- Department of Geology, Lund University, Lund, Sweden
We explore the distribution of sponges along dissolved silica (dSi) concentration gradients to test whether sponge assemblages are related to dSi and to assess the validity of fossil sponges as a palaeoecological tool for inferring dSi concentrations of the past oceans. We extracted sponge records from the publically available Global Biodiversity Information Facility (GBIF) database and linked these records with ocean physiochemical data to evaluate if there is any correspondence between dSi concentrations of the waters sponges inhabit and their distribution. Over 320,000 records of Porifera were available, of which 62,360 met strict quality control criteria. Our analyses was limited to the taxonomic levels of family, order and class. Because dSi concentration is correlated with depth in the modern ocean, we also explored sponge taxa distributions as a function of depth. We observe that while some sponge taxa appear to have dSi preferences (e.g., class Hexactinellida occurs mostly at high dSi), the overall distribution of sponge orders and families along dSi gradients is not sufficiently differentiated to unambiguously relate dSi concentrations to sponge taxa assemblages. We also observe that sponge taxa tend to be similarly distributed along a depth gradient. In other words, both dSi and/or another variable that depth is a surrogate for, may play a role in controlling sponge spatial distribution and the challenge is to distinguish between the two. We conclude that inferences about palaeo-dSi concentrations drawn from the abundance of sponges in the stratigraphic records must be treated cautiously as these animals are adapted to a great range of dSi conditions and likely other underlying variables that are related to depth. Our analysis provides a quantification of the dSi ranges of common sponge taxa, expands on previous knowledge related to their bathymetry preferences and suggest that sponge taxa assemblages are not related to particular dSi conditions.
Introduction
Sponges are aquatic, sessile, benthic, and filter feeding organisms that belong to the animal phylum Porifera. Their body structure is simple, with different kinds of cells specialized in a variety of life functions and organized in different layers according to their function (Hooper and Van Soest, 2002; Van Soest et al., 2012; Maldonado, 2014). Their origins have been placed in the Cambrian (Hooper and Van Soest, 2002; Xiao et al., 2005), or even before as suggested by the discovery of potential sponge biomarkers (Love et al., 2009; Yin et al., 2015; Gold et al., 2016).
Four classes of sponges are currently recognized: Calcarea, which includes species with calcareous skeletons; Hexactinellida, commonly known as glass sponges; Demospongiae, containing the majority of extant sponges; and the Homoscleromorpha (Hooper and Van Soest, 2002; Morrow and Cárdenas, 2015; Van Soest et al., 2017). The last three classes include species with siliceous skeletons cemented by organic components (i.e., a collagen-like protein, spongin), as well as species that lack a mineral (i.e., neither siliceous nor calcareous) skeleton and are constructed with spongin skeletons, occasionally reinforced with foreign inorganic elements (e.g., sand or remains of biogenic silica from other organisms, including sponges; Hooper and Van Soest, 2002).
Through complex enzymatic and metabolic processes (Wang et al., 2011a,b, 2012a), siliceous species of Hexactinellida, Demospongiae and Homoscleromorpha take up Si (predominantly present as silicic acid, H4SiO4 and conventionally referred to as dissolved silica; dSi) from their surrounding water to secrete individual structures known as spicules which provide skeletal support to the organic components of the sponge (Uriz et al., 2003). In some species of hexactinellids and demosponges, skeletal structures are massive and hypersilicified, with stony consistency and can form agglomerates and build reef-like formations (Conway et al., 2001; Uriz et al., 2003; Leys et al., 2004; Maldonado et al., 2015a,b) or “sponge grounds” (Klitgaard and Tendal, 2004). Sponges of these groups may be important players in the global silicon cycle due to their widespread occurrence and high preservation potential (Maldonado et al., 2005, 2010).
Silica uptake and silicification in sponges is regulated by the availability of dSi in the ambient water and dSi uptake conforms to enzymatic (Michalis-Menten) kinetics with maximal uptake rates occurring only above 100–200 μM dSi (Reincke and Barthel, 1997; Maldonado et al., 1999, 2011). These values are higher than typical concentrations in most of the modern ocean, suggesting sponge dSi uptake mechanisms evolved in higher dSi waters (Maldonado et al., 2015a) and dSi is a limiting factor for living sponges (Maldonado et al., 2011). Maldonado et al. (1999) also showed that secretion of certain spicule types is regulated by dSi thresholds and suggested that instances of these spicules in fossil sponges reflect dSi replete environments. Efficient mechanisms to utilize dSi have evolved in sponges (Müller et al., 2003; Schröder et al., 2004; Wang et al., 2012a,b) and involve the presence of silicatein, an enzyme that is able to control and catalyze the intracellular deposition of dSi from an unsaturated environment, as well as proteins that actively transport dSi across the cell membranes (Schröder et al., 2004; Marron et al., 2016).
Additional information about variations in the shape, or size of sponge spicules, or about disrupted spiculogenesis (shape changes, atrophies or other malformation of spicules) in relation to dSi concentration is scattered throughout the literature (Jørgensen, 1944; Hartman, 1958; Stone, 1970; Yourassowsky and Rasmont, 1984; Zea, 1987; Bavestrello et al., 1993; Rützler and Smith, 1993; Schönberg and Barthel, 1997; Mercurio et al., 2000; Valisano et al., 2012; Cárdenas and Rapp, 2013). Overall these studies show that the availability of dSi is a factor that can affect the morphology, composition or size of spicules in some species.
If spicule morphology and/or morphometrics are affected by dSi and given that these are the main underlying characters used for the classification of siliceous sponges (Hooper and Van Soest, 2002), some taxa or taxa assemblages may be characteristic of habitats with particular dSi conditions. These might conceivably have different adaptation mechanisms and therefore, differentially influence the ocean Si cycle. An essential first step to use sponges as indicators is recognizing and quantifying any causal relationship between modern sponge distributions and the underlying environmental drivers; this is the crucial first step in palaeoecological proxy development (e.g., Birks, 1995).
The spatial distribution of Porifera has been studied at local and regional scales and has been related to different environmental factors. Differences in sponge species composition between shallow and deep waters have been acknowledged for decades. De Laubenfels (1936) recognized that the spatial distribution of shallow water sponges is limited by strong environmental barriers (e.g., depth, light or temperature). More recently, the spatial distribution of marine sponges at local scales (e.g., 10 s of km; coral reefs, straits or gulfs) has been correlated to geomorphological features (Przeslawski et al., 2014), sediment properties, depth, distance to the coast, nutrient availability (including dSi), light penetration, hydrodynamics (Huang et al., 2011), deep sea currents (Cárdenas and Rapp, 2015), and biotic factors such as predation or competition for space (Huang et al., 2011; Pawlik et al., 2015; Slattery and Lesser, 2015). In general, these observations are replicated at regional or broader scales (e.g., >>100 km; seas or oceans). Here, sponge distributions have been related to depth, temperature and light availability (Vacelet, 1988; Finks and Keith Rigby, 2003; Leys et al., 2004; Downey et al., 2012), or to biogeographic regions (Hooper and Lévi, 1994; Van Soest, 1994; Van Soest et al., 2012) delimited by underlying abiotic drivers and characteristic marine biotas (e.g., Spalding et al., 2007). Interestingly, results from predictive models using physico-chemical environmental variables (Huang et al., 2011) and regional surveying (Howell et al., 2016) suggest that temperature, depth and nutrient status—including dSi—are major drivers controlling sponge distribution.
There is potential for sponge species composition and spicule morphology in the sedimentary record to yield insight into dSi at the time of their formation. The reconstruction of paleo-dSi is receiving increasing attention, partly motivated by the linkages between the long-term carbon and silicon biogeochemical cycles (Conley et al., 2000; Frings et al., 2016). To date, dSi reconstruction using sponges has been achieved by exploring morphometric relationships of fresh-water spicules through dSi gradients (Kratz et al., 1991). More recently, silicon isotope analysis of sponge spicules has exploited the observation that the fractionation of stable Si isotopes between ambient dSi and the biogenic Si in spicules is a function of dSi concentration in the surrounding environment (Hendry et al., 2010, 2011; Wille et al., 2010; Hendry and Robinson, 2012; Fontorbe et al., 2016, 2017). The development of a complementary palaeoecological approach based on taxa assemblages could greatly extend the understanding of dSi changes in past and present oceans. By estimating the distributions of sponge taxa along a dSi gradient, particular species assemblages can be identified to provide insight into past Si dynamics in ocean and coastal environments.
In this work we explore the distribution of sponges along dSi gradients to test whether sponge assemblages are a function of dSi and to assess the validity of sponge taxonomy as a palaeoecological tool for inferring palaeo-concentrations of dSi. To achieve this we extracted and combined information from publically available oceanographic and biodiversity databases to evaluate the dSi conditions where sponges are found in the global ocean. Because dSi is correlated with depth in the modern ocean, we also explore taxa distributions as a function of depth. In general, we find that while there are hints of an assemblage-dSi relationship, sponges are not sufficiently differentiated as a function of dSi availability to clearly indicate specific dSi concentrations, a conclusion with implications for reconstructions of the ocean Si cycle.
Materials and Methods
Data Sources
We used sponge records held in the Global Biodiversity Information Facility (GBIF) and a gridded climatology of ocean chemistry data, interpolated from measurements, from the World Ocean Circulation Experiment (WOCE) (Gouretski and Koltermann, 2004). This is available with a horizontal grid resolution of 0.5° and with 40 unevenly spaced vertical layers. GBIF is an international open data infrastructure and the world's largest biodiversity database, and provides a freely available, single point access to millions of biodiversity records (http://www.gbif.org/what-is-gbif). GBIF aggregates data from a variety of sources of variable extent, quality and taxonomic precision (see www.gbif.org for more information). WOCE is a global data resource of the US National Oceanic and Atmospheric Administration ultimately based on observations from ships, floats, drifters and satellites. We interrogated GBIF via the online portal (http://www.gbif.org/occurrence) and downloaded 323,953 georeferenced records of Porifera available in November 2016 (GBIF Occurrence Download: https://doi.org/10.15468/dl.viufhq). The taxonomic classification of species in these records follows that of the World Porifera Database (WPD, http://www.marinespecies.org/porifera/news.php?p=show&id=4893). Only records with an associated depth (143,275; 44%) were used in the analyses. Paleontological records and freshwater taxa included in the dataset were also removed (830 and 192 records, respectively). To validate the geolocation data of GBIF, we compared the water depth associated with each record with the ETOPO1 1 Arc-Minute Global Relief Model (Amante and Eakins, 2009). Because both datasets contain variable quality and resolution depth data, records that disagreed by more than 500 m were discarded. Agreement was taken as a sign of confidence in the depth data. This 500 m threshold was selected (rather arbitrarily) to allow for (i) the possibility of steep bathymetry at spatial scales below that of ETOPO1 and (ii) the inclusion of dredge samples, where only the trawl start or finish point is given. This quality control process lead to the exclusion of 5,190 (ca. 3.6%) of datapoints, which in some cases were in disagreement by > 6000 m (see Supplementary Figure 1). In addition, any remaining records which were flagged within GBIF as having a “non-numeric” or “non-metric” depth in the original dataset (13,317 and 5,060, respectively) were removed, to avoid any conversion issues.
Information at the level of class and order, missing in some of the GBIF records although the lower taxonomic rank of family or genus was given, was added following the WPD. GBIF contains a mixture of reliable and unreliable taxonomic information (Van Soest et al., 2012), and includes records with taxonomic identifications derived from photographs and/or video-recordings, which e.g., Leys et al. (2004) have identified as a source of uncertainty. We assumed that the taxonomic identifications included in the GBIF records at the level of order and family are more accurate than those from lower levels (genera and species), which generally require a greater degree of taxonomic expertise. For this reason we limit our analyses to the level of family and above (order and class). Our analyses could be improved and extended to a higher taxonomic resolution with an increase in the reliability of taxonomic identifications of GBIF records.
The final dataset for this study included 62,360 discrete, globally distributed records (Figure 1). Of these, 2,905 are classified only as far as class, 890 only as far as order (31 different orders), 2086 to family level (128 different families), 24,525 to the level of genus (555 different genera) and the remainder (31,955) to species (2,758 different species) or subspecies. For comparison, the World Porifera Database (Van Soest et al., 2017) currently recognizes 32 extant orders, 149 extant families and 740 extant genera, implying we capture a large proportion of total global sponge biodiversity.
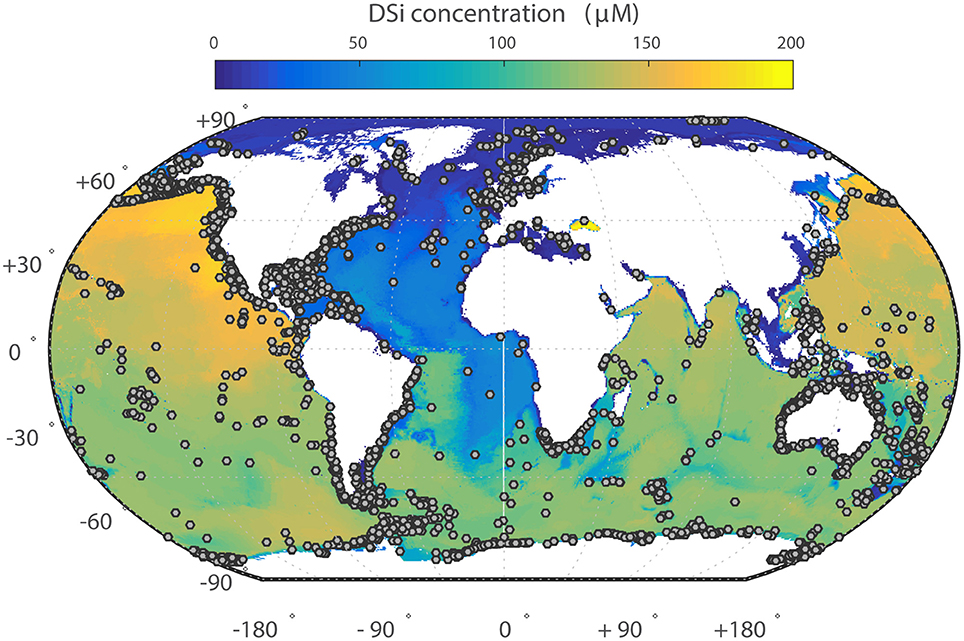
Figure 1. Ocean dissolved silica concentrations in bottom waters (i.e., seafloor). Gray dots indicate the location of individual sponge records included in this manuscript from GBIF.
For each individual sponge observation in GBIF, ocean temperature, salinity and nutrient concentrations (nitrate, phosphate and dSi) were extracted from WOCE (Gouretski and Koltermann, 2004) from the box enclosing the recorded sponge location. A summary of the data, including counts, maxima, minima and averages for each variable on a taxa-by-taxa basis for each of the families represented in the data set is provided (Supplementary Table 1).
Effect of Sampling Techniques and Sampling Effort
dSi concentrations in ocean bottom waters and the locations of the sponge records from the dataset are shown in Figure 1. Most of the records examined are limited to shallow water collections where dSi is generally low, which we attempt to account for in our analysis (see below). However other sampling biases might also be present. For instance, deep sea habitats are usually sampled with trawls or dredges. These generally catch abundant, large or hard specimens, but groups represented by small or fragile individuals (e.g., Cladorhizidae and Calcarea) are often not recovered (Raff et al., 1994), and therefore might be underrepresented. At present, quantifying the scale and significance of this bias is not possible.
We investigated the impact of very intense sampling within small regions by first regarding the taxa as only present or absent for each box in the WOCE dataset, and calculating the global distribution along an environmental gradient using 20 equally spaced bins for each variable (shown for dSi in Figure 2A). The presence/absence aggregation made only a very minor difference to the distribution of taxa along environmental gradients (Figure 2B), so we proceed with the full (i.e., non-aggregated) dataset. To crudely address a sampling bias toward continental shelves and shallow water areas in GBIF records we normalized the taxa distributions to the total Porifera distribution (Figure 2) to obtain a suite of distributions that describe the relative importance of individual taxa to the total Porifera population along any given environmental gradient. This approach implicitly assumes that sampling and identification of the Porifera records is not biased toward one group of taxa and is not suitable for e.g., calibrating individual sponge taxa environmental preferences. However, the intent here is not to estimate the true distribution of sponge taxa in the global ocean, but rather to explore relative differences among taxa. In the Supplementary Table 1 we show a summary of the uncorrected data.
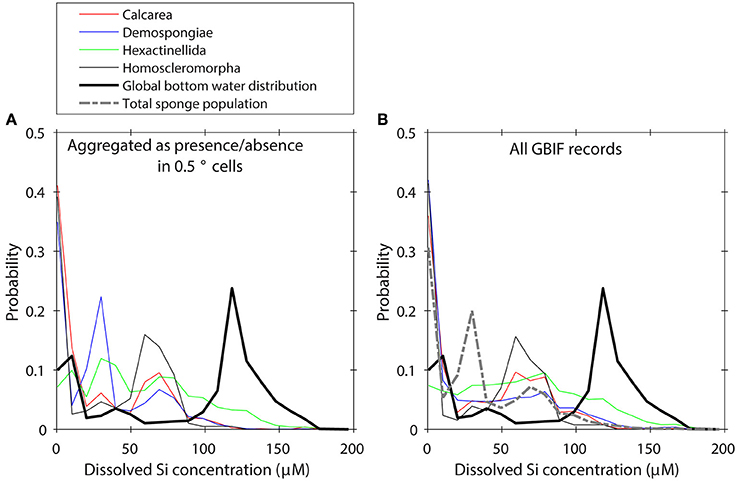
Figure 2. (A) Global distribution of GBIF records aggregated as presence/absence values in 0.5° boxes of the WOCE dataset. (B) Global distribution of GBIF records without correction for sampling bias.
Violin Plots
For taxa that are abundant (more than 100 records in GBIF), violin plots of the normalized data as a function of underlying environmental gradients were created at different levels of taxonomic classification (class, order, and family) and form the basis of further discussion. Where applicable, we include sponge species (referred to as “lithistids” hereafter) with highly silicified skeletons that were previously classified in the polyphyletic order Lithistida (Pisera and Lévi, 2002) but are now divided among unrelated orders of the class Demospongiae (Cárdenas et al., 2012). In these plots, the width of the bars indicates the relative contribution of the taxa to the total of all sponge taxa recorded in GBIF at the corresponding value of the environmental variable; note these are further standardized to the maximum contribution of that taxa, so do not contain any useful information about the absolute abundance of any given taxa, which is not possible with the “presence-only” GBIF data.
Results
The relative frequency distribution of sponge taxa vs. dSi and depth is shown in Figures 3–5. At the level of class the Hexactinellida are found across broad ranges of dSi (0–180 μM; Figure 3A) and depth (0–6,000 m; Figure 3B). Sponges with calcareous skeletons show similar distributions but are a greater relative contributor to total sponge taxa at dSi < 100 μM and < 3,000 m depth. The rest of the classes are also found in the same range, however their major contributions to the total are below 120 μM and shallower than 1,000 m for the siliceous classes Homosclerophorida and Demospongiae.
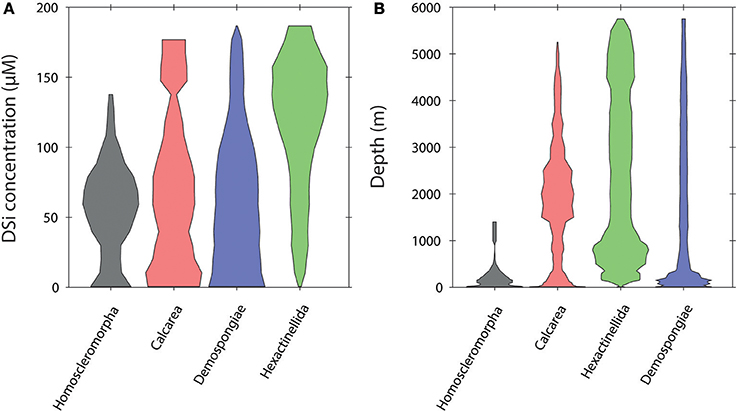
Figure 3. Violin plots showing the distribution of the sponge classes in a dSi gradient (A) and a depth gradient (B).
In our analysis, 19 of the 32 total sponge orders are represented within GBIF in at least 100 boxes of the WOCE dataset (Figure 4). A majority occur seem dominantly at dSi < 50 μM, while other groups of demosponges (Tethyida, Suberitida Polymastiida and Haplosclerida), hexactinellids (Hexactinosa and Lyssacinosida), homosclerophorids and the Clathrinida are more evenly distributed along the dSi gradient. The hexactinellid order Amphidiscosida make their greatest contributions to sponge assemblages in environments with dSi>100 μM and the calcarean order Leucosolenida shows a bimodal distribution. Figure 4B shows the distribution of the same orders as a function of depth. A comparison between the two plots indicates that orders with relatively more frequent occurrences at higher dSi are also represented at depths greater than 2,000 m. The demosponge orders without siliceous skeletons (i.e., Dendroceratida, Verongiida –excluding some species (Ehrlich et al., 2010), and the Clionaida are not found in the GBIF database at depths greater than 1000 m. The distribution of lithistids along the depth gradient indicates this group is a relatively consistent contributor to total sponge assemblages at dSi below 50 μM and is not found at depths greater than 2,500 m.
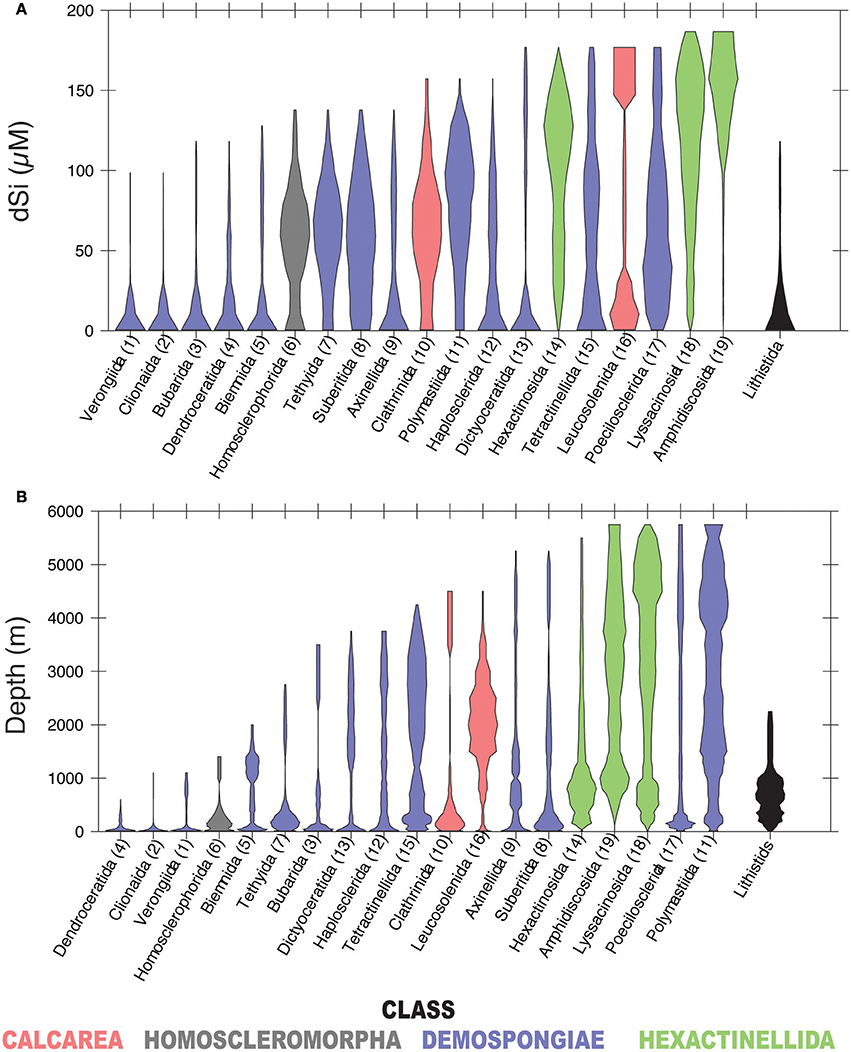
Figure 4. Violin plots showing the distribution of sponge orders along the dSi gradient (A) and the depth gradient (B). Only orders represented within GBIF in at least 100 boxes of WOCE dataset are shown. Numbers in parenthesis are codes given to each of the represented orders. Lithistids includes taxa assigned to this taxon by Pisera and Lévi (2002). Taxa with gray background include sponges without siliceous spicules. Color codes as in Figure 3.
At the highest level of taxonomic resolution that we consider, 43 families within Porifera occur in 100 or more WOCE boxes (for reference, there are 149 currently recognized families within Porifera; Van Soest et al., 2017). Their distributions follow similar tendencies to those shown at the level of order, with some families better represented at dSi < 50 μM, some approximately equally represented along the gradient, some occurring at both high and low dSi and others more likely to be found at dSi>100 μM (Figure 5A). Distribution of these families along the depth gradient (Figure 5B) roughly tracks the dSi gradient. Families that occur at high dSi such as the Cladorhizidae, and Polymastiidae and all of the hexactinellids, are also likely to occur in deep waters. Non-siliceous sponges of the orders Verongiida, Dictyoceratida and Dendroceratida, with the exception of Spongiidae (see discussion below), are found within the group of taxa that occur at dSi < 20 μM, together with the family Clionidae (usually sponges associated with calcium carbonate substrates) but also with families that have siliceous skeletons (i.e., Petrosiidae, Dictyonellidae and Callyspongiidae). These are only found at shallow depths (Figures 5A,B).
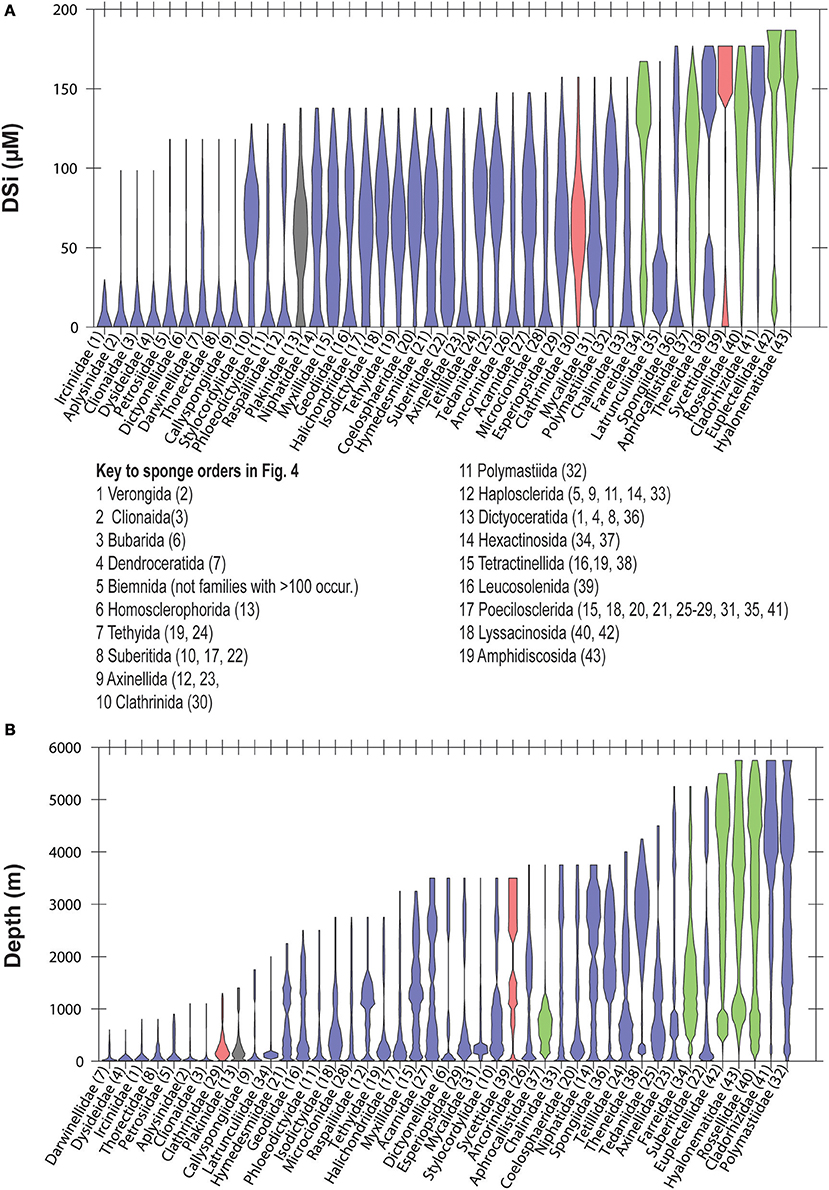
Figure 5. Violin plots showing the distribution of sponge families in a dSi gradient (A) and a depth gradient (B). Only families represented within GBIF in at least 100 boxes of WOCE dataset are shown. Numbers in parenthesis are codes given to each family. Taxa with gray background include sponges without siliceous spicules. A key to the orders represented in Figure 4 is included. Color codes as in Figure 3.
Discussion
Our analysis provides a quantification of the dSi ranges of common sponge taxa and expands on previous knowledge related to their bathymetry (Vacelet, 1988; Maldonado and Young, 1998; Rapp et al., 2011; Downey and Janussen, 2015; Hestetun et al., 2015). The distribution of sponge taxa along a depth gradient exhibits clear similarities to dSi, meaning the challenge is to distinguish between the two.
Below, we discuss the role of dSi availability, and the effects of other variables that might be indirectly influencing the patterns presented here. We also discuss how this data might contribute to clarifying the role of sponges in the ocean Si cycle in the geological past, and the usefulness of sponge assemblage data as a marine palaeoecological tool.
Effects of dSi Availability and Habitat Depth on Sponge Distributions
Depth is an important factor controlling sponge distribution (Vacelet, 1988; Huang et al., 2011; Howell et al., 2016), though it acts indirectly via its correlation with ecologically meaningful parameters (including dSi concentrations, but also light, food availability, temperature, etc.,). It is evident that either dSi, depth or both play a major role controlling the spatial distribution of sponge taxa (Figures 3–5). Our results confirm that the Porifera are not uniformly distributed along either a dSi or a depth gradient. Because the differentiation along the two gradients is similar, it is unclear whether dSi availability, habitat depth, or another unidentified factor (or combination of factors), is driving the spatial distributions of sponges. At least at regional scales, water depth acts as a surrogate variable for light penetration, temperature, and nutrient concentrations; these all are inter-correlated and could be influencing sponge distributions (Vacelet, 1988). Other depth-related factors such as food resources (i.e., particulate and dissolved matter), turbulence, spatial competition, and predation have been suggested as drivers of sponge community structure and distribution (Pawlik et al., 2015; Slattery and Lesser, 2015).
Bottom-water temperature and concentration of some nutrients have also been identified as important variables controlling sponge distribution at local scales (Huang et al., 2011; Howell et al., 2016). However, the distribution of sponges as a function of temperature, dissolved oxygen and nutrient (nitrate and phosphate) concentrations extracted from WOCE (see Supplementary Material) revealed that the distribution of sponge taxa along these gradients is not strongly differentiated, in contrast to the dSi and depth gradients. Although we cannot exclude these factors, the fact that habitat depth shows a similar sponge distribution pattern with dSi is suggestive of dSi availability being an important driver of the spatial distribution of some sponge groups, and implies that sponge distribution as a function of depth simply reflects the general relationship between depth and [Si] (see below; Figure 6). Unfortunately, we cannot strictly identify the direction of causality and rule out the possibility that depth is simply acting as a surrogate for another, as yet unidentified variable.
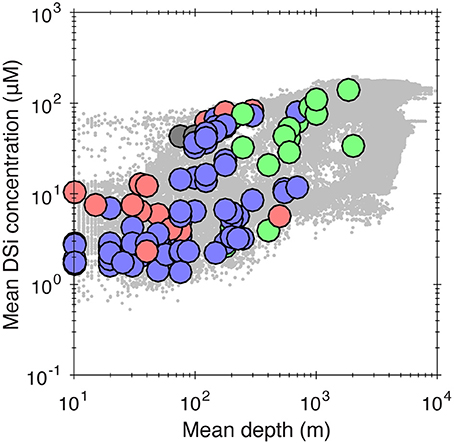
Figure 6. Cross-plot of mean habitat depth and mean ambient dissolved Si concentrations for all sponge families in the GBIF database. See previous figures for key to colors. All taxa are included regardless of their abundance, unlike Figures 3–5 where an occurrence criterion of 100 WOCE boxes is imposed. Data are aggregated at the presence/absence level for individual WOCE boxes. Gray dots in the background are ocean bottom water dSi concentrations (i.e., the same data as Figure 1).
In general, ocean dSi correlates with depth (Sarmiento and Gruber, 2006), as biogenic silica produced by siliceous plankton at the surface progressively dissolves as it sinks through the water column (although this pattern is somewhat complicated by the marine overturning circulation that acts to mix water-masses of varying ages, and therefore accumulated dSi) and because of high diatom production due to upwelling. To further explore this issue, we have plotted dSi and depth for all families included in our dataset in Figure 6. This confirms the presence of a positive relationship between dSi and depth with a similar form to that for the whole ocean (Figure 6).
Sponge Assemblages along dSi And Depth Gradients
Our data show that either depth or dSi play major roles controlling the spatial distribution of some taxa (Figures 3–5). Below we discuss which of those taxa might include species with different dSi uptake requirements and therefore could be indicative of dSi conditions.
Hexactinellida
Species of the order Hexactinosa (e.g., Farrea occa, Aphrocallistes vastus and Heterochone calyx) are known to construct hypersilicified skeletons and to form sponge reefs where dSi is reasonably high (ca. 40 μM), e.g., on the western coast of Canada (Leys et al., 2004; Conway et al., 2005; Whitney et al., 2005; Chu et al., 2011). The occurrence of these reef-building species is thought to be controlled by the combined action of particular oceanographic, geomorphological conditions and biotic factors, including dSi availability (Conway et al., 2005; Maldonado et al., 2015a). Hexactinosa (Figure 4) are important components of the sponge fauna at high dSi and depth together with species belonging to Amphidiscosida, Aulocalycoida, and Lyssacinosida (Rossellidae), which lack a dyctional (fused spicules in a 3D framework) skeleton suitable for reef formation but nevertheless have relatively more dSi per biomass unit than sponges from other sponge classes (Barthel, 1995).
Demospongiae
The families Cladorhizidae, and Polymastiidae are relatively more likely to be found in dSi rich waters (Figure 5A). These families have also a broad distribution range along depth gradients and are likely to be found in deep waters (>2,000 m) where dSi is also high. Whether or not the distribution of these taxa is driven by dSi or by depth remains to be answered. Detailed studies of dSi uptake mechanisms and rates of these sponge species, living in different dSi conditions will contribute to answer this question and to identify species that can be used as indicators of particular dSi ranges.
The remaining siliceous and non-siliceous demosponges are likely to be found in low dSi habitats, although some of those might occur at greater depths with high dSi. Some of Dictyoceratida families (i.e., Spongiidae), which lack siliceous skeletons, are likely to occur at high dSi and depth (Figure 5). This is an interesting observation because members of this group are conventionally thought to be found in tropical latitudes and shallow waters (Vacelet, 1988) and in some species the settlement of their larvae is affected by low temperatures, and light penetration (Maldonado, 2009). As far as we know, species of Spongiidae have not been reported from bathyal depths in the taxonomic literature. It is possible that these records might be misidentified, given that the taxonomy of these sponges is extremely difficult. However, until independent verification of these Dictyoceratida records we assume that their documentation in GBIF is correct, and therefore that other factors correlated to depth (including temperature, light penetration, nitrate or phosphate concentrations) are driving the occurrence of particular species of this group.
Lithistids
Sponges included in this polyphyletic (diverse phylogenetic affinities) group include species with articulated spicules called desmas, that form a rigid skeleton in most species (Pisera and Lévi, 2002). The majority (93%) of lithistid records are from the order Tetractinellida, and the most common families were Theonellidae (21%), Corallistidae (18%), and Scleritodermidae (17%). According to Figure 4, lithistid taxa are more likely to be found at < 60 μM dSi, with the greatest relative frequency at < 20 μM and < 1,000 m. Some of them are known to build reef-like formations in habitats where dSi availability is low relative to that reported for the Hexactinosida reef-like builders (Maldonado et al., 2015a,b). Hypersilicified species lithistid (e.g., Leiodermatium spp) also cohabit with members of sponges without siliceous skeletons (i.e Dictyoceratida, Dendroceratida, and Verongiida) (Przeslawski et al., 2014, 2015) in relatively shallow habitats of northern Australia, with dSi concentrations <5 μM, exposed to strong tidal currents, high turbidity, and substantial sediment mobility (Przeslawski et al., 2011). As suggested by Maldonado et al. (2015b), some lithistid species seem to benefit from heavy sediment deposition, and patterns of local circulation that might deliver food and Si in pulses. It remains to be answered how these hypersilicified skeletons of lithistid species can develop in lower dSi and whether or not these species might have more efficient Si uptake systems than other taxa such as Hexactinosida which tend to be found in comparatively higher dSi (Figures 4, 5).
Calcarea
The GBIF data shows that sponges of the class Calcarea are found in the depth gradient up to 5,000 m with peaks below 3,000 m. This confirms previous findings (Duplessis and Reiswig, 2000; Rapp et al., 2011) and suggest that the diversity of the group at abyssal depths is higher than previously thought, and that they are occasionally capable of growing in environments below carbonate mineral saturation.
Toward Quantification
There is a large body of literature that deals with methods to derive quantitative palaeoenvironmental reconstructions from (micro)fossil assemblages, in terrestrial, freshwater, and marine environments. As summarized in e.g., Telford and Birks (2005) or Juggins (2013), there is an increasing awareness of the limitations of these approaches, even in favorable situations. We have decided against a more quantitative approach for several reasons, including (i) GBIF data are compiled from disparate, unconnected sources with different sampling strategies, (ii) the higher end of the dSi gradient is poorly represented in the data, (iii) the data are “presence-only,” rather than true species abundances, meaning taxa optima can not be easily defined, (iv) we have no independent model verification dataset, (v) variance-partitioning presumably will indicate a high degree of shared variance between depth and dSi, and (vi) the environmental calibration data has a hard-to-quantify noise component, being derived separately from the GBIF data at a lower spatial resolution.
In the future, a true sponge assemblage (with taxa abundances) and environmental variable dataset collected in a region or regions where dSi is decoupled from depth would be able to overcome some of these difficulties. Sponge silicon isotope ratios are becoming more widely used as a proxy for past-dSi concentrations (e.g., Fontorbe et al., 2017). Though not widely used, “mutual-range” approaches (Sinka and Atkinson, 1999), modern analog techniques (MAT; Lytle and Wahl, 2005), or artificial neural networks (Racca et al., 2007) may prove fruitful palaeoecological complements. Finally, lab- to field scale experiments carefully designed to extract the influence of dSi availability on sponge physiology and ecology, or approaches that seek to use individual spicule morphometrics (cf. Kratz et al., 1991) may also be part of the developing palaeo-dSi “toolbox.”
Did dSi Control Sponge Distributions in the Past?
The evolution of the ocean Si cycle has received attention for many decades (e.g., Maliva et al., 1989; Kidder and Tomescu, 2016). The prevailing narrative (Maliva et al., 1989; Siever, 1991; Kidder and Tomescu, 2016) argues for two particularly important events. The first is a transition from a Precambrian ocean saturated with respect to dSi to a slightly undersaturated Mesozoic ocean. The second was a further decrease, sometime in the Cretaceous-Paleogene, in the dSi content of mean ocean water to a modern, dSi-deplete ocean. Both transitions are ascribed to biological innovations—the first to the evolution of silicification by radiolarians and sponges, and the second to the expansion of diatoms—and therefore biology's progressive ability to remove dSi from seawater. Superimposed on this long-term decline in ocean dSi, transient excursions in the ocean dSi inventory have been proposed in response to periods of extensive volcanism (particularly the emplacement of large igneous provinces, LIPs) (Ritterbush et al., 2014, 2015; Kidder and Tomescu, 2016), or orogenesis (e.g., Cermeño et al., 2015). One of the lines of evidence supporting these interpretations is a change in the sedimentary facies associated with sponges in the rock or sediment record (e.g., Kidder and Tomescu, 2016). The link between sponge abundances/distributions and dSi stems from the implicit assumption that siliceous sponges are unable to thrive at low dSi (e.g., Ritterbush et al., 2015; Kidder and Tomescu, 2016). Kidder and Tomescu (2016), for example, interpret an Ordovician retreat in sponges from lagoonal depositional environments toward deeper, shelf settings, as reflecting radiolarian driven dSi depletion of the surface ocean. After the mid-Ordovician, sponge fossils from peritidal and lagoonal facies are apparently rare in the fossil record (see Gammon et al., 2000) for a possible Eocene exception. Maliva et al. (1989) argue that sponges become quantitatively unimportant in the ocean Si cycle during the Cenozoic. To what extent are these putative changes in sponge abundance and distribution driven by dSi availability?
Our dataset shows that all sponge groups that are sufficiently sampled (i.e., >100 WOCE boxes, see Supplementary Table 1) are capable of growing in low dSi environments. Indeed, at least in some isolated instances, siliceous sponge taxa are capable of forming large reef-like aggregations at <10 μM dSi (Maldonado et al., 2015a,b). This implies that while any decline in dSi availability may have been a contributory factor to sponge exclusion from shallow water environments, other factors, perhaps related to the evolution, and ecology of sponge competitors should be taken into account. Therefore the presence of any given taxon cannot be in and of itself be indicative of the prevailing dSi concentrations.
For example, reef-building litihistid species, used as indicators of greater dSi availability in past environments (e.g., Corsetti et al., 2015; Ritterbush et al., 2015), are in our dataset more frequent <20 μM and < 2,500 m. There is currently no evidence to suggest that they were unable to live in similar environments in the geological past (Pisera, 1997). The expansion of certain sponge groups after the Triassic-Jurassic extinction is more likely a consequence of a greater availability of suitable ecological niches vacated after the collapse of calcareous invertebrates in that period (Ritterbush et al., 2014, 2015).
Lastly it is important to mention that generally the majority of siliceous and non-siliceous sponges are not well represented in the fossil record as their skeletons are easily destroyed. Species with rigid skeletons, e.g., lithistids, some hexactinellids and fossil sponges with basal calcareous skeletons (i.e., Chaetetida and Stromatoporoidea) are more likely to be preserved, biasing the stratigraphic record (Pisera, 2006).
Conclusions
We show here that the distribution of sponges classified at the level of family is related to ambient dSi concentrations or habitat depth, or both. The distribution patterns along dSi concentrations or habitat depth indicate that the majority of taxa, including those in the class Calcarea without siliceous skeletons, occur in broad dSi (0–180 μM) and depth (0–~5,500 m) ranges. It also shows that the majority of demosponges without autochthonous siliceous skeletons are found at dSi <20 μM and shallow depths, but together with groups that have skeletons made up of siliceous spicules. Demosponges with rigid siliceous skeletons and traditionally known as lithistids do not seem to be associated with deep environments rich in dSi indicating that these kind of sponges might have evolved particularly efficient mechanisms to incorporate and accumulate dSi in their skeletons. Hexactinellids on the other hand, also builders of strong and rigid siliceous skeletons are distributed along the dSi gradient but are more likely to be present in deep and silica-rich environments. The hexactinellids share this kind of environments with some demosponges evenly distributed along the dSi and depth gradient and mainly from the orders Cladorhizidae and Polymastiida.
The groups skewed to either low or high dSi might include species indicative of specific environmental Si conditions. As no unique or clear assemblage of taxa could be identified, additional information on Si uptake rates for possible indicative species is required to conclusively relate their spatial distribution to dSi levels. One might expect to see greater differentiation along environmental gradients if sponges are considered at the level of genera or even species, though we note that some genera (e.g., Geodia) are highly cosmopolitan. Similarly, studying dSi uptake mechanisms of sponge species occurring at a broad dSi and depth ranges could provide essential information on the adaptation potential of sponge taxa.
Links between the abundance of sponges in sedimentary records and the contemporary dSi concentrations should therefore be interpreted with caution, given these organisms are clearly adapted to live in a range of dSi concentrations and depth habitats, as seen in our results. Other biotic (e.g., competitive interactions) and abiotic factors (e.g., light penetration, temperature, nutrient and food availability), some of which are also related to depth, may be important factors in the distributions of modern sponges - and were probably equally important in palaeo-environments.
Author Contributions
All authors listed have made a substantial, direct and intellectual contribution to the work, and approved it for publication. BA and PF are joint senior authors.
Conflict of Interest Statement
The authors declare that the research was conducted in the absence of any commercial or financial relationships that could be construed as a potential conflict of interest.
Acknowledgments
This study was funded by a grant from the Knut and Alice Wallenberg Foundation to DC. Dmitry Schigel, GBIF and Merrick Ekins, Queensland Museum, provided valuable comments and suggestions related to the data downloaded from the GBIF portal. We thank all the suggestions and revisions of the reviewers.
Supplementary Material
The Supplementary Material for this article can be found online at: https://www.frontiersin.org/articles/10.3389/fmars.2017.00373/full#supplementary-material
References
Amante, C., and Eakins, B. W. (2009). Etopo1 1 Arc-Minute Global Relief Model: Procedures, Data Sources and Analysis. NOAA Technical Memorandum NESDIS NGDC-24. Boulder, CO: National Geophysical Data Center, NOAA.
Barthel, D. (1995). Tissue composition of sponges from the Weddell Sea, Antarctica: not much meat on the bones. Mar. Ecol. Prog. Ser. 123, 149–153. doi: 10.3354/meps123149
Bavestrello, G., Bonito, M., and Sara, M. (1993). Silica content and spicular size variation during an annual cycle in Chondrilla nucula Schmidt (Porifera, Demospongiae) in the Ligurian Sea. Sci. Mar. 57, 421–425.
Birks, H. J. B. (1995). “Quantitative palaeoenvironmental reconstructions,” in Statistical Modelling of Quaternary Science Data, eds D. Maddy and J. S. Brew (Cambridge, Quaternary Research Association), 161–254.
Cárdenas, P., Pérez, T., and Boury-Esnault, N. (2012). “Chapter two - sponge systematics facing new challenges,” in Advances in Marine Biology, eds M. A. Becerro, M. J. Uriz, M. Maldonado, and X. Turon (London: Academic Press), 79–209.
Cárdenas, P., and Rapp, H. T. (2013). Disrupted spiculogenesis in deep-water Geodiidae (Porifera, Demospongiae) growing in shallow waters. Invertebr. Biol. 132, 173–194. doi: 10.1111/ivb.12027
Cárdenas, P., and Rapp, H. T. (2015). Demosponges from the Northern mid-atlantic ridge shed more light on the diversity and biogeography of North Atlantic deep-sea sponges. J. Mar. Biol. Assoc. U.K. 95, 1475–1516. doi: 10.1017/S0025315415000983
Cermeño, P., Falkowski, P. G., Romero, O. E., Schaller, M. F., and Vallina, S. M. (2015). Continental erosion and the Cenozoic rise of marine diatoms. Proc. Natl. Acad. Sci. U.S.A. 112, 4239–4244. doi: 10.1073/pnas.1412883112
Chu, J., Maldonado, M., Yahel, G., and Leys, S. (2011). Glass sponge reefs as a silicon sink. Mar. Ecol. Prog. Ser. 441, 1–14. doi: 10.3354/meps09381
Conley, D. J., Stålnacke, P., Pitkänen, H., and Wilander, A. (2000). The transport and retention of dissolved silicate by rivers in Sweden and Finland. Limnol. Oceanogr. 45, 1850–1853. doi: 10.4319/lo.2000.45.8.1850
Conway, K. W., Krautter, M., Barrie, J. V., and Neuweiler, M. (2001). Hexactinellid sponge reefs on the Canadian continental shelf: a unique “living fossil.” Geosci. Can. 28, 71–78. Available online at: https://journals.lib.unb.ca/index.php/GC/article/view/4076
Conway, K. W., Krautter, M., Barrie, J. V., Whitney, F., Thomson, R. E., Reiswig, H., et al. (2005). Sponge Reefs in the Queen Charlotte Basin, Canada: Controls on Distribution, Growth and Development (Berlin: Springer-Verlag Berlin), 605–621.
Corsetti, F. A., Ritterbush, K. A., Bottjer, D. J., Greene, S., Ibarra, Y., Yager, J. A., et al. (2015). Investigating the paleoecological consequences of supercontinent breakup: sponges clean up in the early Jurassic. Sediment. Rec. 13, 4–10. Available online at: https://www.sepm.org/CM_Files/SedimentaryRecord/SedRecord13-2%235.pdf
De Laubenfels, M. W. (1936). A comparison of the shallow-water sponges near the Pacific end of the Panama Canal with those at the Caribbean end. Proc. U.S. Natl. Mus. 83, 441–464. doi: 10.5479/si.00963801.83-2993.441
Downey, R. V., Griffiths, H. J., Linse, K., and Janussen, D. (2012). Diversity and distribution patterns in high southern latitude sponges. PLoS ONE 7:e41672. doi: 10.1371/journal.pone.0041672
Downey, R. V., and Janussen, D. (2015). New insights into the abyssal sponge fauna of the Kurile–Kamchatka plain and trench region (Northwest Pacific). Deep Sea Res. II Top. Stud. Oceanogr. 111, 34–43. doi: 10.1016/j.dsr2.2014.08.010
Duplessis, K., and Reiswig, H. (2000). Description of a new deep-water calcareous sponge (Porifera: Calcarea) from Northern California. Pac. Sci. 54, 10–14. Available online at: http://hdl.handle.net/10125/1593
Ehrlich, H., Simon, P., Carrillo-Cabrera, W., Bazhenov, V. V., Botting, J. P., Ilan, M., et al. (2010). Insights into chemistry of biological materials: newly discovered silica-aragonite-chitin biocomposites in demosponges. Chem. Mat. 22, 1462–1471. doi: 10.1021/cm9026607
Finks, R. M., and Keith Rigby, J. (2003). “Geographic and stratigraphic distribution,” in Treatise on Invertebrate Paleontology. Part E (Revised), Porifera, Vol. 2, ed R. L. Kaesler (Boulder, CO; Lawrence, KS: The Geological Society of America & The University of Kansas), 275–296.
Fontorbe, G., Frings, P., De La Rocha, C. L., Hendry, K. R., and Conley, D. J. (2016). A silicon depleted North Atlantic since the Palaeogene: evidence from sponge and radiolarian silicon isotopes. Earth Planet. Sci. Lett. 453, 67–77. doi: 10.1016/j.epsl.2016.08.006
Fontorbe, G., Frings, P. J., De La Rocha, C. L., Hendry, K. R., Carstensen, J., and Conley, D. J. (2017). Enrichment of dissolved silica in the deep equatorial Pacific during the Eocene-Oligocene. Paleoceanography 32, 848–863. doi: 10.1002/2017PA003090
Frings, P. J., Clymans, W., Fontorbe, G., De La Rocha, C. L., and Conley, D. J. (2016). The continental Si cycle and its impact on the ocean Si isotope budget. Chem. Geol. 425, 12–36. doi: 10.1016/j.chemgeo.2016.01.020
Gammon, P. R., James, N. P., and Pisera, A. (2000). Eocene spiculites and spongolites in southwestern Australia: Not deep, not polar, but shallow and warm. Geology 28, 855–858. doi: 10.1130/0091-7613(2000)28<855:ESASIS>2.0.CO;2
Gold, D. A., Grabenstatter, J., de Mendoza, A., Riesgo, A., Ruiz-Trillo, I., and Summons, R. E. (2016). Sterol and genomic analyses validate the sponge biomarker hypothesis. Proc. Natl. Acad. Sci. U.S.A. 113, 2684–2689. doi: 10.1073/pnas.1512614113
Gouretski, V. V., and Koltermann, K. P. (2004). WOCE Global Hydrographic Climatology. 35/2004. Berichte des Bundesamtes fur Seeschifffahrt und Hydrographie.
Hartman, W. D. (1958). Natural history of the marine sponges of southern new England. Peabody Museum Nat. Hist. 12, 1–155.
Hendry, K. R., Georg, R. B., Rickaby, R. E. M., Robinson, L. F., and Halliday, A. N. (2010). Deep ocean nutrients during the last glacial maximum deduced from sponge silicon isotopic compositions. Earth Planet. Sci. Lett. 292, 290–300. doi: 10.1016/j.epsl.2010.02.005
Hendry, K. R., Leng, M. J., Robinson, L. F., Sloane, H. J., Blusztjan, J., Rickaby, R. E. M., et al. (2011). Silicon isotopes in Antarctic sponges: an interlaboratory comparison. Antarct. Sci. 23, 34–42. doi: 10.1017/S0954102010000593
Hendry, K. R., and Robinson, L. F. (2012). The relationship between silicon isotope fractionation in sponges and silicic acid concentration: modern and core-top studies of biogenic opal. Geochim. Cosmochim. Acta 81, 1–12. doi: 10.1016/j.gca.2011.12.010
Hestetun, J. T., Fourt, M., Vacelet, J., Boury-Esnault, N., and Rapp, H. T. (2015). Cladorhizidae (Porifera, Demospongiae, Poecilosclerida) of the deep Atlantic collected during Ifremer cruises, with a biogeographic overview of the Atlantic species. J. Mar. Biol. Assoc. U.K. 95, 1311–1342. doi: 10.1017/S0025315413001100
Hooper, J. N. A., and Lévi, C. (1994). “Biogeography of Indo-west Pacific sponges: microcionidae, raspailiidae, axinellidaem,” in Sponges in Time and Space, eds R. W. M. Van Soest, T. M. G. Van Kempen, and J. C. Braekman (Rotterdam: Balkema), 191–212.
Hooper, J. N. A., and Van Soest, R. W. M. (eds.). (2002). Systema Porifera: A Guide to the Classification of Sponges. New York, NY: Kluwer Academic/Plenum Publishers.
Howell, K.-L., Piechaud, N., Downie, A. -L., and Kenny, A. (2016). The distribution of deep-sea sponge aggregations in the North Atlantic and implications for their effective spatial management. Deep Sea Res. I Oceanogr. Res. Pap. 115(Suppl. C), 309–320. doi: 10.1016/j.dsr.2016.07.005
Huang, Z., Brooke, B., and Li, J. (2011). Performance of predictive models in marine benthic environments based on predictions of sponge distribution on the Australian continental shelf. Ecol. Inform. 6, 205–216. doi: 10.1016/j.ecoinf.2011.01.001
Jørgensen, C. B. (1944). On the Spicule Formation of Spongilla lacustris (L.): The Dependence of the Spicule-Formation on the Content of Dissolved and Solid Silicic Acid of the Milieu. København: E. Munksgaard (Bianco Lunos Bogtrykkeri).
Juggins, S. (2013). Quantitative reconstructions in palaeolimnology: new paradigm or sick science? Quat. Sci. Rev. 64, 20–32. doi: 10.1016/j.quascirev.2012.12.014
Kidder, D. L., and Tomescu, I. (2016). Biogenic chert and the Ordovician silica cycle. Palaeogeogr. Palaeoclimatol. Palaeoecol. 458, 29–38. doi: 10.1016/j.palaeo.2015.10.013
Klitgaard, A. B., and Tendal, O. S. (2004). Distribution and species composition of mass occurrences of large-sized sponges in the northeast Atlantic. Prog. Oceanogr. 61, 57–98. doi: 10.1016/j.pocean.2004.06.002
Kratz, T. K., Frost, T. M., Elias, J. E., and Cook, R. B. (1991). Reconstruction of a regional, 12,000-yr silica decline in lakes by means of fossil sponge spicules. Limnol. Oceanogr. 36, 1244–1249. doi: 10.4319/lo.1991.36.6.1244
Leys, S. P., Wilson, K., Holeton, C., Reiswig, H. M., Austin, W. C., and Tunnicliffe, V. (2004). Patterns of glass sponge (Porifera, Hexactinellida) distribution in coastal waters of British Columbia, Canada. Mar. Ecol. Prog. Ser. 283, 133–149. doi: 10.3354/meps283133
Love, G. D., Grosjean, E., Stalvies, C., Fike, D. A., Grotzinger, J. P., Bradley, A. S., et al. (2009). Fossil steroids record the appearance of demospongiae during the cryogenian period. Nature 457, 718–721. doi: 10.1038/nature07673
Lytle, D. E., and Wahl, E. R. (2005). Palaeoenvironmental reconstructions using the modern analogue technique: the effects of sample size and decision rules. Holocene 15, 554–566. doi: 10.1191/0959683605hl830rp
Maldonado, M. (2009). Embryonic development of verongid demosponges supports the independent acquisition of spongin skeletons as an alternative to the siliceous skeleton of sponges. Biol. J. Linn. Soc. 97, 427–447. doi: 10.1111/j.1095-8312.2009.01202.x
Maldonado, M. (2014). “Metazoans,” in The Tree of Life. Systematics and Evolution of the Living Organisms, eds V. Pablo and R. Zardoya (Sunderland, MA: Sinauer), 182–205.
Maldonado, M., Aguilar, R., Bannister, R. J., Bell, J. J., Conway, K. W., Dayton, P. K., et al. (2015a). “Sponge grounds as key marine habitats: a synthetic review of types, structure, functional roles, and conservation concerns,” in Marine Animal Forests: The Ecology of Benthic Biodiversity Hotspots, eds S. Rossi, L. Bramanti, A. Gori, and C. Orejas Saco del Valle (Cham: Springer International Publishing), 1–39.
Maldonado, M., Aguilar, R., Blanco, J., García, S., Serrano, A., and Punzón, A. (2015b). Aggregated clumps of lithistid sponges: a singular, reef-like bathyal habitat with relevant paleontological connections. PLoS ONE 10:e0125378. doi: 10.1371/journal.pone.0125378
Maldonado, M., Carmona, M. C., Uriz, M. J., and Cruzado, A. (1999). Decline in Mesozoic reef-building sponges explained by silicon limitation. Nature 401, 785–788. doi: 10.1038/44560
Maldonado, M., Carmona, M. C., Velásquez, Z., Puig, A., Cruzado, A., López, A., et al. (2005). Siliceous sponges as a silicon sink: an overlooked aspect of benthopelagic coupling in the marine silicon cycle. Limnol. Oceanogr. 50, 799–809. doi: 10.4319/lo.2005.50.3.0799
Maldonado, M., Navarro, L., Grasa, A., Gonzalez, A., and Vaquerizo, I. (2011). Silicon uptake by sponges: a twist to understanding nutrient cycling on continental margins. Sci. Rep. 1:30. doi: 10.1038/srep00030
Maldonado, M., Riesgo, A., Bucci, A., and Tzlerb, K. R. (2010). Revisiting silicon budgets at a tropical continental shelf: silica standing stocks in sponges surpass those in diatoms. Limnol. Oceanogr. 55, 2001–2010. doi: 10.4319/lo.2010.55.5.2001
Maldonado, M., and Young, C. M. (1998). Limits on the bathymetric distribution of keratose sponges: a field test in deep water. Mar. Ecol. Prog. Ser. 174, 123–139. doi: 10.3354/meps174123
Maliva, R. G., Knoll, A. H., and Siever, R. (1989). Secular change in chert distribution: a reflection of evolving biological participation in the silica cycle. Palaios 4, 519–532. doi: 10.2307/3514743
Marron, A. O., Ratcliffe, S., Wheeler, G. L., Goldstein, R. E., King, N., Not, F., et al. (2016). The evolution of silicon transport in Eukaryotes. Mol. Biol. Evol. 33, 3226–3248. doi: 10.1093/molbev/msw209
Mercurio, M., Corriero, G., Scalera Liaci, L., and Gaino, E. (2000). Silica content and spicule size variations in Pellina semitubulosa (Porifera: Demospongiae). Mar. Biol. 137, 87–92. doi: 10.1007/s002270000336
Morrow, C., and Cárdenas, P. (2015). Proposal for a revised classification of the Demospongiae (Porifera). Front. Zool. 12:7. doi: 10.1186/s12983-015-0099-8
Müller, W. E. G., Krasko, A., Le Pennec, G., and Schröder, H. C. (2003). Biochemistry and cell biology of silica formation in sponges. Microsc. Res. Tech. 62, 368–377. doi: 10.1002/jemt.10402
Pawlik, J. R., McMurray, S. E., Erwin, P., and Zea, S. (2015). No evidence for food limitation of Caribbean reef sponges: reply to slattery and lesser. Mar. Ecol. Prog. Ser. 527, 281–284. doi: 10.3354/meps11308
Pisera, A. (1997). Upper jurassic siliceous sponges from the swabian Alb: taxonomy and paleoecology. Paleaeontologia Pol. 57, 1–216.
Pisera, A. (2006). Palaeontology of sponges—a review. Can. J. Zool. 84, 242–261. doi: 10.1139/z05-169
Pisera, A., and Lévi, C. (2002). “Liithistid' Demospongiae,” in Systema Porifera. A Guide to the Classification of Sponges, eds J. N. A. Hooper and R. W. M. Van Soest (New York, NY: Kluwer Academic/Plenum Publishers), 299–301.
Przeslawski, R., Alvarez, B., Battershill, C., and Smith, T. (2014). Sponge biodiversity and ecology of the Van Diemen rise and eastern Joseph Bonaparte Gulf, northern Australia. Hydrobiologia 730, 1–16. doi: 10.1007/s10750-013-1799-8
Przeslawski, R., Alvarez, B., Kool, J., Bridge, T., Caley, J., and Nichols, S. (2015). Implications of sponge biodiversity patterns for the management of a marine reserve in northern Australia. PLoS ONE 10:e0141813. doi: 10.1371/journal.pone.0141813
Przeslawski, R., Daniell, J., Anderson, T., Barrie, J. V., Heap, A., Hughes, M., et al. (2011). Seabed Habitats and Hazards of the Joseph Bonaparte Gulf and Timor Sea, Northern Australia. Geoscience Australia, Record 2011/40, 69.
Racca, J. M. J., Racca, R., Pienitz, R., and Prairie, Y. T. (2007). PaleoNet: new software for building, evaluating and applying neural network based transfer functions in paleoecology. J. Paleolimnol. 38, 467–472. doi: 10.1007/s10933-006-9082-x
Raff, A. R., Marshall, C. R., and Turbeville, J. M. (1994). Using DNA sequences to unravel the cambrian radiation of the animal phyla. Annu. Rev. Ecol. Syst. 25, 351–375. doi: 10.1146/annurev.es.25.110194.002031
Rapp, H. T., Janussen, D., and Tendal, O. S. (2011). Calcareous sponges from abyssal and bathyal depths in the Weddell Sea, Antarctica. Deep Sea Res. II Top. Stud. Oceanogr. 58, 58–67. doi: 10.1016/j.dsr2.2010.05.022
Reincke, T., and Barthel, D. (1997). Silica uptake kinetics of Halichondria panicea in Kiel Bight. Mar. Biol. 129, 591–593. doi: 10.1007/s002270050200
Ritterbush, K. A., Bottjer, D. J., Corsetti, F. A., and Rosas, S. (2014). New evidence on the role of siliceous sponges in ecology and sedimentary facies development in eastern panthalassa following the triassic–jurassic mass extinction. Palaios 29, 652–668. doi: 10.2110/palo.2013.121
Ritterbush, K. A., Rosas, S., Corsetti, F. A., Bottjer, D. J., and West, A. J. (2015). Andean sponges reveal long-term benthic ecosystem shifts following the end-Triassic mass extinction. Palaeogeogr. Palaeoclimatol. Palaeoecol. 420, 193–209. doi: 10.1016/j.palaeo.2014.12.002
Rützler, K., and Smith, K. P. (1993). The genus Terpios and new species in the “Lobiceps” complex. Sci. Mar. 57, 381–393.
Sarmiento, J. L., and Gruber, N. (2006). Ocean Biogeochemical Dynamics. Princeton, NJ: Princeton University Press.
Schönberg, C. H. L., and Barthel, D. (1997). Inorganic skeleton of the demosponge Halichondria panicea. seasonality in spicule production in the baltic sea. Mar. Biol. 130, 133–140. doi: 10.1007/s002270050232
Schröder, H. C., Perović-Ottstadt, S., Rothenberger, M., Wiens, M., Schwertner, H., Batel, R., et al. (2004). Silica transport in the demosponge Suberites domuncula: fluorescence emission analysis using the PDMPO probe and cloning of a potential transporter. Biochem. J. 381(Pt 3), 665–673. doi: 10.1042/BJ20040463
Siever, R. (1991). “Silica in the oceans: biological-geochemical interplay,” in Scientists on Gaia. Papers delivered at the American Geophysical Union's Annual Chapman Conference in March, 1988, eds S. H. Schneider and J. B. Penelope (Cambridge, MA: MIT Press), 287–295.
Sinka, K. J., and Atkinson, T. C. (1999). A mutual climatic range method for reconstructing palaeoclimate from plant remains. J. Geol. Soc. Lond. 156, 381–396. doi: 10.1144/gsjgs.156.2.0381
Slattery, M., and Lesser, M. P. (2015). Trophic ecology of sponges from shallow to mesophotic depths (3 to 150 m): comment on Pawlik et al. (2015). Mar. Ecol. Prog. Ser. 527, 275–279. doi: 10.3354/meps11307
Spalding, M. D., Fox, H. E., Allen, G. R., Davidson, N., Ferdaña, Z. A., Finlayson, M., et al. (2007). Marine ecoregions of the world: a bioregionalization of coastal and shelf areas. Bioscience 57, 573–583. doi: 10.1641/B570707
Stone, A. R. (1970). Seasonal variations of spicule size in hymeniacidon perleve. J. Mar. Biol. Assoc. U.K. 50, 343–348. doi: 10.1017/S0025315400004562
Telford, R. J., and Birks, H. J. B. (2005). The secret assumption of transfer functions: problems with spatial autocorrelation in evaluating model performance. Q. Sci. Rev. 24, 2173–2179. doi: 10.1016/j.quascirev.2005.05.001
Uriz, M. J., Turon, X., Becerro, M., and Agell, G. (2003). Siliceous spicules and skeleton frameworks in sponges: origin, diversity, ultrastructural patterns, and biological functions. Microsc. Res. Tech. 62, 279–299. doi: 10.1002/jemt.10395
Vacelet, J. (1988). Indications de profounderur données par les Spongiaires dans les milieux benthiques actuels. Géologie Méditerranéenne 15, 13–26.
Valisano, L., Pozzolini, M., Giovine, M., and Cerrano, C. (2012). Biosilica deposition in the marine sponge Petrosia ficiformis (Poiret, 1789): the model of primmorphs reveals time dependence of spiculogenesis. Hydrobiologia 687, 259–273. doi: 10.1007/s10750-011-0987-7
Van Soest, R. W. M. (1994). “Demosponge distribution patterns,” in Sponges in Time and Space, eds R. W. M. Van Soest, T. M. G. Van Kempen, and J. C. Braekman (Rotterdam: Balkema), 213–223.
Van Soest, R. W. M., Boury-Esnault, N., Hooper, J., Rützler, K., de Voogd, N. J., Alvarez, B., et al. (2017). World Porifera Database. Available online at: http://www.marinespecies.org/porifera. Last consulted on (Accessed Mar 23, 2017).
Van Soest, R. W. M., Boury-Esnault, N., Vacelet, J., Dohrmann, M., Erpenbeck, D., De Voogd, N. J., et al. (2012). Global diversity of sponges (Porifera). PLoS ONE 7:e35105. doi: 10.1371/journal.pone.0035105
Wang, X., Schloßmacher, U., Wiens, M., Batel, R., Schröder, H. C., and Müller, W. E. G. (2012a). Silicateins, silicatein interactors and cellular interplay in sponge skeletogenesis: formation of glass fiber-like spicules. FEBS J. 279, 1721–1736. doi: 10.1111/j.1742-4658.2012.08533.x
Wang, X., Schroder, H. C., Wang, K., Kaandorp, J. A., and Muller, W. E. G. (2012b). Genetic, biological and structural hierarchies during sponge spicule formation: from soft sol-gels to solid 3D silica composite structures. Soft Matter 8, 9501–9518. doi: 10.1039/c2sm25889g
Wang, X., Wiens, M., Schröder, H. C., Jochum, K. P., Schloßmacher, U., Götz, H., et al. (2011a). Circumferential spicule growth by pericellular silica deposition in the hexactinellid sponge Monorhaphis chuni. J. Exp. Biol. 214, 2047–2056. doi: 10.1242/jeb.056275
Wang, X., Wiens, M., Schröder, H. C., Schloßmacher, U., Pisignano, D., Jochum, K. P., et al. (2011b). Evagination of cells controls bio-silica formation and maturation during spicule formation in sponges. PLoS ONE 6:e20523. doi: 10.1371/journal.pone.0020523
Whitney, F., Conway, K., Thomson, R., Barrie, V., Krautter, M., and Mungov, G. (2005). Oceanographic habitat of sponge reefs on the Western Canadian continental Shelf. Cont. Shelf Res. 25, 211–226. doi: 10.1016/j.csr.2004.09.003
Wille, M., Sutton, J., Ellwood, M. J., Sambridge, M., Maher, W., Eggins, S., et al. (2010). Silicon isotopic fractionation in marine sponges: a new model for understanding silicon isotopic variations in sponges. Earth Planet. Sci. Lett. 292, 281–289. doi: 10.1016/j.epsl.2010.01.036
Xiao, S., Hu, J., Yuan, X., Parsley, R. L., and Cao, R. (2005). Articulated sponges from the lower cambrian hetang formation in southern Anhui, South China: their age and implications for the early evolution of sponges. Palaeogeogr. Palaeoclimatol. Palaeoecol. 220, 89–117. doi: 10.1016/j.palaeo.2002.02.001
Yin, Z., Zhu, M., Davidson, E. H., Bottjer, D. J., Zhao, F., and Tafforeau, P. (2015). Sponge grade body fossil with cellular resolution dating 60 Myr before the Cambrian. Proc. Natl. Acad. Sci. U.S.A. 112, E1453–E1460. doi: 10.1073/pnas.1414577112
Yourassowsky, C., and Rasmont, R. (1984). The differentiation of sclerocytes in fresh-water sponges grown in a silica-poor medium. Differentiation 25, 5–9. doi: 10.1111/j.1432-0436.1984.tb01330.x
Keywords: sponge assemblages, palaeoecological indicators, spatial distribution, dissolved silica gradient, depth gradient, Si cycle
Citation: Alvarez B, Frings PJ, Clymans W, Fontorbe G and Conley DJ (2017) Assessing the Potential of Sponges (Porifera) as Indicators of Ocean Dissolved Si Concentrations. Front. Mar. Sci. 4:373. doi: 10.3389/fmars.2017.00373
Received: 12 April 2017; Accepted: 07 November 2017;
Published: 30 November 2017.
Edited by:
Brivaela Moriceau, Centre National de la Recherche Scientifique (CNRS), FranceReviewed by:
Sönke Hohn, Leibniz Centre for Tropical Marine Research (LG), GermanyPaco Cardenas, Uppsala University, Sweden
Copyright © 2017 Alvarez, Frings, Clymans, Fontorbe and Conley. This is an open-access article distributed under the terms of the Creative Commons Attribution License (CC BY). The use, distribution or reproduction in other forums is permitted, provided the original author(s) or licensor are credited and that the original publication in this journal is cited, in accordance with accepted academic practice. No use, distribution or reproduction is permitted which does not comply with these terms.
*Correspondence: Belinda Alvarez, YmVsaW5kYS5hbHZhcmV6QGdlb2wubHUuc2U=
†Present Address: Patrick J. Frings, Department of Geoscience, Swedish Museum of Natural History, Stockholm, Sweden and Earth Surface Geochemistry, GFZ German Research Centre for Geosciences, Potsdam, Germany
Wim Clymans, Earthwatch Institute (Europe), Oxford, United Kingdom