- 1Programa de Doctorado en Oceanografía, Departamento de Oceanografía, Facultad de Ciencias Naturales y Oceanográficas, Universidad de Concepción, Concepción, Chile
- 2Laboratoire d'Océanographie Microbienne (LOMIC), Observatoire Océanologique, UPMC Université Pierre et Marie Curie, Univ Paris 06, CNRS, Sorbonne Universités, Banyuls Sur Mer, France
- 3FONDAP INCAR Center (15110027), PFB-31 COPAS Sur Austral Program, Departamento de Oceanografía, Universidad de Concepción, Concepción, Chile
- 4Centro de Investigación Dinámica de Ecosistemas Marinos de Altas Latitudes (IDEAL), FONDAP Fondo de Financiamiento de Centros de Investigación en Áreas Prioritarias, Valdivia, Chile
- 5Departamento de Biología and Programa de Biodiversidad, Facultad de Ciencias Naturales y Exactas, Universidad de Playa Ancha, Valparaíso, Chile
- 6Departamento de Oceanografía, Universidad de Concepción, Concepción, Chile
- 7Instituto Milenio de Oceanografía, Universidad de Concepción, Concepción, Chile
Copepods are important suppliers of bioreactive compounds for marine bacteria through fecal pellet production, sloppy feeding, and the excretion of dissolved compounds. However, the interaction between copepods and bacteria in the marine environment is poorly understood. We determined the nitrogen and phosphorus compounds excreted by copepods fed with two natural size-fractionated diets (<20- and 20–150-μm) in the upwelling zone of central/southern Chile in late summer and spring. We then assessed the biogeochemical response of the bacterial community and its structure, in terms of total and active cells, to enrichment by copepod-excreted dissolved compounds. Results revealed that copepods actively excreted nitrogen and phosphorus compounds, mainly in the form of ammonium and dissolved organic phosphorus (DOP), reaching excretion rates of 2.6 and 0.05 μmol L−1h−1, respectively. In both periods, the maximum excretion rates were associated with the 20–150-μm size fraction, but particularly during spring, when a higher organic matter quality was observed in excretion products compared to late summer. There were higher excretion rates of dissolved free amino acids (DFAAs) from copepods fed with the <20-μm size fraction, mainly histidine (HIS) in late summer and glutamic acid (GLU) in spring. A shift in the composition of the active bacterial community was observed between periods and treatments, which was associated with the response of the common seawater surface phyla Proteobacteria and Bacteroidetes. The specific bacterial activity (16S rRNA:rDNA) suggested a different response to the two size-fractionated diets. In late summer, Betaproteobacteria and Bacteroidetes were stimulated by the treatment enriched with excretion products derived from the 20–150-μm and <20-μm size fractions, respectively. In spring, Alphaproteobacteria were active in the treatment enriched with the excretion products of copepods fed with the <20-μm size fraction, whereas they were inhibited in the treatment enriched with excretion products in the 20–150-μm size fraction. Our findings indicate that different copepod diets can have a significant impact on the quantity and quality of their excretion compounds, which can subsequently generate shifts in the active bacterial composition. The bacterial response is probably associated with common-opportunistic sea surface microbes that are adapted to rapidly reacting to environmental offers.
Introduction
Zooplankton are responsible for removing up to 40% of the primary production in marine environments (Calbet, 2001). A portion of the organic material assimilated by zooplankton is used for growth and reproduction. The portion that is not assimilated is released as dissolved compounds, via excretion (Møller, 2007; Saba et al., 2011). There is a lack of knowledge regarding the impact of dissolved compounds released by zooplankton in biogeochemical cycles and in the microbial community structure. Experiments designed to explore the use of these compounds by the microbial community would therefore provide valuable information regarding the role of zooplankton in nitrogen and phosphorus turnover. A recent study of the impact of krill excretion on microbial activity demonstrated that the addition of krill excretion products stimulates bacterial growth and production (Arístegui et al., 2014). Studies have shown that a jellyfish bloom can provide significant amounts of dissolved organic matter (DOM) due to jellyfish degradation and excretion, facilitating the mineralization of dissolved organic carbon (DOC) and dissolved organic nitrogen (DON), followed by a high accumulation of ammonium () and phosphate () that becomes available for microbial communities (West et al., 2009; Blanchet et al., 2015).
Ammonium is recognized as the main excretion product of zooplankton (Bidigare, 1983). However, several studies have recognized DON as an important excreted material, accounting for between 7 and 90% of the total dissolved nitrogen (Miller and Glibert, 1998; Conover and Gustavson, 1999; Steinberg et al., 2000, 2002; Pérez-Aragón et al., 2011). Most previous studies have focused on nitrogen excretion, while only a few studies have focused on phosphorus compounds, and these have mainly considered (Pomeroy et al., 1963; Ikeda et al., 2001; Saba et al., 2009; Alcaraz et al., 2010). The composition and quantity of the compounds excreted by zooplankton are highly dependent on the quantity and quality of the food that they consume. The effect of the food consumed on the content of zooplankton excreta has been reported by several authors (e.g., Miller and Roman, 2008; Alcaraz et al., 2010). However, some of the results are controversial and more studies are needed. Saba et al. (2009) observed that the release of inorganic nitrogen by copepods is variable and dependent on the type of diet, showing that the rate of release was highest when feeding on a carnivorous diet, and lowest while feeding omnivorously. In contrast, organic nitrogen, such as urea, can account for a higher proportion of the total dissolved nitrogen when copepods feed on a mixed diet (omnivorously). Moreover, inorganic phosphorus excretion was higher when the copepod diet was herbivorous.
The importance of excretion products in biogeochemical cycles in the upper ocean has been identified in several studies. These products are recognized as a source of dissolved compounds for the microbial community and phytoplankton, and they also contribute to the vertical transport of bio-elements via diel vertical migration (Steinberg et al., 2002; Escribano et al., 2009; Pérez-Aragón et al., 2011). There have been many studies of the bioreactivity of DOM in recent decades, with DOM recognized as the largest reservoir of nutrients in marine environments. This reservoir can therefore be available for phytoplankton, with the microbial community being rapidly utilized over timescales of minutes to weeks for the most labile fraction (Bronk et al., 2007; Carlson and Hansell, 2015). Recent studies have shown that bacterial community composition can change in response to the addition of organic matter (Landa et al., 2013; Sarmento et al., 2013). DOM release during phytoplankton blooms is associated with important changes in microbial diversity and metabolism (McCarren et al., 2010; Sarmento and Gasol, 2012). Titelman et al. (2008) showed that copepod feeding can enhance organic phosphorus uptake and bacterial activity in a phosphorus-limited system. Therefore, the importance of these predator–prey interactions, as a resource for the microbial food web, depends on the type of DOM substrate and on the prevailing limitations.
Although previous studies have shown the responses of the bacterial community to the input of DOM, the interactions among zooplankton feeding conditions and excretion and the bacterial community have not been examined in the pelagic system. Here, we used an experimental approach, with natural food sources, and simulated in situ conditions to test the hypothesis that zooplankton excretion can significantly reshape the bacterial community structure, with such effects conditioned by the type of food available under a seasonal upwelling situation. For this, we offered sized-fractionated diets to the dominant zooplankton (copepods) and then assessed the effects of copepod excretory products on changes in organic and inorganic nutrients, and on the activity and composition of natural microbial assemblages.
Materials and Methods
Study Area
The study area was located in the coastal upwelling system in central/southern Chile (Supplementary Figure 1A). Sampling was conducted on board the R/V Kay Kay II (University of Concepcion). The sampling stations were located ~22 km from the coast (Station 12; 36° 30′S, 73°04′W) and ~33 km from the coast (Station 18; 36° 30.8′S, 73°08′W). Station 12 was visited on March 23, 2014 and Station 18 on December 12, 2014, which corresponded to late summer and spring, respectively.
Sampling Strategy
At each sampling station, an autonomous oceanographic profiler (CTD-O SeaBird 19, Sea-Bird Scientific, Bellevue, WA, USA) was used to obtain temperature, salinity, and dissolved oxygen measurements. Seawater samples were obtained at 10-m depth, with a Niskin bottle (10 L) for incubations and chemical-biological measurements. Seawater used for incubation was stored in acid-cleaned carboys, in dark conditions, for transfer to the Dichato Marine Station at the University of Concepcion (~1 h). Seawater samples were analyzed on board for , nitrate (), nitrite (), , and bacterioplankton abundance. Samples collected for DNA and RNA analysis (the latter was only analyzed in spring) were immediately filtered upon arrival at the laboratory (~1 h). Measurements of total and size-fractionated chlorophyll-a (Chl-a), and microplankton and nanoplankton abundance, were obtained at each site to determine the in situ food available for copepods (see Materials and Methods in the “Chemical and biological analysis” and “Molecular methods” sections).
Chemical and Biological Analysis
Samples for the analysis of Chl-a were taken in triplicate (300 mL) and filtered through GF/F filters (Whatman, Maidstone, UK). Samples used for the size-fractionated Chl-a analysis were pre-filtered onto a polycarbonate membrane (20-μm mesh). Both samples were measured by a fluorometric method (Parson et al., 1984). Nanoplankton abundance and composition were analyzed by epifluorescence microscopy. Samples were taken in duplicate in centrifuge tubes (50 mL) and immediately preserved with glutaraldehyde (2% final concentration). Microplankton samples were collected in duplicate (250 mL) and preserved with Lugol solution and analyzed by the Utermöhl method, based on inverted microscopy (Villafañe and Reid, 1995). Samples for the determination of bacterioplankton, cyanobacteria, and picoeukaryote abundance were taken in duplicate (1,350 μL in sterile cryovials), fixed with glutaraldehyde (150 μL of 1% glutaraldehyde) and stored at −80°C prior to laboratory analysis. Samples were analyzed by flow cytometry (FACScan, Becton Dickinson) according to Marie et al. (1997).
Duplicate samples for the analysis of nutrients (, , and ) were filtered through 0.7-μm filters (GF/F; Whatman), placed in 11-mL HDPE plastic tubes (acid-washed), and stored at −20°C prior to laboratory analysis. Concentrations of , , and were determined using standard colorimetric techniques (Grasshof, 1983). Duplicate samples for determination (50 ml) were analyzed immediately according to Holmes et al. (1999) using a Turner Designs fluorometer. Samples for DON and dissolved organic phosphorus (DOP; 30 mL) were filtered through pre-combusted (450°C, 6 h) GF/F filters (Whatman), and analyzed by the wet oxidation procedure (Pujo-Pay and Raimbault, 1994). DON and DOP concentrations were corrected for , , and , respectively. Dissolved free amino acid (DFAA) samples (15 mL) were filtered through pre-combusted GF/F filters (Whatman), dispensed in duplicate in acid-cleaned and pre-combusted (450°C, 6 h) glass flasks, and stored immediately at −20°C prior to analysis. DFAA quantification and identification were determined using high-performance liquid chromatography (HPLC; LC-10TA, Shimadzu, Kyoto, Japan) according to Pantoja and Lee (2003).
Zooplankton Collection
Zooplankton were captured at each site by means of vertical hauls of a WP-2 net of 200-μm mesh-size. The net was equipped with a non-filtering cod end to obtain undamaged individuals. Once onboard, the samples were poured into coolers and diluted with surface seawater (10-m depth) to keep the organisms alive and maintain them in a suitable condition prior to sorting at the laboratory (~1 h).
The Collection of Food and the Microbial Community for Use in Experiments
Seawater (20 L), collected at 10-m depth, was used as the medium to evaluate the impact of the type of food ingested by copepods on the composition of dissolved compounds being excreted, and the subsequent impact on bacterial community structure. Seawater was first filtered through a 150-μm sieve to remove large plankton particles and then through a 20-μm sieve. The fraction retained by the 20-μm sieve was re-suspended in filtered seawater (GF/F; Whatman) to produce the 20–150 μm food fraction, while the media passing through the 20-μm sieve was used as the <20-μm food fraction. Both size-fractions were maintained in the dark in a cold room (12°C) until the experiments were conducted (~7 h later).
Different microbial assemblages were targeted for the late-summer and spring experiments. In late summer, a larger-sized microbial community was used for incubations (1.6-μm-filtered seawater; APFA04700 pre-filters; Millipore). This medium enabled potential predation effects to occur during the experiment, which was not possible with the smaller microbial community used in the spring (0.7-μm-filtered seawater; GF/F; Whatman) that was assumed to be free of grazers. The bottles with natural microbial assemblages were prepared before the experiment (~4 h earlier) and kept under similar conditions as the food (12°C).
Experimental Procedures
To explore the interaction between the dissolved compounds excreted by copepods and bacteria, we designed an experiment consisting of four phases: (1) copepod selection and acclimation, (2) copepod feeding, (3) copepod excretion, and (4) the microbial response to dissolved compounds excreted in the previous phase (Supplementary Figure 2). The experimental design of each phase was as follows:
Phase 1. For each sampling event, live zooplankton samples were sorted using a stereomicroscope under low-light conditions. The number of individuals used in both experiments was determined based on the mean abundance observed at Station 18 in the Center for Oceanographic Research in the eastern South Pacific (COPAS) time series (Escribano et al., 2007; Hidalgo et al., 2010). In late summer, a mix of two abundant adult copepods, Acartia tonsa (71%) and Paracalanus cf indicus (29%) was sorted, whereas, in spring, a mix of three adult copepods was sorted, P. cf indicus (37.5%), Calanoides patagoniensis (45%), and Spinocalanus sp. (17.5%). On both sampling dates, four groups of copepods, containing the same community, were isolated from the surrounding water using cylinders with a 200-μm mesh inside to prevent the escape of copepods, and were maintained in two glass incubators (previously washed with 10% HCl) filled with 10 L of 0.7-μm-filtered seawater (GF/F; Whatman). The acclimation was conducted under dark conditions for 6 h.
Phase 2. The four copepod groups selected were then transferred for feeding purposes into two 10-L incubators containing different size fractions of natural food, <20-μm and 20–150-μm (two copepod groups for each incubator). This feeding phase was performed under dark conditions (12°C) and lasted 4 h.
Phase 3. The copepod groups were transferred to a different set of duplicate incubators containing 0.2-μm-filtered seawater (Durapore; Millipore). This was done to minimize the presence of microbial assemblages during the excretion phase. The incubator system consisted of six chambers (6 L each), two for the treatment fed with the 20–150-μm size-fraction diet, two for the treatment fed with the <20-μm size-fraction diet, and two incubators used as controls (without copepods). For the feeding and acclimation phase, copepods were isolated in the chamber using cylinders with a 200-μm mesh inside. Each chamber was equipped with a sampling faucet to retrieve subsamples with minimal disturbance. At time intervals of 0 h (T0), 1 h (T1: T0+1h), and 2 h (T2: T0+2h) samples were collected in duplicate from the sampling faucet with a 0.5-L glass flask (acid-washed and autoclaved; Duran Schott) for the analysis of , , , , DON, DOP, and DFAA. Bacterioplankton abundance was monitored during all incubation times to evaluate the potential influence of small size microbial communities.
Phase 4. To evaluate the microbial response to dissolved compounds provided by copepod excretion, 10 glass bottles containing 850 mL of seawater with natural microbial assemblages (10-m depth), were enriched with 150-mL aliquots of the excretion products derived from phase 3. The ten glass bottles consisted of four bottles for the treatments derived from copepods fed with the 20–150-μm size-fraction diet, four bottles for the treatments derived from copepods fed with the <20-μm size-fraction diet, and two control bottles that were derived from one of the chambers without copepods from the previous phase 3. We discarded one of the control chambers from the previous phase because of the limited time available to handle the samples.
Samples were collected in duplicate at time intervals of 0 h (T0), 2 h (T1: T0+2 h), 4 h (T2: T0 +4 h), and 6 h (T3: T0+6 h) for the analysis of , , , , DON, DOP, DFAA, RNA, bacterioplankton, cyanobacteria, and picoeukaryote abundance. DNA and RNA samples were taken to assess the changes in the bacterial community structure, so that the assessment included the whole bacterial assemblage and the active assemblage. The same protocol was applied for DNA samples, but in 4-L polycarbonate bottles (Nalgene). In this analysis, 3.4 L of the natural microbial assemblage was inoculated with 600-mL aliquots of dissolved compounds excreted by copepods from each chamber of the previous phase. In total, six bottles were inoculated (Supplementary Figure 2). DNA samples were taken at the initial (T0) and final incubation time (T3: T0+6h), whereas RNA samples were taken at all-time intervals.
The mean concentration of , , DON, DOP, and DFAAs in the control was subtracted from the results for the treatments so as to obtain the concentration related to excretion during the incubation periods. The excretion rates of each of these nitrogen and phosphorus compounds were estimated using the slope of the linear regression fitted to the concentration as a function of incubation time. The concentrations obtained in the controls during microbial response phase 4 were averaged and subtracted from the treatments at each time (T0, T1, T2, and T3), as was done for excretion phase 3. From these values, we estimated the turnover rate of , , , DON, DOP, and DFAAs every 2 h (T0-T1, T1-T2, and T2-T3). The rates were estimated using an end-point approach as follows:
A positive value indicated a net accumulation, whereas a negative value indicated a net nutrient consumption.
Molecular Methods
DNA samples for the initial characterization of bacterial communities were collected at 10-m depth at each sampling site and were maintained in acid-cleaned carboys prior to arrival at the laboratory. Samples (2 L) were filtered through cellulose ester filters (0.22 μm; Millipore) using a peristaltic pump and stored with RNAlater solution (Ambion, Waltham, MA, USA) at −20°C until extraction procedures. DNA samples (2 L) obtained during phase 4 (microbial response) were filtered (0.22 μm; Millipore) directly from 4-L carboys (see “Experimental procedures” section). Samples were taken in duplicate and stored as indicated above. However, only one of the duplicate samples was extracted for each incubation time. DNA extraction was conducted as described in (Levipan et al., 2014), using the PowerSoil DNA Isolation Kit (MoBio Laboratories, Carlsbad, CA, USA) in accordance with the manufacturer's specifications. For the late-summer experiment, samples were analyzed by spectrophotometry (NanoDrop ND-1000 Spectrophotometer, Thermo Fisher Scientific, Waltham, MA, USA), whereas for the spring experiment the sample concentrations were obtained from Qubit assays (Invitrogen, Carlsbad, CA, USA). Details are given in Supplementary Table 1.
For the initial characterization of the active bacterial community at 10-m depth, RNA samples were collected only in the experiment performed in the spring, whereas for the microbial response phase, RNA samples were collected in both experiments (late summer and spring). Using a sterilized syringe and a 25-mm Swinnex filter, seawater (100 mL) was filtered through 0.22-μm hydrophilic PVDF filters (Millipore). The filters were preserved with RNAlater solution (Ambion) and stored at −80°C prior to RNA extraction. RNA samples were extracted using a Mirvana kit (AM1560; Ambion) in accordance with the manufacturer's instructions, including a mechanical disruption step and homogenization using 200-μm diameter zirconium beads (low-binding zirconium beads, OPS Diagnostics, Lebanon, NJ, USA) and homogenized twice at ~3,000 rpm for 30 s using a Mini-Beadbeater-8™ (Biospec Products, Bartlesville, OK, USA). To remove traces of DNA, RNA was treated with a TURBO DNA-free kit™ (Ambion). Finally, for the samples from the late-summer experiment, the concentration and quality (A260/A280 ratio) of RNA and DNA extracts were determined by spectrophotometry (NanoDrop ND-1000 Spectrophotometer). For the spring experiment, the sample concentrations were obtained using a Qubit fluorometer (Invitrogen), following the manufacturer's instructions. The details are given in Supplementary Table 1.
Bacterial 16S rRNA Gene Analysis Using the Illumina MiSeq Platform
Bacterial community structure was analyzed in terms of both total and active fractions by the Illumina high-throughput sequencing method, using cDNA as the template and 16S rDNA (Campbell and Kirchman, 2013). The cDNA was generated using random primers provided by the ImProm-II™ Reverse Transcription System (Promega, Madison, WI, USA). Bacterial 16S rRNA genes from the V1–V3 region (27F-519R) were generated at the Research and Testing Laboratory (RTL, Lubbock, TX, USA).
The 16S rRNA and rDNA gene sequences were processed using Mothur software v1.35.1 (Schloss et al., 2009). Sequencing data sets were curated by quality filtration, to minimize the effects of random sequencing errors, by eliminating sequence reads <200 bp and trimming sequences that contained more than one undetermined nucleotide and sequences with a maximum homopolymer length of eight nucleotides. Thereafter, chimeric sequences were identified using the chimera UCHIME algorithm (Edgar et al., 2011) and removed to so as to retain high-quality reads.
The 16S rRNA and rDNA gene sequences were taxonomically classified using the automatic software pipeline SILVAngs available from https://www.arb-silva.de/ (Quast et al., 2013). The libraries were deposited in the European Nucleotide Archive (ENA) under study accession PRJE21106, with the following run access numbers: ERS1770858 and ERS1775609-ERS1775646.
The composition was analyzed at the phylum, class, and order taxonomic levels and categorized as abundant (>0.5%), semi-rare (0.1–0.5%), and rare (<0.1%) in the total sequences retrieved from each library (Pedrós-Alió, 2012). The Chao, Shannon, and Evenness indices were calculated using Past3 software. Previously, because the number of sequences per sample was variable, the diversity indices were normalized to the same number of sequences per sample (3,320 in late summer and 757 in spring) using Mothur software, version 1.35.1 (Schloss et al., 2009).
Statistical Analyses
For results obtained during the excretion phase, the statistical analysis of the effect of different treatments on the chemical parameters associated with excretion products was performed using a paired t-test. During microbial response phase, a two-way analysis of variance (ANOVA) was applied using treatments and time as levels, after checking for normality assumptions (Kolmogorov-Smirnov test) and homoscedasticity (Levene's test). A pairwise multiple comparison was performed using a Tukey test as an a posteriori analysis.
Bacterial community structure was compared using ordination Bray–Curtis similarities, visualized in Unweighted Pair Group Method with Arithmetic mean (UPGMA) dendrograms, whose nodes were further tested using a bootstrap analysis. A multivariate analysis was also used to analyze the variability of biological and environmental variables, using PRIMER v.6 and the add-on PERMANOVA+ software package. The permutational multivariate analysis of variance (PERMANOVA) with a fixed factor was used to investigate the differences in bacterial community composition for treatments and as a control in both experiments. Principal co-ordinate analysis (PCO) was performed to visualize patterns of the operational taxonomic units (OTUs) of the bacterial community in response to different treatments.
Results
Hydrographic, Biogeochemical, and Biological Background
The hydrographic conditions during sampling events are shown in Supplementary Figure 1. In late summer 2014 (March; Supplementary Figure 1B), the temperature varied between 12.5°C in surface waters and 10.6°C near the bottom layers. Salinity varied between 34.6 and 34.9, with a surface minimum value at 10-m depth. The oxycline was very sharp, and was characterized by a strong reduction in oxygen at 20-m depth, reaching a concentration of <0.4 mL L−1 from 20-m depth to the bottom layers. In spring 2014 (December; Supplementary Figure 1C), the thermocline and oxycline were shallower than in the previous period, and were characterized by a similar temperature range (13°C in surface waters to 10.6°C in the bottom layers), but with a higher oxygen concentration in the surface and bottom layers. Salinity was less variable (34.6 and 34.7) than in the previous period, and the salinity minimum observed in the spring was absent.
Nutrient analyses at 10-m depth are shown in Table 1 for both periods. The concentration was similar between both periods, while , , and concentrations were higher under late-summer conditions compared to spring. During spring, the DFAA concentration reached 1.7 μmol L−1 and was dominated by isoleucine (ILE), followed by histidine (HIS) (see details in Supplementary Table 2).
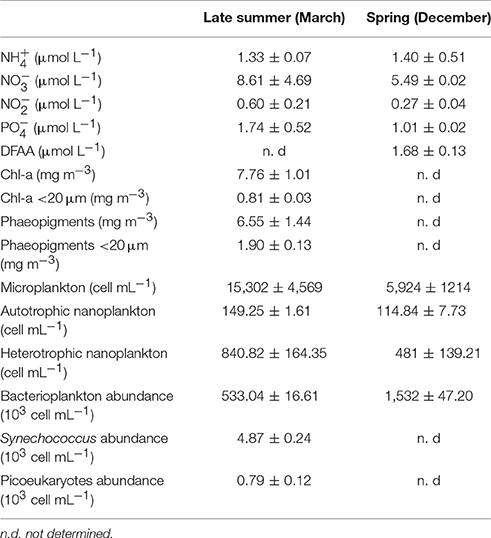
Table 1. Initial nutrient concentration, dissolved free amino acid (DFAA) concentration, photosynthetic pigments, and the abundances of nanoplankton, microphytoplankton, bacterioplankton, cyanobacteria, and picoeukaryotes s in late summer and spring (mean ± standard deviation).
During late summer, a greater Chl-a concentration was associated with the 20–150-μm size fraction, and the <20-μm size fraction and phaeopigments accounted for 10.4 and 29% of the total pigment stock, respectively. Unfortunately, there was no data available for comparison during spring (Table 1). Microphytoplankton abundances were higher during late summer compared to spring, and there was a low abundance of heterotrophic and autotrophic nanoplankton (0.9 and 5.2%, respectively). The microphytoplankton composition was dominated by Thalassiosira sp. and Chaetoceros sp. in both sampling seasons, and the bacterioplankton abundance was approximately three times higher in spring than in late summer (Table 1).
Excretion Phase: Evolution of Nitrogen and Phosphorus Compounds
During late summer (March 2014), the initial incubation time (T0) was characterized by a high concentration of , , , and compared to the in situ concentrations (Figure 1 and Table 1). The concentrations in the control were higher compared to the treatments and this difference became smaller at the end of incubation (Figure 1A). The difference between the control and treatments was only significant in the treatment fed with the 20–150-μm size fraction (t-test, P = 0.03). There was a significantly higher concentration of DON (Figure 1C) in the control than in the treatment fed with the 20–150-μm size-fraction diet (t-test, P = 0.04), and this difference increased at 2 h of incubation. The concentrations (Figure 1E) did not change significantly between the treatments (20–150 μm and <20 μm) and control (t-test, P = 0.13 and P = 0.17, respectively), but there were significant differences between the two treatment diets (t-test, P = 0.04). The initial concentrations of DOP (Figure 1G), were higher in the treatment fed with the 20–150-μm size-fraction diet (1.18 ± 0.6 μmol L−1) compared to the control and the second treatment (<20 μm). However, this difference was not significant between treatments or between the control and treatments (20–150 μm and <20 μm; t-test, P = 0.32, P = 0.21, P = 0.14, respectively). A significantly higher concentration of DFAAs (Figure 1I) was found in control compared to the treatments (20–150 and <20 μm; t-test, P < 0.001 and P < 0.001, respectively), but there were no significant differences between treatments (t-test, P = 0.06).
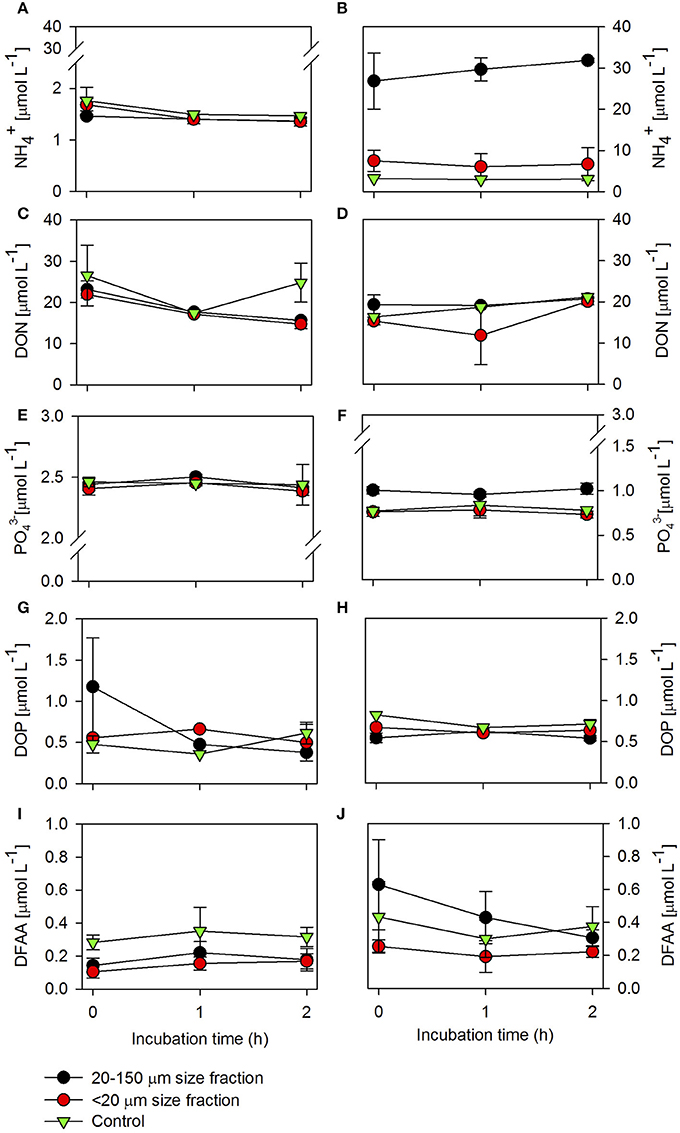
Figure 1. Ammonium () (A,B), dissolved organic nitrogen (DON) (C,D), phosphate () (E,F) dissolved organic phosphorus (DOP) (G,H), and dissolved free amino acid (DFAA) (I,J) concentrations throughout incubation in the excretion phase for both treatments and controls in late summer and spring. Errors bars show the standard deviation (n = 4).
In spring 2014, concentrations (Figure 1B) were significantly higher in the treatment in which copepods were fed with the 20–150-μm size-fraction diet than in the treatment fed with the <20-μm size-fraction diet, or the control (t-test, P < 0.001 and P < 0.001, respectively); and concentrations for the treatment fed with the <20-μm size fraction were also significantly higher than the control (t-test, P = 0.02). It is noteworthy that the initial concentration in the treatment fed with the 20–150-μm size fraction was 18 times higher in spring (27 μmol L−1) compared with the late-summer experiments (1.5 μmol L−1). The DON concentrations (Figure 1D) did not change significantly between the treatments and control (t-test, 20-150 μm P = 0.22, <20 μm P = 0.18), but DON in the treatment with the 20–150-μm size-fraction diet was significantly higher than in the treatment with the <20-μm size-fraction diet (t-test, P = 0.04). The concentrations (Figure 1F) were significantly higher in the treatment with the 20–150-μm size-fraction diet compared to the treatment with the <20-μm size-fraction diet (t-test, P < 0.001), or the control (t-test, P < 0.001). The DOP concentrations were significantly higher in both treatments (20–150 and <20 μm) than in the control (t-test, P = 0.01 and P = 0.02, respectively), but there were no significant changes between treatment diets (t-test, P = 0.78). Finally, there were significantly higher DFAA concentrations (Figure 1J) in the treatment with the 20–150-μm size-fraction diet than in the treatment with the <20-μm size-fraction diet (t-test, P = 0.03), but this difference became smaller by the end of incubation. At this stage, significant differences were only observed for the treatment with the <20-μm size-fraction diet compared to the control (t-test, P < 0.001).
In both experiments, was the main product excreted by copepods and was associated with the treatment fed with 20–150-μm size-fraction (Table 2). When standardized to the number of copepods in the incubations, the excretion rates per individual were 0.0028 and 0.0648 μmol L−1 h−1 in late summer and spring, respectively. DFAAs were excreted in late summer and spring by the copepods fed with the <20-μm size-fraction diet, with an excretion rate of 0.0003 μmol L−1 h−1. Copepods fed with the 20–150-μm size-fraction diet only excreted DFAA during late summer (0.001 μmol L−1 h−1). In both treatments, the organic form of phosphorus, DOP, was excreted only during late summer, with excretion rates of 0.0013 μmol L−1 h−1 for copepods fed with the 20–150-μm size-fraction diet and 0.001 μmol L−1 h−1 for copepods fed with the <20-μm size-fraction diet. The inorganic form of phosphorus, , was excreted to a different extent in both experiments. Under late-summer conditions, it was excreted only by copepods fed with the <20-μm size-fraction diet, while under spring conditions it was excreted by copepods fed with the 20–150-μm size-fraction diet, which corresponded to 3.57 × 10−5 and 1 × 10−4 μmol L−1 h−1 per copepod, respectively.
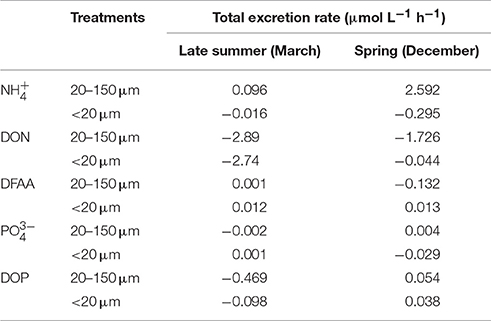
Table 2. Total excretion rates for ammonium (), dissolved organic nitrogen (DON), dissolved free amino acid (DFAA), phosphate (), and dissolved organic phosphorus (DOP), in late summer and spring.
The composition of the DFAAs excreted during the different periods and under the different treatments was variable (Figure 2). During the late-summer experiment, there was a larger accumulation of HIS in the DFAAs excreted by copepods fed with the <20-μm size-fraction diet, compared to the DFAAs excreted by copepods fed with the 20–150-μm size-fraction diet, in which serine (SER) was accumulated (Figure 2A). During the spring experiment, the higher excretion rate was found to be related to glutamic acid (GLU) production in both treatments, but this was three times higher when copepods were fed with the <20-μm size-fraction diet (Figure 2B).
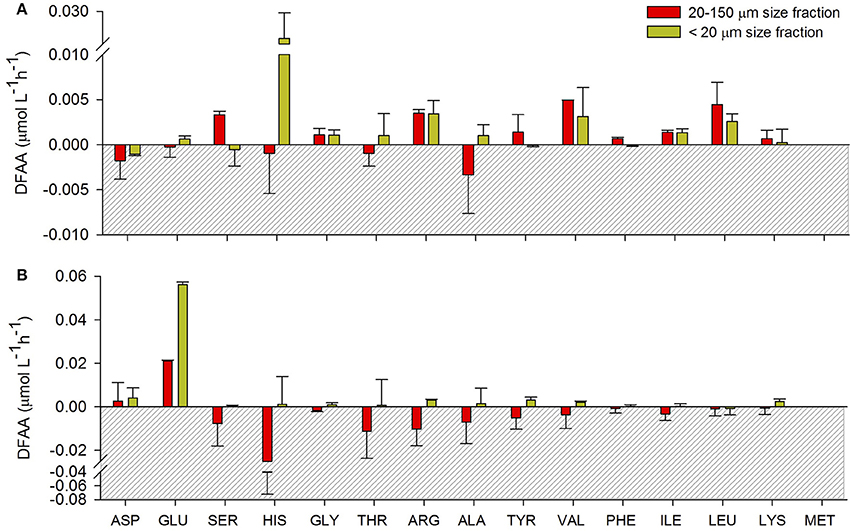
Figure 2. Excretion rates obtained for aspartic acid (ASP), glutamic acid (GLU), serine (SER), histidine (HIS), glycine (GLY), threonine (THR), arginine (ARG), alanine (ALA), tyrosine (TYR), valine (VAL), phenylalanine (PHE), isoleucine (ILE), leucine (LEU), lysine (LYS), and methionine (MET) in (A) late summer and (B) spring for both treatments. Errors bars show the standard deviation (n = 4).
Despite our efforts to avoid losses of excreted nitrogen and phosphorus due to microbial metabolism during the incubation, negative excretion rates were observed in the experiments (e.g., DON; Table 2). Flow cytometry data indicated that bacterioplankton abundances were lower and constant in both the treatments and the control during late-summer incubation (27 ± 2.5 × 103 cell mL−1; Supplementary Figure 3). In spring, both the control and the treatment in which copepods were fed with the 20–150-μm size-fraction diet had lower and constant bacterioplankton abundances during all incubations (control: 299 ± 64 × 103 cell mL−1; 20–250-μm size-fraction diet: 537 ± 14 × 103 cell mL−1), but the treatment with copepods fed with the <20-μm size-fraction diet had higher abundances, exceeding the in situ conditions (2,637 ± 67 × 103 cell mL−1).
Microbial Response Phase: Turnover Rate of Nitrogen and Phosphorus Compounds
There were variable patterns of consumption and accumulation in the microbial response to enrichments with copepod excretion products from the previous phase, which were dependent on the incubation time and origin of the excretion products (Figures 3, 4, Supplementary Figures 4). In late summer (Figure 3), the microbial response was characterized by a significant accumulation of and DON at first hour of incubation compared with the other incubation periods (ANOVA and Tukey's test; P < 0.05; Supplementary Table 3), while DOP and were consumed during the first hour of incubation in the treatment amended with excretion products from the <20-μm size-fraction diet. During the second period of incubation (T1-T2), a high consumption of nitrogen and large amounts of phosphorous production occurred. During the T2-T3 incubation period, differences between the enrichment treatments were observed for almost all the nitrogen and phosphorous compounds analyzed, although these differences were not significant (ANOVA; P > 0.05). Both and were rapidly recycled, shifting from accumulation to consumption every 2 h, but only showed a significant consumption in T1-T2 compared to the initial and final periods (ANOVA and Tukey's test, P < 0.05; Supplementary Table 3).
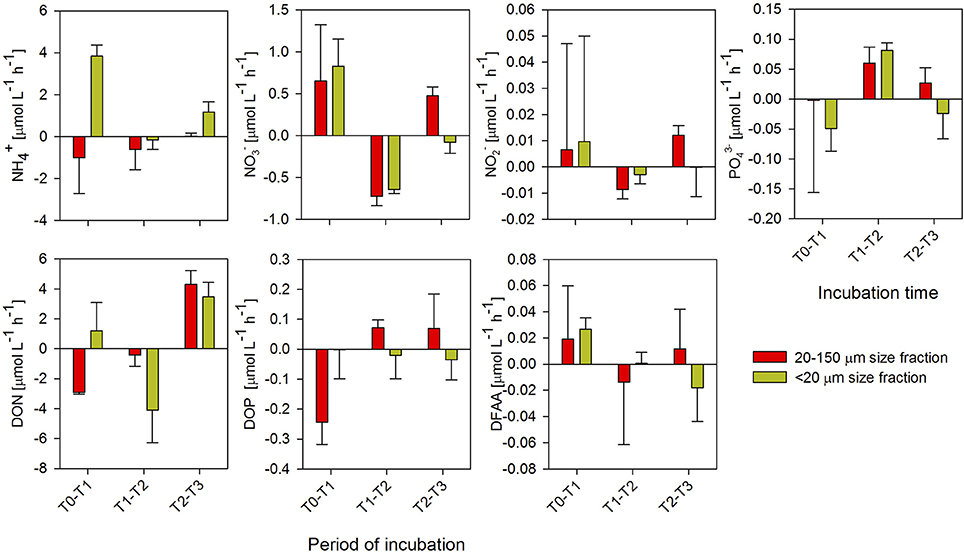
Figure 3. Turnover rates of ammonium (), nitrate (), nitrite (), phosphate (), dissolved organic nitrogen (DON), dissolved organic phosphorus (DOP), and dissolved free amino acids (DFAAs) estimated for every 2 h of incubation, during the microbial response phase in late summer. Each point represents four replicates (mean ± standard deviation).
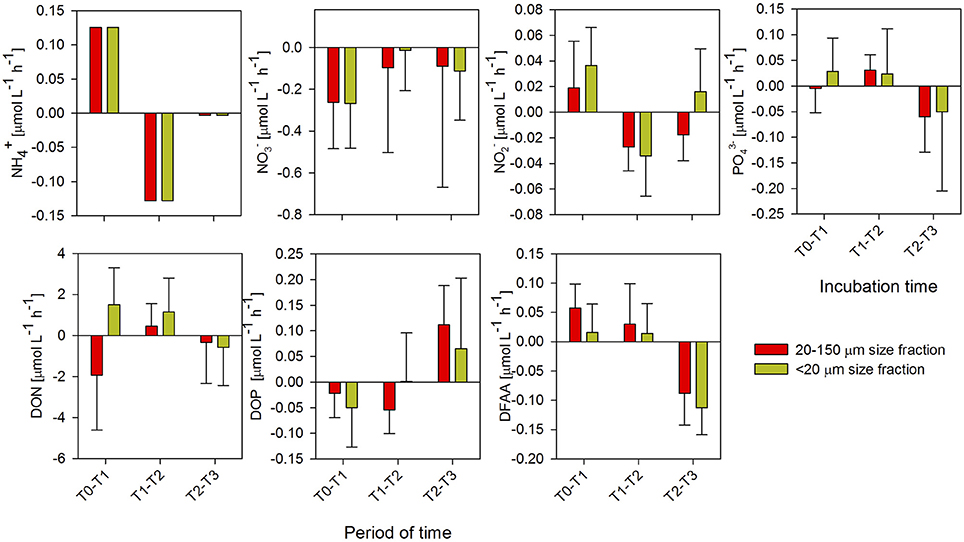
Figure 4. Turnover rates of ammonium (), nitrate (), nitrite (), phosphate (), dissolved organic nitrogen (DON), dissolved organic phosphorus (DOP), and dissolved free amino acids (DFAAs) estimated for every 2 h of incubation, during the microbial response phase in spring. Each point represents four replicates (mean ± standard deviation).
During spring (Figure 4), the only clear difference between the enrichment treatments was observed for DON, with an accumulation of DON during the first incubation time (T0-T1) in the treatment enriched with excretion products from the <20-μm size-fraction diet. However, the differences in DON between enrichment treatments were not significant (ANOVA; P > 0.05). The and DFAAs were the only compounds for which there were significant differences during the incubation in both enrichment treatments; the behavior was characterized by an accumulation during T0-T1 (Figure 4), when there were higher production rates in the treatment with the 20–150-μm size-fraction diet, and a marked consumption in the final incubation period, in both enrichment treatments (ANOVA and Tukey's test, P < 0.05; Supplementary Table 3).
A non-metric multidimensional scaling analysis (MDS) of amino acid composition from the treatments in both experiments is shown in Figure 5. In late summer, a large dissimilarity between the DFAA composition was observed, which was associated with the incubation time. The contribution of specific amino acids was analyzed based on a superimposed correlation, which revealed a strong correlation between methionine (MET) and the first period of incubation in both treatments during the late-summer experiments. During this period, in both treatments, MET was accumulated, while during the other periods it was consumed (Supplementary Figure 5). Additionally, threonine (THR) was correlated with the third time-period (T2-T3) in the treatment with the <20-μm size-fraction diet. In the spring experiment, as in late summer, the DFAA composition differed according to incubation-time treatment, but only in the first period (T0-T1, Figure 4B). The correlation analysis indicated that glycine (GLY) was associated with the treatment enriched with compounds excreted by copepods fed with the 20–150-μm size-fraction diet, and GLU was associated with the treatment derived from copepods fed with the <20-μm size-fraction diet (Figure 5 and Supplementary Figure 5).
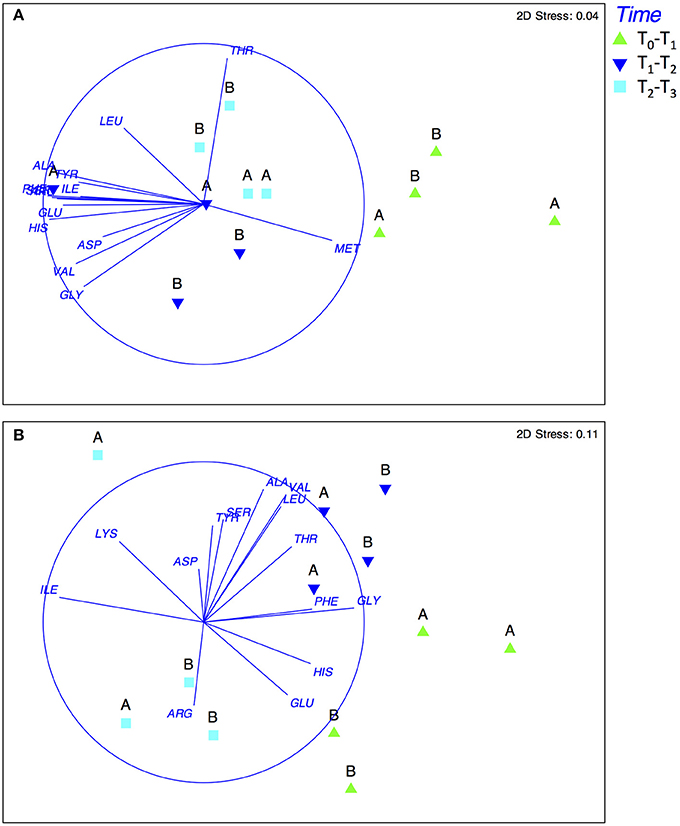
Figure 5. Non-metric multidimensional scaling analysis (MDS) of the amino acid profile based on Euclidean distance in late summer (A) and spring (B). In (A) the treatment was enriched with dissolved compounds excreted by copepods fed with a 20–150-μm size-fraction diet. In (B) the treatment was enriched with dissolved compounds excreted by copepods fed with a <20-μm size fraction diet.
The bacterioplankton abundance during the microbial response phase is presented in Supplementary Figure 6. During both experiments, bacterioplankton abundance increased throughout the incubation time, both in treatments and controls (Supplementary Figures 6A,D). In late summer, differences between the enrichment treatments and control were only observable for the treatment inoculated with compounds excreted from the 20–150-μm size-fraction diet, with lower bacterioplankton abundances being found compared to the control and to the treatment derived from copepods fed with the <20-μm size-fraction diet (t-test, P < 0.05). Cyanobacteria and picoeukaryotes were found throughout the incubation, with a high degree of variability in late summer (Supplementary Figures 6B,C) and overall higher abundances in late summer compared to spring (Supplementary Figure 6D).
Microbial Response Phase: Changes in Total and Active Bacterial Community Structure
During late summer, a total of 166,480 sequences were analyzed, ranging between 3,225 and 29,856 sequences for each library (Supplementary Figure 7A). The high number of sequences retrieved corresponded to DNA samples at the initial time (T0) in the treatment inoculated with compounds excreted by copepods fed with the <20-μm size-fraction diet. For the second experiment, in spring, a total of 113,979 sequences were analyzed, with the higher number of sequences corresponding to the sample cDNA 20–150 μm at T1 (Supplementary Figure 7B). Almost none of the rarefaction curves reached a plateau. Hence, our sequencing efforts did not completely cover the bacterial diversity. Thus, our results underestimated the alpha diversity expected in the water column; however, because the sequence data were normalized to compare libraries of different size, we were able to compare the potential response of the active community using this index throughout the experiment. The alpha diversity derived from normalized 16S rDNA libraries is shown in Figures 6C–F (late summer) and Figures 7C–F (spring) for comparison, with relevant taxa and clustering analyses. Compared with the in situ bacterial community, the taxonomic richness that was determined (i.e., OTUs) and expected based on the Chao1 index was lower in the treatments in the late-summer and spring experiments. In accordance, the Shannon index diversity showed that the bacterial community obtained in situ and at the initial time had higher values than those observed throughout the incubation in both experiments (Figures 6C,F).
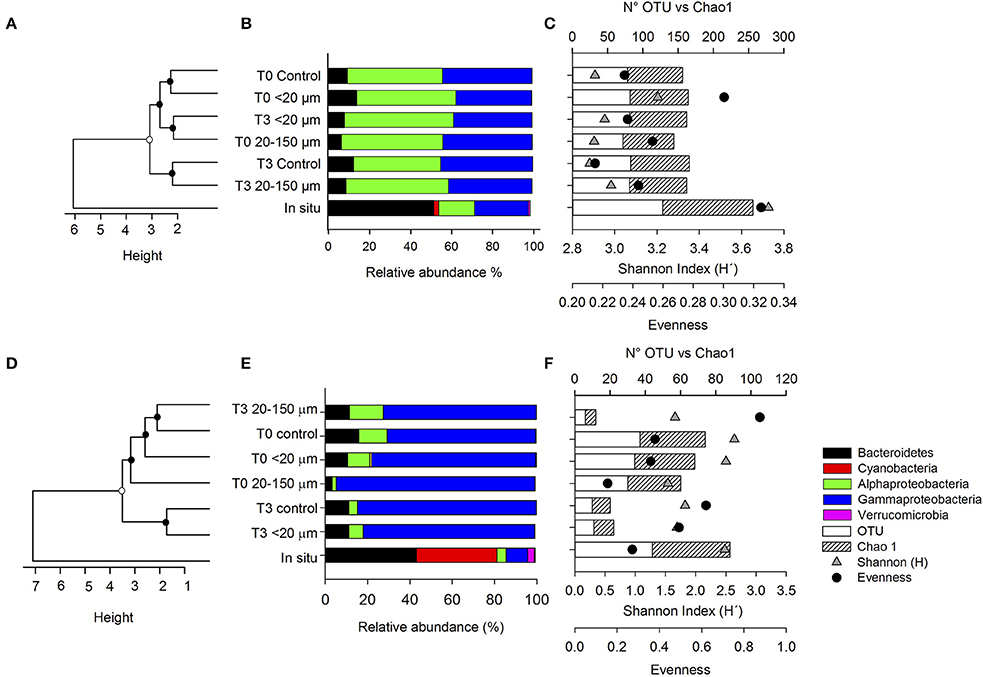
Figure 6. (A–D) Unweighted Pair Group Method with Arithmetic mean (UPGMA) clustering of the bacteria community structure in late summer and spring. Bootstrap values obtained from 1,000 interactions are depicted as circles on nodes (black: >80% and white: <70%), (B–E) taxonomic composition of abundant (>0.5%) phyla and Proteobacteria classes, comparing treatments and controls in late summer and spring, respectively and (C–F) the richness, coverage of specific libraries, Shannon (H') index, and evenness in the late summer and spring experiments.
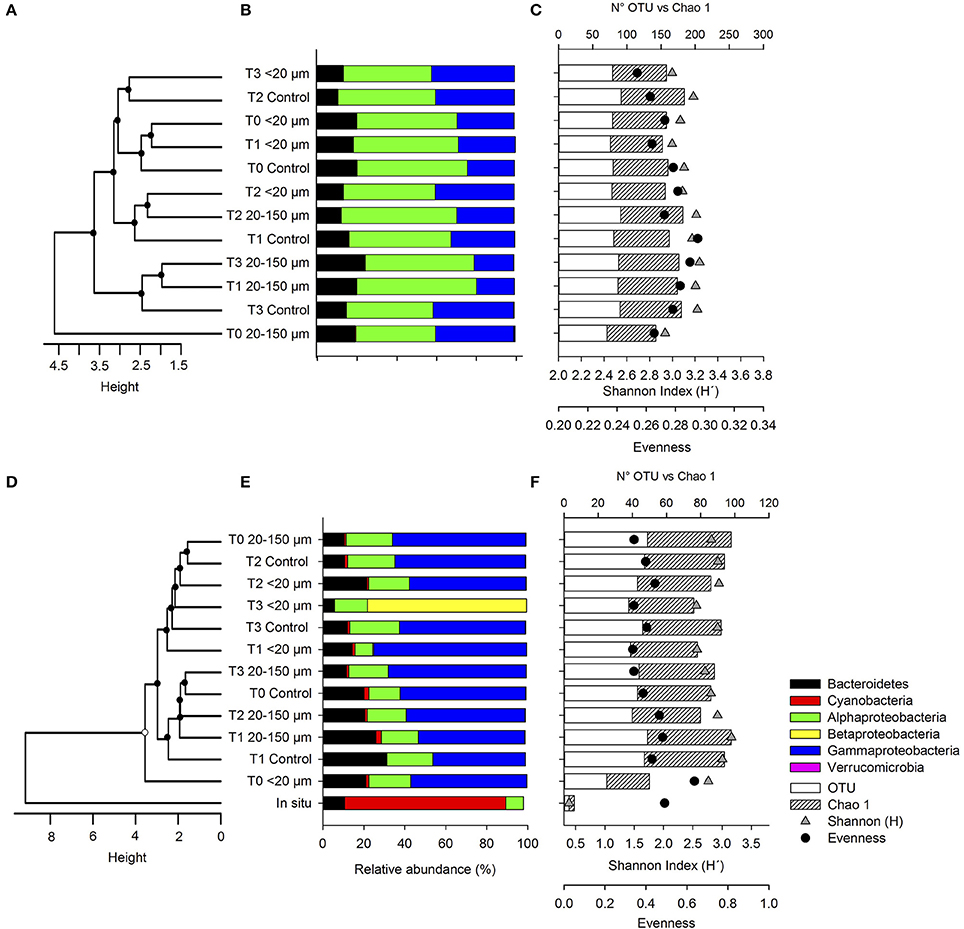
Figure 7. (A–D) Unweighted Pair Group Method with Arithmetic mean (UPGMA) clustering of active bacteria community structure in late summer and spring. Bootstrap values obtained from 1,000 interactions are depicted as circles on nodes (black: >80% and white: <70%), (B–E) taxonomic composition of abundant (>0.5%) phyla and Proteobacteria classes, comparing treatments and controls in late summer and spring, respectively and (C–F) the richness, coverage of specific libraries, Shannon (H') index, and evenness in the late summer and spring experiments.
The bacterial community composition was analyzed by comparing the relative abundances of the major phylogenetic groups (Figures 6B,E). The in situ community present at Station 18 in late summer (Figure 6B) was dominated by Bacteroidetes (51%), followed by Gammaproteobacteria (26%), Alphaproteobacteria (17%), Cyanobacteria (3%), and Verrucomicrobia (1%). A similar composition was observed at Station 12 during spring, although there was a higher contribution of Cyanobacteria (12 times higher than that during the late-summer sampling) and a lower contribution of Gammaproteobacteria (10%) and Alphaproteobacteria (4%). In both cases, these communities changed substantially during the incubation, and were characterized initially by an increase in the relative contribution of Alphaproteobacteria and Gammaproteobacteria, and a decrease in the contribution of Bacteroidetes and the disappearance of Cyanobacteria and Verrucomicrobia.
The cluster analysis associated with the contribution of the orders in the different libraries indicated high levels of dissimilarity between the in situ bacteria and the community associated with the different treatments in both experiments (Figures 6A,D). Additionally, the total bacterial community from the experiments was mainly grouped according to the incubation time, T0 vs. T3 in both experiments.
The alpha diversity derived from the normalized 16S rRNA libraries (similar sequence numbers) for comparison is shown in Figures 7A–C (late summer) and Figures 7D–F (spring), together with the relevant taxa and results of the clustering analyses. The active OTUs were slightly different between treatments and controls, with no visible trend in the diversity index (Figure 7C) during the late-summer experiments. In spring, the number of OTUs and Chao1 index values were lower under in situ conditions than in the incubations (Figure 7F). The diversity, based on the Shannon index, was lower under in situ conditions than during incubation in the spring experiment. Both the control and the treatment inoculated with dissolved compounds excreted by copepods fed with the 20–150-μm size-fraction were characterized by a decreasing trend in diversity over time. In contrast, in the treatment inoculated with dissolved compounds excreted by copepods fed with the <20-μm size-fraction diet, there was an increase in the Shannon index followed by a decrease every 2 h (Figure 7F).
The active in situ bacterial community composition was only available during the spring (Figure 7E) and was dominated mainly by Cyanobacteria (79%), followed by Bacteroidetes (11%), and Alphaproteobacteria (9%). There were substantial changes in composition throughout the incubation, which presented significant changes during the incubation. These were characterized by an increase in Bacteroidetes and Alphaproteobacteria, and a substantial decrease in Cyanobacteria (>75%). In late summer, the bacterial community was mainly represented by Alphaproteobacteria, Gammaproteobacteria, and Bacteroidetes (Figure 7B).
Cluster analysis results for the late summer (Figure 7A) indicated that the higher dissimilarities were observed at the initial time for the treatment fed with the 20–150-μm size-fraction diet and no significant differences were found between times and treatments. In contrast, in spring, more dissimilarities were observed for the in situ bacteria compared with the community associated with the different enrichment treatments (Figure 7D).
The 16S rRNA:rDNA ratio (Figure 8) showed a different response between treatments and periods. Compared to spring, in late summer (Figure 8A) a larger number of bacteria taxa increased their activity after the enrichments, particularly in relation to the excretion products from copepods fed with the 20–150-μm size-fraction diet. The activity of Betaproteobacteria and Deltaproteobacteria increased at the end of the experiment (T3), while the activity of Verrucomicrobia and Bacteroidetes decreased. In the enrichment derived from copepods fed with the <20-μm size-fraction diet, the activity of Bacteroidetes increased. Conversely, during spring (Figure 8B), a slightly higher activity of Alphaproteobacteria was observed at the end of the experiment in the treatment inoculated with compounds excreted by copepods fed with the <20-μm size-fraction diet at the end of the experiment, whereas this Proteobacteria class had a low activity in the treatment derived from copepods fed with the 20–150-μm size-fraction diet at the end of the incubation.
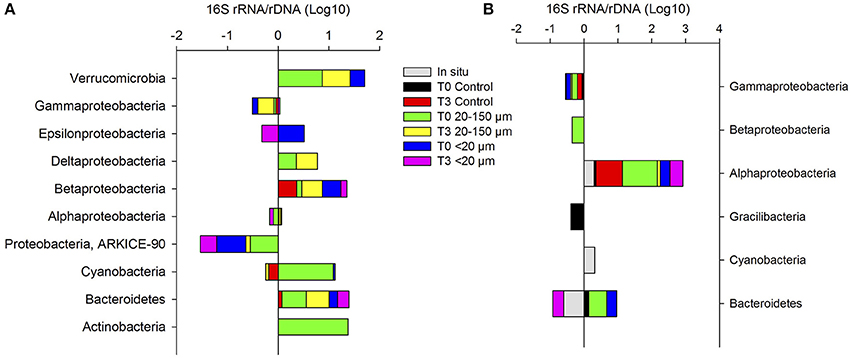
Figure 8. The 16S rRNA/rDNA ratio at the initial and final incubation times (T0-T3) for Phyla and Proteobacteria class in late summer (A) and spring (B).
The responses of the initial (in situ abundant >0.5%, semi-rare 0.5–0.1% and rare <0.1%) bacterial class to the enriched treatment compared to the controls are shown in Figures 9, 10, for late summer and spring, respectively. Considering the initial composition of the bacterial community, it was mainly the abundant and semi-rare bacteria that responded to the treatment (generally positive values after subtraction of the control), whereas rare groups were not favored by copepod enrichment products (generally negative values after subtraction of the control). The initially abundant bacteria (Figure 9) were characterized by an increase in the contribution of Alphaproteobacteria in both treatments. On the other hand, the active class had a high relative abundance of Gammaproteobacteria at T0, which decreased throughout the incubation. A large increase in Flavobacteria (Bacteroidetes) was observed in the treatment derived from copepods fed with the 20–150-μm size-fraction diet, which was also observed between T1 and T2 in the treatments derived from copepods fed with the <20-μm size-fraction diet. The semi-rare class was characterized by a large increase in the contribution of ARKICE-90 (Proteobacteria) in the treatment derived from copepods fed with the 20–150-μm size-fraction diet compared to the control, while in the treatment derived from copepods fed with the <20-μm size-fraction diet, the contribution of Betaproteobacteria showed a slight increase compared to the control. Furthermore, the active semi-rare classes in both treatments were characterized by a rapid response (2 h) of ARKICE-90 (Proteobacteria) and an increase in the relative contribution of Sphingobacteria compared to the controls. The response of the rare bacteria (in situ) to the enrichment was characterized by a higher contribution in the controls compared to the treatments (Figure 9). In addition, many rare classes were not detected in the libraries when compared to the in situ conditions, including Bacteroidetes BD2-2, Melainabacteria, derived from Gracilibacteria, Elev-16S-509 (Proteobacteria), TA18 (Proteobacteria), and the OPB35 soil group (Verrucomicrobia). The specific contribution of the orders is shown in Supplementary Figure 8.
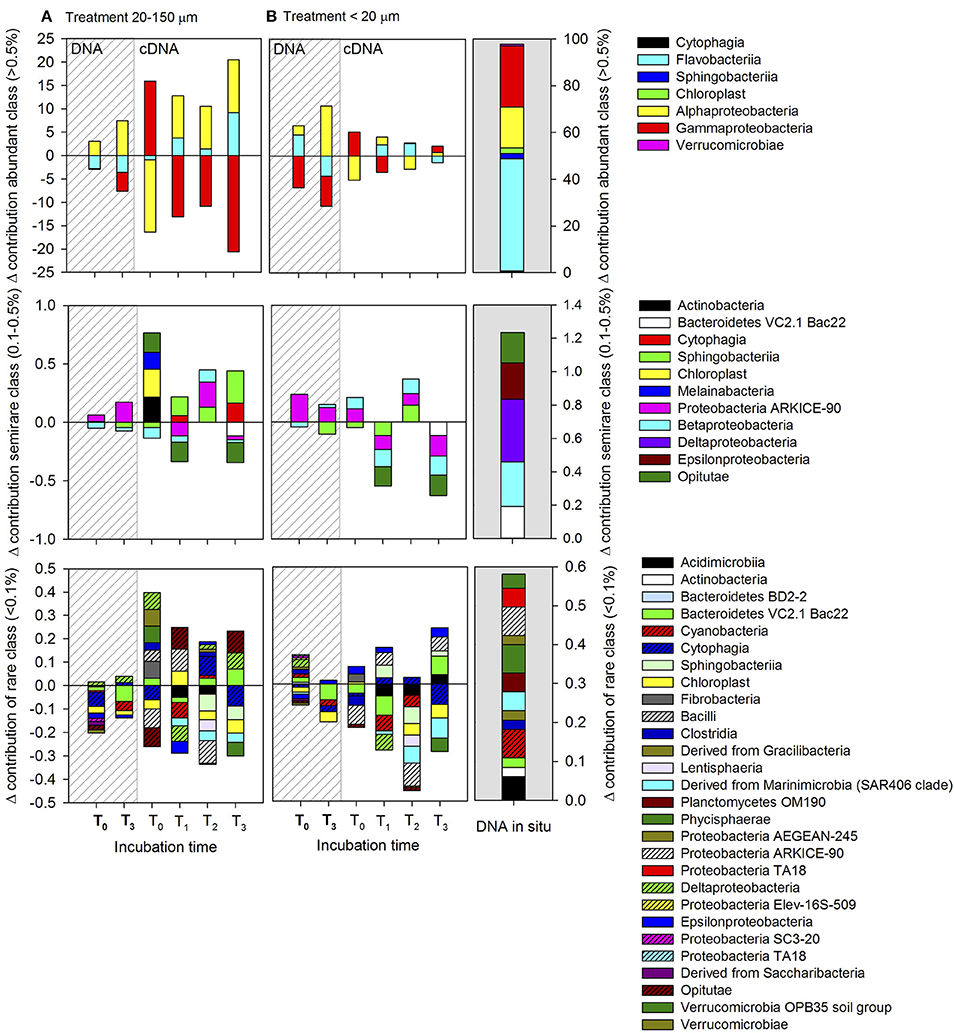
Figure 9. Abundant (>0.5%), semi-rare (0.1–0.5%), and rare (<0.1%) classes, classified according to their initial in situ contribution throughout the whole incubation period during late summer (March). The relative abundances of each class in the control were subtracted from the treatments. Positive values indicate a higher presence in treatments than in the control, and vice versa for negative values. (A) Treatment inoculated with dissolved compounds excreted by copepods fed with the 20–150-μm size-fraction diet, and (B) treatment inoculated with dissolved compounds excreted by copepods fed with the <20-μm size-fraction diet. The dashed lines indicate the composition of the total bacterial community (DNA-based).
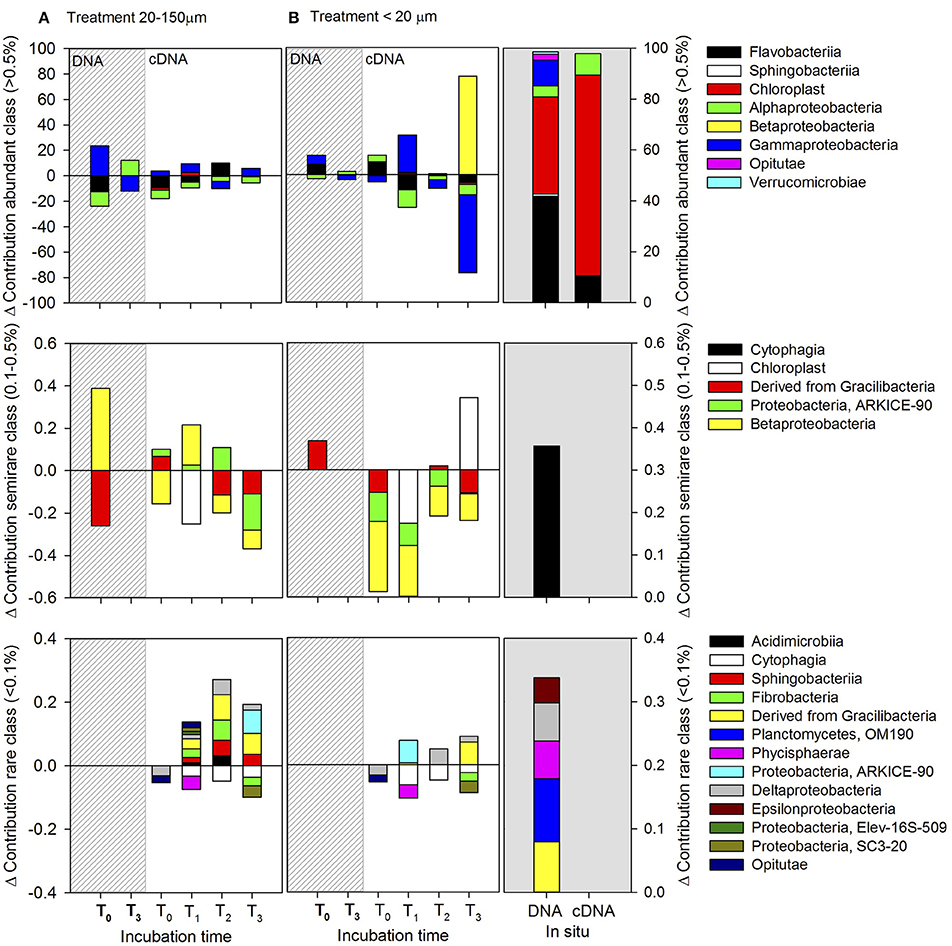
Figure 10. Abundant (>0.5%), semi-rare (0.1–0.5%), and rare (<0.1%) classes, classified according to their initial in situ contribution throughout the whole incubation period during spring (December). The relative abundances of each class in the control were subtracted from the treatments. Positive values indicate a higher presence in treatments than in the control and vice versa for negative values. (A) Treatment inoculated with dissolved compounds excreted by copepods fed with 20–150-μm size-fraction diet, and (B) treatment inoculated with dissolved compounds excreted by copepods fed with the <20-μm size-fraction diet. The dashed lines indicate the composition of the total bacterial community (DNA-based).
During spring, only abundant and semi-rare bacteria responded to the enrichment associated with copepod excretion products (Figure 10). This was characterized by an increase in the contribution of Flavobacteria and Alphaproteobacteria in the treatment inoculated with compounds excreted by copepods fed with the 20–150-μm size-fraction diet, while in the active semi-rare class there was a large increase in the contribution of Betaproteobacteria (at 2 h) and ARKICE-90 (Proteobacteria) (at 4 h). In the second treatment (<20 μm), there was a large increase in the contribution of Betaproteobacteria after 6 h of incubation. The specific contribution of each is shown in Supplementary Figure 9.
A similarity percentage analysis (SIMPER) indicated that different bacteria contributed most to the dissimilarities following the addition of excreted compounds by copepods fed with the <20 and 20–150-μm diets (Supplementary Table 3). In late summer, 20 OTUs explained 70% of the dissimilarity between the total (DNA) and active (cDNA) orders in treatments (<20 and 20–150-μm). The contributions of Alteromonadales (Gammaproteobacteria) and Flavobacteriales (Bacteroidetes) could explain many of the dissimilarities in both the DNA and cDNA pools, whereas the contribution of Rhodobacterales (Alphaproteobacteria) explained many of the dissimilarities in cDNA. During spring, the same bacteria, as well as Oceanospirillales, were identified as potential contributors, which could explain the differences between treatments (Supplementary Table 4).
In a principal coordinate analysis (PCA) plot, the two first axes explained 61.9% of the variance in the late summer experiment and 56.6% in the spring. During late summer and spring (Figures 11A,B), the active bacterial community structure within the experiment was not associated with the different treatments or times. Analysis by PERMANOVA did not identify significant differences among all samples regarding the different treatments (20–150 and <20 μm; PERMANOVA, P = 0.2 and P = 0.1) and times (PERMANOVA, P = 0.6 and P = 0.5). However, the 16S rRNA/rDNA ratio clearly differed between times and treatments (Figure 8) and the overlaid correlation analyses associated with organic and inorganic compounds, which changed throughout the experiments, indicated that the active bacterial community structure from the late incubation period was associated with the DFAA and concentration, though mainly during spring.
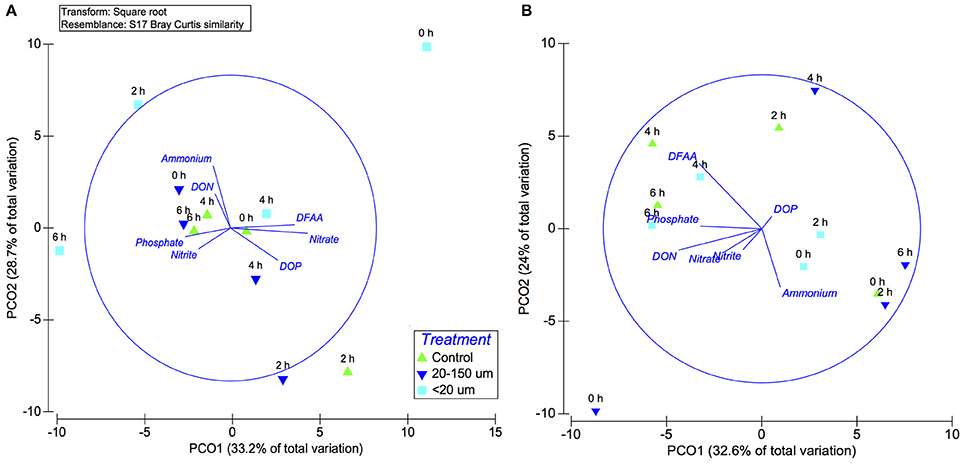
Figure 11. Principal coordinate analysis (PCA) based on Bray–Curtis similarity for the active bacterial community structure, at the order level, for different treatments and the control. (A) Late summer and (B) spring. Vectors indicate the best environmental variables (normalized transformed) correlated with ordination coordinates and vector lengths correspond to the correlation values.
Discussion
During our study, the hydrological characteristics were typical of late-summer and spring conditions (March and December, respectively), and were associated with an active upwelling period. In the study area, the upwelled water is nutrient-rich and has an oxygen deficiency, generating high photoautotrophic activity and consequently reaching high levels of primary production. However, this pattern varies throughout the upwelling period due to alongshore southerly winds during September–October, which vary over different time scales (Daneri et al., 2000; Montero et al., 2007; Sobarzo et al., 2007). In accordance with the seasonal pattern in this region (Sobarzo et al., 2007), both experiments were conducted under upwelling conditions. One was conducted during the upwelling season (December), which was close to the high solar radiation maximum (January), and the other was conducted at the end of the upwelling period (March). In fact, during late summer, , , and were found at higher concentrations compared to spring, possibly due to the higher remineralization at the end of the upwelling season. These nutrient concentrations were within the range of values previously reported by the time-series program at Station 18 (Supplementary Figure 1; Cornejo et al., 2007; Montero et al., 2007; Morales and Anabalón, 2012).
Zooplankton are mostly represented by copepods in this zone (70% of the total zooplankton abundance; Escribano et al., 2007). Acartia tonsa, P. cf indicus, and C. patagoniensis are representative species of this area, with higher abundances during spring/summer, accounting for more than 30% of the relative copepod abundance (Escribano et al., 2007; Hidalgo et al., 2010). In contrast, Spinocalanus sp. is not a species commonly found at this site, and has been reported as inhabiting deeper waters (>1,000-m depth) in the western North Pacific (Ikeda et al., 2006). However, in our study this species was present with a high abundance in shallow waters, and was a representative species for this particular sampling event.
Excretion of Nitrogen and Phosphorus Compounds
During our study, the copepod excretion products were associated with the different size fractions of food provided, influencing the type and magnitude of inorganic and organic compound excreted, including the specific composition of DFAAs. When the copepods were fed with the 20–150-μm size-fraction diet a higher accumulation of nitrogen and phosphorus compounds was observed at higher excretion rates, mainly in the form of and DOP, compared with copepods fed with the <20-μm size-fraction diet. Studies of nitrogen excretion in this study area have shown that the main product excreted by copepods in spring and winter is DON (Pérez-Aragón et al., 2011), although DON excretion was six times higher in winter than spring. In the same study, production was not detected in spring, in contrast with our study, in which was the main compound excreted by copepods. Additionally, the excretion rates observed in the current study were higher than those reported in this area and were also in the range of those reported in other upwelling zones (Fernández-Urruzola et al., 2016; Kiko et al., 2016).
The type and magnitude of the excretion products observed in our experiment compared with previous investigations in the study area and other upwelling zones could be related to the type of diet the copepods have. In the study area, copepods are capable of shifting their diet throughout the year, feeding on heterotrophic flagellates in winter and on chain-forming diatoms in spring (Vargas et al., 2006, 2010). During our study, which was conducted in spring and summer, the prevailing food available for copepods was microplankton, mainly diatoms, followed by heterotrophic nanoplankton (Table 1). The effect of diet on the main excreted compounds has been studied by several authors (Miller and Glibert, 1998; Miller and Roman, 2008; Saba et al., 2009), and it has been reported that copepods excrete more when fed on a diet based on diatoms (Miller and Roman, 2008). Regarding the potential effects of food quantity on the excretion products, it may be possible that size-fractioned food can result in a variable biomass content of phytoplankton. However, studies in this upwelling zone have shown that the biomass in terms of the carbon content is similar for the <20 and 20–150-μm size-fractions (Vargas et al., 2006, 2010).
Organic and inorganic phosphorus excretion has been less extensively studied than that of nitrogen compounds. Our results indicate that DOP was the main product excreted, accounting for 93% of the total dissolved phosphorus under late summer conditions in copepods fed with the 20–150-μm size-fraction. These results were in agreement with Johannes (1964), who reported that one-third of soluble phosphorus released by zooplankton (amphipods) was in the organic form. The same pattern was found by Satomi and Pomeroy (1965), who found that a large proportion (60%) of the phosphorus excreted by zooplankton was in the organic form. It has recently been shown that the prevailing compound being excreted by copepods is strongly dependent on the food consumed, with DOP release only being detectable in diets containing microzooplankton (Saba et al., 2009). However, these results include the nutrients released by sloppy feeding and leaching of fecal pellets, which are two other ways in which zooplankton can release dissolved compounds (Saba et al., 2011). Due to the large variability of these findings and the scarce information available, more studies of phosphorus release by zooplankton are necessary.
During our study, the specific composition of DON associated with DFAAs was determined, indicating shifts in the profile and magnitude between the treatment fed with the <20- and 20–150-μm size-fraction diets, mainly during spring. The information available about the composition of DFAAs is scarce and they are considered secondary excretory products. They account for between 5 and 48% of the total dissolved nitrogen excreted (Steinberg and Saba, 2008). The composition of the DFAA in the copepod excretion products was also variable during our experiments, with higher excretion rates in the treatment with copepods fed with the <20-μm size-fraction diet. In the late-summer experiment, the predominant amino acid excreted was HIS, while in spring it was GLU. It has been reported that GLY and alanine (ALA) are the predominant amino acids excreted, while cysteine (CYS) was absent in almost all estimations (Webb and Johannes, 1967), in contrast with our results in which ALA was excreted, but at low rates, and MET was absent in both experiments (Figure 2). As with phosphorus, there are few studies available regarding DFAA excretion. Thus, further studies are required to improve our understanding of surface nitrogen and phosphorus turnover.
Negative excretion rates were found during the excretion phase, mostly for organic compounds (DON, DOP, and DFAAs). These negative rates indicate that the consumption was higher than the input provided to the copepods. The presence of these negative rates for DFAAs suggested a significant uptake of certain DFAAs. For example, in the late-summer experiment, a higher consumption of ALA and aspartic acid (ASP) was observed when copepods were fed with the 20–150-μm and <20-μm size-fraction diets, respectively. In contrast, in the spring experiment, a higher consumption of almost all DFAAs was observed in copepods fed with the 20–150-μm size-fraction diet, with the highest consumption rate for HIS (0.05 μmol L−1 h−1). This could reflect the high lability of these compounds, which should be easily assimilated by microbial communities. Despite the attempts to minimize bacterioplankton abundance in the excretion phase, i.e., filtering the seawater to <0.2 μm, bacterioplankton were present (Supplementary Figure 3), and could rapidly use these compounds.
Potential Fate of Dissolved Organic and Inorganic Compounds Derived from Copepod Excretion
The excretion products of copepods generated changes in the composition of the active bacterial community, and the extent of organic and inorganic nitrogen and phosphorus recycling. Our results suggested a rapid recycling of all nitrogen and phosphorus compounds, shifting from accumulation to consumption (or vice versa) after every 2 h of incubation. In the late-summer experiment, was consumed throughout the incubation in the treatment with copepods fed with the 20–150-μm size-fraction diet. DON, , and were accumulated toward the final period of the experiment. These results suggest a coupled uptake–remineralization process of and DON, which is probably associated with heterotrophic microbial activities. The and accumulation in the treatment with copepods fed with the 20–150-μm size-fraction diet suggested that nitrification processes occurred during the incubations. This was expected considering the previous findings of a tight coupling between excretion and ammonia oxidation (Molina et al., 2005, 2012) and the functional microbial groups involved (Valdés et al., 2017). During the late summer experiment, the treatment with copepods fed with the <20-μm size-fraction diet was characterized by a high consumption of nitrogen compounds between the initial period and 4 h of incubation, with an and DON accumulation in the final period. These results suggest a high bacterioplankton demand for DON remineralization/ammonification. This resulted in the accumulation of at the end of the experiment, which was not present in the treatment with copepods fed with the 20–150-μm size-fraction diet.
In addition, the DOM quality, evaluated by changes in the DON:DOP ratio (Supplementary Figure 10), could influence the microbial response to copepod excretion product enrichments. During spring, copepod excretion products contained a higher quality of DOM compared with late summer, generating a high turnover and consumption of DFAAs during the microbial response phase. This result supports the relevance of DFAAs as a relatively small but highly labile pool of DOM, which can support a large fraction of bacterial production in the ocean (Nagata, 2000). Moreover, the specific composition of DFAAs also indicated differences in the microbial response phase associated with the enrichment treatments. MET was the only amino acid accumulated during the first hour of incubation in both treatments, whereas THR was accumulated in the treatment enriched with compounds derived from copepods fed with the <20-μm size-fraction diet. DFAA concentrations are low in the marine system and their turnover can be quite fast, e.g., <30 min (Fuhrman, 1987). Our results showed that GLU and HIS were actively used by microbial communities at a low rate in late summer compared to spring, when the concentration throughout the incubation was higher and the uptake of DFAAs was mainly THR and HIS.
In relation to phosphorus excretion and use, our results showed that DOP in the treatment inoculated with compounds excreted by copepods fed with the 20–150-μm size-fraction diet in the late-summer experiment had high consumption rates during the first 2 h, while for the inorganic form there was a higher accumulation between T1-T2. However, the estimated rate of DOP consumption was four times higher than that of production. The same pattern was found in the treatment derived from copepods fed with the <20-μm size-fraction diet, but the difference between the DOP consumption and accumulation rates was close to zero, with negative DOP consumption rates during all incubations. In addition, a lower DOP concentration and rate of change over time were observed in late summer than in spring. This could be in response to the quality of the food provided to copepods. A coupling between uptake and DOP production was also observed at the end of the incubation for both treatments. There was an accumulation of in the first 4 h of incubation, while at the same time DOP was consumed. However, in the final 2 h (T2-T3) was consumed at a similar rate at which DOP was produced (Figure 3). In accordance with our results, the rapid utilization of phosphorus compounds (less than a few hours), and the coupled DOP production–utilization had been reported by other studies using labeled phosphorus in experiments in marine environments (Dolan et al., 1995; Lovdal et al., 2007).
Bacterial Response to the Copepod Excretion Products
During our experiment, we determined the response of the microbial community in terms of any changes in abundance and specific bacterial composition. Increases in the abundance of bacterioplankton communities were predominantly associated with the <20-μm enrichment, and this was particularly true for the spring experiment, in which there was an increase of ~400 × 103 cell mL−1 between T0 and T3. These results are in agreement with a recent study of the addition of compounds excreted by krill to bacterial assemblages, which showed that they stimulated bacterial growth and production in terms of biomass production, oxygen consumption, and growth (Arístegui et al., 2014).
In this study area, Bacteroidetes, Proteobacteria, Cyanobacteria, and Verrucomicrobia were the most abundant total (DNA) and active (RNA) phyla detected in situ during both sampling periods, and in late summer they were mostly represented by Flavobacteria, Gammaproteobacteria, Alphaproteobacteria, Sphingobacteria, Chloroplast, Cytophagia, and Verrucomicrobiae. In spring, all these classes were detected, with a greater contribution by Cyanobacteria and a smaller contribution by Gammaproteobacteria and Alphaproteobacteria, compared to late summer. Our results were in agreement with surface water diversity surveys in this study area based on metagenomics and an analysis of Station 18 during upwelling conditions (Murillo et al., 2014), and also the results of another study based on active bacteria 16S rRNA (Levipan et al., 2016).
The PERMANOVA results did not reveal significant differences between the treatments derived from copepods fed with the different size-fractionated diets. However, there were differences in the response of the composition of active bacteria to the compounds excreted by copepods based on the changes in their relative contribution, 16S rRNA:rDNA ratio, and the SIMPER analysis results. This suggests that the bacterial degradation of dissolved compounds is conducted by phylogenetically diverse communities, some generalist and others more specialized, that are associated with the upwelling periods. In the late-summer experiment, the treatment inoculated with dissolved compounds excreted by copepods fed with the 20–150-μm size-fraction diet had a higher contribution from Alphaproteobacteria in the first hour of incubation. This was mainly Rhodobacterales, which together with Flavobacteriales from the Bacteroidetes phyla, are very active bacterial communities. In the enrichment derived from copepods fed with the <20-μm size-fraction diet, Alphaproteobacteria were responsible for the differences found among treatment enrichments and controls during late summer. In contrast, in the spring experiment, Alphaproteobacteria were active in the enrichment derived from copepods fed with the <20-μm size-fraction diet, but were inhibited in the enrichment derived from copepods fed with the 20–150-μm size-fraction diet. A high 16S rRNA:rDNA ratio was also observed in spring. Alphaproteobacteria are aerobic heterotrophs that preferentially use monomers, such as amino acids and N-acetylglucosamine, and are therefore important competitors for amino acids in the ocean (Cottrell and Kirchman, 2000). In addition, copepods fed with the <20-μm size-fraction diet had higher DFAA excretion rates compared to the copepods fed with the 20–150-μm size-fraction diet, and during the microbial response phase these treatments were associated with THR in late summer, and with GLU and arginine (ARG) in spring. These results suggest that the response of microbial communities was more strongly associated with the quality of the food provided than with the amount of the compounds excreted, e.g., DON.
The uptake of specific DOM compounds by marine microorganisms has been studied by microautoradiography combined with in situ hybridization (MAR-FISH), and several studies have reported different uptake patterns of simple, low-molecular-weight compounds (Ouverney and Fuhrman, 1999; Cottrell and Kirchman, 2000; Vila et al., 2004). These results have demonstrated that Alphaproteobacteria are responsible in large part for the uptake of low-molecular-weight DOM, while Bacteroidetes are more specialized in the uptake of high-molecular-weight DOM. Alonso-Saez and Gasol (2007) demonstrated that DFAAs were readily taken up by Alphaproteobacteria and Gammaproteobacteria, but not by Bacteroidetes. Furthermore, the same authors showed that ATP was consumed in high proportions by Alphaproteobacteria, Gammaproteobacteria, and Bacteroidetes. Our experiment was conducted in late summer, and was characterized by the presence of complex structural biopolymers (cell wall components) associated with the large size (1.6 μm) of the organisms in the microbial community. The decay of this community by predation or autolysis could provide complex substrates in our incubation, enhancing the activity of Bacteroidetes and other groups, including semi-rare classes (e.g., Betaproteobacteria). There were differences in the 16S rRNA:rDNA ratio between treatments. Betaproteobacteria (semi-rare) were significantly active at the end of the experiment with copepods fed with the 20–150-μm size-fraction diet in late summer. Betaproteobaceria (e.g., Bourkholderiales, Hydrogenophilales, and Methylophilaceae) are commonly found in productive coastal waters (Rappé et al., 2000), including the area studied here (Levipan et al., 2016), and some are methylotrophs that can use C1 compounds, such as methanol, as a source of carbon and energy. Moreover, in the treatments derived from copepods fed with the <20-μm size-fraction diet, Bacteroidetes were active at the end of the incubation, and were related to the Flavobacteria. This group is one the most abundant phyla in coastal areas, where they represent between 10 and 30% of the total relative abundance of the bacterial community (Alonso-Saez and Gasol, 2007). They are able to degrade complex organic matter and are specialized in the degradation of biopolymers, such as proteins (Pinhassi et al., 1999; Teeling et al., 2012; Fernández-Gómez et al., 2013).
However, many groups were shifted during the incubation, including Gammaproteobacteria, which are mainly associated with the Alteromonadales, Cellvibrionales, and Oceanospirillales orders, particularly during spring. These groups are considered to be opportunistic bacteria in surface waters, and they responded rapidly to the enrichment derived from copepod excretion. Several studies have suggested that enrichment experiments tend to enhance the abundance of microorganisms rarely found in nature, but that have opportunistic and copiotrophic qualities that allow them to rapidly adapt to changes in environmental conditions, outcompeting abundant groups in the field (Nelson and Wear, 2014; Pedler et al., 2014; Logue et al., 2016). Lauro et al. (2009) argued that copiotroph bacteria have a higher genetic potential to sense and rapidly respond to a sudden nutrient influx, compared to the more widely distributed oligotrophic bacteria. Stocker et al. (2008) demonstrated that bacteria can gain growth advantages if they can exploit ephemeral nutrient patches, for example, those originating from phytoplankton photosynthetic products, or cell lysiate and organic matter leaking from particles and zooplankton excretion.
Conclusion
We conclude that and DOP are the main compounds excreted by the copepod community in the coastal upwelling zone of central Chile under active upwelling conditions. Our data also suggests a direct relationship between and DOP excretion and the dominant food available to copepods during this season (i.e., microplankton in the 20–150-μm size-fraction), but this is not true for DFAAs.
Our results suggest a tightly coupled remineralization and uptake, potentially linked to the assimilation process over short response times (<2 h), and particularly associated with the <20-μm size fraction. The results suggest that the response of microbial communities is associated with the quality of the compounds provided by copepod excretion. The response was characterized by a high turnover of DFAAs and the contribution of active typical surface water fast-responsive bacteria to the new source of organic matter available during late summer and spring, i.e., Alphaproteobacteria and Bacteroidetes, respectively.
Further studies of organic excretion (e.g., DOP and DFAA) and the design of experiments to explore the use of specific compounds released by zooplankton by the bacterial communities will provide valuable insights into the role of zooplankton in fueling the microbial loop.
Author Contributions
VV, CF, RE, and VM designed the experiment setup. VV, CF, and RE carried out the sample collection, incubation experiments, and sample analysis. VV and VM participated in the molecular analyses. FJ assist in paper writing and data analyses. VV wrote the paper with equal contribution of all co-authors.
Conflict of Interest Statement
The authors declare that the research was conducted in the absence of any commercial or financial relationships that could be construed as a potential conflict of interest.
Acknowledgments
The authors are grateful to Claudia Muñoz and Katherine Aniñir for assistance during experiments and the analysis of samples. This work was supported by the Comisión Nacional de Investigación Científicas y Tecnológicas (CONICYT) through Grants FONDECYT No 1130511 and 1150891, FONDAP grants IDEAL 15150003 and INCAR (15110027), Instituto Milenio de Oceanografía (IMO) Grant IC120019 and CONICYT PIA PFB-31 Copas Sur austral program. The work was developed in the frame of LIA MORFUN and it is a contribution to PROMO ECOS CONICYT Project, ECOS 12026. VV was funded by CONICYT Scholarship.
Supplementary Material
The Supplementary Material for this article can be found online at: https://www.frontiersin.org/articles/10.3389/fmars.2017.00343/full#supplementary-material
References
Alcaraz, M., Almeda, R., Calbet, A., Saiz, E., Duarte, C. M., Lasternas, S., et al. (2010). The role of arctic zooplankton in biogeochemical cycles: respiration and excretion of ammonia and phosphate during summer. Polar Biol. 33, 1719–1731. doi: 10.1007/s00300-010-0789-9
Alonso-Saez, L., and Gasol, J. M. (2007). Seasonal Variations in the Contributions of Different Bacterial Groups to the Uptake of Low-Molecular-Weight Compounds in Northwestern Mediterranean Coastal Waters. Appl. Environ. Microbiol. 73, 3528–3535. doi: 10.1128/AEM.02627-06
Arístegui, J., Duarte, C. M., Reche, I., and Gómez-Pinchetti, J. L. (2014). Krill excretion boosts microbial activity in the Southern Ocean. PLoS ONE 9:e89391. doi: 10.1371/journal.pone.0089391
Bidigare, R. R. (1983). “Nitrogen excretion by marine zooplankton,” in Nitrogen in the Marine Environment, eds E. J. Carpenter and D. G. Capone (New York, NY: Academic Press), 385–409.
Blanchet, M., Pringault, O., Bouvy, M., Catala, P., Oriol, L., Caparros, J., et al. (2015). Changes in bacterial community metabolism and composition during the degradation of dissolved organic matter from the jellyfish Aurelia aurita in a Mediterranean coastal lagoon. Environ. Sci. Pollut. Res. 22, 13638–13653. doi: 10.1007/s11356-014-3848-x
Bronk, D. A., See, J. H., Bradley, P., and Killberg, L. (2007). DON as a source of bioavailable nitrogen for phytoplankton. Biogeosciences 4, 283–296. doi: 10.5194/bg-4-283-2007
Calbet, A. (2001). Mesozooplankton grazing effect on primary production: a global comparative analysis in marine ecosystems. Limnol. Oceanogr. 46, 1824–1830. doi: 10.4319/lo.2001.46.7.1824
Campbell, B. J., and Kirchman, D. L. (2013). Bacterial diversity, community structure and potential growth rates along an estuarine salinity gradient. ISME J. 7, 210–220. doi: 10.1038/ismej.2012.93
Carlson, C. A., and Hansell, D. A. (2015). “DOM sources, sink, reactivity and budgets,” in Biogeochemestry of Marine Dissolved Organic Matter, eds C. A. Carlson and D. A. Hansell (Boston: Academic Press), 66–109.
Conover, R. J., and Gustavson, K. R. (1999). Sources of urea in arctic seas: zooplankton metabolism. Mar. Ecol. Prog. Ser. 179, 41–54. doi: 10.3354/meps179041
Cornejo, M., Farías, L., and Gallegos, M. (2007). Seasonal cycle of N2O vertical distribution and air–sea fluxes over the continental shelf waters off central Chile (36°S). Prog. Oceanogr. 75, 383–395. doi: 10.1016/j.pocean.2007.08.018
Cottrell, M. T., and Kirchman, D. L. (2000). Natural assemblages of marine proteobacteria and members of the Cytophaga-Flavobacter cluster consuming low-and high-molecular-weight dissolved organic matter. Appl. Environ. Microbiol. 66, 1692–1697. doi: 10.1128/AEM.66.4.1692-1697.2000
Daneri, G., Dellarossa, V., Qui-ones, R., Jacob, B., Montero, P., and Ulloa, O. (2000). Primary production and community respiration in the Humboldt Current System off Chile and associated oceanic areas. Mar. Ecol. Prog. Ser. 197, 41–49. doi: 10.3354/meps197041
Dolan, J. R., Thingstad, T. F., and Rassoulzadegan, F. (1995). Phosphate tranfer between microbial size-fractions in Villefranche Bay (N. W. Mediterranean Sea), France in autumn 1992. Ophelia 41, 71–85. doi: 10.1080/00785236.1995.10422038
Edgar, R. C., Haas, B. J., Clemente, J. C., Quince, C., and Knight, R. (2011). UCHIME improves sensitivity and speed of chimera detection. Bioinformatics. 27, 2194–2200. doi: 10.1093/bioinformatics/btr381
Escribano, R., Hidalgo, P., and Krautz, C. (2009). Zooplankton associated with the oxygen minimum zone system in the northern upwelling region of Chile during March 2000. Deep Sea Res. II Top. Stud. Oceanogr. 56, 1083–1094. doi: 10.1016/j.dsr2.2008.09.009
Escribano, R., Hidalgo, P., González, H., Giesecke, R., Riquelme-Bugue-o, R., and Manríquez, K. (2007). Seasonal and inter-annual variation of mesozooplankton in the coastal upwelling zone off central-southern Chile. Prog. Oceanogr. 75, 470–485. doi: 10.1016/j.pocean.2007.08.027
Fernández-Gómez, B., Richter, M., Schüler, M., Pinhassi, J., Acinas, S. G., González, J. M., et al. (2013). Ecology of marine Bacteroidetes: a comparative genomics approach. ISME J. 7, 1026–1037. doi: 10.1038/ismej.2012.169
Fernández-Urruzola, L., Osma, N., Packard, T. T., Maldonado, F., and Gómez, M. (2016). Spatio-temporal variability in the GDH activity to ammonium excretion ratio in epipelagic marine zooplankton. Deep Sea Res. Part Oceanogr. Res. Pap. 117, 61–69 doi: 10.1016/j.dsr.2016.09.005
Fuhrman, J. (1987). Close coupling between release and uptake of dissolved free amino acids in seawater studied by an isotope dilution approach. Mar. Ecol. Prog. Ser. 37, 45–52. doi: 10.3354/meps037045
Grasshof, K. (1983). “Determination of nutrients,” in Methods of Seawater Analysis, eds K. Grasshoff, M. Ehrhard, and K. Kremling (Florida: Verlag Chemie), 125–187.
Hidalgo, P., Escribano, R., Vergara, O., Jorquera, E., Donoso, K., and Mendoza, P. (2010). Patterns of copepod diversity in the Chilean coastal upwelling system. Deep Sea Res. II Top. Stud. Oceanogr. 57, 2089–2097. doi: 10.1016/j.dsr2.2010.09.012
Holmes, R. A., Aminot, R., Kérouel, B., Hooker, B., and Peterson (1999). A simple and precise method for measuring ammonium in marine and freshwater ecosystems. Can. J. Fish. Aquat. Sci. 56, 1801–1808 doi: 10.1139/f99-128
Ikeda, T., Kanno, Y., Ozaki, K., and Shinada, A. (2001). Metabolic rates of epipelagic marine copepods as a function of body mass and temperature. Mar. Biol. 139, 587–596. doi: 10.1007/s002270100608
Ikeda, T., Sano, F., Yamaguchi, A., and Matsuishi, T. (2006). Metabolism of mesopelagic and bathypelagic copepods in the western North Pacific Ocean. Mar. Ecol. Prog. Ser. 322, 199–211. doi: 10.3354/meps322199
Johannes, R. E. (1964). Uptake and release of dissolved organic phosphorus by representatives of a coastal marine ecosystem. Limnol Ocean. 9, 224–234. doi: 10.4319/lo.1964.9.2.0224
Kiko, R., Hauss, H., Buchholz, F., and Melzner, F. (2016). Ammonium excretion and oxygen respiration of tropical copepods and euphausiids exposed to oxygen minimum zone conditions. Biogeosci. Discuss. 12, 17329–17366. doi: 10.5194/bgd-12-17329-2015
Landa, M., Cottrell, M., Kirchman, D., Blain, S., and Obernosterer, I. (2013). Changes in bacterial diversity in response to dissolved organic matter supply in a continuous culture experiment. Aquat. Microb. Ecol. 69, 157–168. doi: 10.3354/ame01632
Lauro, F. M., McDougald, D., Thomas, T., Williams, T. J., Egan, S., Rice, S., et al. (2009). The genomic basis of trophic strategy in marine bacteria. Proc. Natl. Acad. Sci. U.S.A. 106, 15527–15533. doi: 10.1073/pnas.0903507106
Levipan, H. A., Molina, V., and Fernandez, C. (2014). Nitrospina -like bacteria are the main drivers of nitrite oxidation in the seasonal upwelling area of the Eastern South Pacific (Central Chile ~36°S): Nitrite-oxidizing bacteria in the seasonal upwelling system off Concepcion. Environ. Microbiol. Rep. 6, 565–573. doi: 10.1111/1758-2229.12158
Levipan, H. A., Molina, V., Anguita, C., Rain-Franco, A., Belmar, L., and Fernandez, C. (2016). Variability of nitrifying communities in surface coastal waters of the Eastern South Pacific (~36°S): microbial communities in shallow coastal waters. Environ. Microbiol. Rep. 8, 851–864. doi: 10.1111/1758-2229.12448
Logue, J. B., Stedmon, C. A., Kellerman, A. M., Nielsen, N. J., Andersson, A. F., Laudon, H., et al. (2016). Experimental insights into the importance of aquatic bacterial community composition to the degradation of dissolved organic matter. ISME J. 10, 533–545. doi: 10.1038/ismej.2015.131
Lovdal, T., Tanaka, T., and Thingstad, T. F. (2007). Algal-bacterial competition for phosphorus from dissolved DNA, ATP, and orthophosphate in a mesocosm experiment. Limnol. Oceanogr. 52:1407. doi: 10.4319/lo.2007.52.4.1407
Marie, D. F., Partensky, S., Jacquet, D., and Vaulot (1997). Enumeration and cell cycle analysis of natural populations of marine picoplankton by flow cytometry using the nucleic acid stain SYBR Green I. Appl. Environ. Microbiol. 63, 186–193
McCarren, J., Becker, J. W., Repeta, D. J., Shi, Y., Young, C. R., Malmstrom, R. R., et al. (2010). Microbial community transcriptomes reveal microbes and metabolic pathways associated with dissolved organic matter turnover in the sea. Proc. Natl. Acad. Sci. U.S.A. 107, 16420–16427. doi: 10.1073/pnas.1010732107
Miller, C. A., and Glibert, P. M. (1998). Nitrogen excretion by the calanoid copepod Acartia tonsa: results of mesocosm experiments. J. Plankton Res. 20, 1767–1780. doi: 10.1093/plankt/20.9.1767
Miller, C. A., and Roman, M. R. (2008). Effects of food nitrogen content and concentration on the forms of nitrogen excreted by the calanoid copepod, Acartia tonsa. J. Exp. Mar. Biol. Ecol. 359, 11–17. doi: 10.1016/j.jembe.2008.02.016
Molina, V., Farías, L., Eissler, Y., Cuevas, L. A., Morales, C. E., and Escribano, R. (2005). Ammonium cycling under a strong oxygen gradient associated with the Oxygen Minimum Zone off northern Chile (~23 S). Mar. Ecol. Prog. Ser. 288, 35–43. doi: 10.3354/meps288035
Molina, V., Morales, C. E., Farías, L., Cornejo, M., Graco, M., Eissler, Y., et al. (2012). Potential contribution of planktonic components to ammonium cycling in the coastal area off central-southern Chile during non-upwelling conditions. Prog. Oceanogr. 92–95, 43–49. doi: 10.1016/j.pocean.2011.07.006
Møller, E. F. (2007). Production of dissolved organic carbon by sloppy feeding in the copepods Acartia tonsa, Centropages typicus, and Temora longicornis. Limnol. Oceanogr. 52, 79–84. doi: 10.4319/lo.2007.52.1.0079
Montero, P., Daneri, G., Cuevas, L. A., González, H. E., Jacob, B., Lizárraga, L., et al. (2007). Productivity cycles in the coastal upwelling area off Concepción: the importance of diatoms and bacterioplankton in the organic carbon flux. Prog. Oceanogr. 75, 518–530. doi: 10.1016/j.pocean.2007.08.013
Morales, C. E., and Anabalón, V. (2012). Phytoplankton biomass and microbial abundances during the spring upwelling season in the coastal area off Concepción, central-southern Chile: variability around a time series station. Prog. Oceanogr. 92–95, 81–91. doi: 10.1016/j.pocean.2011.07.004
Murillo, A. A., Ramirez-Flandes, S., DeLong, E. F., and Ulloa, O. (2014). Enhanced metabolic versatility of planktonic sulfur-oxidizing γ-proteobacteria in an oxygen-deficient coastal ecosystem. Front. Mar. Sci. 1:18. doi: 10.3389/fmars.2014.00018
Nagata, T. (2000). “Production mechanisms of dissolved organic matter,” in Microbial Ecology of the Oceans, ed D. L. Kirchman (New York, NY: John Wiley), 121–152.
Nelson, C. E., and Wear, E. K. (2014). Microbial diversity and the lability of dissolved organic carbon. Proc. Natl. Acad. Sci. U.S.A. 111, 7166–7167. doi: 10.1073/pnas.1405751111
Ouverney, C. C., and Fuhrman, J. A. (1999). Combined microautoradiography−16S rRNA probe technique for determination of radioisotope uptake by specific microbial cell types in situ. Appl. Environ. Microbiol. 65, 1746–1752.
Pantoja, S., and Lee, C. (2003). Amino acid remineralization and organic matter lability in Chilean coastal sediments. Org. Geochem. 34, 1047–1056. doi: 10.1016/S0146-6380(03)00085-8
Parson, T., Maita, Y., and Lalli, C. (1984). A Manual of Chemical and Biological Methods for Seawater Analysis. New York, NY: Pergamon Press.
Pedler, B. E., Aluwihare, L. I., and Azam, F. (2014). Single bacterial strain capable of significant contribution to carbon cycling in the surface ocean. Proc. Natl. Acad. Sci. U.S.A. 111, 7202–7207. doi: 10.1073/pnas.1401887111
Pedrós-Alió, C. (2012). The Rare Bacterial Biosphere. Annu. Rev. Mar. Sci. 4, 449–466. doi: 10.1146/annurev-marine-120710-100948
Pérez-Aragón, M., Fernandez, C., and Escribano, R. (2011). Nitrogen excretion by mesozooplankton in a coastal upwelling area: seasonal trends and implications for biological production. J. Exp. Mar. Biol. Ecol. 406, 116–124. doi: 10.1016/j.jembe.2011.05.029
Pinhassi, J., Azam, F., Hemphäl,ä, J., Long, R. A., Martinez, J., Zweifel, U. L., et al. (1999). Coupling between bacterioplankton species composition, population dynamics, and organic matter degradation. Aquat. Microb. Ecol. 17:13. doi: 10.3354/ame017013
Pomeroy, L. R., Mathews, H. M., and Min, H. S. (1963). Excretion of phosphate and soluble organic phosphorus compounds by zooplankton. Limnol. Oceanogr. 8, 50–55. doi: 10.4319/lo.1963.8.1.0050
Pujo-Pay, M., and Raimbault, P. (1994). Improvement of the wet-oxidation procedure for simultaneous determination of particulate organic nitrogen and phosphorus collected on filters. Mar. Ecol. Prog. Ser. 105, 203–203. doi: 10.3354/meps105203
Quast, C., Pruesse, E., Yilmaz, P., Gerken, J., Schweer, T., Yarza, P., et al. (2013). The SILVA ribosomal RNA gene database project: improved data processing and web-based tools. Nucleic Acids Res. 41, D590–D596. doi: 10.1093/nar/gks1219
Rappé, M. S., Vergin, K., and Giovannoni, S. J. (2000). Phylogenetic comparisons of a coastal bacterioplankton community with its counterparts in open ocean and freshwater systems. FEMS Microbiol. Ecol. 33, 219–232. doi: 10.1016/S0168-6496(00)00064-7
Saba, G. K., Steinberg, D. K., and Bronk, D. A. (2011). The relative importance of sloppy feeding, excretion, and fecal pellet leaching in the release of dissolved carbon and nitrogen by Acartia tonsa copepods. J. Exp. Mar. Biol. Ecol. 404, 47–56. doi: 10.1016/j.jembe.2011.04.013
Saba, G., Steinberg, D., and Bronk, D. (2009). Effects of diet on release of dissolved organic and inorganic nutrients by the copepod Acartia tonsa. Mar. Ecol. Prog. Ser. 386, 147–161. doi: 10.3354/meps08070
Sarmento, H., and Gasol, J. M. (2012). Use of phytoplankton-derived dissolved organic carbon by different types of bacterioplankton: use of phytoplankton-derived DOC by bacterioplankton. Environ. Microbiol. 14, 2348–2360. doi: 10.1111/j.1462-2920.2012.02787.x
Sarmento, H., Romera-Castillo, C., Lindh, M., Pinhassi, J., Sala, M. M., Gasol, J. M., et al. (2013). Phytoplankton species-specific release of dissolved free amino acids and their selective consumption by bacteria. Limnol. Oceanogr. 58, 1123–1135. doi: 10.4319/lo.2013.58.3.1123
Satomi, M., and Pomeroy, L. R. (1965). Respiration and phosphorus excretion in some marine populations. Ecology 46, 877–881. doi: 10.2307/1934025
Schloss, P. D., Westcott, S. L., Ryabin, T., Hall, J. R., Hartmann, M., Hollister, E. B., et al. (2009). Introducing mothur: open-Source, platform-independent, community-supported software for describing and comparing microbial communities. Appl. Environ. Microbiol. 75, 7537–7541. doi: 10.1128/AEM.01541-09
Sobarzo, M., Bravo, L., Donoso, D., Garcés-Vargas, J., and Schneider, W. (2007). Coastal upwelling and seasonal cycles that influence the water column over the continental shelf off central Chile. Prog. Oceanogr. 75, 363–382. doi: 10.1016/j.pocean.2007.08.022
Steinberg, D. K., and Saba, G. K. (2008). “Nitrogen consumption and metabolism in marine zooplankton,” in Nitrogen in the Marine Environment, ed D. G. Capone (Boston: Academic Press), 1135–1196.
Steinberg, D. K., Carlson, C. A., Bates, N. R., Goldthwait, S. A., Madin, L. P., and Michaels, A. F. (2000). Zooplankton vertical migration and the active transport of dissolved organic and inorganic carbon in the Sargasso Sea. Deep Sea Res. Part Oceanogr. Res. Pap. 47, 137–158. doi: 10.1016/S0967-0637(99)00052-7
Steinberg, D. K., Goldthwait, S. A., and Hansell, D. A. (2002). Zooplankton vertical migration and the active transport of dissolved organic and inorganic nitrogen in the Sargasso Sea. Deep Sea Res. Part Oceanogr. Res. Pap. 49, 1445–1461. doi: 10.1016/S0967-0637(02)00037-7
Stocker, R., Seymour, J. R., Samadani, A., Hunt, D. E., and Polz, M. F. (2008). Rapid chemotactic response enables marine bacteria to exploit ephemeral microscale nutrient patches. Proc. Natl. Acad. Sci. U.S.A. 105, 4209–4214. doi: 10.1073/pnas.0709765105
Teeling, H., Fuchs, B. M., Becher, D., Klockow, C., Gardebrecht, A., Bennke, C. M., et al. (2012). Substrate-controlled succession of Marine Bacterioplankton Populations Induced by a Phytoplankton Bloom. Science 336, 608–611. doi: 10.1126/science.1218344
Titelman, J., Riemann, L., Holmfeldt, K., and Nilsen, T. (2008). Copepod feeding stimulates bacterioplankton activities in a low phosphorus system. Aquat. Biol. 2, 131–141. doi: 10.3354/ab00042
Valdés, V., Fernandez, C., Molina, V., and Escribano, R. (2017). Nitrogen excretion by copepods and its effect on ammonia-oxidizing communities from a coastal upwelling zone. Limnol. Oceanogr. doi: 10.1002/lno.10629. [Epub ahead of print].
Vargas, C. A., Escribano, R., and Poulet, S. (2006). Phytoplankton food quality determines time windows for successful zooplankton reproductive pulses. Ecology 87, 2992–2999. doi: 10.1890/0012-9658(2006)87[2992:PFQDTW]2.0.CO;2
Vargas, C. A., Martínez, R. A., Escribano, R., and Lagos, N. A. (2010). Seasonal relative influence of food quantity, quality, and feeding behaviour on zooplankton growth regulation in coastal food webs. J. Mar. Biol. Assoc. U.K. 90, 1189–1201. doi: 10.1017/S0025315409990804
Vila, M., Simo, R., Kiene, R. P., Pinhassi, J., Gonzalez, J. M., Moran, M. A., et al. (2004). Use of microautoradiography combined with fluorescence in situ hybridization to determine Dimethylsulfoniopropionate Incorporation by Marine Bacterioplankton Taxa. Appl. Environ. Microbiol. 70, 4648–4657. doi: 10.1128/AEM.70.8.4648-4657.2004
Villafañe, V., and Reid, F. (1995). “Métodos de microscopía para la cuantificación de fitoplancton,” in Manual de Métodos Ecológicos, eds K. Alveal, M. Ferrario, E. Oliveira, and E. Sar (Concepción: Editorial Aníbal Pinto), 169–185.
Webb, K. L., and Johannes, R. E. (1967). Studies of the release of dissolved free amino acids by marine zooplankton. Limnol. Oceanogr. 12, 376–382. doi: 10.4319/lo.1967.12.3.0376
Keywords: zooplankton, excretion, size-fractioned diets, microbial community structure, coastal upwelling
Citation: Valdés VP, Fernandez C, Molina V, Escribano R and Joux F (2017) Dissolved Compounds Excreted by Copepods Reshape the Active Marine Bacterioplankton Community Composition. Front. Mar. Sci. 4:343. doi: 10.3389/fmars.2017.00343
Received: 23 June 2017; Accepted: 12 October 2017;
Published: 07 November 2017.
Edited by:
Christian Lønborg, Australian Institute of Marine Science, AustraliaReviewed by:
Federico Baltar, University of Otago, New ZealandTaichi Yokokawa, Japan Agency for Marine-Earth Science and Technology, Japan
Olga Sánchez, Universitat Autònoma de Barcelona, Spain
Copyright © 2017 Valdés, Fernandez, Molina, Escribano and Joux. This is an open-access article distributed under the terms of the Creative Commons Attribution License (CC BY). The use, distribution or reproduction in other forums is permitted, provided the original author(s) or licensor are credited and that the original publication in this journal is cited, in accordance with accepted academic practice. No use, distribution or reproduction is permitted which does not comply with these terms.
*Correspondence: Valentina P. Valdés, dnZhbGRlc2NAdWRlYy5jbA==