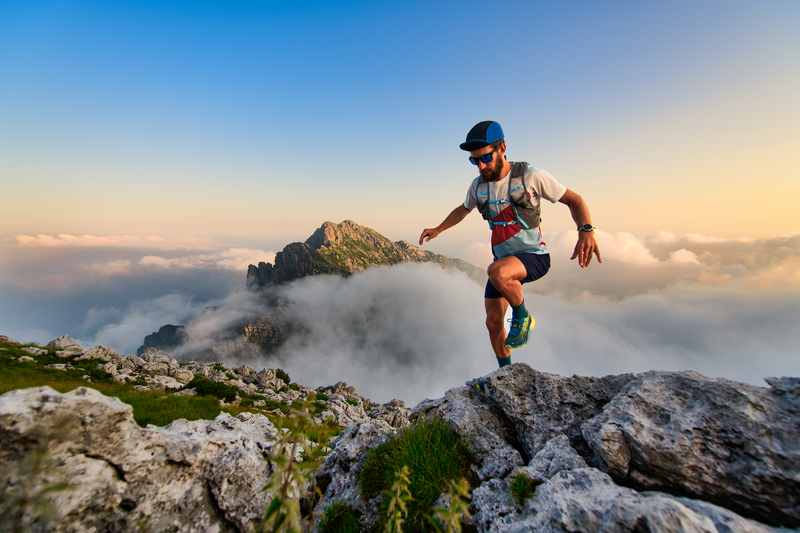
95% of researchers rate our articles as excellent or good
Learn more about the work of our research integrity team to safeguard the quality of each article we publish.
Find out more
PERSPECTIVE article
Front. Mar. Sci. , 22 November 2017
Sec. Marine Ecosystem Ecology
Volume 4 - 2017 | https://doi.org/10.3389/fmars.2017.00374
Effectively managing human pressures on tropical seascapes (mangrove forests, seagrass beds, and coral reefs) requires innovative approaches that go beyond the ecosystem as the focal unit. Recent advances in scientific understanding of long-distance connectivity via extended ecosystem engineering effects and on-going rapid developments in monitoring and data-sharing technologies provide viable tools for novel management approaches that use positive across-ecosystem interactions (for example, hydrodynamics). Scientists and managers can now use this collective knowledge to develop monitoring and restoration protocols that are specialized for cross ecosystem fluxes (waves, sediments, nutrients) on a site-specific basis for connected tropical seascape (mangrove forests, seagrass beds, and coral reefs).
Coastal zones support many people within relatively small land areas (Neumann et al., 2015) but are highly threatened by human activities (Szabo et al., 2015; Heery et al., 2017). This is particularly true of tropical coastal regions, where one-third of humanity is supported on 4% of the total world land area (Barbier et al., 2008). Increased human exploitation of these coastal zones has caused declines in the health and extent of mangrove forests (35% Valiela et al., 2001), seagrass beds (30%; Waycott et al., 2009, and coral reefs (20%; Bellwood et al., 2004) over the last 20–40 years (Figure 1A). The decreased area and functioning of these ecosystems implies concomitant loss of the ecosystem services they provide, particularly coastal defense in the face of projected sea level rise (Temmerman et al., 2013).
Figure 1. Global area loss, restoration, and management efforts for the three dominant kinds of tropical coastal ecosystems: coral reefs, seagrass beds, and mangrove forests. (A) Percentage loss in area over the last 20–40 years. Data sources: Mangrove forests (Valiela et al., 2001); seagrass beds (Waycott et al., 2009); coral reefs (Bellwood et al., 2004). (B) Percent restoration failure calculated from the number of restoration projects (106 mangrove forests; 141 seagrass beds; 293 coral reefs) and the number judged unsuccessful (Bayraktarov et al., 2016). (C) Global distribution of regions with these ecosystem types considered most threatened for mangrove forests (Polidoro et al., 2010; green lines), seagrass beds (Short et al., 2011; yellow lines), and coral reefs (Burke et al., 2011) (purple lines). Management strategies: Triangles, Marine protected areas; Circles, integrated coastal zone management; Squares, restoration efforts; Star and clear Polygon, Land management and water monitoring; respectively (Gladstone, 2009; Bayraktarov et al., 2016).
Observed declines in ecosystem area and functioning have led to increased, costly (Bayraktarov et al., 2016), restoration efforts in all three ecosystems, with limited success (Figure 1B). High failure rates indicate new strategies are needed to improve restoration success and to prevent further degradation of the tropical seascape. Many attempts target one ecosystem without considering interactions among many. Interactions among adjacent ecosystems and their associated ecosystem engineers may influence local management success, but are rarely included despite general recognition of the potential importance of seascape-scale connectivity to ecosystem-based management (McLeod and Leslie, 2009; Saunders et al., 2014; Long et al., 2015). A review of 49 management plans across eight coastal systems (including the Great Barrier Reef and the Everglades) only 6% of the management objectives included consideration of connectivity (Arkema et al., 2006). As far as we can ascertain, such cross-ecosystem connections have not been used in restoration efforts.
The reasons why ecosystem connectivity may not have been central in restoration is the practical challenge of determining the relevant energetic and material inter-connections and their influences (Melia et al., 2016): i.e., what are the fluxes among proximal ecosystems, what determines variation in their magnitude and their effects, and how do you measure them? Research in tropical coastal seascapes has revealed ecosystem engineering species within some ecosystems (i.e., species that physically modify the abiotic environment Jones et al., 1994) can significantly reduce wave energy from the ocean to the land and reduce nutrient and sediment fluxes from the land to the ocean (Gillis et al., 2014a; Saunders et al., 2014; Koppel et al., 2015; Guannel et al., 2016). Effects can occur over distances sufficient to encompass and positively affect other ecosystem types lying within the energetic and material flux paths from ocean to land and vice versa (Gillis et al., 2014a; Saunders et al., 2014; Koppel et al., 2015; Guannel et al., 2016). In this perspective, we will argue that new technologies and knowledge can link together to provide the foundations for innovative inter-ecosystem connectivity management (monitoring and restoration). We indicate globally where this type of management can be used to improve the success and reduce the cost of protecting the connected tropical seascape.
Ecosystem engineers physically modify environmental conditions, thereby allowing for greater resource availability (Jones et al., 1994). We are aware local effects can extend beyond the ecosystem and its ecosystem engineers to affect other types of ecosystems this can be positive (sediment buffering in turbid waters) or negative (nutrient retention in oligotrophic areas; Sheaves, 2009). Here, we concentrate only on how local physical modification of the abiotic environment can have extended spatial influence on flux exchanges among different ecosystems within landscapes and seascapes (Gillis et al., 2014a; Koppel et al., 2015). In tropical coastal seascapes, the physical structure of “donor” ecosystems can affect the establishment and persistence of “recipient” ecosystems positively, including the engineering species they contain, the structures they create, and the effects of these structures (Gillis et al., 2014a) (Figure 2, panel 1).
Figure 2. The left hand side panel 1, representatives tropical coastal seascape showing a mangrove forest, a seagrass bed, and a coral reef; energetic and material exchanges among them; and illustrative monitoring technologies (A–E). All three ecosystem types can be both donor ecosystems (DS) and recipient ecosystems (RS) with respect to energetic and material exchange (see text). Ecosystem engineering within each ecosystem type modifies exchanges among ecosystems of hydrodynamic energy (blue arrows and numbered text boxes), nutrients (green arrows and numbered text boxes—N: Nitrogen. P: Phosphorus), and sediments (brown arrows and numbered text boxes). Arrows indicate the direction of exchanges from donor to recipient ecosystems. Numbered boxes show the connection between the donor and most proximate recipient type of ecosystem; the kind of donor ecosystem effect on a recipient ecosystem; and the known range of this effect magnitude. A variety of monitoring techniques, often complementary, can be used to: Determine if ecosystem proximity is sufficient for connectivity; measure energetic and material exchanges among donor and recipient ecosystems; ascertain the physical structures of donor ecosystems responsible for the extended ecosystem engineering effects and measure the responses of recipient ecosystems (see text for details). Illustrated here are: A. Satellite detecting changes in cover; B. Sediment trap; C. Secchi disk measuring water transparency; D. Water quality sampling; E. Acoustic Doppler Current Profiler (ADCP) measuring water flux. The right hand side panel 2, showing a flow chart of the steps and tools that can be utilizing to integrate connectivity into tropical management. The beginning of the flow chart (step 1) shows connected unites of mangrove forest, seagrass bed, and coral reefs from left to right. Where ecosystem-engineering reduction of fluxes is indicated. The physical structure of the ecosystem system engineers and flux dynamics can be monitored either traditional monitoring or new technologies (step 2). The data from monitoring can be integrated for management utilizing established tools (step 3), which in turn can be used for both intervention in the tropical coastal seascape (i.e., restoration, step 4), or continued monitoring (step 2).
Coral reefs, for example, are adversely affected by high sediment and nutrient loads (Erftemeijer et al., 2012; Rasher et al., 2012); seagrass beds are negatively disturbed by high sediment/nutrient loads and high wave energy (Koch, 2001; Burkholder et al., 2007). Mangrove forests are adversely affected by high wave energy (Balke et al., 2014). Coral reef structure can decrease wave heights reaching seagrass beds (Figure 2, panel 1, box 1), whose structure can further reduce wave heights encroaching on mangrove forests (Figure 2, box 2). Seagrass beds take up nutrients and trap suspended sediments that reach coral reefs (Figure 2, panel 1, boxes 3, 4). Mangrove forests and their physical presence can trap nutrients and sediments that influence seagrass beds (Figure 2, panel 1, boxes 5, 6). Donor ecosystems positively influence recipient ecosystems whenever an ecosystem engineer has a large effect on the magnitude of the fluxes. The physical structures made by species in all three kinds of ecosystems create habitats and harbor species moving amongst them (Nagelkerken, 2009), which will have a positive effect on adjacent ecosystems (we will not further address this as this aspect is beyond the scope of the perspective).
Although some current management approaches reach across landscapes to include several ecosystems (Figure 1C; Gladstone, 2009), these management strategies do not target connections between ecosystems and the engineers controlling these connections (Pressey and Bottrill, 2009). This omission may, be due to connections between ecosystems being diverse and seemingly complex. However, in the tropical coastal seascapes we know the most important fluxes (waves, sediment, and nutrients) and the ecosystem engineers with their traits controlling these fluxes. This information can be used directly in management via monitoring the magnitude not only on a local scale, but at a larger scale encompassing long-distance effects.
If all three types of ecosystems are both donors and recipients, when they are sufficiently proximate, concentrating on one ecosystem as a single unit risks management failure especially when interconnections influence ecosystem functioning (Lovett et al., 2005; Koppel et al., 2015; Guannel et al., 2016). In such cases, a seascape approach to management is needed. This approach is not only true for natural seascapes, but for modified seascapes with artificial structures, which affect connectivity (Barbier et al., 2008; Bishop et al., 2017; Heery et al., 2017).
The existence of functionally significant interconnections among the three different ecosystems and their associated ecosystem engineers requires developing a holistic marine management regime (Figure 2, panel 2). Firstly, identifying ecosystems and fluxes within the seascape. Secondly acquiring empirical data on the state of donor ecosystems/ecosystem engineers, the flux input/ouput and their effects on fluxes to recipient ecosystems, and prioritizing the important fluxes between the ecosystems via monitoring (Figure 2, panel 2, step 1). If a decline is detected in a recipient ecosystem, then from a connectivity perspective, the positive effects of donor ecosystems/ecosystem engineers on a recipient ecosystem have declined or been lost due to donor degradation.
Thirdly, utilizing established tools for integrating the monitoring of connectivity into ecosystem management (Figure 2, panel 2, step 3). Managers could, for example ensure an integration of a variety of stakeholders via creating new institutes with the responsibility to coordinate actions (Fowler, 2009). If this is not possible then common arenas for facilitation (Fowler, 2009) via a specialized “knowledge broker” to serve as a bridge between producers and users of knowledge and to facilitate interactions between groups (Naylor et al., 2012). In-situ data collection methods can be expensive, as the ecosystems should be monitored continuously over time. An approach showing increasing interest from scientists and managers is using citizen scientists, which has many benefits not only financially but also socially (Dickinson et al., 2012; Vermeiren et al., 2016; Figure 2, panel 2, step 3).
Stakeholders could develop an integrated ecosystem assessment (IEA) for all ecosystems at the landscape scale, i.e., including fluxes (Rodriguez, 2017). This assessment should establish evaluation criteria for example Is the reciprocal ecosystem degraded? (Figure 2, panel 2). Where the variables and goals are clearly defined and the IEA is adaptive so new information and knowledge are fed back into the IEA to facilitate evaluation and assessment (Rodriguez, 2017). Allowing managers to develop strategies (i.e., restoration) to address challenges (Figure 2, panel 2, step 4), implement policies and set objectives for management of connective fluxes.
More physical processes, fluxes, and ecosystem engineering traits are being monitored than before, but not in a way elucidating interactions between ecosystems. Monitoring is used to understand individual units, for example, sequestration of carbon (McLeod et al., 2011). An important step would be to monitor fluxes between ecosystems with new developing techniques. High-resolution airborne Light Detection And Ranging (LIDAR) bathymetry has already been used to estimate wave energy dissipation over a coral reef (Figure 2; Huang et al., 2012). A new innovative tool could be developing LIDAR to estimate wave energy over seagrass beds and entering mangrove forests, we could therefore monitor how wave energy changes from one ecosystem to another in combination with ecosystem engineering distribution. Such remote estimates can be complemented using, sediment traps and Secchi disks for turbidity, water quality samples for nutrients, and Acoustic Doppler Current Profiling for water fluxes (Figure 2; Talbot and Wilkinson, 2001). Methods are already in use for local singular ecosystem-based monitoring but this could be expanded to determine how changes occur between one ecosystem and another. Remote and local data can then be integrated using satellite maps and spatial analysis to help create spatial explicit maps of fluxes across the seascape, which will cover mechanisms at the level of ecosystem extrapolated to the large-scale seascape (Brodie et al., 2010; Petus et al., 2016).
Many of the remote and local monitoring techniques can be used to assess structural characteristics of donor ecosystems relevant to their ecosystem engineering effects on fluxes but currently they have not. For example, LIDAR bathymetry and acoustic ground determination of coral reefs can estimate reef rugosity—key to determining effects on wave energy (Walker et al., 2008). LIDAR could quantify structural elements of seagrass beds relevant to wave energy attenuation and sediment trapping. A cutting edge development would be to combine LIDAR data of (i) fluxes across ecosystems and (ii) information on ecosystem engineers, to map connectivity across the seascape to determine the potential controls of connectivity. Airborne and satellite optical sensing could be utilized to establish the extent of these ecosystems and therefore the potential connective pathways (Dierssen et al., 2003; Paul et al., 2011; Hedley et al., 2016). Airborne and satellite optical sensing could be utilized to establish the extent of these ecosystems and therefore the potential connective pathways (Dierssen et al., 2003; Paul et al., 2011; Hedley et al., 2016). Local measures of structure could be used to assess the extent of the ecosystem (Talbot and Wilkinson, 2001): for example, Line/Point Intercept Transect assessment methods for corals; quadrats for seagrasses; and transects for mangroves (Talbot and Wilkinson, 2001). The resulting information could be used to validate remote data and provide information that cannot be obtained remotely (e.g., the species contributing to the structural heterogeneity responsible for engineering effects). When new data and knowledge are available from monitoring (i.e., the ecosystem attributes and the fluxes), they can be fed back into for example a IEA and used to assess the efficiency and effectiveness of the connectivity based management programs (Figure 2, panel 2; Lovett et al., 2007).
Knowing if at least two or all three of these ecosystem types in an area are sufficiently proximate (or were so, if lost) requires mapping their distribution. This information can then be combined with models that estimate effects on wave energy, nutrients, and sediments as a function of distance and ecosystem engineering attributes. This can serve as an effective tool for accessing how to bring connectivity into management. While feasible using satellite imagery and the development of models from current data, it has yet to be done. NASA and the European Copernicus program have developed a strategy for the global acquisition of freely available satellite imagery for the next decades (Skidmore et al., 2015). Combined with advancements in sensor technology such as NASA's Global Ecosystem Dynamics Investigation (GEDI) LIDAR and the German Aerospace Center's high resolution and wide spectrum satellite EnMAP (Skidmore et al., 2015), this platform will allow a wide range of ecosystem attributes and interactions to be monitored to an adequate spatio-temporal resolutions. These developments facilitate cost-efficient use of remote sensing technology, and compensate for required resources toward specialized image processing, analysis, validation, and interpretation.
If an ecosystem is in a pristine state or has a natural recovery potential, monitoring of fluxes, and ecosystem engineering traits should continue as long as no other stressors occur (Figure 2, panel 2, step 2). When donor ecosystems have deteriorated to the point and they have no positive influence on recipient ecosystems, or when a connected ecosystem type has been destroyed, restoration of donor ecosystems is required in order to manage and restore recipient ecosystems (Figure 2, panel 2, step 4). We believe one should target those ecosystem-engineering donor species that alter fluxes for the persistence or establishment of recipient ecosystems (Figure 2). This is an essential tool for managing and restoring connectivity fluxes between connected ecosystems.
Current understanding can help guide restoration. Restoring connectivity in tropical seascapes means restoring donor ecosystems and their ecosystem engineers to the point where there is sufficient relevant physical structure for the positive effects on the recipient ecosystems (van der Heide et al., 2007; Bouma et al., 2009). In some cases artificial structures may be preventing connected fluxes between adjacent ecosystems, however the scale and impact of artificial structures is still relatively unknown (Bishop et al., 2017; Heery et al., 2017). Further qualification and quantification is urgently needed in this respect to understand how coastal modifications will affect restoration of fluxes.
The need to rapidly restore physical structure in donor ecosystems to prevent further deterioration in recipient ecosystems suggests choices among native species. The re-establishment of fast-growing stony corals can rapidly increase reef surface roughness, therefore decreasing wave energy that uproots seagrass beds (Yap, 2000) and erode mangrove forests and/or prevent seedlings from establishing (Balke et al., 2013). Other, slower-growing coral species, or species that have little high rugosity (e.g., brain corals), may be less appropriate initially. Replanting fast-growing seagrass species is more likely to lead to rapid nutrient uptake and sediment trapping that debilitates coral reefs (Yap, 2000). Fast-growing mangrove species with extensive prop root systems (i.e., complex architecture at the water/sediment interface) are most likely to rapidly re-establish the capacity to trap sediments whose export can inhibit seagrass beds and coral reefs (Yap, 2000).
Successful establishment of ecosystem engineers will not lead to restoration of connectivity until there is sufficient physical structure-thus time for growth is needed. When loss of ecosystem connectivity adversely affects establishment and growth of the engineering species (e.g., wave erosion of mangroves and seagrasses, Koch, 2001; Balke et al., 2014; sediment burial of seagrasses, Cabaço et al., 2008; nutrient and sediment inhibition of corals, Erftemeijer et al., 2012; Rasher et al., 2012), it may be necessary to use temporary constructions whose structure mimics engineering effects to make local conditions suitable. For example, ecological restoration using the natural seeding potential of mangrove forests, rather than planting seedlings, has been successful when the hydraulic thresholds (Olds et al., 2012) have been restored (Lewis, 2000, 2005).
In areas such as the Caribbean and South-East Asia (Figure 1C), practices already consider management at the ecosystem scale (Figure 1C), but they do not explicitly consider connectivity. Other areas have shown ridge-to-reef management (The Great Barrier Reef), but without concentrating on fluxes between ecosystems (Figure 1C). Nevertheless, in these regions, restoration has not generally been successful for example using inappropriate species (Yap, 2000). Combining our understanding of connectivity and ecological restoration may be the most efficient and effective way to successfully restore and manage ecosystems.
We question why this approach has not yet been used more broadly? Many of the scientific challenges of connective seascape management and restoration have become solvable with recent progress in science and technology such as our understanding of long distance fluxes, satellite imagery, and data sharing. There are, of course, many challenges to implementation e.g., cost, insufficient interaction between scientists and managers, mandates of management agencies, national policies (Mumby et al., 1999; Forst, 2009; Brodie and Waterhouse, 2012). Utilizing local stakeholder knowledge of ecosystem connectivity, and citizen science that helps monitor changes in connectivity, can, with freely available satellite images (and tools for analyzing them), be central to better seascape-level management, especially when financial resources are limited.
Some of the most threatened mangrove forests, seagrass beds, and corals reefs show the greatest prevalence of these ecosystems adjacent to each other (and therefore the highest chance of connected ecosystems; Figure 1C). Conventional management in these regions already exists but it does not appear to be the most effective in stopping further degradation of ecosystems (Figure 1C). In these regions, because of the potential high prevalence of connected ecosystems, integrating connectivity into management at the tropical seascape level is viable. Seascape-scale management, such as ecological restoration, could reverse degradation in addition to restoring coastal ecosystems (as well as their services and functions). Given the current estimates of costs of restoration (mangrove forests 8,961, seagrass beds 106,782 and coral reefs 165, 607 US$ ha−1; Bayraktarov et al., 2016) and the failure rates (Figures 1A,B), developing management with ecological restoration should be a priority.
Using specific ecosystem engineers known to develop long-distance flux exchanges should be the focal point of restoration and management efforts. The necessary knowledge regarding the fluxes needed to be monitored and the ecosystem engineering traits altering specific fluxes is now readily available. Using ecosystem engineers, which by their own physical structure affect landscape scale connectivity, to restore and manage tropical coastal zones is a challenging and promising opportunity. This approach demands integrating connectivity principles into management strategies. Moreover, it is timely to do so, as we are now facing a growing need for implementing nature-based coastal protection.
LG is the principle author and analyses. CJ helped develop the idea and gave feedback on writing, AZ provided strong support on writing and provided feedback to the original idea, DvdW supported and provided information for the monitoring section, AB supported social science and restoration sections, and TB helped with the perceived idea and developed writing.
The authors declare that the research was conducted in the absence of any commercial or financial relationships that could be construed as a potential conflict of interest.
Arkema, K. K., Abramson, S. C., and Dewsbury, B. M. (2006). Marine ecosystem-based management: from characterization to implementation. Front. Ecol. Environ. 4, 525–532. doi: 10.1890/1540-9295(2006)4[525:MEMFCT]2.0.CO;2
Balke, T., Herman, P. M. J., and Bouma, T. J. (2014). Critical transitions in disturbance-driven ecosystems: identifying windows of opportunity for recovery. J. Ecol. 102, 700–708. doi: 10.1111/1365-2745.12241
Balke, T., Webb, E. L., van den Elzen, E., Galli, D., Herman, P. M. J., and Bouma, T. J. (2013). Seedling establishment in a dynamic sedimentary environment: a conceptual framework using mangroves. J. Appl. Ecol. 50, 740–747. doi: 10.1111/1365-2664.12067
Barbier, E. B., Koch, E. W., Silliman, B. R., Hacker, S. D., Wolanski, E., Primavera, J., et al. (2008). Coastal ecosystem-based management with nonlinear ecological functions and values. Science 319, 321–323. doi: 10.1126/science.1150349
Bayraktarov, E., Saunders, M. I., Abdullah, S., Mills, M., Beher, J., Possingham, H. P., et al. (2016). The cost and feasibility of marine coastal restoration. Ecol. Appl. 26, 1055–1074. doi: 10.1890/15-1077
Bellwood, D. R., Hughes, T. P., Folke, C., and Nystrom, M. (2004). Confronting the coral reef crisis. Nature 429, 827–833. doi: 10.1038/nature02691
Bishop, M. J., Mayer-Pinto, M., Airoldi, L., Firth, L. B., Morris, R. L., Loke, L. H. L., et al. (2017). Effects of ocean sprawl on ecological connectivity: impacts and solutions. J. Exp. Mar. Biol. Ecol. 492, 7–30. doi: 10.1016/j.jembe.2017.01.021
Bouma, T. J., Friedrichs, M., Van Wesenbeeck, B. K., Temmerman, S., Graf, G., and Herman, P. M. J. (2009). Density-dependent linkage of scale-dependent feedbacks: a flume study on the intertidal macrophyte Spartina anglica. Oikos 118, 260–268. doi: 10.1111/j.1600-0706.2008.16892.x
Brodie, J., Schroeder, T., Rohde, K., Faithful, J., Masters, B., Dekker, A., et al. (2010). Dispersal of suspended sediments and nutrients in the Great Barrier Reef lagoon during river-discharge events: conclusions from satellite remote sensing and concurrent flood-plume sampling. Mar. Freshw. Res. 61, 651–664. doi: 10.1071/MF08030
Brodie, J., and Waterhouse, J. (2012). A critical review of environmental management of the ‘not so Great’ Barrier Reef. Estuarine Coast. Shelf Sci. 104–105, 1–22. doi: 10.1016/j.ecss.2012.03.012
Burke, L., Reytar, K., Spalding, M., and Perry, A. (2011). Reefs at Risk Revisited. Washington, DC: World Resources Institute.
Burkholder, J. M., Tomasko, D. A., and Touchette, B. W. (2007). Seagrasses and eutrophication. J. Exp. Mar. Biol. Ecol. 350, 46–72. doi: 10.1016/j.jembe.2007.06.024
Cabaço, S., Santos, R., and Duarte, C. M. (2008). The impact of sediment burial and erosion on seagrasses: a review. Estuar. Coast. Shelf Sci. 79, 354–366. doi: 10.1016/j.ecss.2008.04.021
Dickinson, J. L., Shirk, J., Bonter, D., Bonney, R., Crain, R. L., Martin, J., et al. (2012). The current state of citizen science as a tool for ecological research and public engagement. Front. Ecol. Environ. 10, 291–297. doi: 10.1890/110236
Dierssen, H. M., Zimmerman, R. C., Leathers, R. A., Downes, T. V., and Davis, C. O. (2003). Ocean color remote sensing of seagrass and bathymetry in the Bahamas Banks by high-resolution airborne imagery. Limnol. Oceanogr. 48, 444–455. doi: 10.4319/lo.2003.48.1_part_2.0444
Erftemeijer, P. L., Riegl, B., Hoeksema, B. W., and Todd, P. A. (2012). Environmental impacts of dredging and other sediment disturbances on corals: a review. Mar. Pollut. Bull. 64, 1737–1765. doi: 10.1016/j.marpolbul.2012.05.008
Forst, M. F. (2009). The convergence of integrated coastal zone management and the ecosystems approach. Ocean Coast. Manage. 52, 294–306. doi: 10.1016/j.ocecoaman.2009.03.007
Fowler, C. W. (2009). Systemic Management: Sustainable Human Interactions with Ecosystems and the Biosphere. Oxford: Oxford University Press.
Gillis, L. G., Bouma, T. J., Jones, C. G., van Katwijk, M. M., Nagelkerken, I., Jeuken, C. J. L., et al. (2014a). Potential for landscape-scale positive interactions among tropical marine ecosystems. Mar. Ecol. Prog. Ser. 503, 289–303. doi: 10.3354/meps10716
Gladstone, W. (2009). Conservation and Management of Tropical Coastal Ecosystems. Nijmegen: Springer Netherlands.
Guannel, G., Arkema, K., Ruggiero, P., and Verutes, G. (2016). The power of three: coral reefs, seagrasses and mangroves protect coastal regions and increase their resilience. PLoS ONE 11:e0158094. doi: 10.1371/journal.pone.0158094
Hedley, J. D., Roelfsema, C. M., Chollett, I., Harborne, A. R., Heron, S. F., Weeks, S. J., et al. (2016). Remote sensing of coral reefs for monitoring and management: a review. Remote Sens. 8:118. doi: 10.3390/rs8020118
Heery, E. C., Bishop, M. J., Critchley, L. P., Bugnot, A. B., Airoldi, L., Mayer-Pinto, M., et al. (2017). Identifying the consequences of ocean sprawl for sedimentary habitats. J. Exp. Mar. Biol. Ecol. 492, 31–48. doi: 10.1016/j.jembe.2017.01.020
Huang, Z. C., Reineman, B. D., Lenain, L., Melville, W. K., and Middleton, J. H. (2012). Airborne lidar measurements of wave energy dissipation in a coral reef lagoon system. J. Geophys. Res. Oceans 117:13. doi: 10.1029/2011JC007203
Jones, C. G., Lawton, J. H., and Shachak, M. (1994). Organisms as ecosystem engineers. Oikos 69, 373–386. doi: 10.2307/3545850
Koch, E. W. (2001). Beyond light: physical, geological, and geochemical parameters as possible submersed aquatic vegetation habitat requirements. Estuaries 24, 1–17. doi: 10.2307/1352808
Koppel, J., van der Heide, T., Altieri, A. H., Eriksson, B. K., Bouma, T. J., Olff, H., et al. (2015). Long-distance interactions regulate the structure and resilience of coastal ecosystems. Ann. Rev. Mar. Sci. 7, 139–158. doi: 10.1146/annurev-marine-010814-015805
Lewis, R. R. (2000). Ecologically based goal setting in mangrove forest and tidal marsh restoration. Ecol. Eng. 15, 191–198. doi: 10.1016/S0925-8574(00)00070-7
Lewis, R. R. (2005). Ecological engineering for successful management and restoration of mangrove forests. Ecol. Eng. 24, 403–418. doi: 10.1016/j.ecoleng.2004.10.003
Long, R. D., Charles, A., and Stephenson, R. L. (2015). Key principles of marine ecosystem-based management. Mar. Policy 57, 53–60. doi: 10.1016/j.marpol.2015.01.013
Lovett, G. M., Jones, C. G., Turner, M. G., and Weathers, K. C. (2005). Ecosystem Function in Heterogeneous Landscapes. Nijmegen: Springer Netherlands.
Lovett, G. M., Burns, D. A., Driscoll, C. T., Jenkins, J. C., Mitchell, M. J., Rustad, L., et al. (2007). Who needs environmental monitoring? Front. Ecol. Envir. 5, 253–260. doi: 10.1890/1540-9295(2007)5[253:WNEM]2.0.CO;2
McLeod, E., Chmura, G. L., Bouillon, S., Salm, R., Björk, M., Duarte, C. M., et al. (2011). A blueprint for blue carbon: toward an improved understanding of the role of vegetated coastal habitats in sequestering CO2. Front. Ecol. Environ. 9, 552–560. doi: 10.1890/110004
McLeod, K. L., and Leslie, H. M. (2009). “Why ecosystem-based management?,” in Ecosystem-Based Management for the Oceans, eds K. L. McLeod and H. M. Leslie (Washington, DC: Island Press,) 3–12.
Melia, P., Schiavina, M., Rossetto, M., Gatto, M., Fraschetti, S., and Casagrandi, R. (2016). Looking for hotspots of marine metacommunity connectivity: a methodological framework. Sci. Rep. 6:23705. doi: 10.1038/srep23705
Mumby, P. J., Green, E. P., Edwards, A. J., and Clark, C. D. (1999). The cost-effectiveness of remote sensing for tropical coastal resources assessment and management. J. Environ. Manage. 55, 157–166. doi: 10.1006/jema.1998.0255
Nagelkerken, I. (2009). Ecological Connectivity among Tropical Coastal Ecosystems, 1st Edn. Nijmegen: Springer Netherlands.
Naylor, L. A., Coombes, M. A., Venn, O., Roast, S. D., and Thompson, R. C. (2012). Facilitating ecological enhancement of coastal infrastructure: the role of policy, people and planning. Environ. Sci. Policy 22, 36–46. doi: 10.1016/j.envsci.2012.05.002
Neumann, B., Vafeidis, A. T., Zimmermann, J., and Nicholls, R. J. (2015). Future coastal population growth and exposure to sea-level rise and coastal flooding - a global assessment. PLoS ONE 10:e0118571. doi: 10.1371/journal.pone.0118571
Olds, A. D., Connolly, R. M., Pitt, K. A., and Maxwell, P. S. (2012). Habitat connectivity improves reserve performance. Conserv. Lett. 5, 56–63. doi: 10.1111/j.1755-263X.2011.00204.x
Paul, M., Lefebvre, A., Manca, E., and Amos, C. L. (2011). An acoustic method for the remote measurement of seagrass metrics. Estuarine Coast. Shelf Sci. 93, 68–79. doi: 10.1016/j.ecss.2011.04.006
Petus, C., Devlin, M., Thompson, A., McKenzie, L., da Silva, E. T., Collier, C., et al. (2016). Estimating the exposure of coral reefs and seagrass meadows to land-sourced contaminants in river flood plumes of the great barrier reef: validating a simple satellite risk framework with environmental data. Remote Sens. 8:210. doi: 10.3390/rs8030210
Polidoro, B. A., Carpenter, K. E., Collins, L., Duke, N. C., Ellison, A. M., Ellison, J. C., et al. (2010). The loss of species: mangrove extinction risk and geographic areas of global concern. PLoS ONE 5:e10095. doi: 10.1371/journal.pone.0010095
Pressey, R. L., and Bottrill, M. C. (2009). Approaches to landscape- and seascape-scale conservation planning: convergence, contrasts and challenges. Oryx 43, 464–475. doi: 10.1017/S0030605309990500
Rasher, D. B., Engel, S., Bonito, V., Fraser, G. J., Montoya, J. P., and Hay, M. E. (2012). Effects of herbivory, nutrients, and reef protection on algal proliferation and coral growth on a tropical reef. Oecologia 169, 187–198. doi: 10.1007/s00442-011-2174-y
Rodriguez, N. J. I. (2017). A comparative analysis of holistic marine management regimes and ecosystem approach in marine spatial planning in developed countries. Ocean Coast. Manage. 137, 185–197. doi: 10.1016/j.ocecoaman.2016.12.023
Saunders, M. I., Leon, J. X., Callaghan, D. P., Roelfsema, C. M., Hamylton, S., Brown, C. J., et al. (2014). Interdependency of tropical marine ecosystems in response to climate change. Nat. Clim. Change 4, 724–729. doi: 10.1038/nclimate2274
Sheaves, M. (2009). Consequences of ecological connectivity: the coastal ecosystem mosaic. Mar. Ecol. Prog. Ser. 391, 107–115. doi: 10.3354/meps08121
Short, F. T., Polidoro, B., Livingstone, S. R., Carpenter, K. E., Bandeira, S., Bujang, J. S., et al. (2011). Extinction risk assessment of the world's seagrass species. Biol. Conserv. 144, 1961–1971. doi: 10.1016/j.biocon.2011.04.010
Skidmore, A. K., Pettorelli, N., Coops, N. C., Geller, G. N., Hansen, M., Lucas, R., et al. (2015). Environmental science: agree on biodiversity metrics to track from space. Nature 523, 403–405. doi: 10.1038/523403a
Szabo, S., Renaud, F. G., Hossain, M. S., Sebesvari, Z., Matthews, Z., Foufoula-Georgiou, E., et al. (2015). Sustainable development goals offer new opportunities for tropical delta regions. Environment 57, 16–23. doi: 10.1080/00139157.2015.1048142
Talbot, F., and Wilkinson, C. (2001). Coral reefs, Mangroves and Seagrasses: A Sourcebook for Managers. Townsville: Australian Insitute of Marine Science.
Temmerman, S., Meire, P., Bouma, T. J., Herman, P. M. J., Ysebaert, T., and De Vriend, H. J. (2013). Ecosystem-based coastal defence in the face of global change. Nature 504, 79–83. doi: 10.1038/nature12859
Valiela, I., Bowen, J. L., and York, J. K. (2001). Mangrove forests: one of the world's threatened major tropical environments. Bioscience 51, 807–815. doi: 10.1641/0006-3568(2001)051[0807:MFOOTW]2.0.CO;2
van der Heide, T., van Nes, E. H., Geerling, G. W., Smolders, A. J. P., Bouma, T. J., and van Katwijk, M. M. (2007). Positive feedbacks in seagrass ecosystems: implications for success in conservation and restoration. Ecosystems 10, 1311–1322. doi: 10.1007/s10021-007-9099-7
Vermeiren, P., Munoz, C., Zimmer, M., and Sheaves, M. (2016). Hierarchical toolbox: ensuring scientific accuracy of citizen science for tropical coastal ecosystems. Ecol. Indic. 66, 242–250. doi: 10.1016/j.ecolind.2016.01.031
Walker, B. K., Riegl, B., and Dodge, R. E. (2008). Mapping coral reef habitats in southeast Florida using a combined technique approach. J. Coast. Res. 24, 1138–1150. doi: 10.2112/06-0809.1
Waycott, M., Duarte, C. M., Carruthers, T. J., Orth, R. J., Dennison, W. C., Olyarnik, S., et al. (2009). Accelerating loss of seagrasses across the globe threatens coastal ecosystems. Proc. Natl. Acad. Sci. U.S.A. 106, 12377–12381. doi: 10.1073/pnas.0905620106
Keywords: facilitation, ecosystem engineers, mangrove forests, seagrass beds, coral reefs, monitoring
Citation: Gillis LG, Jones CG, Ziegler AD, van der Wal D, Breckwoldt A and Bouma TJ (2017) Opportunities for Protecting and Restoring Tropical Coastal Ecosystems by Utilizing a Physical Connectivity Approach. Front. Mar. Sci. 4:374. doi: 10.3389/fmars.2017.00374
Received: 28 February 2017; Accepted: 07 November 2017;
Published: 22 November 2017.
Edited by:
Angel Borja, AZTI Pasaia, SpainReviewed by:
Katherine Dafforn, University of New South Wales, AustraliaCopyright © 2017 Gillis, Jones, Ziegler, van der Wal, Breckwoldt and Bouma. This is an open-access article distributed under the terms of the Creative Commons Attribution License (CC BY). The use, distribution or reproduction in other forums is permitted, provided the original author(s) or licensor are credited and that the original publication in this journal is cited, in accordance with accepted academic practice. No use, distribution or reproduction is permitted which does not comply with these terms.
*Correspondence: Lucy G. Gillis, bHVjeS5naWxsaXNAbGVpYm5pei16bXQuZGU=
Disclaimer: All claims expressed in this article are solely those of the authors and do not necessarily represent those of their affiliated organizations, or those of the publisher, the editors and the reviewers. Any product that may be evaluated in this article or claim that may be made by its manufacturer is not guaranteed or endorsed by the publisher.
Research integrity at Frontiers
Learn more about the work of our research integrity team to safeguard the quality of each article we publish.