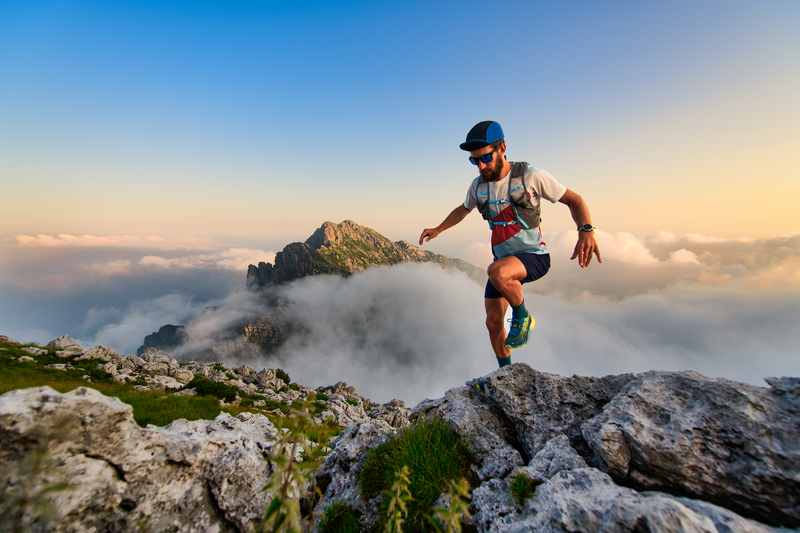
95% of researchers rate our articles as excellent or good
Learn more about the work of our research integrity team to safeguard the quality of each article we publish.
Find out more
ORIGINAL RESEARCH article
Front. Mar. Sci. , 16 November 2017
Sec. Marine Evolutionary Biology, Biogeography and Species Diversity
Volume 4 - 2017 | https://doi.org/10.3389/fmars.2017.00356
This article is part of the Research Topic Antarctic Biology: Scale Matters View all 21 articles
Recent genetic investigations have uncovered a high proportion of cryptic species within Antarctic polychaetes. It is likely that these evolved in isolation during periods of glaciation, and it is possible that cryptic populations would have remained geographically restricted from one another occupying different regions of Antarctica. By analysing the distributions of nine morphospecies, (six of which contained potential cryptic species), we find evidence for widespread distributions within the West Antarctic. Around 60% of the cryptic species exhibited sympatric distributions, and at least one cryptic clade was found to be widespread. Additional DNA barcodes from GenBank and morphological records extended the observed range of three species studied here, and indicate potential circum-Antarctic traits. Particle tracking analyses were used to model theoretical dispersal ranges of pelagic larvae. Data from these models suggest that the observed species distributions inferred from genetic similarity could have been established and maintained through the regional oceanographic currents, including the Antarctic Circumpolar Current (ACC) and its coastal counter current. Improved understanding of the distribution of Antarctic fauna is essential for predicting the impacts of environmental change and determining management strategies for the region.
Enclosed by both the Antarctic Circumpolar Current (ACC) and frontal systems the Southern Ocean is often described as an isolated marine environment, Figure 1. These oceanographic features act as a physical barrier, which are thought to have prevented species movement into and out of the Southern Ocean. For this reason early predictions suggested that the majority of benthic fauna within Antarctic waters would be endemic to the Southern Ocean (Ekman, 1953; Hedgpeth, 1969). Endemism has since been observed in many major taxonomic groups based on species records from mostly morphological species identification (see reviews Dell, 1972; Arntz et al., 1997; Clarke and Johnston, 2003; Thorpe et al., 2007; De Broyer and Danis, 2011; Brandt et al., 2012; Kaiser et al., 2013). Current estimates of endemism, as reported in the recently published Southern Ocean Biogeographic Atlas, ranged from 50 to 97% between taxa (De Broyer et al., 2014).
Figure 1. The locations from which polychaetes were collected during the BIOPEARL and JR275 expeditions (black dots) as well as the locations of additonal GenBank sequences used in haplotype networks (gray dots). SR, Shag Rocks; SG, South Georgia; ST, Southern Thule; PB, Powel Basin; EI, Elephant Island; LI, Livingston Island; AS, Amundsen Sea; WS, Weddell Sea; RS, Ross Sea; RS_O, Ross Sea offshore. Position and direction of oceanographic currents including the Antarctic Circumpolar Current (ACC), East Wind Drift (counter current) (EWD) and the Weddell and Ross Sea Gyres. Position of the Polar Front (PF), the Southern Antarctic Circumpolar Current Front (SACCF), Subantarctic Front (SAF) and the Southern Subtropical Front (SSTF).
A further biogeographic pattern that has long been associated with Antarctic marine fauna is the circum-polarity or circum-Antarctic distributions (Arntz et al., 1997; Clarke and Johnston, 2003). These distributions could be a result of a combination of factors that could have a homogenizing effect on the faunal communities. The continuous coastline around the continent itself provides connectivity between the different seas around Antarctica. Furthermore, given the relatively uniform physical conditions across the continental shelf, individual settlement and survival is not restricted by their physiology, e.g., temperature tolerance (Arntz and Gallardo, 1994). Larval dispersal around the continent could be aided by oceanographic currents, including the ACC, its coastal counter current (also referred to as East Wind Drift), and the Weddell and Ross Sea gyres, Figure 1 (Orsi et al., 1993, 1995; Fahrbach et al., 1994; Linse et al., 2007).
Antarctic benthic fauna often have extended depth ranges and are considered to be eurybathic, i.e., capable of living in benthic habitats within both shallow and deep water. Some of the first suggestions for this were made by Dell (1972) and Knox and Lowry (1977) for various taxa including sponges, corals, polychaetes and molluscs. Broad depth distributions of Antarctic fauna are thought to be associated with the advance, and retreat of shelf ice during interglacial cycles (Brey et al., 1996). During periods of glacial expansion some “shelf fauna” may have been moved down slope into ice free habitats. In the following glacial retreat the now “slope fauna” could then recolonize shallower shelf areas thus evolving eurybathic distributions (Clarke and Johnston, 2003). This movement was possible due to similar physical conditions (e.g., temperature) on the shelf, slope and deep-sea floor, thus reducing the need for specific adaptations needed to survive in these environments (Clarke et al., 2009; Clarke and Crame, 2010).
Some of these generalized patterns have been challenged when it comes to the deep-sea benthos. Although the ACC and Polar Front may affect the movement of pelagic and shallow water species it may not be a barrier to the benthos (Clarke, 2003). Antarctic polychaetes seem to have some of the broadest geographical distribution ranges amongst the Antarctic benthic macrofaunal invertebrates (Schüller and Ebbe, 2007). During analysis of the ANtarctic benthic DEEP-sea biodiversity (ANDEEP) samples, it was noted that more than half of all polychaetes identified matched species found north of the Polar Front, 20% of which are also found in the northern hemisphere (Brandt et al., 2007). However, in recent works by Neal et al. (2017) depth was identified as the main factor structuring the polychaete communities within the Amundsen and Scotia Sea, contradicting the broad depth distributions often associated with Southern Ocean benthic marine fauna.
Large-scale sampling programs and species databases are providing valuable insight to the biogeography of many species, however, these analyses are predominantly based on morphological identifications. Furthermore, early identifications of Antarctic benthos reflect the species names on pre-existing monographs of, for example, European fauna. Given the abundance of undescribed deep-sea species, the number of specimens collected on large sampling programs and the potential for identifying features to be damaged on collection we use molecular taxonomy to validate the accuracy of biogeographic records. Schüller and Ebbe (2014), investigated all georeferenced Register of Antarctic Marine Species (RAMS) polychaetes within the Scientific Committee for Antarctic Research Marine Biodiversity Information Network (SCAR-MarBIN), concluding that while Southern Ocean polychaete taxonomy is improving with new species descriptions, there are still many “cosmopolitan” species which are most probably Antarctic species different to their Northern counterparts. This can be easily tested using DNA barcoding where comparative sequences exist (Brasier et al., 2016).
Our ability to define the geographic distribution, or biogeography, of species including how their distribution was established and is maintained, has also progressed with the development of DNA sequencing, phylogeography and population genetics (Riesgo et al., 2015). These methods allow us to visualize and, with sufficient sample numbers, calculate the level of population connectivity between known populations of species from different localities, which can be controlled by several interacting biological, physical and chemical factors. Since the application of genetics in Antarctic diversity and biogeographic studies many species presumed to be circum-Antarctic or cosmopolitan based on morphological analysis are actually comprised of more geographically restricted and separated cryptic clades. For example genetically distinct restricted populations of the isopod Betamorpha fusiformis (Raupach et al., 2007), the cephalopod Pareledone spp. (Allcock et al., 2011) and the crinoid Promachorinus kerguelensis (Wilson et al., 2007) have been identified by comparing mitochondrial DNA. In other cases, DNA barcoding has provided evidence for circum-Antarctic distributions, e.g., genetic homogeneity within benthic invertebrates has been recorded in the nemertean ribbon worm Parabolisa corrugatus (Thornhill et al., 2008), the two shrimp species Chorismus antarcticus and Nematocarcinus lanceopes (Raupach et al., 2010) and the pycnogonid Nymphon australe (Arango et al., 2011).
This study uses mitochondrial DNA barcoding data from one of the largest Antarctic polychaete barcoding projects to date (Brasier et al., 2016), to investigate the distributions of nine polychaete morphospecies previously considered to be cosmopolitan or circum-Antarctic. We combine our genetic analyses with particle tracking models to examine the directionality and distance of pelagic polychaete larvae and the potential to maintain genetic connectivity across widely distributed populations. Together with our knowledge of Southern Ocean glaciations and polychaete larval biology we aim to improve our understanding of the biological and physical factors influencing the distribution of polychaete species within the Southern Ocean. We hypothesize that although many morphospecies of polychaetes exhibit “characteristic” distributional patterns for Southern Ocean species including endemism, eurybathy and circum-Antarctic distributions (Griffiths et al., 2009; Brandt et al., 2012), their cryptic clades may be more restricted than their moprhospecies, i.e., cryptic clades are found within a single region of Antarctica, which may be an artifact of their evolution during glacial periods when populations were isolated from one another.
Polychaetes were collected from three locations within the Southern Ocean; the Scotia Arc containing six sampling sites and the Amundsen Sea and Weddell Sea both containing four sampling sites, Figure 1. All polychaetes were identified based on morphological characters, some individuals could not be identified to any currently described species and were considered new to science. These results are presented in Neal et al. (2017) and all voucher specimens were deposited in the Natural History Museum London, details can be found online using the Darwin Core Archive https://doi.org/10.5519/0068114. DNA was extracted and sequenced from nine polychaete morphospecies (211 specimens in all); for details on the phylogenetic analysis of these sequences including the identification of cryptic species see Brasier et al. (2016). Prior to DNA barcoding, a biogeographic distribution classification; cosmopolitan, circum-Antarctic or restricted, was allocated to each initial morphospecies analyzed (Table 1), where: “cosmopolitan” species are those that have been recorded throughout the majority of the world's oceans and both hemispheres; “cicrum-Antarctic” species are those that have been collected within different regions of the Southern Ocean and are considered to be widespread around the Antarctic and “restricted” species are those only recorded in one Antarctic region or location with no records within the Register of Antarctic Marine Species (RAMS) recorded or Basic Local Alignment Search Tool (BLAST) matches from other localities.
Table 1. Species for which the geographic distribution will be investigated including their primary identification based on morphological analysis alone (in Neal et al., 2017) and their secondary identification from combined DNA and morphological analysis (in Brasier et al., 2016).
These definitions are coherent with the most recent biogeographic review of Antarctic polychaetes, Schüller and Ebbe (2014), who considered species circum-Antarctic if there were georeferenced RAMS records from at least the Weddell Sea, West Antarctic Peninsula (WAP) or the Scotia Arc, and the Ross Sea or Eastern Antarctica. The presumed distributions in Table 1 were defined using taxonomic databases including the Encyclopedia of Life (EOL) and RAMS. For undetermined morphospecies, their distribution was deciphered by their presence within samples collected in the each of the regions as well as any BLAST matches with georeferenced sequence including larval matches within the Ross Sea (Heimeier et al., 2010; Gallego et al., 2014).
The genetic diversity of the DNA barcodes generated from the nine morphospecies was investigated in Brasier et al. (2016). Phylogenetic and distance analyses found evidence for twelve cryptic species, increasing the number of species in this study from nine to seventeen. In Brasier et al. (2016) clades were assigned MB# to distinguish species, including cryptic species, e.g., Scalibregma sp. MB1 and Scalibregma sp. MB2. Cryptic species were referred to as either “Genus cf species MB#,” “Genus sp. MB#” or if no genus could be assigned “Family sp. MB#.” The use of “cf” or “sp” was associated with the type locality of the original species and likelihood of the Antarctic specimens being the “true” species, for more details see Brasier et al. (2016). Using haplotype networks we have analyzed the distribution of each genetically identified species, morphological and cryptic, listed in Table 1.
As the recovery of 16S sequences was greater than COI in all morphospecies examined, this marker was used to construct georeferenced haplotype networks of the sequenced specimens to visualize species distributions and speculate biogeographic patterns. An exception was Hesionidae sp. A for which cryptic species were revealed in COI but not 16S. If sequence matches within the GenBANK database were found during phylogenetic analysis these were also included in the network (Table 2). To avoid problems with gaps, all sequences of the same species were trimmed in Mesquite (Version 2.75) to the same length (Table 1) following MAFFT (for 16S) or MUSCLE (for COI) sequence alignment in Geneious (R7). GPS coordinates were assigned to each sequence for its given sample location, and networks constructed using statistical parsimony (Templeton et al., 1992) and the TCS programme (Clement et al., 2000) in PopART for editing in CorelDRAWX8. For the number of sequences per species by site used in the georeferenced haplotype networks, please see Supplementary Material. Haplotype networks with depth referenced sequences were also created using the five depth bins; < 500 m, 500–1,000 m, 1,001–1,500 m, 1,501–2,000 m and >2,000 m. The depth bins were chosen based on the discrete depth horizons sampled during collection expeditions (for details see Griffiths et al., 2008 and Linse et al., 2013). Most sequenced specimens were obtained from depths of 500, 1,000, 1,500, or 2,000 m, some specimens were collected from 100 and 200 m and were included in the < 500 m depth bin and those at depths greater than 2,000 m were also binned together.
Table 2. Additional sequences included in the haplotype networks, including the MB species they matched, the gene sequenced, specimen locality, GenBANk names and accession number.
To gain an insight into the potential pathways of larval dispersal between the sampled localities a particle tracking model was employed (ARIANE; Blanke and Raynaud, 1997) to the output of a NEMO (Madec, 2008) ocean general circulation model (OGCM). Five day mean ocean current data obtained from the National Oceanography Centre, U.K., NEMO 1/12° OGCM (Duchez et al., 2014), for the period 2000-2009, was used to calculate the 3D trajectories of passive particles released from 17 sites from four Antarctic locations, Table 3. Within each model grid cell co-located with a sampled site, eight evenly distributed particles were released at every model depth level and at 5 day intervals for the period 2000-2009. These particles were then tracked for 1 year. No vertical or horizontal dispersion was added to the particle motion and no buoyancy terms were included, so the subsequent particle pathway is purely an advective one. A 75 km search radius or “trap” at each sample site location for the full water column was used to determine whether foreign particles passed close by and were therefore potentially connected to another sample site.
Table 3. The release sites used in particle tracking analysis by location with latitude and longitude position and the depth range in which particles were released.
Particle tracking analysis is relevant to polychaete reproductive modes because six of the eight families included in this study are considered to have pelagic larvae (Table 4), with five of these known to have feeding (plankotrophic) larvae. The reproductive traits of each species examined in this study were inferred from family level trait data available from the largest polychaete trait database Polytraits1. Species level reproductive traits are rare, especially for those that are the hardest to collect and identify, e.g., deep sea and Antarctic species. We know from genetic studies that three of our species, Hesionidae sp. (MB1), Aglaophamus trissophyllus and Laonice weddellia have pelagic larvae in Antarctic waters (Heimeier et al., 2010; Gallego et al., 2014).
Table 4. Reproductive traits of the 8 polychaete families containing species from which DNA barcodes were collected in this study.
As discussed in our interpretation several aspects of larval biology were not incorporated into this model including larval mortality and behavior. These factors can lead to increased rentention of larvae within their source location and thus it is possible that our observations of the distance and direction of dispersal are overestimated (e.g., Cowen et al., 2006; Levin, 2006). Additionally we have not examined the seasonal differences in the release of larvae. Although some studies have found evidence for seasonal reproductive cycles in benthic fauna, different larval stages have been observed within the water column through the year (Bowden et al., 2009; Sewell and Jury, 2011). In this study particle tracking analyses are used purely to gain insight into how the oceanography of the Southern Ocean may theoretically affect population connectivity by its directionality and distance of dispersal. Furthermore, we do not know enough about Antarctic polychaete larvae to estimate these behavioral or biological model constraints.
The distribution patterns of seventeen genetically-determined polychaete species are based on the observed distributions from our haplotype networks and GenBank comparisons. In this study we found no evidence for cosmopolitan species. The genetic difference between our Antarctic specimens of Glycera capitata and Scalibregma inflatum, both considered to be cosmopolitan, with publically available sequence data from their Northern representatives [which were closer to their type localities, Greenland (G. capitata) and Norway (S. inflatum)], deemed the Antarctic clades to be cryptic species and so are examined for widespread Antarctic distributions, Figure 2.
Figure 2. Georeferenced (color) and depth binned (grayscale) haplotype networks for (A) Glycera spp. and (B) Scalibregma spp. Networks were constructed using 16S sequences, numbers indicate the number of nucleotide differences between haplotypes. Circle size reflects the number of individuals with that haplotype, black dots indicate missing haplotypes and n, the number of individuals for each potential species. Colored circles/segments relate to collection site where SR, Shag Rocks; SG, South Georgia; ST, Southern Thule; PB, Powel Basin; EI, Elephant Island; LI, Livingston Island; AS, Amundsen Sea; WS, Weddell Sea, RS, Ross Sea, grayscale for depth binned networks.
The most common distribution observed was widespread Antarctic, with 71% of the species investigated recorded to have genetically similar specimens in at least two Antarctic locations. The most abundant were Laonice weddellia, Harmothoe fuligineum and Aricidea simplex, Figure 3. A. simplex was sequenced from all three Antarctic locations sampled. The distribution of L. weddellia was also extended to the Ross Sea by identical sequences to that of a larval specimen from the Ross Sea (Gallego et al., 2014). No specimens of H. fuligineum were identified from the Weddell Sea, but specimens were found within the Amundsen and Scotia Arc regions.
Figure 3. Georeferenced (color) and depth binned (grayscale) haplotype networks for (A) Laonice weddellia, (B) Aricidea simplex, (C) Aricidea cf belgicae spp., (D) Harmothoe fuligineum, and (E) Euphrosinella cf cirratoformis spp. Networks were constructed using 16S sequences, numbers indicate the number of nucleotide differences between haplotypes. Circle size reflects the number of individuals with that haplotype, black dots indicate missing haplotypes and n, the number of individuals for each potential species. Colored circles/segments relate to collection site where SR, Shag Rocks; SG, South Georgia; ST, Southern Thule; PB, Powel Basin; EI, Elephant Island; LI, Livingston Island; AS, Amundsen Sea; WS, Weddell Sea, RS, Ross Sea; grayscale for depth binned networks.
Most of the widespread Antarctic cryptic species were sequenced from two of the sampled locations, e.g., the Scotia Arc and Amundsen Sea [Glycera sp. (MB1) and (MB2), Scalibregma sp. (MB3)]. Aglaophamus sp. (MB2) was the only cryptic species sequenced from all three locations. Aglaophamus sp. (MB1) was only sequenced from specimens in the Scotia Arc but was recorded as widespread because sequenced specimens from the Scotia Arc matched larval sequences from the Ross Sea (Heimeier et al., 2010), Figure 4A. Further larval matches from the Ross Sea were found with Hesionidae sp. (MB2). In comparison to Hesionidae sp. (MB2) the distribution of Hesionidae sp. (MB1) was reduced with representation from the Scotia Arc (Southern Thule) and the Amundsen Sea only, Figure 4B.
Figure 4. Georeferenced (color) and depth binned (grayscale) haplotype networks for (A) Aglaophamus spp. and (B) Hesionidae spp. Networks were constructed using 16S (Aglaophamus spp.) and COI (Hesionidae spp.) sequences, numbers indicate the number of nucleotide differences between haplotypes. Circle size reflects the number of individuals with that haplotype, black dots indicate missing haplotypes and n, the number of individuals for each potential species. Colored circles/segments relate to collection site where SR, Shag Rocks; SG, South Georgia; ST, Southern Thule; PB, Powel Basin; EI, Elephant Island; LI, Livingston Island; AS, Amundsen Sea; WS, Weddell Sea; RS, Ross Sea, grayscale for depth binned networks.
Whilst both Scalibregma sp. (MB1) and Eurphrosinella cf cirratoformis (MB1) were collected from more than one Antarctic region, their cryptic counterparts Scalibregma sp. (MB2) and (MB3) and Eurphrosinella cf cirratoformis (MB2) were only sequenced from one location including; Southern Thule, Elephant Island and the Amundsen Sea respectively, Figure 2B. Scalibregma sp. (MB2) was only represented by a single individual but Scalibregma sp. (MB3) and Eurphrosinella cf cirratoformis (MB2) were represented by four and three individuals respectively. Although these clades appear more restricted compared to their cryptic counterparts they exist sympatrically within the same regions. The only cryptic species that appear to exist allopatrically are the three clades of the morphospecies Aricidea belgicae. Each species was sequenced from a single separate region, Aricidea cf belgicae (MB1) from the Amundsen Sea, Aricidea cf belgicae (MB2) from the Weddell Sea and Aricidea cf belgicae (MB3) from the Scotia Arc, Figure 3C.
The maximum depth range that could be recorded was 100–2,000 m. The greatest depth distribution recorded in this study was found in Laonice weddellia, which were collected at depths between 200 and 1,500 m. Aricidea simplex had a similar depth range but the deepest specimens were collected from 1,000 m, whilst Harmothoe fuligineum had the narrowest depth range for the non-cryptic species from 200 to 500 m. Figure 3.
Some patterns in the depth distributions of cryptic species were observed. For example Glycera sp. (MB1) was collected from 200 to 500 m and Glycera sp. (MB2) from 500 to 1,000 m depth, Figure 1A. Similar patterning was also observed between Hesionidae sp. (MB1) and Hesionidae sp. (MB2), Euphrosinella cf cirratoformis (MB1) and Euprhosinella cf cirratoformis (MB2) and, Aglaphamus cf trissophyllus (MB1) and Aglaophamus sp. (MB2), Figures 3, 4. Two of the cryptic species of Scalibregma, (MB1) and (MB3) exhibited the same depth distribution, 200–500 m, whilst the single representative of Scalibregma sp. (MB2) was only found at 1000 m, Figure 2B. For the allopatric cyprtic caldes of Aricidea belgicae, it was Aricidea cf belgicae (MB1) that exhibited the greatest depth distribution of 500–1,000 m, whilst Aricidea cf belgicae (MB2) and Aricidea cf belgicae (MB3) were only observed at 200 and 600 m respectively, Figure 3B.
Particles released within the Ross Sea shelf and slope sites were transported westward around the Antarctic continent in the counter current and thus away from the other sample locations, Figure 5. In contrast, particles released within the offshore site within the Ross Sea were carried eastward in the direction of the WAP but away from the continental slope in the ACC. Sites within the Amundsen Sea followed the same direction of dispersal to the west with those on the edge of the continental shelf (AS_BIO3 and AS_BIO6) transporting particles toward the Ross Sea sites, Figure 6 (AS_BIO3). Particles from release sites at the northern tip of the WAP (Elephant Island, Livingston Island and Powell Basin) were transported in both directions, but with greater dispersal westwards across the Scotia Sea reaching the Shag Rocks and South Georgia sites, Figure 7. Particles from Shag Rocks and South Georgia, were also transported east and northward being advected by the ACC. Particle release sites within the Weddell Sea were well connected by the Weddell Sea gyre however, the extent of dispersal varied between sites. For the inshore locations (WS_FTSE and WS_FSCE) particles were transported westward and up the WAP (Figure not shown). Whilst those released further offshore (WS_CS; Figure 8) reached further into the Scotia Sea and into the ACC, crossing though the Shag Rocks and South Georgia sites as well as those closer to the WAP: Powell Basin, Elephant Island and Livingston Island.
Figure 5. Binned particle trajectories released from the Ross Sea Shelf site (RS Sh; indicated by the white star) during the period 2000-2009. The mean 2°C isotherm at 100 m for this period is also plotted as a proxy indicator of the Polar Front (blue line). Values indicate the total number of particles passing through a hexbin over the sample period. For site abbreviations see Table 3.
Figure 6. Binned particle trajectories released from the Outer Amundsen Sea site (AS BIO3; indicated by the white star) during the period 2000-2009. The mean 2°C isotherm at 100 m for this period is also plotted as a proxy indicator of the Polar Front (blue line). Values indicate the total number of particles passing through a hexbin over the sample period. For site abbreviations see Table 3.
Figure 7. Binned particle trajectories released from the Livingston Island site (LI; indicated by the white star) during the period 2000-2009. The mean 2°C isotherm at 100 m for this period is also plotted as a proxy indicator of the Polar Front (blue line). Values indicate the total number of particles passing through a hexbin over the sample period. For site abbreviations see Table 3.
Figure 8. Binned particle trajectories released from the Weddell Sea Continental Slope site (WS CS; indicated by the white star) during the period 2000-2009. The mean 2°C isotherm at 100 m for this period is also plotted as a proxy indicator of the Polar Front (blue line). Values indicate the total number of particles passing through a hexbin over the sample period. For site abbreviations see Table 3.
Statistical analysis in Figures 9, 10 show the percentage of particles passing through a 75 km radius or “trap” surrounding each of the other sites, and their mean time and depth of arrival. As expected, particles traveling the greatest distances were found in the surface layers as a result of the stronger advection (indicated by the lighter shaded squares in Figures 9, 10 and the lower percentages). In general, connectivity within regions was greater than between regions, for example 60% of particles from Elephant Island passed within 75 km of Livingston Island. However, this connectivity was unidirectional as only < 1% of particles released from Livingston Island were observed in the vicinity of Elephant Island. The level of inter- and intra-regional connectivity is highly dependent on the release site location in relation to local current pathways, and to a lesser degree the physical distance between the release sites. For example, there appears to be no connectivity between AS_BIO6 and AS_BIO4/5, yet the Ross Sea sites receive particles from all Amundsen Sea sites. Southern Thule was the least connected site receiving particles < 1% of particles from the Powell Basin site. Overall, the WS_CS had the highest connectivity to sites outside of its region with particles reaching all sites within the Scotia Arc.
Figure 9. Site connectivity matrix. The values in the matrix indicate the percentage of particles that pass between two of the sample sites (origin: y-axis, destination: x-axis). The color shading indicates the mean transit time of the particles from point of origin to the site in question. The gray shading indicates that there is no connectivity (within 360 days) between two sites. For site abbreviations please see Table 3.
Figure 10. Site connectivity matrix. The values in the matrix indicate the percentage of particles that pass between two of the sample sites (origin: y-axis, destination: x-axis). The color shading indicates the mean depth at which the particles arrive at the site in question. The gray shading indicates that there is no connectivity (within 360 days) between two sites. For site abbreviations please see Table 3.
In this study, nine of the seventeen genetically-identified species revealed different biogeographic patterns to their distributions inferred from morphology based identification records of the initial morphospecies and the RAMS database (Table 1). These nine species, have been described as cryptic species in Brasier et al. (2016), and their haplotype networks confirmed this displaying characteristics for cryptic speciation as described in Allcock and Strugnell (2012). In some cases, e.g., Aricidea cf belgicae (MB1), (MB2), and (MB3), more restricted distributions were observed but most remained widespread and potentially cicrum-Antarctic. Additionally, the networks showed the majority of cryptic species exhibited sympatric distributions, occupying the same geographic locations.
Matching haplotypes between individuals of the same species from at least two of the sampled regions as well as matches with larval DNA from the Ross Sea (Heimeier et al., 2010; Gallego et al., 2014) suggests an abundance of widespread polychaete species. Our results suggest that the three morphospeices, Laonice weddellia, Harmothoe fuligineum, and Aricidea simplex, are widespread within the West Antarctic, with potentially circum-Antarctic distributions. Similar results were also found for the Dorvilleidae polychaete Ophryotrocha orensanzi by comparing COI data from west and east Antarctic populations (Paxton et al., 2016). Eight of the cryptic species sequenced in this study appear to be widespread occurring in multiple regions within the West Antarctic. The matching haplotypes between Antarctic regions may indicate continued dispersal and ongoing gene flow between these regions that maintain genetic similarity (Arango et al., 2011; Soler-Membrives et al., 2017). These results are supported by the model findings, documenting the potential for oceanographic currents to carry particles or “larvae” between Antarctic regions in both directions around the continent.
The majority of polychaete cryptic species studied exist sympatrically, covering the same or overlapping regions of the West Antarctic. How these species and their current distributions were established and maintained is most probably a result of several complex biophysical interactions over geological time. Their evolution and distribution is likely to be influenced by the relative roles of vicariance and dispersal (Clarke, 1992; Aronson et al., 2007; Waters, 2008; González-Wevar et al., 2011). Although many records of cryptic species in the Antarctic are restricted to certain locations, widespread cryptic species have been recorded in several taxa. Examples of which include: the crinoid Promachocrinus kerguelensis, where six genetically distinct phylogroups were considered circumpolar, sympatric and eurybathic (Hemery et al., 2012); the brittle star Ophionotus victoriae, and the amphipod Nymphon australe, where although some clades appear restricted others were considered widespread (Galaska et al., 2017; Soler-Membrives et al., 2017). Within the literature similar insights into larval dispersal have also been obtained from oceanographic observations in conjunction with genetic analysis. For example Matschiner et al. (2009) concluded that the lack of genetic structuring between populations of the notothenioid, Gobionotothen gibberifrons, throughout the Scotia Sea could be assigned to the passive transport of their larvae during the pelagic development phase by the ACC as indicated by surface drifter trajectories. The geographic distribution of marine taxa within the Southern Ocean reflects the species life-history traits (including bathymetric ranges, developmental modes and larval lifespans) and the influence of circum-Antarctic current systems (Young et al., 2015; González-Wevar et al., 2017; Moreau et al., 2017).
It is generally considered that the majority of cryptic species within Antarctic waters arose from physically separated populations over multiple glaciations (Allcock et al., 2001; Thatje et al., 2005; Galaska et al., 2017). Thus, the existence of sympatric species could suggest they evolved within the same area due to another method of isolation, for example differences in reproductive traits (Palumbi, 1994), responses to competition (Alizon et al., 2008) or predation (Wilson et al., 2013). An alternative explanation is that these species did evolve in geographic isolation and their widespread distribution was established after they evolved, possibly during post-glacial re-colonization. Given the limited size and mobility of these benthic polychaetes it is unlikely that adult specimens migrate between the three regions sampled. Instead, it is probable that genetic connectivity between adult populations is maintained by larval dispersal (Fraser et al., 2012).
The reproductive traits of polychaetes can vary on many taxonomic levels. For example within the family Polynoidae the brooding of eggs is considered to be relatively rare (Giangrande, 1997). However, Gambi et al. (2001) recorded brooding of eggs under dorsal elytra in three Antarctic Harmothoe species. Within Spionidae, different populations of Polydora species are known to have different PLDs and feeding modes, exhibiting adelphophagy, the production of both planktotophic and adelphophagic larvae (where unfertilized eggs are ingested by the developing larvae) (Blake, 1969; Mackay and Gibson, 1999). Other spionids such as Streblospio benedicti exhibit poecilogony, producing both planktotrophic and lecithotrophic larvae (Levin, 1984). Given the recorded variation in polychaete reproduction, the reproductive modes of the species studied may not reflect their generalized family level trait data (Table 4). However, many species may still have a larval dispersal phase (Blake and Arnofsky, 1999; Faulwetter et al., 2014). Even brooding species can still possess a dispersal phase as brooders can release pelagic planktotrophic larvae as indicated by egg size in Gambi et al. (2001). Thus, it is possible that the widespread distribution of polychaetes observed here, were established and are maintained by larval dispersal by circum-Antarctic oceanographic currents (Starmans et al., 1999).
Earlier studies of eurybathy suggested that the depth distributions of Antarctic polychaetes were comparable to that of European species. Thus, polychaetes did not confirm to the general “eurybathic” characteristics assigned to Antarctic taxa due to reduced physical changes with depth, which could influence physiology (Brey et al., 1996). In most oceans there is a noticeable change in faunal composition on the shelf break (Gage and Tyler, 1991). However, in Antarctica where the continental shelf is much deeper the change in species composition with depth occurs at about 2,000 m (Brandt et al., 2007). Sequenced specimens from the Amundsen Sea and Scotia Arc were collected from depths of 500–1,500 m and the additional samples from the Weddell Sea were collected between depths of 400–2,000 m. Compared to studies such as Brandt et al. (2007) the depth-range sampled may not be large enough to visualize any depth dependence in distribution patterns. Thus, for those species that appear eurybathic within this study may actually be a result of the limited depth range (up to 2,000 m). If deeper slope and abyssal communities were included observed depth related patterns may have been different. However, Neal et al. (2017) observed depth patterns within the range of this study. Neal et al. (2017) noted greatest similarity in polychaete community composition between 500 m stations on the inner and outer shelf of the Amundsen sea, when compared to the communities from the same station at 1,000 and 1,500 m depths.
In conjunction with their geographic distribution many cryptic species appear to exist sympatrically or have overlapping depth distributions. For example, Hesionidae sp. (MB1) was sampled from depths of 500 m or more, whereas Hesionidae sp. (MB2) was only collected at 500 m depth or shallower. It is possible that Hesionidae sp. (MB2) is more dominant at shallower depths, whilst Hesionidae sp. (MB1), is outcompeted. This interaction could then be reversed at the deeper sites and is potentially associated with functional differences between cryptic species. The true absence of species at certain depths, i.e., truly restricted species, are very difficult to confirm and in some cases are questioned by later studies. For example, Schüller (2011) described three cryptic clades of Glycera spp. from the Weddell Sea, two clades were thought to be restricted to 2,000 m. This is in contrast to this study, as one of the clades from Schüller (2011) matched the Glycera sp. (MB2) specimens that were sequenced from stations 500 m and shallower.
Previous studies uncovered restricted cryptic populations in species from several phyla. For example Held (2003) uncovered two cryptic clades of Ceratoserolis trilobitoides, one of which was found only on the WAP the other extending to the Weddell Sea. Furthermore, Linse et al. (2007) compared DNA sequences of the widely distributed bivalve Lissarca notorcadensis and uncovered four explicit haplotype groups within the Scotia Sea. Similar results have been recorded for the isopod Glyptonotus antarcticus (Held and Wägele, 2005) as well as the cephalopod genus Pareledone (Allcock et al., 2011). This high diversity has previously been assigned to the fragmented nature and limited accessibility of available habitats in this region favoring speciation by population fragmentation (Allcock et al., 2011), especially in species with limited dispersal capacities (Strugnell and Allcock, 2013). In comparison to pelagic species there is generally a higher level of genetic structuring in benthic invertebrates, in Rogers (2007) this was assigned to the lower dispersal capabilities of benthic species at both larval and adult life stages. Within this study some of the cryptic clades of presumed circum-Antarctic species were only found within a limited number of sampled stations within a single region. This was the case for Scalibregma sp. (MB2) and (MB3), and Euphrosinella cf cirratoformis (MB2) that were more restricted than their broadly distributed, potentially circum-Antarctic, sister cryptic clades. Most of the restricted clades listed above were limited to sites within the Scotia Arc, an area known for particularly high biodiversity within Antarctica (Linse et al., 2007; Allcock et al., 2011; Neal et al., 2017). However, we acknowledge that restricted species are difficult to define, as all these clades contained fewer individuals (< 4) this result could be an artifact of undersampling.
The three potential cryptic species of Aricidea cf. belgicae (MB1), (MB2), and (MB3) were each restricted to a single region; Scotia Arc (MB1), Amundsen Sea (MB2) and Weddell Sea (MB3). This is the only example within this study of allopatric cryptic species if their distribution evolved from geographically isolated populations and was maintained by limited dispersal capabilities. Again we do not know the reproductive mode of these clades and Paraonidae are known to undergo both direct and indirect development making it difficult to speculate their dispersal (Table 4). Furthermore, even broadcasting species may not be able to overcome geographic isolation (Galaska et al., 2017). As mentioned these “restricted” distsributions could again be an artifact of undersampling as clades (MB2) and (MB3) were only represented by two and one individuals respectively. Within undersampled environments it is difficult to determine the presence of both restricted and rare species (Smith et al., 2006). This is complicated further by the presence of cryptic diversity and thus the submission of DNA sequences to open access databases is extremely important in the future assessment of species biogeography.
Here we use the model results presented to speculate on the direction and potential distances of passive larval dispersal within the Southern Ocean from our sampled sites. Particles released in the Amundsen Sea were transported counter clockwise around the continent. This transport is likely to be due to the Antarctic coastal counter current. Topographically constrained by the Antarctic Slope Front (ASF) this westward current encircles the coastal margins of Antarctica (Thompson et al., 2009). The ASF is found consistently above or just offshore of the shelf break and the coastal counter current is found broadly over the continental shelf (Heywood et al., 1998). While the ACC is considered the dominant current in maintaining circumpolar connections, the coastal counter current has been shown to connect many regions of high krill density (Thorpe et al., 2007). In the Ross Sea, particles released on the shelf were tracked westward in the coastal counter current; whereas at the offshore location, the Ross Sea gyre and ACC advect the particles eastward. Releases on the Ross Sea slope appear to be influenced by both regimes, but with the majority of particles following the coastal counter current pathway. The ACC provides a pathway to connect regions in the opposite direction to the coastal counter current; particles released within the Scotia Arc transported eastward out into the Scotia Sea. This movement is likely to be driven by the ACC meandering as they are transported further off shelf (Young et al., 2015). Similar particle movements have been observed in Lagrangian tracking models used to estimate krill connectivity (Hofmann and Murphy, 2004; Piñones et al., 2013). Particle dispersal from the Weddell Sea sites is dictated by the cyclonic Weddell Sea gyre. Releases above the continental slope travel the farthest distance, with the majority of particles tracking eastwards once reaching the Scotia Arc. Sites closer to the coast sites within the Weddell Sea see a slower spreading toward the WAP, with particles advected both eastward toward the Scotia sites and westward in the coastal counter current.
The passive movement of particles around Antarctica within the PLD time frame indicates the possibility that larvae may be recruited into non-parent populations substantial distances from their origin. If larvae are able to settle, grow and reproduce in these locations this could maintain genetic connectivity and limit the potential for genetic differentiation between regions. Examples of source populations transporting larvae westward may include those within the Amundsen Sea supplying larvae to the Ross Sea and the Ross Sea shelf transporting larvae along the continental shelf. The Weddell Sea sites appear to be well connected and may provide larvae to sites along the WAP such as Livingston Island and Elephant Island, which in turn may supply larvae to northern sites within the Scotia Sea. However, it should be noted that given the observed depth ranges of these species (0–1,500 m) many of the particles advected over the deep open ocean may no longer be in suitable locations to establish populations, restricted by their physiology. If larvae are unable to disperse over unsuitable habitats this can prevent the degree of population connectivity (Rogers et al., 2006). This may be the case for dispersal from sites within the Scotia Arc, in which particles were transported north into the Scotia Sea. These sites included Shag Rocks and South Georgia but also Southern Thule from which no particles reached other locations. Thus, these sites may act as sink populations rather than source other regions.
The maintenance of widespread distributions by pelagic larval dispersal and recruitment may not applicable to all of the species, as we cannot confirm the presence of free-living larvae. Furthermore, Antarctic taxa in general are considered to lack free-living larval stages (Pearse et al., 1991, 2009) and the existence of circum-Antarctic brooding species is highly unlikely (Lörz et al., 2009). A potential explanation for the maintenance of genetic connectivity in brooding species or those lacking pelagic larvae includes the passive rafting of larvae or adults on floating substrate or ocean debris (Waters, 2008). Leese et al. (2010) suggested that this method maintained connected shallow water isolated populations of the isopod Septemserolis septemcarinata across the sub-Antarctic. Furthermore, direct evidence of rafting on kelp has been observed in the widespread sub-Antarctic brooding bivalve Gasimardia trapesina (Helmuth et al., 1994), the sea slug Onchidella marginata (Cumming et al., 2014) and two species of sub-Antarctic amphipods (Nikula et al., 2010). An additional factor that could influence population connectivity is anthropogenic transport (David and Loveday, 2017). We have limited knowledge of the extent or potential growth of this in the Southern Ocean however there is evidence of anthropogenic transport of non-native species into the Antarctic (Lee and Chown, 2007).
Given inherent difficulties of directly measuring dispersal when larvae are minute (~200 μm) compared to the potential scale of dispersal (~km) (Gilg and Hilbish, 2003) dispersal distance is more often estimated using coupled biophysical models. The model scenario used in this study was based limited biological traits data. Limited biological knowledge is considered to be the main challenge when attempting to predict and validate dispersal pathways and distance (Levin, 2006; Metaxas and Saunders, 2009; Hilário et al., 2015). In this study we tracked particles for a maximum of 1 year, however PLD can be highly variable, for example recorded PLD in polychaetes has ranged from 13 to 150 days for planktotrophic larvae and 1–25 days for lechitrophic larvae from California (Carson and Hentschel, 2006). Within the Southern Ocean region, the life histories of marine organisms are often much slower than similar temperate and tropical taxa (Pearse et al., 1991), for example the lifespan of Antarctic echinoderm larvae is thought to exceed 1 year at a temperature of −1.5°C (Shilling and Manahan, 1994; Marsh et al., 1999).
If the PLD of the species studied is less than 360 days, the sites reached by particles may be an overestimate of larval dispersal. Figure 9 presents the mean time of arrival of particles and can be used to estimate the distance of dispersal over shorter PLDs. With a shorter larval duration of 3–6 months (90–180 days in Figure 9) most particles will only reach locations within their region. For example most sites within the Weddell Sea would remain connected but particles would not reach the sites in the Scotia Arc. A shorter PLD would greatly increase particle retention within the site or region of release therefore reducing the potential connectivity between regions (e.g., Shanks, 2009; Faurby and Barber, 2012).
As well as PLD our knowledge of polychaete larval behavior is very limited. Matschiner et al. (2009) showed that many larvae are capable of active vertical migration, which could lead to the avoidance of advection and increased retention near their sight of dispersal (Swearer et al., 2002). Additionally, in our study, particles were only released from sites we sampled. The existence of populations in between our sites would be important if the PLD and dispersal distance is shorter than predicted. These in-between populations would provide additional sites for genetic mixing between populations and larval release, and so contribute to the maintenance of circum-Antarctic genetic connectivity.
This study showed that the previously accepted biogeographic patterns of a third of the nine morphospecies examined should be questioned or re-described. Widespread distributions within the West Antarctic were recorded in 12 of the 17 species. These included 9 cryptic species existing sympatrically. The presence of widespread morphological and cryptic species is likely to be explained by their larval dispersal between populations as demonstrated by particle tracking models. The lack of consistency between the biogeography of cryptic species, some being widespread and potentially circum-Antarctic, whilst others are restricted, recorded within this study demonstrates the complexity of Southern Ocean biogeography (Brandão et al., 2010; Strugnell and Allcock, 2013; Chown et al., 2015). Fine scale differences in species distributions may be a result of variable life histories, habitat preferences, biological responses and ecological interactions within and between species through past and present physical conditions rather than a lack of transport connectivity from oceanographic currents. To fully appreciate why some species may be more dominant at certain depths, e.g., Hesionidae spp., or some widespread, whilst others are restricted, e.g., Scalibregma spp., would require investigations into their ecological traits to understand their functional differences. This is important because species with restricted distributions or limited dispersal capacities are often considered more vulnerable to extinction or less likely to recover from physical disturbances, as disturbed or removed populations may not be resupplied if they do not inhabit neighboring sites (Chown et al., 2015).
Our results presented here have valuable implications, improving our understanding of the drivers of biogeography and their implications for marine management under changing environmental conditions. The use of multiple data sets such as diversity, biogeographic and genetic data together with ocean physics model data, are valuable tools for the designation of effective marine management practices such as Marine Protected Areas (MPAs) and fishing restrictions (Robinson et al., 2017). The effectiveness of an MPA is, in part, reliant on the ability of species within the MPA to source external populations, thus mapping the likely dispersal pathways and distance of known species provides biological evidence for designation (e.g., Le Quesne and Codling, 2009; Planes et al., 2009). With increased benthic sampling and DNA barcoding these data can be used to assess the level of genetic connectivity between different polychaete populations, assess the abundance of rare and restricted species and provide further insight into the processes that determine species distributions.
This paper includes the main finding of the second chapter of MB's PhD thesis. MB conducted all laboratory DNA data collection, led the analysis and wrote this manuscript. JH ran the particle tracking analyses, produced model figures and conducted statistical analyses on the particle data. HW significantly contributed to the acquisition, analysis and interpretation of the genetic data. RJ contributed to the conception of this project and manuscript editing. KL donated all of the specimens used in this project and assisted in the interpretation and discussion of the DNA data collected. HR contributed to hypothesis framing, model design making a substantial contribution to its application and contributed to the interpretation of results. AG led the polychaete sampling at sea of the BIOPEARLII samples, and project-managed the sorting and identification of all BIOPEARL polychaetes. AG also contributed to the conception of this project and manuscript editing. All authors contributed to the revised article and are accountable for all aspects of the work should the accuracy and integrity be questioned.
All DNA barcoding was supported by MB's PhD research training grant received from the University of Liverpool and the National Oceanography Centre. The expeditions JR144, JR179 (BIOPEARL I and II) and JR275 were part of the British Antarctic Survey core programmes “Global Science in the Antarctic Context” and “Polar Science for Planet Earth” funded by The Natural Environment Research Council.
The authors declare that the research was conducted in the absence of any commercial or financial relationships that could be construed as a potential conflict of interest.
We would like to thank the crew and scientists who participated in the three research cruises that collected the polychaete specimens used in this study. We are particularly grateful to the BIOPEARL sample sorters that worked at sea, in the NHM Deep Sea Lab, and the BAS Lab: Rebekah Baker, David Barnes, Stefanie Kaiser, Ondine Cornubert, Adam Reed, Michael Mende, Wencke Krings, Moritz Stäbler and Chester Sands. Adrian Glover acknowledges the Natural Environment Research Council Collaborative Gearing Scheme for funding to participate in JR179 research cruise. Model data analyzed in this study was generated using the ARCHER UK National Supercomputing Service (http://www.archer.ac.uk). Particle tracking analysis was performed on the NERC funded JASMIN super-data-cluster facility (http://www.jasmin.ac.uk). Additional thanks are due to Lenka Neal for her help with specimen identification prior to DNA analyses. We are also grateful for the constructive comments provided by both the reviewers and editorial team.
The Supplementary Material for this article can be found online at: https://www.frontiersin.org/articles/10.3389/fmars.2017.00356/full#supplementary-material
1. ^PolyTraits Team. Data from: Polytraits: A database on biological traits of polychaetes. Hellenic Centre for Marine Research: Lifewatch, Greece (2017) http://polytraits.lifewatchgreece.eu
Alizon, S., Kucera, M., and Jansen, V. A. (2008). Competition between cryptic species explains variations in rates of lineage evolution. Proc. Natl. Acad. Sci. U.S.A. 105, 12382–12386. doi: 10.1073/pnas.0805039105
Allcock, A. L., and Strugnell, J. M. (2012). Southern Ocean diversity: new paradigms from molecular ecology. Trends Ecol. Evol. (Amst). 27, 520–528. doi: 10.1016/j.tree.2012.05.009
Allcock, A. L., Barratt, I., Eléaume, M., Linse, K., Norman, M. D., Smith, P. J., et al. (2011). Cryptic speciation and the circumpolarity debate: a case study on endemic Southern Ocean octopuses using the COI barcode of life. Deep-Sea Res. Part. II. Top. Stud. Oceanogr. 58, 242–249. doi: 10.1016/j.dsr2.2010.05.016
Allcock, A. L., Piatkowski, U., Rodhouse, P. G., and Thorpe, J. P. (2001). A study on octopodids from the eastern Weddell Sea, Antarctica. Polar. Biol. 24, 832–838. doi: 10.1007/s003000100288
Arango, C. P., Soler-Membrives, A., and Miller, K. J. (2011). Genetic differentiation in the circum-Antarctic sea spider Nymphon australe (Pycnogonida; Nymphonidae). Deep-Sea Res. Part. II. Top. Stud. Oceanogr. 58, 212–219. doi: 10.1016/j.dsr2.2010.05.019
Arntz, W. E., and Gallardo, V. A. (1994). “Antarctic benthos: present position and future prospects,” in Antarctic Science, eds G. Hempel (Berlin; Heidelberg: Springer), 243–277.
Arntz, W. E., Gutt, J., and Klages, M. (1997). “Antarctic marine biodiversity: an overview,” in Antarctic Communities: Species, Structure and Survival, eds B. Battaglia, J. Valencia and D. W. H. Walton (Cambridge: Cambridge University Press), 3–14.
Aronson, R. B., Thatje, S., Clarke, A., Peck, L. S., Blake, D. B., Wilga, C. D., et al. (2007). Climate change and invasibility of the antarctic benthos. Annu. Rev. Ecol. Syst. 38, 129–154. doi: 10.1146/annurev.ecolsys.38.091206.095525
Beesley, P. L., Ross, G. J. B., and Glasby, C. J. (2000). Polychaetes and Allies: The Southern Synthesis. Melbourne, VIC: CSIRO Publishing.
Bhaud, M. (1988). The two planktonic larval periods of Lanice conchilega (Pallas, 1766) Annelida Polychaeta, a peculiar example of the irreversibility of evolution. Ophelia 29, 141–152. doi: 10.1080/00785326.1988.10430825
Blake, J. A. (1969). Reproduction and larval development of Polydora from Northern New England (Polychaeta: Spionidae). Ophelia 7, 1–63. doi: 10.1080/00785326.1969.10419288
Blake, J. A., and Arnofsky, P. L. (1999). Reproduction and larval development of the spioniform Polychaeta with application to systematics and phylogeny. Hydrobiologia 402, 57–106. doi: 10.1023/A:1003784324125
Blanke, B., and Raynaud, S. (1997). Kinematics of the Pacific equatorial undercurrent: an Eulerian and Lagrangian approach from GCM results. J. Phys. Oceanogr. 27, 1038–1053. doi: 10.1175/1520-0485(1997)027<1038:KOTPEU>2.0.CO;2
Bowden, D. A., Clarke, A., and Peck, L. S. (2009). Seasonal variation in the diversity and abundance of pelagic larvae of Antarctic marine invertebrates. Mar. Biol. 156, 2033–2047. doi: 10.1007/s00227-009-1235-9
Brandão, S. N., Sauer, J., and Schön, I. (2010). Circumantarctic distribution in Southern Ocean benthos? A genetic test using the genus Macroscapha (Crustacea, Ostracoda) as a model. Mol. Phylogenet. Evol. 55, 1055–1069. doi: 10.1016/j.ympev.2010.01.014
Brandt, A., De Broyer, C., Ebbe, B., Ellingsen, K. E., Goodat, A. J., Janussen, D., et al. (2012). “Southern ocean deep benthic biodiversity,” in Antarctic Ecosystems: An Extreme Environment in a Changing World, eds A. D. Rogers, N. M. Johnston, E. J. Murphy, and A. Clarke (Oxford: Blackwell Publishing Limited), 291–334.
Brandt, A., Gooday, A. J., Brandão, S. N., Brix, S., Brökeland, W., Cedhagen, T., et al. (2007). First insights into the biodiversity and biogeography of the Southern Ocean deep sea. Nature 447, 307–311. doi: 10.1038/nature05827
Brasier, M. J., Wiklund, H., Neal, L., Jeffreys, R., Linse, K., Ruhl, H., et al. (2016). DNA barcoding uncovers cryptic diversity in 50% of deep-sea Antarctic polychaetes. R. Soc. Open Sci. 3:160432. doi: 10.1098/rsos.160432
Brey, T., Dahm, C., Gorny, M., Klages, M., Stiller, M., and Arntz, W. (1996). Do Antarctic benthic invertebrates show an extended level of eurybathy? Antart. Sci. 8, 3–6. doi: 10.1017/S0954102096000028
Carson, H. S., and Hentschel, B. T. (2006). Estimating the dispersal potential of polychaete species in the Southern California Bight: implications for designing marine reserves. Mar. Ecol. Prog. Ser. 316, 105–113. doi: 10.3354/meps316105
Chown, S. L., Clarke, A., Fraser, C. I., Cary, S. C., Moon, K. L., and McGeoch, M. A. (2015). The changing form of Antarctic biodiversity. Nature 522, 431–438. doi: 10.1038/nature14505
Clarke, A. (2003). “The polar deep seas,” in Ecosystems of the Deep Ocean (Ecosystems of the World), ed P. A. Tyler (Elsevier Science), 241–260.
Clarke, A. (1992). “Temperature and evolution: southern ocean cooling and antarctica marine fauna,” in Antarctic Ecosystems: Ecological Change and Conservation, eds K. R. Kerry and G. Hempel (Berlin: Springer Verlag), 9–22.
Clarke, A., and Crame, J. A. (2010). Evolutionary dynamics at high latitudes: speciation and extinction in polar marine faunas. Philos. Trans. R. Soc. B. Biol. Sci. 365, 3655–3666. doi: 10.1098/rstb.2010.0270
Clarke, A., and Johnston, N. M. (2003). Antarctic marine benthic diversity. Oceanogr. Mar. Biol. Annu. Rev. 41, 47–114.
Clarke, A., Griffiths, H. J., Barnes, D. K., Meredith, M. P., and Grant, S. M. (2009). Spatial variation in seabed temperatures in the Southern Ocean: implications for benthic ecology and biogeography. J. Geophys. Res. Biogeosci. 114:G03003. doi: 10.1029/2008JG000886
Clement, M., Posada, D., and Crandall, K. A. (2000). TCS: a computer program to estimate gene genealogies. Mol. Ecol. 9, 1657–1659. doi: 10.1046/j.1365-294x.2000.01020.x
Cowen, R. K., Paris, C. B., and Srinivasan, A. (2006). Scaling of connectivity in marine populations. Science 311, 522–527. doi: 10.1126/science.1122039
Cumming, R., Nikula, R., Spencer, H., and Waters, J. (2014). Transoceanic genetic similarities of kelp-associated sea slug populations: long-distance dispersal via rafting? J. Biogeogr. 41, 2357–2370. doi: 10.1111/jbi.12376
David, A. A., and Loveday, B. R. (2017). The role of cryptic dispersal in shaping connectivity patterns of marine populations in a changing world. J. Mar. Biol. Assoc. U.K. doi: 10.1017/S0025315417000236. [Epub ahead of print].
De Broyer, C., and Danis, B. (2011). How many species in the Southern Ocean? Towards a dynamic inventory of the Antarctic marine species. Deep Sea Res. Part. II. Top. Stud. Oceanogr. 58, 5–17. doi: 10.1016/j.dsr2.2010.10.007
De Broyer, C., Koubbi, P., Griffiths, H. J., Raymond, B., d'Udekem d'Acoz, C., Van de Putte, A. P., et al. (2014). Biogeographic Atlas of the Southern Ocean. Cambridge, UK: SCAR.
Dell, R. K. (1972). “Antarctic benthos,” in Advances in Marine Biology, eds S. R. Frederick and Y. Maurice (London: Academic Press), 1–216.
Duchez, A., Frajka-Williams, E., Castro, N., Hirschi, J., and Coward, A. (2014). Seasonal to interannual variability in density around the Canary Islands and their influence on the Atlantic meridional overturning circulation at 26 N. J. Geophys. Res. Oceans 119, 1843–1860. doi: 10.1002/2013JC009416
Fahrbach, E., Rohardt, G., Schröder, M., and Strass, V. (1994). Transport and structure of the Weddell Gyre. Ann. Geophys. 12, 840–855. doi: 10.1007/s00585-994-0840-7
Fauchald, K. (1983). Life diagram patterns in benthic polychaetes. Proc. Biol. Soc. Wash. 96, 160–177.
Faulwetter, M. S., Markantonatou, M. V., Pavloudi, M. C., Papageorgiou, N., Keklikoglou, M. K., Chatzinikolaou, E., et al. (2014). Polytraits: a database on biological traits of marine polychaetes. Biodivers. Data J. 2:e1024. doi: 10.3897/BDJ.2.e1024
Faurby, S., and Barber, P. H. (2012). Theoretical limits to the correlation between pelagic larval duration and population genetic structure. Mol. Ecol. 21, 3419–3432. doi: 10.1111/j.1365-294X.2012.05609.x
Fraser, C. I., Nikula, R., Ruzzante, D. E., and Waters, J. M. (2012). Poleward bound: biological impacts of Southern Hemisphere glaciation. Trends Ecol. Evol. (Amst). 27, 462–471. doi: 10.1016/j.tree.2012.04.011
Gage, J. D., and Tyler, P. A. (1991). Deep-Sea Biology: A Natural History of Organisms at the Deep-Sea Floor. Cambridge: Cambridge University Press.
Galaska, M. P., Sands, C. J., Santos, S. R., Mahon, A. R., and Halanych, K. M. (2017). Geographic structure in the Southern Ocean circumpolar brittle star Ophionotus victoriae (Ophiuridae) revealed from mtDNA and single-nucleotide polymorphism data. Ecol. Evol. 7, 475–485. doi: 10.1002/ece3.2617
Gallego, R., Lavery, S., and Sewell, M. (2014). The meroplankton community of the oceanic Ross Sea during late summer. Antarct. Sci. 26, 345–360. doi: 10.1017/S0954102013000795
Gambi, M. C., Patti, F. P., Micaletto, G., and Giangrande, A. (2001). Diversity of reproductive features in some Antarctic polynoid and sabellid polychaetes, with a description of Demonax polarsterni sp. n. (Polychaeta, Sabellidae). Polar Biol. 24, 883–891. doi: 10.1007/s003000100287
Giangrande, A. (1997). Polychaete reproductive patterns, life cycles and life histories: an overview. Oceanogr. Mar. Biol. Annu. Rev. 35, 323–386.
Gilg, M. R., and Hilbish, T. J. (2003). The geography of marine larval dispersal: coupling genetics with fine-scale physical oceanography. Ecology 84, 2989–2998. doi: 10.1890/02-0498
González-Wevar, C. A., David, B., and Poulin, E. (2011). Phylogeography and demographic inference in Nacella (Patinigera) concinna (Strebel, 1908) in the western Antarctic Peninsula. Deep Sea Res. Part. II. Top. Stud. Oceanogr. 58, 220–229. doi: 10.1016/j.dsr2.2010.05.026
González-Wevar, C. A., Hüne, M., Segovia, N. I., Nakano, T., Spencer, H. G., Chown, S. L., et al. (2017). Following the Antarctic Circumpolar Current: patterns and processes in the biogeography of the limpet Nacella (Mollusca: Patellogastropoda) across the Southern Ocean. J. Biogeogr. 44, 861–874. doi: 10.1111/jbi.12908
Griffiths, H. J., Barnes, D. K., and Linse, K. (2009). Towards a generalized biogeography of the Southern Ocean benthos. J. Biogeogr. 36, 162–177. doi: 10.1111/j.1365-2699.2008.01979.x
Griffiths, H. J., Linse, K., and Barnes, D. K. (2008). Distribution of macrobenthic taxa across the Scotia Arc, Southern Ocean. Antarc. Sci. 20, 213–226. doi: 10.1017/S0954102008001168
Hedgpeth, J. W. (1969). “Distribution of selected groups of marine invertebrates in waters south of 35oS latitude,“ in Antarctic Map Folio Series, eds V. Bushnell and J. W. Hedgpeth (New York, NY: American Geographical Society), 1–9.
Heimeier, D., Lavery, S., and Sewell, M. A. (2010). Using DNA barcoding and phylogenetics to identify Antarctic invertebrate larvae: lessons from a large scale study. Mar. Genomics 3, 165–177. doi: 10.1016/j.margen.2010.09.004
Held, C. (2003). “Molecular evidence for cryptic speciation within the widespread Antarctic crustacean Ceratoserolis trilobitoides (Crustacea, Isopoda),” in Antarctic Biology in a Global Context, eds A. H. Huiskes, W. W. Gieskes, R. M. Rozema, S. M. Schorno, S. M. Van Der Vies, and W. J. Wolff (Leiden: Backhuys Publishers), 135–139.
Held, C., and Wägele, J.-W. (2005). Cryptic speciation in the giant Antarctic isopod Glyptonotus antarcticus (Isopoda: Valvifera: Chaetiliidae). Sci. Mar. 69, 175–181. doi: 10.3989/scimar.2005.69s2175
Helmuth, B., Veit, R., and Holberton, R. (1994). Long-distance dispersal of a subantarctic brooding bivalve (Gaimardia trapesina) by kelp-rafting. Mar. Biol. 120, 421–426. doi: 10.1007/BF00680216
Hemery, L., Eléaume, M., Roussel, V., Améziane, N., Gallut, C., Steinke, D., et al. (2012). Comprehensive sampling reveals circumpolarity and sympatry in seven mitochondrial lineages of the Southern Ocean crinoid species Promachocrinus kerguelensis (Echinodermata). Mol. Ecol. 21, 2502–2518. doi: 10.1111/j.1365-294X.2012.05512.x
Heywood, K. J., Locarnini, R. A., Frew, R. D., Dennis, P. F., and King, B. A. (1998). “Transport and water masses of the Antarctic Slope Front system in the eastern Weddell Sea,” in Ocean, Ice, and Atmosphere: Interactions at the Antarctic Continental Margin, Antarct. Res. Ser., eds S. S. Jacobs and R. F. Weiss (Washington, DC: American Geophysical Union), 203–214.
Hilário, A., Metaxas, A., Gaudron, S. M., Howell, K. L., Mercier, A., Mestre, N. C., et al. (2015). Estimating dispersal distance in the deep sea: challenges and applications to marine reserves. Front. Mar. Sci. 2:6. doi: 10.3389/fmars.2015.00006
Hofmann, E. E., and Murphy, E. J. (2004). Advection, krill, and Antarctic marine ecosystems. Antarct. Sci. 16, 487–499. doi: 10.1017/S0954102004002275
Kaiser, S., Brandão, S. N., Brix, S., Barnes, D. K., Bowden, D. A., Ingels, J., et al. (2013). Patterns, processes and vulnerability of Southern Ocean benthos: a decadal leap in knowledge and understanding. Mar. Biol. 160, 2295–2317. doi: 10.1007/s00227-013-2232-6
Knox, G. A., and Lowry, G. K. (1977). “A comparison between the benthos of the Southern Ocean and the North Polar Ocean with special reference to the Amphipoda and Polychaeta,” in Polar Oceans, ed M. J. Dunbar (Calgary: Artic Institute of North America), 423–462.
Le Quesne, W. J. F., and Codling, E. A. (2009). Managing mobile species with MPAs: the effects of mobility, larval dispersal, and fishing mortality on closure size. ICES J. Mar. Sci. 66, 122–131. doi: 10.1093/icesjms/fsn202
Lee, J., and Chown, S. (2007). Mytilus on the move: transport of an invasive bivalve to the Antarctic. Mar. Ecol. Prog. Ser. 339, 307–310. doi: 10.3354/meps339307
Leese, F., Agrawal, S., and Held, C. (2010). Long-distance island hopping without dispersal stages: transportation across major zoogeographic barriers in a Southern Ocean isopod. Naturwissenschaften 97, 583–594. doi: 10.1007/s00114-010-0674-y
Levin, L. A. (1984). Multiple patterns of development in Streblospio benedicti Webster (Spionidae) from three coasts of North America. Biol. Bull. 166, 494–508. doi: 10.2307/1541157
Levin, L. A. (2006). Recent progress in understanding larval dispersal: new directions and digressions. Integr. Comp. Biol. 46, 282–297. doi: 10.1093/icb/icj024
Linse, K., Cope, T., Lörz, A.-N., and Sands, C. (2007). Is the Scotia Sea a centre of Antarctic marine diversification? Some evidence of cryptic speciation in the circum-Antarctic bivalve Lissarca notorcadensis (Arcoidea: Philobryidae). Polar Biol. 30, 1059–1068. doi: 10.1007/s00300-007-0265-3
Linse, K., Griffiths, H. J., Barnes, D. K. A., Brandt, A., Davey, N., David, B., et al. (2013). The macro- and megabenthic fauna on the continental shelf of the eastern Amundsen Sea, Antarctica. Cont. Shelf Res. 68, 80–90. doi: 10.1016/j.csr.2013.08.012
Lörz, A.-N., Maas, E. W., Linse, K., and Coleman, C. O. (2009). Do circum-Antarctic species exist in peracarid Amphipoda? A case study in the genus Epimeria Costa, 1851 (Crustacea, Peracarida, Epimeriidae). ZooKeys 18, 91–128. doi: 10.3897/zookeys.18.103
Mackay, J., and Gibson, G. (1999). The influence of nurse eggs on variable larval development in Polydora cornuta (Polychaeta: Spionidae). Invertebr. Repr. Dev. 35, 167–176. doi: 10.1080/07924259.1999.9652383
Madec, G. (2008). Nemo Ocean Engine: Note du Pole de Modélisation 27. France: Institut Pierre-Simon Laplace.
Marsh, A. G., Leong, P. K., and Manahan, D. T. (1999). Energy metabolism during embryonic development and larval growth of an Antarctic sea urchin. J. Exp. Biol. 202, 2041–2050.
Matschiner, M., Hanel, R., and Salzburger, W. (2009). Gene flow by larval dispersal in the Antarctic notothenioid fish Gobionotothen gibberifrons. Mol. Ecol. 18, 2574–2587. doi: 10.1111/j.1365-294X.2009.04220.x
Metaxas, A., and Saunders, M. (2009). Quantifying the “bio-” components in biophysical models of larval transport in marine benthic invertebrates: advances and pitfalls. Biol. Bull. 216, 257–272. doi: 10.1086/BBLv216n3p257
Moreau, C., Saucede, T., Jossart, Q., Agüera, A., Brayard, A., and Danis, B. (2017). Reproductive strategy as a piece of the biogeographic puzzle: a case study using Antarctic sea stars (Echinodermata, Asteroidea). J. Biogeogr. 44, 848–860. doi: 10.1111/jbi.12965
Neal, L., Linse, K., Brasier, M. J., Sherlock, E., and Glover, A. G. (2017). Comparative marine biodiversity and depth zonation in the Southern Ocean: evidence from a new large polychaete dataset from the Scotia and Amundsen Sea. Mar. Biodiv. 47, 1–21. doi: 10.1007/s12526-017-0735-y
Neal, L., Wiklund, H., Muir, A. I., Linse, K., Glover, A. G., et al. (2014). The identity of juvenile Polynoidae (Annelida) in the Southern Ocean revealed by DNA taxonomy, with notes of the status of Herdmanella gracilis Ehlers sensu Augener. Mem. Mus. Vic. 71, 203–216.
Nikula, R., Fraser, C., Spencer, H., and Waters, J. (2010). Circumpolar dispersal by rafting in two subantarctic kelp-dwelling crustaceans. Mar. Ecol. Prog. Ser. 405, 221–230. doi: 10.3354/meps08523
Orsi, A. H., Nowlin, W. D., and Whitworth, T. (1993). On the circulation and stratification of the Weddell Gyre. Deep Sea Res. Part. I. Oceanogr. Res. Pap. 40, 169–203. doi: 10.1016/0967-0637(93)90060-G0
Orsi, A. H., Whitworth III, T., and Nowlin, W. D. Jr. (1995). On the meridional extent and fronts of the antarctic circumpolar current. Deep Sea Res. Part. I. Oceanogr. Res. Pap. 42, 641–673. doi: 10.1016/0967-0637(95)00021-W
Palumbi, S. R. (1994). Genetic divergence, reproductive isolation, and marine speciation. Ann. Rev. Ecol. Syst. 25, 547–572. doi: 10.1146/annurev.es.25.110194.002555
Paxton, H., Wiklund, H., Alexander, F., and Taboada, S. (2016). Is the Antarctic Ophryotrocha orensanzi (Annelida: Dorvilleidae) a circumpolar non-specialized opportunist? Syst. Biodivers. 2, 105–114. doi: 10.1080/14772000.2016.1218371
Pearse, J. S., McClintock, J. B., and Bosch, I. (1991). Reproduction of Antarctic benthic marine invertebrates: tempos, modes, and timing. Am. Zool. 31, 65–80. doi: 10.1093/icb/31.1.65
Pearse, J. S., Mooi, R., Lockhart, S. J., and Brandt, A. (2009). “Brooding and species diversity in the Southern Ocean: selection for brooders or speciation within brooding clades?” in Smithsonian at the Poles: Contributions to International Polar Year Science, eds I. Krupnik, M. A. Lang, and S. E. Miller (Washington, DC: Smithsonian Institution), 181–196.
Pernet, B., Qian, P., Rouse, G. W., Young, C. M., and Eckelbarger, K. J. (2002). “Chapter 12: phylum annelida: polychaeta,” in Atlas of Marine Invertebrate Larvae, eds C. M. Young, M. A. Sewell, and M. E. Rice (Cambridge, MA: Academic Press Inc.), 209–243.
Piñones, A., Hofmann, E. E., Daly, K. L., Dinniman, M. S., and Klinck, J. M. (2013). Modeling the remote and local connectivity of Antarctic krill populations along the western Antarctic Peninsula. Mar. Ecol. Prog. Ser. 481, 69–92. doi: 10.3354/meps10256
Planes, S., Jones, G. P., and Thorrold, S. R. (2009). Larval dispersal connects fish populations in a network of marine protected areas. Proc. Natl. Acad. Sci. U.S.A. 106, 5693–5697. doi: 10.1073/pnas.0808007106
Raupach, M. J., Malyutina, M., Brandt, A., and Wägele, J.-W. (2007). Molecular data reveal a highly diverse species flock within the munnopsoid deep-sea isopod Betamorpha fusiformis (Barnard, 1920) (Crustacea: Isopoda: Asellota) in the Southern Ocean. Deep Sea Res. Part. II. Top. Stud. Oceanogr. 54, 1820–1830. doi: 10.1016/j.dsr2.2007.07.009
Raupach, M. J., Thatje, S., Dambach, J., Rehm, P., Misof, B., and Leese, F. (2010). Genetic homogeneity and circum-Antarctic distribution of two benthic shrimp species of the Southern Ocean, Chorismus antarcticus and Nematocarcinus lanceopes. Mar. Biol. 157, 1783–1797. doi: 10.1007/s00227-010-1451-3
Riesgo, A., Taboada, S., and Avila, C. (2015). Evolutionary patterns in Antarctic marine invertebrates: an update on molecular studies. Mar. Genomics 23, 1–13. doi: 10.1016/j.margen.2015.07.005
Robinson, J., New, A., Popova, E., Srokosz, M., and Yool, A. (2017). Far-field connectivity of the UK's four largest marine protected areas: four of a kind? Earths Future 5, 475–494. doi: 10.1002/2016EF000516
Rogers, A. (2007). Evolution and biodiversity of Antarctic organisms: a molecular perspective. Philos. Trans. R. Soc. B. 362, 2191–2214. doi: 10.1098/rstb.2006.1948
Rogers, A. D., Morley, S., Fitzcharles, E., Jarvis, K., and Belchier, M. (2006). Genetic structure of Patagonian toothfish (Dissostichus eleginoides) populations on the Patagonian Shelf and Alantic and Western Indian Ocean Sectors of the Southern Ocean. Mar. Biol. 149, 915–924. doi: 10.1007/s00227-006-0256-x
Rouse, G. W., and Pleijel, F. (2006). Reproductive Biology and Phylogeny of Annelida. Enfield, CT: Science Publishers.
Schüller, M. (2011). Evidence for a role of bathymetry and emergence in speciation in the genus Glycera (Glyceridae, Polychaeta) from the deep Eastern Weddell Sea. Polar Biol. 34, 549–564. doi: 10.1007/s00300-010-0913-x
Schüller, M., and Ebbe, B. (2007). Global distributional patterns of selected deep-sea Polychaeta (Annelida) from the Southern Ocean. Deep Sea Res. Part. II. Top. Stud. Oceanogr. 54, 1737–1751. doi: 10.1016/j.dsr2.2007.07.005
Schüller, M., and Ebbe, B. (2014). “Chapter 5.1 polychaetes,” in Biogeographic Atlas of the Southern Ocean, eds C. De Broyer, P. Koubbi, H. Griffiths, S. Grant, and E. Al (Cambridge: Scientific Committee on Antarctic Research), 134–137.
Sewell, M. A., and Jury, J. A. (2011). Seasonal patterns in diversity and abundance of the high Antarctic meroplankton: plankton sampling using a Ross Sea desalination plant. Limnol. Oceanogr. 56, 1667–1681. doi: 10.4319/lo.2011.56.5.1667
Shanks, A. L. (2009). Pelagic larval duration and dispersal distance revisited. Biol. Bull. 216, 373–385. doi: 10.1086/BBLv216n3p373
Shilling, F. M., and Manahan, D. T. (1994). Energy metabolism and amino acid transport during early development of Antarctic and temperate echinoderms. Biol. Bull. 187, 398–407. doi: 10.2307/1542296
Smith, C. R., Drazen, J., and Mincks, S. L. (2006). “Deep-sea biodiversity and biogeography: perspectives from the abyss,” in International Seabed Authority Seamount Biodiversity Symposium (San Diego, CA).
Soler-Membrives, A., Katrin, K., Miller, K. J., and Arango, C. P. (2017). Genetic signature of Last Glacial Maximum regional refugia in a circum-Antarctic sea spider. R. Soc. Open Sci. 4:170615. doi: 10.1098/rsos.170615
Starmans, A., Gutt, J., and Arntz, W. (1999). Mega-epibenthic communities in Arctic and Antarctic shelf areas. Mar. Biol. 135, 269–280. doi: 10.1007/s002270050624
Strelzov, V. (1979). Polychaete Worms of the Family Paraonidae Cerruti, 1909. Washington, DC: Smithsonian Institution.
Strugnell, J. M., and Allcock, A. L. (2013). “Southern Ocean evolution in a global context: a molecular viewpoint,” in Adaptation and Evolution in Marine Environments, Vol. 2, eds C. Verde and G. di Prisco (New York, NY: Springer), 35–53.
Swearer, S. E., Shima, J. S., Hellberg, M. E., Thorrold, S. R., Jones, G. P., Robertson, D. R., et al. (2002). Evidence of self-recruitment in demersal marine populations. Bull. Mar. Sci. 70, 251–271.
Templeton, A. R., Crandall, K. A., and Sing, C. F. (1992). A cladistic analysis of phenotypic associations with haplotypes inferred from restriction endonuclease mapping and DNA sequence data. III. Cladogram estimation. Genetics 132, 619–633.
Thatje, S., Hillenbrand, C. D., and Larter, R. (2005). On the origin of Antarctic marine benthic community structure. Trends Ecol. Evol. (Amst). 20, 534–540. doi: 10.1016/j.tree.2005.07.010
Thompson, A. F., Heywood, K. J., Thorpe, S. E., Renner, A. H., and Trasviña, A. (2009). Surface circulation at the tip of the Antarctic Peninsula from drifters. J. Phys. Oceanogr. 39, 3–26. doi: 10.1175/2008JPO3995.1
Thornhill, D. J., Mahon, A. R., Norenburg, J. L., and Halanych, K. M. (2008). Open-ocean barriers to dispersal: a test case with the Antarctic Polar Front and the ribbon worm Parborlasia corrugatus (Nemertea: Lineidae). Mol. Ecol. 17, 5104–5117. doi: 10.1111/j.1365-294X.2008.03970.x
Thorpe, S., Murphy, E., and Watkins, J. (2007). Circumpolar connections between Antarctic krill (Euphausia superba Dana) populations: investigating the roles of ocean and sea ice transport. Deep Sea Res. Part. I. Oceanogr. Res. Pap. 54, 792–810. doi: 10.1016/j.dsr.2007.01.008
Van Dover, C. L., Trask, J., Gross, J., and Knowlton, A. (1999). Reproductive biology of free-living and commensal polynoid polychaetes at the Lucky Strike hydrothermal vent field (Mid-Atlantic Ridge). Mar. Ecol. Prog. Ser. 181, 201–214. doi: 10.3354/meps181201
Waters, J. (2008). Driven by the west wind drift? A synthesis of southern temperate marine biogeography, with new directions for dispersalism. J. Biogeogr. 35, 417–427. doi: 10.1111/j.1365-2699.2007.01724.x
Wilson, N. G., Maschek, J. A., and Baker, B. J. (2013). A species flock driven by predation? Secondary metabolites support diversification of slugs in Antarctica. PLoS ONE 8:e80277. doi: 10.1371/journal.pone.0080277
Wilson, N., Hunter, R., Lockhart, S., and Halanych, K. (2007). Multiple lineages and absence of panmixia in the “circumpolar” crinoid Promachocrinus kerguelensis from the Atlantic sector of Antarctica. Mar. Biol. 152, 895–904. doi: 10.1007/s00227-007-0742-9
Keywords: circumpolar, biogeography, deep-sea, cryptic species, Southern Ocean, benthos
Citation: Brasier MJ, Harle J, Wiklund H, Jeffreys RM, Linse K, Ruhl HA and Glover AG (2017) Distributional Patterns of Polychaetes Across the West Antarctic Based on DNA Barcoding and Particle Tracking Analyses. Front. Mar. Sci. 4:356. doi: 10.3389/fmars.2017.00356
Received: 13 July 2017; Accepted: 24 October 2017;
Published: 16 November 2017.
Edited by:
Wei-Jen Chen, National Taiwan University, TaiwanReviewed by:
Stephane Hourdez, Centre National de la Recherche Scientifique (CNRS), FranceCopyright © 2017 Brasier, Harle, Wiklund, Jeffreys, Linse, Ruhl and Glover. This is an open-access article distributed under the terms of the Creative Commons Attribution License (CC BY). The use, distribution or reproduction in other forums is permitted, provided the original author(s) or licensor are credited and that the original publication in this journal is cited, in accordance with accepted academic practice. No use, distribution or reproduction is permitted which does not comply with these terms.
*Correspondence: Madeleine J. Brasier, bS5icmFzaWVyQGxpdmVycG9vbC5hYy51aw==
Disclaimer: All claims expressed in this article are solely those of the authors and do not necessarily represent those of their affiliated organizations, or those of the publisher, the editors and the reviewers. Any product that may be evaluated in this article or claim that may be made by its manufacturer is not guaranteed or endorsed by the publisher.
Research integrity at Frontiers
Learn more about the work of our research integrity team to safeguard the quality of each article we publish.