacteria for N2 fixation in Mauritanian sediments. Sequences clustered with several sulfate reducers related to Desulfovibrio spp., which was found earlier to be involved in benthic N2 fixation (Bertics et al., 2013; Fulweiler et al., 2013; Gier et al., 2016). Likewise, nifH gene analysis indicated a potential link between N2 fixation and sulfate reduction in OMZ sediments off Peru (Gier et al., 2016). However, the Pearson correlation coefficient identified only a low positive relationship between the two processes with a p-value of p = 0.43. Moreover, individual peaks of N2 fixation and sulfate reduction often did not match (Figure 5). Our analyses indicate that N2 fixation could be partially associated with sulfate reducers, but at the same time, each process may be influenced by different environmental factors. It should, however, be emphasized that N2 fixation, sulfate reduction, and porewater data were each determined from different replicate MUC cores with a sampling distance of up to 50 cm. Lateral heterogeneity and vertical gradients could obscure the actual correlations. Finally, it should be noted that the addition of acetylene could have induced a shift in the benthic diazotrophic community, as it has been previously demonstrated using high-throughput sequencing of sediments with and without the addition of acetylene (Fulweiler et al., 2015). Nevertheless, we expect that a community shift would rather lead to an underestimation of absolute N2 fixation rates.
Burrowing sediment infauna has a significant impact on sediment biogeochemistry through its bioturbating (sediment mixing) and bioirrigating (sediment flushing) activity (Kristensen et al., 2012). Mauritanian sediments <400 m are classified as permeable sands inhabited by burrowing macrofauna (Mosch et al., 2012) whose irrigation activity was quantified, here, using an inert tracer (Figure 3). It should be noted that even though we did not measure bioturbation directly, we expect bioturbation and bioirrigation to be closely coupled (Aller and Aller, 1986; Kristensen, 2000).
N2 fixation was repeatedly low in the sediment surface (0–2 cm) and increased only in deeper layers (Figure 5). This observation coincided with the intersection of oxidized water with the seafloor (O2 > 30 μM; up to 123 and 138 μM at 47 and 1,108 m, respectively; Figures 1, 2), and with bioirrigation. These factors together could lead to a relatively deep penetration of O2 into the sediment (Revsbech et al., 1980; Ziebis et al., 1996; Kristensen, 2000; Bertics and Ziebis, 2009). O2 is a known inhibitor of the nitrogenase enzyme (Postgate, 1998; Dixon and Kahn, 2004) and an oxic layer at the sediment surface would potentially suppress N2 fixation activity. The Pearson correlation coefficient points to a low negative relationship between integrated N2 fixation rates and bottom water O2 concentrations, suggesting a potential minor impact of the water column O2 concentrations on diazotrophic activity. Alternatively, several marine diazotrophs have developed strategies to protect the nitrogenase from O2 (Jørgensen, 1977; Krekeler et al., 1998; Cypionka, 2000; Muyzer and Stams, 2008).
In contrary to the inhibiting effect of bioirrigation through the introduction of O2, burrowing organisms can also increase microbial activity in sediments by facilitating burial of organic matter (Aller and Aller, 1986; Christensen et al., 2000; Bertics and Ziebis, 2010; Kristensen et al., 2012). In fact, the organic carbon content was found to be one of the most essential environmental factors that control benthic diazotrophs (Hartwig and Stanley, 1978; Jørgensen, 1983; Howarth et al., 1988; Fulweiler et al., 2007; Gier et al., 2016). However, while a positive correlation between N2 fixation and organic carbon content was identified for the Peruvian OMZ sediments (Gier et al., 2016), a negative correlation was detected for Mauritania OMZ sediments (Table 2). The Pearson correlation coefficient results also showed a low to moderate negative relationship between N2 fixation and the C/N ratio (Table 2). In addition, burrowing animals can change redox processes by providing additional electron acceptors into the sediment and thereby creating favorable microniches for benthic diazotrophs (Gundersen and Jørgensen, 1990; Ziebis et al., 1996). The potential removal of ammonium by nitrification may provide favorable microniches for N2 fixation (Wenzhöfer and Glud, 2004; Zorn et al., 2006; Bertics et al., 2010) even in the presence of O2 (Krekeler et al., 1998; Cypionka, 2000; Muyzer and Stams, 2008). Diazotrophs are regarded as being inhibited by high concentrations of (Knapp, 2012 and references therein); however, it remains unknown, why N2 fixation is sometimes still found at high concentrations of a bioavailable inorganic N species despite its high energetically costs (Capone, 1988). Given the many positive effects, bioirrigation can have on N2 fixation, we expected N2 fixation to be overall enhanced at stations with high bioirrigation signals and to find niches of N2 fixation in deeper sediment layers as a result of organic matter burial. Indeed, integrated rates of N2 fixation were high at the shallow sites (47–236 m) (Figure 6), coinciding with the highest integrated bioirrigation rates (Figure 3B). Additionally, the Pearson correlation coefficient identified a strong positive relationship between N2 fixation and bioirrigation (Table 2B), but with a p-value of 0.3 (Table S2b) indicating no significance. Moreover, no evidence for microniches of elevated N2 fixation in deeper sediment layers was found. Only the sulfate reduction peak observed at the 90 m site (8–12 cm, Figure 5) coincided with high bioirrigation at this station and could be a result of organic matter introduction by burrowing activity; however, no anomalies were detected in the profiles for organic matter content or C/N ratio (Figure 2). In fact, the Pearson correlation coefficient found a moderate and strong negative relationship between N2 fixation and organic matter depth profiles (p = −0.45) and integrated rates (p = 0.81), respectively, in sediments (Tables 2A,B). In contrast, organic matter and integrated N2 fixation correlated well in Peruvian OMZ sediments (Gier et al., 2016). However, Peruvian sediments and Mauritanian sediments have different environmental characteristics. While the Peruvian sediments are considered as organic-rich mud with an organic carbon content up to 15 wt% (Dale et al., 2015), Mauritanian sediments are more sandy with lower organic carbon content. Sandy sediments display high metabolic activity with rapid organic carbon oxidation (Boudreau and Westrich, 1984; Huettel et al., 2003). Overall, these results suggest that the organic carbon content and the C/N ratio are not the major factors controlling N2 fixation in Mauritanian sediments. As another study showed, the increase of organic matter does not necessary lead to an increase of N2 fixation. Fulweiler et al. (2007) observed a switch from denitrification (N loss) to N2 fixation (N gain) in the sediments of Narrangaset Bay caused by a lower organic matter deposition to the sediments.
The Mauritanian sediments are regarded as sink for dissolved inorganic N, with denitrification being the main N removal process (Dale et al., 2014). In order to determine the relevance of benthic N2 fixation in the Mauritanian OMZ, we compared N2 fixation rates determined in this study with denitrification rates from Dale et al. (2014) and Sokoll et al. (2016). Denitrification by Dale et al. (2014) was investigated along a similar depth transect (18°N) and in similar water depth. Denitrification at the shelf site (98 m) was 1.8 mmol m−2 d−1, while the rate was 0.2 mmol m−2 d−1 at the deepest site (1108 m). Benthic integrated N2 fixation at the corresponding sites was highest (0.15 ± 0.004 mmol m−2 d−1) on the shelf and lowest (0.08 ± 0.002 mmol m−2 d−1) at the deepest site. Calculating the above mentioned N source and sink processes, benthic N2 fixation could compensate for about 8–40% of the N loss between the shelf and the deepest site, respectively.
However, in the study by Sokoll et al. (2016) denitrification rates along the 18°N transect were found to be higher at similar water depths (53–787 m) and significantly correlated with grain size, a parameter that was not investigated during our sampling survey. Comparing the highest N2 fixation (90 m, 0.15 ± 0.004 mmol m−2 d−1) with the highest denitrification rate (96 m, 4.24 mmol m−2 d−1; Sokoll et al., 2016), as well as the lowest denitrification (787 m, 0.77 mmol m−2 d−1; Sokoll et al., 2016) with the corresponding N2 fixation rate, results in a lower compensation when compared to denitrification rates determined by Dale et al. (2014). According to the findings of Sokoll et al. (2016), benthic N2 fixation would compensate for only 4–10% of the N loss by denitrification. In summary, diazotrophs in the Mauritanian OMZ sediments may have, at certain sites, a considerable attenuating effect on the loss of fixed N from the benthic environment.
As OMZs are predicted to increase globally (Diaz, 2001; Stramma et al., 2008; Keeling et al., 2010), it is crucial to understand their biogeochemical processes and feedbacks and make predictions how relatively oxygenated areas, such as the Mauritanian OMZ, will be affected if O2 becomes further depleted, as long term trends indicate (Stramma et al., 2008). A comparison between the eastern tropical north Atlantic OMZ off Mauritania and the eastern tropical south Pacific OMZ off Peru (Gier et al., 2016) should aid in our understanding of the magnitude of N2 fixation rates in O2 deficient environments and the relevant environmental factors (Table 3).
Table 3. Integrated (0–20 cm) rates of N2 fixation and sulfate reduction (SR) from 0 to 20 cm from this study compared to the Peruvian OMZ (from Gier et al., 2016) as well as environmental parameters.
Integrated (0–20 cm) benthic N2 fixation rates from the Mauritanian upwelling were lower (0.08–0.15 mmol m−2 d−1) than those reported for the Peruvian OMZ (0.01–0.41 mmol m−2 d−1). Off Mauritania, N2 fixation peaked deeper than 2 cm sediment depth, while N2 fixation off Peru was highest in the surface sediments (0–2 cm). Overall, statistical analysis revealed that benthic diazotrophs off Peru were positively correlated with sulfate reduction and organic matter. Our results from Mauritania instead show that there is no single environmental variable controlling diazotrophs, pointing to more subtle relationships including a positive correlation with sulfate reduction and negative correlation with organic matter content. In addition, the filamentous nitrate-storing sulfide-oxidizing bacteria of the genus Thioploca have been observed to densely colonize Peruvian OMZ sediments (Schulz and Jørgensen, 2001; Sommer et al., 2016). These bacteria perform dissimilatory nitrate reduction to ammonium (DNRA) and prevent the accumulation of sulfide in sediments (Fossing et al., 1995; Zopfi et al., 2001; Bohlen et al., 2011). Thus, sediments off Peru represent a recycling site for dissolved inorganic N, releasing high amounts of ammonium into the water column (Bohlen et al., 2011). The interplay between N2 fixation in predominantly N-recycling vs. denitrifying sediments deserves further attention in future studies.
Our findings add to the growing knowledge of benthic N cycling in upwelling regions and will aid in our understanding of potential environmental factors that control benthic diazotrophs. N2 fixation occurred throughout the sediment and activity widely coincided with sulfate reduction activity. This result was supported by molecular analysis of the nifH gene, which confirmed the presence of several sulfate-reducing bacteria related to Desulfovibrio spp. Molecular analysis further pointed toward a role of iron-reducing bacteria for benthic diazotrophy and N2 fixation, which overlapped with the presence of ferrous iron in the sediment porewater. However, none of the above correlations between N2 fixation and sulfate or iron reduction were supported by statistical analyses. We further found no significant effect of bioirrigation on benthic diazotrophs. Our inability to unambiguously correlate observed biogeochemical and environmental parameters to N2 fixation highlights that potentially more complex interactions with multiple effects exist in the field. A comparison with denitrification rates from the same study area highlighted the ability of benthic diazotrophs to counteract between 4 and 40% of the benthic N loss. Overall, benthic N2 fixation in the sediments below the weak Mauritanian OMZ was at least one-third of the predominantly anoxic Peruvian OMZ.
JG and TT: designed the study; JG: performed nitrogen fixation experiments; TT: conducted sulfate reduction experiments; SS and AD: measured fluxes, performed bioirrigation experiments, modeling, and corresponding data analysis; JG, TT, and CL: analyzed the microbial rate data; JG and CL: performed molecular and statistical analysis; UL: measured porewater iron; JG: prepared the manuscript with contributions from all co-authors.
The authors declare that the research was conducted in the absence of any commercial or financial relationships that could be construed as a potential conflict of interest.
We would like to thank the captain and the crew of the RV Meteor cruise M107, as well as S. Kriwanek, A. Petersen and M. Türk of the GEOMAR Technology and Logistics Center, for all of their assistance in field sampling. We also thank B. Domeyer, A. Bleyer, U. Lomnitz, R. Suhrberg, S. Trinkler, and V. Thoenissen for supporting the geochemical analyses. Additional thanks goes to the members of the Treude and Schmitz-Streit working groups, especially V. Bertics for her methological guidance, G. Schuessler, P. Wefers, and B. Mensch for their laboratory assistance and to J. Maltby and S. Krause for scientific discussions. We further thank the authorities of Mauritania for the permission to work in their territorial waters. This study is a contribution of the Sonderforschungsbereich 754 “Climate - Biogeochemistry Interactions in the Tropical Ocean,” which is supported by the German Research Foundation. CL received additional funding from the European Union H2020 (NITROX, #704274), the DFG-funded cluster of excellence “The Future Ocean,” and the Danish Institute for Advanced Study (D-IAS).
The Supplementary Material for this article can be found online at: https://www.frontiersin.org/articles/10.3389/fmars.2017.00390/full#supplementary-material
Aller, J. Y., and Aller, R. C. (1986). Evidence for localized enhancement of biological activity associated with tube and burrow structures in deep-sea sediments at the HEBBLE site, western North Atlantic. Deep Sea Res. 33, 755–790. doi: 10.1016/0198-0149(86)90088-9
Baker, A. R., Jickells, T. D., Witt, M., and Linge, K. L. (2006). Trends in the solubility of iron, aluminium, manganese and phosphorus in aerosol collected over the Atlantic Ocean. Mar. Chem. 98, 43–58. doi: 10.1016/j.marchem.2005.06.004
Barton, E. D., Aristegui, J., Tett, P., Cantón, M., Garcia-Braun, J., Hernández-León, S., et al. (1998). The transition zone of the canary current upwelling region. Prog. Oceanogr. 41, 455–504. doi: 10.1016/S0079-6611(98)00023-8
Bentzon-Tilia, M., Traving, S. J., Mantikci, M., Knudsen-Leerbeck, H., Hansen, J. L. S., Markager, S., et al. (2015). Significant N2 fixation by heterotrophs, photoheterotrophs and heterocystous cyanobacteria in two temperate estuaries. ISME J. 9, 273–285. doi: 10.1038/ismej.2014.119
Bertics, V. J., and Ziebis, W. (2009). Biodiversity of benthic microbial communities in bioturbated coastal sediments is controlled by geochemical microniches. ISME J. 3, 1269–1285. doi: 10.1038/ismej.2009.62
Bertics, V. J., and Ziebis, W. (2010). Bioturbation and the role of microniches for sulfate reduction in coastal marine sediments. Environ. Microbiol. 12, 3022–3034. doi: 10.1111/j.1462-2920.2010.02279.x
Bertics, V. J., Löscher, C. R., Salonen, I., Dale, A. W., Gier, J., Schmitz, R. A., et al. (2013). Occurrence of benthic microbial nitrogen fixation coupled to sulfate reduction in the seasonally hypoxic Eckernförde Bay, Baltic Sea. Biogeosciences 10, 1243–1258. doi: 10.5194/bg-10-1243-2013
Bertics, V. J., Sohm, J. A., Magnabosco, C., and Ziebis, W. (2012). Denitrification and nitrogen fixation dynamics in the area surrounding an individual ghost shrimp (Neotrypaea californiensis) burrow system. Appl. Environ. Microbiol. 78, 3864–3872. doi: 10.1128/AEM.00114-12
Bertics, V. J., Sohm, J., Treude, T., Chow, C., Capone, D., Fuhrman, J., et al. (2010). Burrowing deeper into benthic nitrogen cycling: the impact of bioturbation on nitrogen fixation coupled to sulfate reduction. Mar. Ecol. Prog. Ser. 409, 1–15. doi: 10.3354/meps08639
Bohlen, L., Dale, A. W., Sommer, S., Mosch, T., Hensen, C., Noffke, A., et al. (2011). Benthic nitrogen cycling traversing the Peruvian oxygen minimum zone. Geochim. Cosmochim. Acta 75, 6094–6111. doi: 10.1016/j.gca.2011.08.010
Boudreau, B. P., and Westrich, J. T. (1984). The dependence of bacterial sulfate reduction on sulfate concentration in marine sediments. Geochim. Cosmochim. Acta 48, 2503–2516. doi: 10.1016/0016-7037(84)90301-6
Boudreau, B. P., Huettel, M., Forster, S., Jahnke, R. A., McLachlan, A., Middelburg, J. J., et al. (2001). Permeable marine sediments: overturning an old paradigm. EOS 82, 133–136. doi: 10.1029/EO082i011p00133-01
Brandes, J. A., and Devol, A. H. (2002). A global marine-fixed nitrogen isotopic budget: implications for holocene nitrogen cycling. Glob. Biogeochem. Cycles 16, 1–14. doi: 10.1029/2001GB001856
Capone, D. G. (1988). “Benthic nitrogen fixation,” in Nitrogen Cycling in Coastal Marine Environments, eds T. H. Blackburn and J. Sorensen (New York, NY: John Wiley & Sons Ltd.), 85–123.
Capone, D. G. (1993). “Determination of nitrogenase activity in aquatic samples using the acetylene reduction procedure,” in Handbook of Methods in Aquatic Microbial Ecology, eds P. F. Kemp, B. F. Sherr, E. B. Sherr, and J. J. Coles (Boca Raton, FL: CRC Press LLC), 621–631.
Capone, D. G., Burns, J. A., Montoya, J. P., Subramaniam, A., Mahaffey, C., Gunderson, T., et al. (2005). Nitrogen fixation by Trichodesmium spp.: an important source of new nitrogen to the tropical and subtropical North Atlantic Ocean. Glob. Biogeochem. Cycles 19, 1–17. doi: 10.1029/2004GB002331
Carr, M.-E. (2001). Estimation of potential productivity in eastern boundary currents using remote sensing. Deep Sea Res. II Top. Stud. Oceanogr. 49, 59–80. doi: 10.1016/S0967-0645(01)00094-7
Chavez, F. P., and Messié, M. (2009). A comparison of Eastern boundary upwelling ecosystems. Prog. Oceanogr. 83, 80–96. doi: 10.1016/j.pocean.2009.07.032
Christensen, B., Vedel, A., and Kristensen, E. (2000). Carbon and nitrogen fluxes in sediment inhabited by suspension-feeding (Nereis diversicolor) and non-suspension-feeding (N. virens) polychaetes. Mar. Ecol. Prog. Ser. 192, 203–217. doi: 10.3354/meps192203
Cypionka, H. (2000). Oxygen respiration by desulfovibrio species. Annu. Rev. Microbiol. 54, 827–848. doi: 10.1146/annurev.micro.54.1.827
Dale, A. W., Sommer, S., Bohlen, L., Treude, T., Bertics, V. J., Bange, H. W., et al. (2011). Rates and regulation of nitrogen cycling in seasonally hypoxic sediments during winter (Boknis Eck, SW Baltic Sea): sensitivity to environmental variables. Estuar. Coas. Shelf Sci. 95, 14–28. doi: 10.1016/j.ecss.2011.05.016
Dale, A. W., Bertics, V. J., Treude, T., Sommer, S., and Wallmann, K. (2013). Modeling benthic-pelagic nutrient exchange processes and porewater distributions in a seasonally-hypoxic sediment: evidence for massive phosphate release by Beggiatoa? Biogeosciences 10, 629–651. doi: 10.5194/bg-10-629-2013
Dale, A. W., Sommer, S., Lomnitz, U., Montes, I., Treude, T., Liebetrau, V., et al. (2015). Organic carbon production, mineralisation and preservation on the Peruvian margin. Biogeosciences 12, 1537–1559. doi: 10.5194/bg-12-1537-2015
Dale, A. W., Sommer, S., Ryabenko, E., Noffke, A., Bohlen, L., Wallmann, K., et al. (2014). Benthic nitrogen fluxes and fractionation of nitrate in the Mauritanian oxygen minimum zone (Eastern Tropical North Atlantic). Geochim. Cosmochim. Acta 134, 234–256. doi: 10.1016/j.gca.2014.02.026
Diaz, R. J. (2001). Overview of hypoxia around the world. J. Environ. Qual. 30, 275–281. doi: 10.2134/jeq2001.302275x
Dixon, R., and Kahn, D. (2004). Genetic regulation of biological nitrogen fixation. Nat. Rev. Microbiol. 2, 621–631. doi: 10.1038/nrmicro954
Edgar, R. C., Haas, B. J., Clemente, J. C., Quince, C., and Knight, R. (2011). UCHIME improves sensitivity and speed of chimera detection. Bioinformatics 27, 2194–2200. doi: 10.1093/bioinformatics/btr381
Fossing, H., Gallardo, V. A., Jørgensen, B. B., Hüttel, M., Nielsen, L. P., Schulz, H., et al. (1995). Concentration and transport of nitrate by the mat-forming sulphur bacterium Thioploca. Nature 374, 713–715. doi: 10.1038/374713a0
Fulweiler, R. W., Heiss, E. M., Rogener, M. K., Newell, S. E., LeCleir, G. R., Kortebein, S. M., et al. (2015). Examining the impact of acetylene on N-fixation and the active sediment microbial community. Front. Microbiol. 6:418. doi: 10.3389/fmicb.2015.00418
Fulweiler, R. W., Nixon, S. W., Buckley, B. A., and Granger, S. L. (2007). Reversal of the net dinitrogen gas flux in coastal marine sediments. Nature 448, 180–182. doi: 10.1038/nature05963
Fulweiler, R., Brown, S., Nixon, S., and Jenkins, B. (2013). Evidence and a conceptual model for the co-occurrence of nitrogen fixation and denitrification in heterotrophic marine sediments. Mar. Ecol. Prog. Ser. 482, 57–68. doi: 10.3354/meps10240
Galloway, J. N., Dentener, F. J., Capone, D. G., Boyer, E. W., Howarth, R. W., Seitzinger, S. P., et al. (2004). Nitrogen cycles: past, present, and future. Biogeochemistry 70, 153–226. doi: 10.1007/s10533-004-0370-0
Gier, J., Sommer, S., Löscher, C. R., Dale, A. W., Schmitz, R. A., and Treude, T. (2016). Nitrogen fixation in sediments along a depth transect through the Peruvian oxygen minimum zone. Biogeosciences 13, 4065–4080. doi: 10.5194/bg-13-4065-2016
Grasshoff, K., Kremlingl, K., and Ehrhardt, M. (1999). Methods of Seawater Analysis, 3rd Edn. Weinheim: Wiley–VCH.
Griffen, B., DeWitt, T., and Langdon, C. (2004). Particle removal rates by the mud shrimp Upogebia pugettensis, its burrow, and a commensal clam: effects on estuarine phytoplankton abundance. Mar. Ecol. Prog. Ser. 269, 223–236. doi: 10.3354/meps269223
Gruber, N. (2008). “The marine nitrogen cycle: overview and challenges,” in Nitrogen in the Marine Environment, 2nd Edn., eds D. G. Capone, D. A. Bronk, M. R. Mulholland, and E. J. Carpenter (Amsterdam: Elsevier), 1–50.
Guerinot, M. L., West, P. A., Lee, J. V., and Colwell, R. R. (1982). Vibrio diazotrophicus sp. nov., a marine nitrogen-fixing bacterium. Int. J. Syst. Bacteriol. 32, 350–357. doi: 10.1099/00207713-32-3-350
Gundersen, J. K., and Jørgensen, B. B. (1990). Microstructure of diffusive boundary layers and the oxygen uptake of the sea floor. Lett. Nat. 345, 604–607. doi: 10.1038/345604a0
Hartwig, E. O., and Stanley, S. O. (1978). Nitrogen fixation in Atlantic deep-sea and coastal sediments. Deep Sea Res. 25, 411–417. doi: 10.1016/0146-6291(78)90567-2
Howarth, R. W., Marino, R., Lane, J., and Cole, J. J. (1988). Nitrogen fixation in freshwater, estuarine, and marine ecosystems. 1. rates and importance. Limnol. Oceanogr. 33, 669–687. doi: 10.4319/lo.1988.33.4_part_2.0669
Huettel, M., and Rusch, A. (2000). Transport and degradation of phytoplankton in permeable sediment. Limnol. Oceanogr. 45, 534–549. doi: 10.4319/lo.2000.45.3.0534
Huettel, M., Roy, H., Precht, E., and Ehrenhauss, S. (2003). Hydrodynamical impact on biogeochemical processes in aquatic sediments. Hydrobiologia 494, 231–236. doi: 10.1023/A:1025426601773
Huntsman, S. A., and Barber, R. T. (1977). Primary production off northwest Africa: the relationship to wind and nutrient conditions. Deep Sea Res. 24, 25–33. doi: 10.1016/0146-6291(77)90538-0
Janssen, F., Huettel, M., and Witte, U. (2005). Pore-water advection and solute fluxes in permeable marine sediments (II): benthic respiration at three sandy sites with different permeabilities (German Bight, North Sea). Limnol. Oceanogr. 50, 779–792. doi: 10.4319/lo.2005.50.3.0779
Jørgensen, B. B. (1977). The sulfur cycle of a coastal marine sediment (Limfjorden, Denmark). Limnol. Oceanogr. 22, 814–832. doi: 10.4319/lo.1977.22.5.0814
Jørgensen, B. B. (1978). A comparison of methods for the quantification of bacterial sulfate reduction in coastal marine sediments. Geomicrobiol. J. 1, 11–27. doi: 10.1080/01490457809377721
Jørgensen, B. B. (1983). “SCOPE 21 -The major biogeochemical cycles and their interactions,” in Processes at the Sediment-Water Interface, eds B. Bolin and R. Cook (New York, NY: John Wiley & Sons).
Kallmeyer, J., Ferdelman, T. G., Weber, A., Fossing, H., and Jørgensen, B. B. (2004). Evaluation of a cold chromium distillation procedure for recovering very small amounts of radiolabeled sulfide related to sulfate reduction measurements. Limnol. Oceanogr. Methods 2, 171–180. doi: 10.4319/lom.2004.2.171
Karstensen, J., Stramma, L., and Visbeck, M. (2008). Oxygen minimum zones in the eastern tropical Atlantic and Pacific oceans. Prog. Oceanogr. 77, 331–350. doi: 10.1016/j.pocean.2007.05.009
Keeling, R. E., Körtzinger, A., and Gruber, N. (2010). Ocean deoxygenation in a warming world. Ann. Rev. Mar. Sci. 2, 199–229. doi: 10.1146/annurev.marine.010908.163855
Knapp, A. N. (2012). The sensitivity of marine N2 fixation to dissolved inorganic nitrogen. Front. Microbiol. 3:374. doi: 10.3389/fmicb.2012.00374
Kozich, J. J., Westcott, S. L., Baxter, N. T., Highlander, S. K., and Schloss, P. D. (2013). Development of a dual-index sequencing strategy and curation pipeline for analyzing amplicon sequence data on the MiSeq Illumina sequencing platform. Appl. Environ. Microbiol. 79, 5112–5120. doi: 10.1128/AEM.01043-13
Krekeler, D., Teske, A., and Cypionka, H. (1998). Strategies of sulfate-reducing bacteria to escape oxygen stress in a cyanobacterial mat. FEMS Microbiol. Ecol. 25, 89–96. doi: 10.1111/j.1574-6941.1998.tb00462.x
Kristensen, E. (2000). Organic matter diagenesis at the oxic/anoxic interface in coastal marine sediments, with emphasis on the role of burrowing animals. Hydrobiologia 426, 1–24. doi: 10.1023/A:1003980226194
Kristensen, E., Penha-Lopes, G., Delefosse, M., Valdemarsen, T., Quintana, C., and Banta, G. (2012). What is bioturbation? The need for a precise definition for fauna in aquatic sciences. Mar. Ecol. Prog. Ser. 446, 285–302. doi: 10.3354/meps09506
Langlois, R., Großkopf, T., Mills, M., Takeda, S., and LaRoche, J. (2015). Widespread distribution and expression of Gamma A (UMB), an uncultured, diazotrophic, γ-proteobacterial nifH phylotype. PLoS ONE 10:e0128912. doi: 10.1371/journal.pone.0128912
Letunic, I., and Bork, P. (2011). Interactive tree of Life v2: online annotation and display of phylogenetic trees made easy. Nucleic Acids Res. 39, 1–4. doi: 10.1093/nar/gkr201
Lobo, S. A. L., Melo, A. M., Carita, J. N., Teixeira, M., and Saraiva, L. M. (2007). The anaerobe Desulfovibrio desulfuricans ATCC 27774 grows at nearly atmospheric oxygen levels. FEBS Lett. 581, 433–436. doi: 10.1016/j.febslet.2006.12.053
Löscher, C. R., Bourbonnais, A., Dekaezemacker, J., Charoenpong, C. N., Altabet, M. A., Bange, H. W., et al. (2016). N2 fixation in eddies of the eastern tropical South Pacific Ocean. Biogeosciences 13, 2889–2899. doi: 10.5194/bg-13-2889-2016
Löscher, C. R., Großkopf, T., Desai, F. D., Gill, D., Schunck, H., Croot, P. L., et al. (2014). Facets of diazotrophy in the oxygen minimum zone waters off Peru. ISME J. 8, 1–13. doi: 10.1038/ismej.2014.71
Lovley, D. R., Phillips, E. J., Lonergan, D. J., and Widman, PK. (1995). Fe(III) and S0 reduction by Pelobacter carbinolicus. Appl. Environ. Microbiol. 61, 2132–2138.
Meysman, F. J., Middelburg, J. J., and Heip, C. H. (2006). Bioturbation: a fresh look at Darwin's last idea. Trends Ecol. Evol. 21, 688–695. doi: 10.1016/j.tree.2006.08.002
Mosch, T., Sommer, S., Dengler, M., Noffke, A., Bohlen, L., Pfannkuche, O., et al. (2012). Factors influencing the distribution of epibenthic megafauna across the Peruvian oxygen minimum zone. Deep Sea Res. I Oceanogr. Res. Papers 68, 123–135. doi: 10.1016/j.dsr.2012.04.014
Muyzer, G., and Stams, A. J. (2008). The ecology and biotechnology of sulphate-reducing bacteria. Nat. Rev. Microbiol. 6, 441–454. doi: 10.1038/nrmicro1892
Orcutt, K. M., Lipschultz, F., Gundersen, K., Arimoto, R., Michaels, A. F., Knap, A. H., et al. (2001). A seasonal study of the significance of N2 fixation by Trichodesmium spp. at the Bermuda Atlantic time-series Study (BATS) site. Deep Sea Res. II Top. Stud. Oceanogr. 48, 1583–1608. doi: 10.1016/S0967-0645(00)00157-0
Patriquin, D., and Knowles, R. (1972). Nitrogen fixation in the rhizosphere of marine angiosperms. Mar. Biol. 16, 49–58. doi: 10.1007/BF00347847
Postgate, J. R., and Campbell, L. L. (1966). Classification of Desulfovibrio species, the nonsporulating sulfate-reducing bacteria. Bacteriol. Rev. 30, 732–737.
Quintana, C. O., Tang, M., and Kristensen, E. (2007). Simultaneous study of particle reworking, irrigation transport and reaction rates in sediment bioturbated by the polychaetes Heteromastus and Marenzelleria. J. Exp. Mar. Biol. Ecol. 352, 392–406. doi: 10.1016/j.jembe.2007.08.015
Rainey, F. A., Donnison, A. M., Janssen, P. H., Saul, D., Rodrigo, A., Bergquist, P. L., et al. (1994). Description of Caldicellulosiruptor saccharolyticus gen. nov., sp. nov: an obligately anaerobic, extremely thermophilic, cellulolytic bacterium. FEMS Microbiol. Lett. 120, 263–266. doi: 10.1111/j.1574-6968.1994.tb07043.x
Revsbech, N. P., Jorgensen, B. B., and Blackburn, T. H. (1980). Oxygen in the sea bottom measured with a microelectrode. Science 207, 1355–1356. doi: 10.1126/science.207.4437.1355
Ribes, M., Dziallas, C., Coma, R., and Riemann, L. (2015). Microbial diversity and putative diazotrophy in high- and low-microbial-abundance mediterranean sponges. Appl. Environ. Microbiol. 81, 5683–5693. doi: 10.1128/AEM.01320-15
Riederer-Henderson, M.-A., and Wilson, P. W. (1970). Nitrogen fixation by sulphate-reducing bacteria. J. Gen. Microbiol. 61, 27–31. doi: 10.1099/00221287-61-1-27
Riemann, L., Farnelid, H., and Steward, G. F. (2010). Nitrogenase genes in non-cyanobacterial plankton: prevalence, diversity and regulation in marine waters. Aquat. Microbial Ecol. 61, 235–247. doi: 10.3354/ame01431
Røy, H., Weber, H. S., Tarpgaard, I. H., Ferdelman, T. G., and Jørgensen, B. B. (2014). Determination of dissimilatory sulfate reduction rates in marine sediment via radioactive 35 S tracer. Limnol. Oceanogr. Methods 12, 196–211. doi: 10.4319/lom.2014.12.196
Rusch, A., and Huettel, M. (2000). Advective particle transport into permeable sediments–evidence from experiments in an intertidal sandflat. Limnol. Oceanogr. 45, 523–533. doi: 10.4319/lo.2000.45.3.0525
Schafstall, J., Dengler, M., Brandt, P., and Bange, H. (2010). Tidal-induced mixing and diapycnal nutrient fluxes in the Mauritanian upwelling region. J. Geophys. Res. 115, 1–19. doi: 10.1029/2009JC005940
Schulz, H. N., and Jørgensen, B. B. (2001). Big bacteria. Annu. Rev. Microbiol. 55, 105–137. doi: 10.1146/annurev.micro.55.1.105
Shum, K. T., and Sundby, B. (1996). Organic matter processing in continental shelf sediments - the subtidal pump revisited. Mar. Chem. 53, 81–87. doi: 10.1016/0304-4203(96)00014-X
Sisler, F. D., and ZoBell, C. E. (1951). Nitrogen fixation by sulfate-reducing bacteria indicated by nitrogen/argon ratios. Science 113, 511–512. doi: 10.1126/science.113.2940.511
Sohm, J. A., Webb, E. A., and Capone, D. G. (2011). Emerging patterns of marine nitrogen fixation. Nat. Rev. Microbiol. 9, 499–508. doi: 10.1038/nrmicro2594
Sokoll, S. (2013). Transformation Processes of Nitrogen, Phosphorus and Iron in Sub-Euphotic Waters and Surface Sediments. Bremen: University of Bremen.
Sokoll, S., Lavik, G., Sommer, S., Goldhammer, T., Kuypers, M. M. M., and Holtappels, M. (2016). Extensive nitrogen loss from permeable sediments off North-West Africa. J. Geophys. Res. G Biogeosci. 121, 1144–1157. doi: 10.1002/2015JG003298
Sommer, S., Gier, J., Treude, T., Lomnitz, U., Dengler, M., Cardich, J., et al. (2016). Depletion of oxygen, nitrate and nitrite in the Peruvian oxygen minimum zone cause an imbalance of benthic nitrogen fluxes. Deep Sea Res. I 112, 113–122. doi: 10.1016/j.dsr.2016.03.001
Steenkamp, D. J., and Peck, H. D. (1981). Proton translocation associated with nitrite respiration in Desulfovibrio desulfuricans. J. Biol. Chem. 256, 5450–5458.
Stewart, W. D., Fitzgerald, G. P., and Burris, R. H. (1967). In situ studies on N2 fixation using the acetylene reduction technique. Proc. Natl. Acad. Sci. U.S.A. 58, 2071–2078. doi: 10.1073/pnas.58.5.2071
Stramma, L., Johnson, G. C., Sprintall, J., and Mohrholz, V. (2008). Expanding oxygen-minimum zones in the tropical oceans. Science 320, 655–658. doi: 10.1126/science.1153847
Tamura, K., Stecher, G., Peterson, D., Filipski, A., and Kumar, S. (2013). MEGA6: molecular evolutionary genetics analysis version 6.0. Mol. Biol. Evol. 30, 2725–2729. doi: 10.1093/molbev/mst197
van Niel, E. W. J., Pedro Gomes, T. M., Willems, A., Collins, M. D., Prins, R. A., and Gottschal, J. C. (1996). The role of polyglucose in oxygen-dependent respiration by a new strain of Desulfovibrio salexigens. FEMS Microbiol. Ecol. 21, 243–253. doi: 10.1111/j.1574-6941.1996.tb00121.x
Vandieken, V., Nickel, M., and Jørgensen, B. (2006). Carbon mineralization in arctic sediments northeast of Svalbard: Mn(IV) and Fe(III) reduction as principal anaerobic respiratory pathways. Mar. Ecol. Prog. Ser. 322, 15–27. doi: 10.3354/meps322015
Ward, B. B., and Bronk, D. A. (2001). Net nitrogen uptake and DON release in surface waters: importance of trophic interactions implied from size fractionation experiments. Mar. Ecol. Prog. Ser. 219, 11–24. doi: 10.3354/meps219011
Wenzhöfer, F., and Glud, R. N. (2004). Small-scale spatial and temporal variability in coastal benthic O 2 dynamics: effects of fauna activity. Limnol. Oceanogr. 49, 1471–1481. doi: 10.4319/lo.2004.49.5.1471
Zehr, J. P., and Turner, P. J. (2001). “Nitrogen fixation: nitrogenase genes and gene expression,” in Methods in Microbiology, Vol. 30, ed J. H. Paul (San Diego, CA: Academic Press), 271–286.
Zehr, J. P., and Ward, B. B. (2002). MINIREVIEW - nitrogen cycling in the ocean: new perspectives on processes and paradigms. Appl. Environ. Microbiol. 68, 1015–1024. doi: 10.1128/AEM.68.3.1015-1024.2002
Ziebis, W., Forster, S., Huettel, M., and Jørgensen, B. B. (1996). Complex burrows of the mud shrimp Callianassa truncata and their geochemical impact in the sea bed. Nature 382, 619–622. doi: 10.1038/382619a0
Zopfi, J., Kjaer, T., Nielsen, L. P., and Joergensen, B. B. (2001). Ecology of Thioploca spp.: nitrate and sulfur storage in relation to chemical microgradients and influence of Thioploca spp. on the sedimentary nitrogen cycle. Appl. Environ. Microbiol. 67, 5530–5537. doi: 10.1128/AEM.67.12.5530-5537.2001
Keywords: diazotrophs, nifH gene, sulfate reduction, bioirrigation, organic matter, sediment, upwelling
Citation: Gier J, Löscher CR, Dale AW, Sommer S, Lomnitz U and Treude T (2017) Benthic Dinitrogen Fixation Traversing the Oxygen Minimum Zone Off Mauritania (NW Africa). Front. Mar. Sci. 4:390. doi: 10.3389/fmars.2017.00390
Received: 06 January 2017; Accepted: 20 November 2017;
Published: 21 December 2017.
Edited by:
Carol Robinson, University of East Anglia, United KingdomReviewed by:
Jason Michel Smith, University of California, Santa Barbara, United StatesCopyright © 2017 Gier, Löscher, Dale, Sommer, Lomnitz and Treude. This is an open-access article distributed under the terms of the Creative Commons Attribution License (CC BY). The use, distribution or reproduction in other forums is permitted, provided the original author(s) or licensor are credited and that the original publication in this journal is cited, in accordance with accepted academic practice. No use, distribution or reproduction is permitted which does not comply with these terms.
*Correspondence: Jessica Gier, amdpZXJAZ2VvbWFyLmRl
Tina Treude, dHRyZXVkZUBnLnVjbGEuZWR1
Disclaimer: All claims expressed in this article are solely those of the authors and do not necessarily represent those of their affiliated organizations, or those of the publisher, the editors and the reviewers. Any product that may be evaluated in this article or claim that may be made by its manufacturer is not guaranteed or endorsed by the publisher.
Research integrity at Frontiers
Learn more about the work of our research integrity team to safeguard the quality of each article we publish.