- 1Department of Plant and Environmental Sciences, University of Copenhagen, Frederiksberg, Denmark
- 2Centre National de la Recherche Scientifique/UPMC, UMR 8227, Integrative Biology of Marine Models, Station Biologique de Roscoff, CS 90074, Roscoff, France
- 3School of Agriculture, Food and Rural Development, Newcastle University, Newcastle upon Tyne, United Kingdom
Despite the biological importance and pharmacological potential of glycans from marine organisms, there are many unanswered questions regarding their distribution, function, and evolution. Here we describe microarray-based glycan profiling of a diverse selection of marine animals using antibodies raised against fucoidan isolated from a brown alga. We demonstrate the presence of two fucoidan epitopes in six animals belonging to three phyla including Porifera, Molusca, and Chordata. We studied the spatial distribution of these epitopes in Cliona celata (“boring sponge”) and identified their restricted localization on the surface of internal chambers. Our results show the potential of high-throughput screening and probes commonly used in plant and algal cell wall biology to study the diversity and distribution of glycan structures in metazoans.
Introduction
Unlike plant cells which are covered by polysaccharide-rich cell walls, most animal cells produce relatively small amounts of structural polysaccharides and these are usually distinct from those found in plants and algae. For instance, the most abundant opistokont-specific glycan polymer is chitin, forming exoskeletons of arthropods, and beaks in cephalopods (Muzzarelli, 1986). However, chitin synthesis was recently identified in vertebrates as well (Tang et al., 2015). Glycosaminoglycans (GAGs) like chondroitin or heparan sulfate are the main type of structural polysaccharides in higher animals (Esko et al., 2009). In some, relatively rare cases, animals produce homologous polymers, which are normally typical components of plant or algal cell walls. The most remarkable example is tunicin—a cellulose-like compound present in tunicates. Synthesis of tunicin in tunicates is supposedly the result of a horizontal gene transfer event dating to ~300 million years ago (Belton et al., 1989; Nakashima et al., 2004; Sagane et al., 2010).
Fucoidans or fucose-containing sulfated polysaccharides (FCSPs) are compositionally highly diverse polysaccharides containing L-fucose in their structural backbone with varying degrees of sulfation or other substitutions. Fucoidans are abundant in cell walls of brown algae (Li et al., 2008), but early work also reported presence of fucoidans in echinoderms, namely eggshells of sea urchin (Vasseur et al., 1948) and latterly also in the coat of sea cucumbers (Mulloy et al., 1994). Sulfated polysaccharides' anti-inflammatory, anticancer, and antimicrobial abilities are of a great interest in pharmaceutical research (Pereira et al., 1999; Pomin and Mourão, 2008; Ale et al., 2011; Marques et al., 2016). Despite this, relatively little is known about the fucoidan biosynthesis, precise biological functions, and their turnover in marine ecosystems (Li et al., 2008).
The Comprehensive Microarray Polymer Profiling (CoMPP) technique is a well-established semi-quantitative method used to analyse plant or plant-derived material for the presence of carbohydrates epitopes. The microprints populated with a series of extractions are then probed using a large panel of anti-glycan antibodies and the signal intensities are quantified (Moller et al., 2007). This method has been already used to analyse the origins and diversity of polysaccharides in algae and land plants (Sørensen et al., 2008, 2011; Hervé et al., 2015; Salmeán et al., 2017).
Here we investigated the possibility to use CoMPP in a similar manner to screen extractions from animal tissues. We utilized recently reported mAbs directed against the fucoidan preparation from Fucus vesiculosus (BAM series of mAbs; Torode et al., 2015) with distinct specificities to search for the presence of BAM epitopes in a selection of marine metazoans. Our approach identified several BAM1 and BAM4-positive species belonging to four phyla. Further, we characterized the cell-type specific localization of BAM1 epitope in Cliona celata (common boring sponge) and we show that this epitope is possibly not a component of aggregation factors between cells but it rather occurs within the glycocalyx covering the internal chambers.
Materials and Methods
Collection of Animal Specimens and Bioethics Statement
The collection of wild organisms from the local environment was undertaken by staff of the Marine Biological Resource Centre according to the conditions of the scientific sampling permit accorded to the Roscoff Biological Station by the Brittany Prefecture (Permit 2013-6793 awarded 13/03/2013). The animal species used are not locally endangered and were collected in minimal quantities necessary to perform the study. The use of Sepia officinalis complied with the EU directive 2010/63/EU. No vertebrates were used in this study. Sponges [Pachymatisma johnstonia Bowerbank in Johnston, 1842, Polymastia sp. Bowerbank, 1863; C. celata Grant, 1826, Tethya aurantia (Pallas, 1766), Hymeniacidon sp. Bowerbank, 1858, Axinella sp. Schmidt, 1862 and Raspailia sp. Nardo, 1833], anemones and hydras [Actinia fragacea Tugwell, 1856, Eunicella verrucosa (Pallas, 1766), Alcyonium digitatum Linnaeus, 1758 and Alcyonium glomeratum Hassall, 1843], bryozoans [Flustra foliacea (Linnaeus, 1758)], echinoderms [Asteria rubens Linnaeus, 1758 and Paracentrotus lividus (Lamarck, 1816)] were collected in the vicinity of Roscoff (France, GPS coordinates 48.72, −3.98). S. officinalis Linnaeus, 1758 and Ciona intestinalis (Linnaeus, 1767) were cultivated in Roscoff Marine Station. After collection they were extensively washed with clean sea water to remove possible contaminations or epiphytes. Finally, they were frozen for at least 48 h at −80°C to be terminated, lyophilized and were finely ground using metallic balls and tissuelyser (Quiagen), at a frequency of 27 s−1 until fine powder was obtained.
CoMPP
Alcohol insoluble residues (AIR) from samples were obtained as described in Hervé et al. (2015). Briefly, powdered materials were consecutively washed with an orbital shaker in 70% ethanol, 96% ethanol, chloroform: methanol (1:1, v/v), and acetone intercalated by spin down of materials (1,500 g, 15 min). Pellets and supernatants were kept and reserved at −80°C for further analysis. The final air-dried pellets were chemically fractionated using five sequential extractions: 2% CaCl2 at 80°C, 0.2 M HCl, 3% Na2CO3, 50 mM diamino-cyclo-hexane-tetra-acetic acid (CDTA) and 4 M NaOH with 1% v/v NaBH4 to avoid β elimination. The extractions were applied for 60 min and soft shaking (4 s−1) with a tissuelyser. Each extractions were intercalated by a centrifugation step to separate pellet from supernatant (1,500 g, 15 min). The supernatants obtained from each extraction were frozen at –80°C and lyophilized. Each lyophilized sample was re-suspended in printing buffer (55.2% glycerol, 44% water, 0.8% Triton X-100) at 10 mg/300 μl and printed onto nitrocellulose membrane with a pore size of 0.45 μm (Whatman) at 22°C and 55% humidity) using an Arrayjet Sprint microarray printers and probed as described by Moller et al. (2007) with the following monoclonal antibodies (mAbs) or carbohydrate binding modules (CBM): anti-fucoidan (BAM1, BAM2, BAM3, and BAM4), anti-alginate (BAM7 and BAM10), anti-homogalacturonan (LM19), anti-extensin (LM1), anti-arabinogalactan proteins or AGP (JIM8), anti-xyloglucan (LM25) anti-xylan (LM10), and two CBMs against cellulose/xyloglucan epitopes (CBM30 and CBM3a) (see also Table 2). Probing procedure in brief: the printed arrays were blocked 1 h in PBS (140 mM NaCl, 2.7 mM KCl, 10 mM Na2HPO4, 1.7 mM KH2PO4, pH 7.5) with 5% w/v low fat milk powder (MPBS). Then, the arrays were incubated 2 h with the aforementioned mAbs and CBMs. Probes were diluted 1:10 and 10 μg/ml, respectively, in MPBS. Afterwards, the arrays were washed thoroughly in PBS and incubated for 2 h with secondary antibodies conjugated to alkaline phosphatase, anti-rat (Jackson Immuno Research) for the mAbs (BAM1, BAM2, BAM3 and BAM4, BAM7 and BMA10, LM19, LM1, JIM8, LM10 and LM25) and anti-His for the two CBMs (Sigma-Aldrich) diluted 1:5,000 (anti-rat) or 1/1,500 (anti-His tag) in MPBS. Once washed in PBS and distilled water, microarrays were developed in a solution containing 5-bromo-4-chloro-3-indolylphosphate and nitro blue tetrazolium (Sigma-Aldrich) in alkaline phosphatase buffer (100 mM NaCl, 5 mM MgCl2, 100 mM diethanolamine, pH 9.5). The probed and developed arrays were scanned on a flatbed scanner and the heatmap was calculated as described by Hervé et al. (2015).
Immunolocalisation and Microscopy
Piece of the tissue of ~1 cm3 size surrounding the ostium was excised from the specimen. The sample was fixed in 4% formaldehyde in PBS for 1 h, washed twice with PBS and dehydrated in a series of ethanol:water solutions (30%, 70% and twice with 100%, 30 min incubation time each step) followed by clearing with HistoChoice (Sigma-Aldrich) for 24 h. The HistoChoice solution was gradually replaced with melted paraffin (Sigma-Aldrich). The embedding was performed for 2 days at 60°C. The paraffin block was sectioned on the microtome (Thermo HM355) to generate 10 μm-thick sections which were adhered to SuperFrost slides. Slides were deparaffinised in pure xylene and rehydrated in ethanol:water series (100, 96, 70 50, 10%, pure water; 5 min incubation time each step). Haematoxylin and Eosin (H&E) staining was performed according to the manufacturer instructions (Fischer Scientific). For immunolocalisation sections were individualized using PapPen, blocked with 5% skimmed milk in PBS for 30 min, probed with primary antibodies diluted in 5% milk/PBS (1:10), incubated for 1 h washed twice with PBS and incubated for 1 h with anti-rat secondary antibody conjugated with Alexa Fluor 488 diluted in 1% BSA in PBS (1:500, Sigma). Sections were washed twice with PBS, stained with 1 μM propidium iodide in PBS, washed once and mounted in CitiFluor (Agar Scientific). Laser scanning confocal microscopy (LSCM) was done on Leica SP5 equipped with argon and argon/krypton lasers. At least 3 sections were observed in 2 independent experiment observed with the same confocal settings. The pictures were processed using GIMPII software.
Results
CoMPP Profiling of Marine Animals Using Antibodies against Algal Fucoidan
For our analysis, we assembled a collection of 18 specimens of marine animals common for the Mediterranean and Northern Atlantic rim, collected from these marine environments or cultured at Station Biologique de Roscoff (Roscoff, France). The species were selected to represent a range of early emerging metazoans. Table 1 lists all analyzed species and their phylogenetic standing (according the Systema Naturae 2000). The specimens were frozen, lyophilized, homogenized and the resulting powders used to obtain alcohol insoluble residues AIR to remove non-polysaccharide components including proteins, lipids and metabolites. AIR was then fractionated by sequential extractions in the following order: (1) 2% CaCl2 at 80°C, (2) 0.2M HCl, (3) 3% Na2CO3, (4) CDTA, and (5) 4 M NaOH with 1% NaBH4.The rationale behind each extraction step was; the first two solvents (1, 2) were used to precipitate any negatively charged polysaccharides usually forming intermolecular bridges with calcium (e.g., alginates and pectins) and then to extract them with the a mild base (3). This would allow extracellular matrixes to become more accessible for further glycan removal by (4) which is a chelating agent and was used to complete the extraction of charged polysaccharides. The last solvent (5) is a well-established solvent for less soluble cell wall glycan components (for example hemicelluloses when applied to land plants) and can also partially dissolve cellulosic materials.
The extractions were printed as microarrays and probed with four anti-fucoidan mAbs (designated BAM1 to BAM4) recognizing distinct epitopes on fucoidan polysaccharides from the brown alga F. vesiculosus (Torode et al., 2015). In addition, we used a selection of probes directed against typical algal and plant cell wall components. These included: anti-alginates BAM7 and BAM10 mAbs (Torode et al., 2016); LM19 mAb recognizing homogalacturonan (HG) with low degree of esterification (Verhertbruggen et al., 2009); LM1 and JIM8 mAbs recognizing cell wall proteoglycans extensins and AGPs respectively (Pennell et al., 1991; Smallwood et al., 1995) and mAbs recognizing LM25 (xyloglucan; Pedersen et al., 2012), LM10 (xylan; McCartney et al., 2005). In addition to mAbs we used β-(1,4)-glucan (cellulose and xyloglucan)-specific carbohydrate binding modules CBM3a (Blake et al., 2006; Hernandez-Gomez et al., 2015) and CBM30 (Najmudin et al., 2006). We expected that these CBMs would bind to tunicin of C. intestinalis and hence serve as a positive control. Figure 1 shows simplified schematic of the whole CoMPP procedure and the Table 2 summarizes the probes used and the epitopes they recognized.
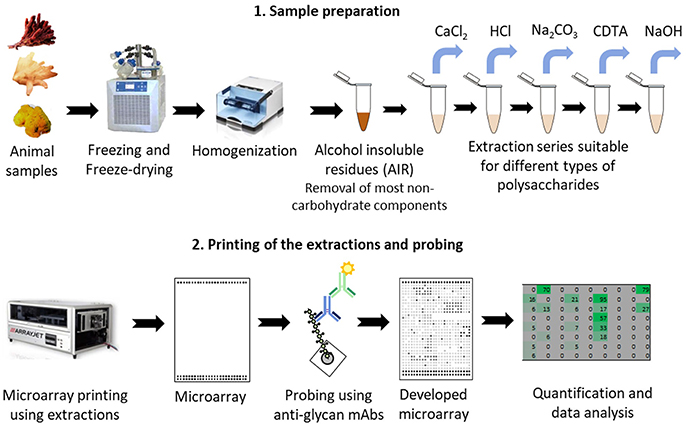
Figure 1. Schematic of the procedure for glycan profiling using Comprehensive Microarray Polymer Profiling (CoMPP) of marine animal tissues. CoMPP is consist of two parts: (1) preparation of the samples and series of extractions; (2) printing the extractions using microarray printers. Small microprints (arrays) are generated which are probed using a set of mAbs. After secondary probing and development the generated signals are quantified and analyzed.
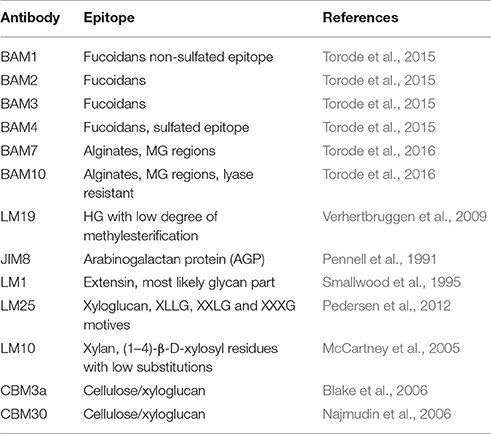
Table 2. The list of mAbs and CBMs used in this study, description of the epitopes they recognize and the references.
The quantification of the resulting developed arrays is presented as a heatmap where numbers are mean signal intensities resulting from antibody binding (Figure 2). We detected the presence of fucoidan epitopes in five organisms belonging to cephalopods, sponges, and tunicates. Signals were observed with BAM1 and BAM4 mAbs in six species whilst no binding was observed for BAM2 and BAM3 mAbs in any of the species (Figure 2). BAM4, which is specific for a sulfated epitope, bound to the S. officinalis (cephalopod) and C. intestinalis (tunicate) fractions, whereas BAM1 recognizing a non-sulfated epitope bound strongly to C. celata (demosponges). Interestingly, the effectiveness of different extraction buffers varied between the species. For instance the Na2CO3 extraction was the most efficient in extraction of BAM1 and BAM4 epitopes in several species, on the other hand only NaOH extraction revealed higher amounts of BAM4 epitopes in C. intestinalis (Figure 2). The differential extractability and binding support the notion of high structural diversity of these compounds in animals and possibly their intimate relationship (possibly covalent) to other matrix components. Interestingly, in our sample set up we could not detect fucoidans in echinoderms, which may be due to different structure not recognizable by BAM mAbs. Indeed Pereira et al. (1999), showed that fucoidans from sea urchin are more linear and repetitive than branched and heterogeneous as is the case in brown algae.
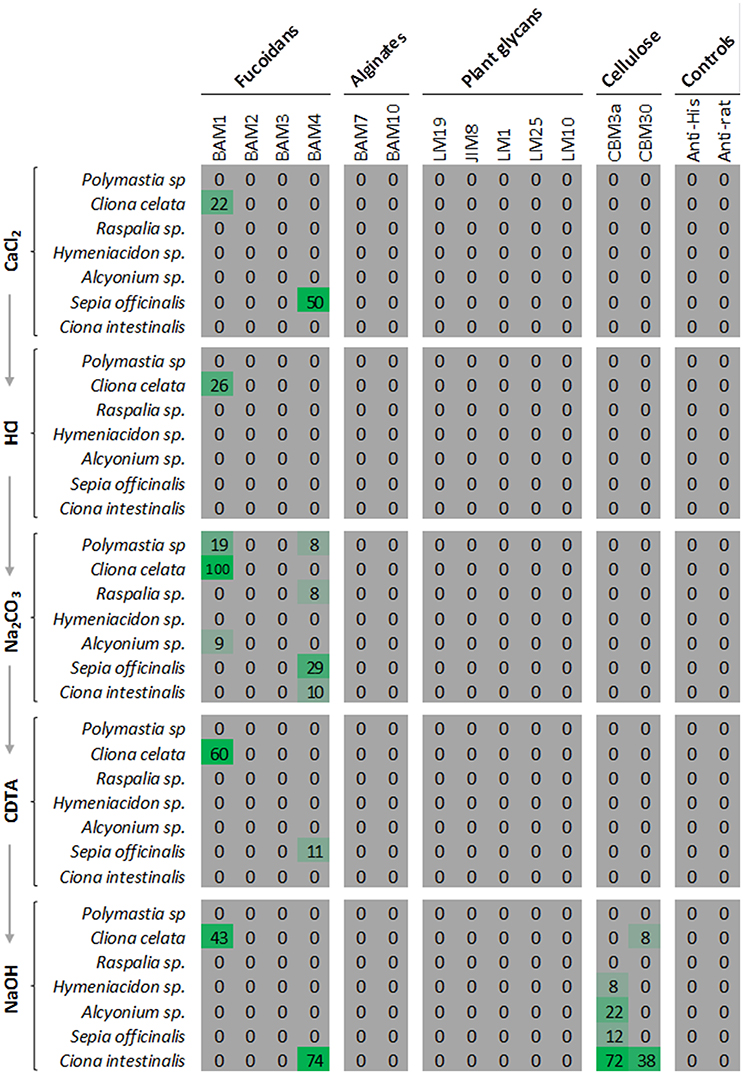
Figure 2. Results of the glycan profiling of marine animals using CoMPP. The quantification of the binding of mAb tested in a form of heatmap. Samples were extracted sequentially as indicated by the arrows. Values are calculated according to signal intensities. Only species with significant signals detected anti-fucoidan mAbs are shown. No BAM2 and BAM3 binding was observed. The carbohydrate binding modules CBM3a and CBM30, which bind to cellulose show strong binding to extraction of Ciona intestinalis producing tunicin. The intensity of green color represents the strength of the signal.
In the case of other plant-specific mAbs no significant binding was observed. Although low signal of two mAbs (mannan and xyloglucan) could be detected in the NaOH fractions of some species, binding signals were very low and fell below the 5% lower cut off used on the heat maps. On the other hand, as expected, both CBM3a and CBM30 bound strongly to NaOH fraction of C. intestinalis producing tunicin, demonstrating selectivity and sensitivity of the approach.
Immunohistochemistry of Fucoidan Epitopes in Cliona celata
Profiling of animal samples for the presence of glycan structures in homogenized samples can be hampered by the contamination with plant matter ingested as food, or by the presence of algal or bacterial endosymbionts. To partially address this, we also undertook immunolocalisation analysis in C. celata. Sponges are considered to be the simplest form of animals (Gold et al., 2016) and the presence of some polysaccharides in the sponges' bodies could be an ancestral trait shared with other eukaryotic lineages. However, sponges are also known to host symbiotic microorganism (Hentschel et al., 2012) which increase the possibility of false positive hits. Sulfated polysaccharides have been reported in many species of sponges including C. celata, although not yet directly classified as fucoidans (Zierer and Mourão, 2000; Esteves et al., 2011). Glycan chains which contain fucose are also a part of the sponges' aggregation factors–a highly complex and diverse class of circular proteoglycans also called spongicans. These glycan structures of aggregation factors are responsible for mediating the cell-to-cell adhesion via calcium bridges (Misevic and Burger, 1993; Fernandez-Busquets and Burger, 2003; Bucior et al., 2004; Vilanova et al., 2009, 2016).
We therefore decided to further explore the presence of fucoidan epitopes in situ and to characterize their localization in C. celata using immunolocalisation. A piece of tissue surrounding the ostium (inhalant pore) was embedded in paraffin and the sections were probed with BAM1 and BAM4 and observed using laser scanning confocal microscope (LSCM) (Figure 3). The morphological structure and different cell types are shown in the Figure 3B. BAM1 labeled exclusively the visceral layer of the internal chambers (spongocoel; Figures 3C,D). Also BAM1 did not strongly label any other cell types like sclerocytes—the cells producing needle-like structures spicules (Figure 3E). BAM4 labeling corroborated the results from CoMPP and no specific labeling could be observed in any of the other tissues (Figure 3F). The close observation showed that the BAM1 labels exclusively the layer of the cells forming the internal chambers (spongocoel) and no signal could be detected between the cells (Figure 3G). This indicates that this particular epitope is most likely not a part of the aggregation factors. On the other hand, previously it has been shown that glycoprotein-rich mucus (glycocalyx) connects collars of choanocytes with function in filtering or trapping food particles (Leys and Hill, 2012). Hence, our results suggest that fucoidan epitopes in sponges are actually part of the components of this type of extracellular matrix in some sponges.
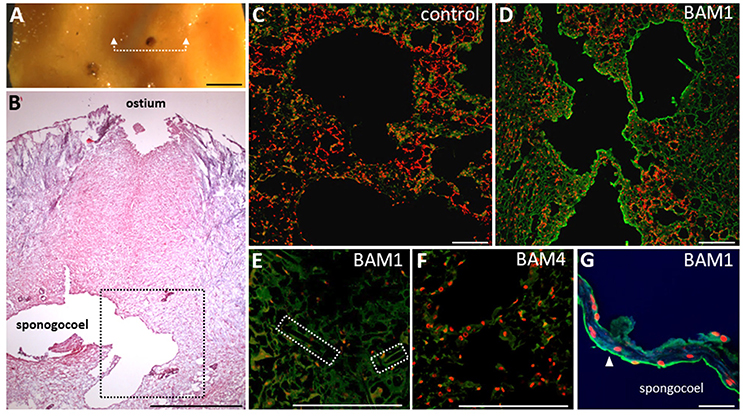
Figure 3. Immunolocalisation of fucoidan epitopes in Cliona celata. (A) Image of the sponge sample analyzed with indicated positions of sections over ostium by the white dashed line. (B) Analysis of morphology by H and E staining of the paraffin section with indicated tissues. (C,D) Staining of the paraffin sections by BAM1 antibody as a primary antibody and anti-rat Alexa Fluor 488 conjugated secondary antibody (green channel) of the region indicated by black square in (B). Propidium iodide (red channel) was used as a counterstain to stain nuclei. (C) Control sample with no primary antibody added. Only faint green signal emanating from autofluorescence of the sample could be observed. (D) BAM1 mAb labeling scanned with same settings revealed fucoidan epitopes on the surface of the internal chambers (spongocoel). (E) No BAM1 specific labeling can be observed in sclerocytes (producing skeletal elements spicules, position marked by dashed square) suggesting tissue specific expression of BAM1 epitope. (F) Anti-fucoidan mAb BAM4 did not label any tissue. (G) Close-up scan of the cells (pinacocytes or choanocytes) forming internal layer of chambers. Note the polarized localization on the surface (arrowhead) but not between the cells. Scale bars: 1 mm in (A,B); 100 μm in (C–F); 10 μm in (G).
Discussion
Our results demonstrated the feasibility to extend the application possibilities of the immuno-glycoprofiling method originally developed for plant material to analyse glycans in metazoans. Although the abundance of polysaccharides in animal bodies is generally lower than in plants, CoMPP proved to be sensitive enough to detect specific glycans in AIR extractions of some animal tissues. This approach enabled us to show that fucoidan epitopes typical for brown algae are relatively widely, but not ubiquitously present in diverse marine organisms. Although, the fine molecular structure of these polymers has yet to be determined by other analytical methods, our results further suggest heterogeneity and diversity of glycans in animals particularly sponges (Zierer and Mourão, 2000). For instance only three out of seven species of sponges were positive on BAM epitopes, with significant differences in their abundance. The interesting question is whether the synthesis of these glycan epitopes is an ancient remnant of the common ancestry with brown algae (Michel et al., 2010), the result of horizontal gene transfer or convergent evolution. In order to reconcile the nature of the origin of fucoidan biosynthesis in eukaryotes more molecular and in silico analyses would be necessary.
The traditional way for identification and characterization of glycans is extraction and purification (ion exchange or size exclusion chromatography) followed by subsequent analyses which include series of biochemical or biophysical methods including determination of monosaccharide composition, mass spectroscopy (MS), nuclear magnetic resonance (NMR), or Fourier-transformed infrared spectroscopy (FT-IR). CoMPP does not remove the necessity of these additional methods which are required to elucidate the molecular structure of the glycans, but it may enable the screening of large number of samples in a high-throughput, sensitive and selective way for the presence of the analyzed epitopes. This provide important preliminary information before doing above mentioned more elaborative analyses.
The usage of CoMPP largely relies on the availability of glycan antibodies. However, we showed that CoMPP can be used to profile carbohydrates for which the antibodies are available, regardless of the organismal kingdom. The field of plant glycobiology provides a large set of anti-glycan antibodies and CBMs. Although most of them are specific for plant glycans some of them can recognize cross-kingdom polysaccharides with structural similarities like recently reported starch/glycogen specific mAb (Rydahl et al., 2017). Smaller selection of antibodies have been generated against animal structural polysaccharides like chondroitin sulfate (Avnur and Geiger, 1984) but we expect that the number of antibodies, also those unique for marine organisms will grow in the future. One of the drawback of CoMPP is that it is semi-quantitative and does not provide information about the absolute quantities. It also involves extractions which can alter the studied material e.g., alkaline β-elimination (e.g., NaOH step). The printability and adherence of carbohydrates to nitrocellulose or other membranes might be also compromised. Finally, the specificity of antibodies is also an important factor to consider during interpretation of the data especially during cross-kingdom analyses.
In conclusion, the present approach in some cases may serve to identify horizontal gene transfer or convergent evolution events in glycan biology. Finally, many polysaccharides derived from marine organism are important nutra- and pharmaceuticals so this approach can help in bioprospecting of valuable materials or other rare polysaccharides with special structural features for novel applications.
Author Contributions
AS, WW, and JM designed the research; AS and CH collected, classified and prepared the specimens for microscopy; AS performed the CoMPP profiling and generated the heatmaps; JM performed the microscopy experiments; WW and BJ provided funding and management, all authors contributed in writing the manuscript.
Funding
This work was funded by The Danish Strategic Research Council project SET4Future—Sustainable Enzyme Technologies for Future Bioenergy (11-116795) and The Villum Foundation Project PLANET (00009283).
Conflict of Interest Statement
The authors declare that the research was conducted in the absence of any commercial or financial relationships that could be construed as a potential conflict of interest.
Acknowledgments
We would like to thank Drs. Murielle Jam, Mirjam Czjzek, and the staff from the Marine Service at the Station Biologique de Roscoff for the cultivation, sampling, and preparation of marine species, Kristoffer A. Kristensen for the help in preparation of the extractions, Prof. Paul Knox from the University of Leeds for providing the BAM antibodies and Center for Advance Bioimaging (CAB), University of Copenhagen for providing the sectioning and imaging equipment.
References
Ale, M. T., Mikkelsen, J. D., and Meyer, A. S. (2011). Important determinants for fucoidan bioactivity: a critical review of structure-function relations and extraction methods for fucose-containing sulfated polysaccharides from brown seaweeds. Mar. Drugs 9, 2106–2130. doi: 10.3390/md9102106
Avnur, Z., and Geiger, B. (1984). Immunocytochemical localization of native chondroitin-sulfate in tissues and cultured cells using specific monoclonal antibody. Cell 38, 811–822. doi: 10.1016/0092-8674(84)90276-9
Belton, P., Tanner, S., and Cartier, N. (1989). High-resolution solid-state carbon-13 nuclear magnetic resonance spectroscopy of tunicin, an animal cellulose. Macromolecules 22, 1615–1617. doi: 10.1021/ma00194a019
Blake, A. W., McCartney, L., Flint, J. E., Bolam, D. N., Boraston, A. B., Gilbert, H. J., et al. (2006). Understanding the biological rationale for the diversity of cellulose-directed carbohydrate-binding modules in prokaryotic enzymes. J. Biol. Chem. 281, 29321–29329. doi: 10.1074/jbc.M605903200
Bucior, I., Scheuring, S., Engel, A., and Burger, M. M. (2004). Carbohydrate–carbohydrate interaction provides adhesion force and specificity for cellular recognition. J. Cell Biol. 165, 529–537. doi: 10.1083/jcb.200309005
Esko, J., Kimata, K., and Lindahl, U. (2009). “Chapter 16: Proteoglycans and sulfated glycosaminoglycans,” in Essentials of Glycobiology, 2nd Edn., A. Varki, R. D. Cummings, J. D. Esko, H. H. Freeze, P. Stanley, C. R. Bertozzi, G. W. Hart, and M. E. Etzler (Cold Spring Harbor, NY: Cold Spring Harbor Laboratory Press), 229–248.
Esteves, A. I., Nicolai, M., Humanes, M., and Goncalves, J. (2011). Sulfated polysaccharides in marine sponges: extraction methods and anti-HIV activity. Mar. Drugs 9, 139–153. doi: 10.3390/md9010139
Fernandez-Busquets, X., and Burger, M. M. (2003). Circular proteoglycans from sponges: first members of the spongican family. Cell. Mol. Life Sci. 60, 88–112. doi: 10.1007/s000180300006
Gold, D. A., Grabenstatter, J., de Mendoza, A., Riesgo, A., Ruiz-Trillo, I., and Summons, R. E. (2016). Sterol and genomic analyses validate the sponge biomarker hypothesis. Proc. Natl. Acad. Sci. U.S.A. 113, 2684–2689. doi: 10.1073/pnas.1512614113
Hentschel, U., Piel, J., Degnan, S. M., and Taylor, M. W. (2012). Genomic insights into the marine sponge microbiome. Nat. Rev. Microbiol. 10, 641–654. doi: 10.1038/nrmicro2839
Hernandez-Gomez, M. C., Rydahl, M. G., Rogowski, A., Morland, C., Cartmell, A., Crouch, L., et al. (2015). Recognition of xyloglucan by the crystalline cellulose-binding site of a family 3a carbohydrate-binding module. FEBS Lett. 589, 2297–2303. doi: 10.1016/j.febslet.2015.07.009
Hervé, C., Siméon, A., Jam, M., Cassin, A., Johnson, K. L., Salmeán, A. A., et al. (2015). Arabinogalactan proteins have deep roots in eukaryotes: identification of genes and epitopes in brown algae and their role in Fucus serratus embryo development. N. Phytol. 209, 1428–1441. doi: 10.1111/nph.13786
Leys, S. P., and Hill, A. (2012). The physiology and molecular biology of sponge tissues. Adv. Mar. Biol. 62, 1–56. doi: 10.1016/B978-0-12-394283-8.00001-1
Li, B., Lu, F., Wei, X., and Zhao, R. (2008). Fucoidan: structure and Bioactivity. Molecules 13, 1671–1695. doi: 10.3390/molecules13081671
Marques, J., Vilanova, E., Mourao, P. A. S., and Fernandez-Busquets, X. (2016). Marine organism sulfated polysaccharides exhibiting significant antimalarial activity and inhibition of red blood cell invasion by Plasmodium. Sci. Rep. 6:24368. doi: 10.1038/srep24368
McCartney, L., Marcus, S. E., and Knox, J. P. (2005). Monoclonal antibodies to plant cell wall xylans and arabinoxylans. J. Histochem. Cytochem. 53, 543–546. doi: 10.1369/jhc.4B6578.2005
Michel, G., Tonon, T., Scornet, D., Cock, J. M., and Kloareg, B. (2010). The cell wall polysaccharide metabolism of the brown alga Ectocarpus siliculosus. Insights into the evolution of extracellular matrix polysaccharides in Eukaryotes. N. Phytol. 188, 82–97. doi: 10.1111/j.1469-8137.2010.03374.x
Misevic, G. N., and Burger, M. M. (1993). Carbohydrate-carbohydrate interactions of a novel acidic glycan can mediate sponge cell adhesion. J. Biol. Chem. 268, 4922–4929.
Moller, I., Sorensen, I., Bernal, A. J., Blaukopf, C., Lee, K., Obro, J., et al. (2007). High-throughput mapping of cell-wall polymers within and between plants using novel microarrays. Plant J. 50, 1118–1128. doi: 10.1111/j.1365-313X.2007.03114.x
Mulloy, B., Ribeiro, A. C., Alves, A. P., Vieira, R. P., and Mourao, P. A. S. (1994). Sulfated fucans from echinoderms have a regular tetrasaccharide repeating unit defined by specific patterns of sulfation at the 0-2 and 0-4 positions. J. Biol. Chem. 269, 22113–22123.
Najmudin, S., Guerreiro, C. I., Carvalho, A. L., Prates, J. A., Correia, M. A., Alves, V. D., et al. (2006). Xyloglucan is recognized by carbohydrate-binding modules that interact with beta-glucan chains. J. Biol. Chem. 281, 8815–8828. doi: 10.1074/jbc.M510559200
Nakashima, K., Yamada, L., Satou, Y., Azuma, J. I., and Satoh, N. (2004). The evolutionary origin of animal cellulose synthase. Dev. Genes Evol. 214, 81–88. doi: 10.1007/s00427-003-0379-8
Pedersen, H. L., Fangel, J. U., McCleary, B., Ruzanski, C., Rydahl, M. G., Ralet, M. C., et al. (2012). Versatile high resolution oligosaccharide microarrays for plant glycobiology and cell wall research. J. Biol. Chem. 287, 39429–394738. doi: 10.1074/jbc.M112.396598
Pennell, R. I., Janniche, L., Kjellbom, P., Scofield, G. N., Peart, J. M., and Roberts, K. (1991). Developmental regulation of a plasma membrane arabinogalactan protein epitope in oilseed rape flowers. Plant Cell 3, 1317–1326. doi: 10.1105/tpc.3.12.1317
Pereira, M. S., Mulloy, B., and Mourão, P. A. S. (1999). Structure and anticoagulant activity of sulfated fucans. J. Biol. Chem. 274, 7656–7667. doi: 10.1074/jbc.274.12.7656
Pomin, V. H., and Mourão, P. A. S. (2008). Structure, biology, evolution, and medical importance of sulfated fucans and galactans. Glycobiology 18, 1016–1027. doi: 10.1093/glycob/cwn085
Rydahl, M. G., Kracun, S. K., Fangel, J. U., Michel, G., Guillouzo, A., Genicot, S., et al. (2017). Development of novel monoclonal antibodies against starch and ulvan - implications for antibody production against polysaccharides with limited immunogenicity. Sci. Rep. 7:9326. doi: 10.1038/s41598-017-04307-2
Sagane, Y., Zech, K., Bouquet, J.-M., Schmid, M., Bal, U., and Thompson, E. M. (2010). Functional specialization of cellulose synthase genes of prokaryotic origin in chordate larvaceans. Development 137, 1483–1492. doi: 10.1242/dev.044503
Salmeán, A. A., Duffieux, D., Harholt, J., Qin, F., Michel, G., Czjzek, M., et al. (2017). Insoluble (1 → 3),(1 → 4)-β-D-glucan is a component of cell walls in brown algae (Phaeophyceae) and is masked by alginates in tissues. Sci. Rep. 7:2800. doi: 10.1038/s41598-017-03081-5
Smallwood, M., Martin, H., and Knox, J. P. (1995). An epitope of rice threonine- and hydroxyproline-rich glycoprotein is common to cell wall and hydrophobic plasma-membrane glycoproteins. Planta 196, 510–522. doi: 10.1007/BF00203651
Sørensen, I., Pettolino, F. A., Bacic, A., Ralph, J., Lu, F., O'Neill, et al. (2011). The charophycean green algae provide insights into the early origins of plant cell walls. Plant J. 68, 201–211. doi: 10.1111/j.1365-313X.2011.04686.x
Sørensen, I., Pettolino, F. A., Wilson, S. M., Doblin, M. S., Johansen, B., Bacic, A., et al. (2008). Mixed-linkage (1–>3),(1–>4)-beta-D-glucan is not unique to the Poales and is an abundant component of Equisetum arvense cell walls. Plant J. 54, 510–521. doi: 10.1111/j.1365-313X.2008.03453.x
Tang, W. J., Fernandez, J. G., Sohn, J. J., and Amemiya, C. T. (2015). Chitin is endogenously produced in vertebrates. Curr. Biol. 25, 897–900. doi: 10.1016/j.cub.2015.01.058
Torode, T. A., Marcus, S. E., Jam, M., Tonon, T., Blackburn, R. S., Herve, C., et al. (2015). Monoclonal antibodies directed to fucoidan preparations from brown algae. PLoS ONE 10:e0118366. doi: 10.1371/journal.pone.0118366
Torode, T. A., Siméon, A., Marcus, S. E., Jam, M., Le Moigne, M.-A., Duffieux, D., et al. (2016). Dynamics of cell wall assembly during early embryogenesis in the brown alga Fucus. J. Exp. Bot. 67, 6089–6100. doi: 10.1093/jxb/erw369
Vasseur, E., Setälä, K., and Gjertsen, P. (1948). Chemical studies on the jelly coat of the sea-urchin egg. Acta Chem. Scand. 2, 900–913. doi: 10.3891/acta.chem.scand.02-0900
Verhertbruggen, Y., Marcus, S. E., Haeger, A., Ordaz-Ortiz, J. J., and Knox, J. P. (2009). An extended set of monoclonal antibodies to pectic homogalacturonan. Carbohydr. Res. 344, 1858–1862. doi: 10.1016/j.carres.2008.11.010
Vilanova, E., Coutinho, C. C., and Mourao, P. A. S. (2009). Sulfated polysaccharides from marine sponges (Porifera): an ancestor cell-cell adhesion event based on the carbohydrate-carbohydrate interaction. Glycobiology 19, 860–867. doi: 10.1093/glycob/cwp059
Vilanova, E., Santos, G. R. C., Aquino, R. S., Valle-Delgado, J. J., Anselmetti, D., Fernandez-Busquets, X., et al. (2016). Carbohydrate-Carbohydrate interactions mediated by sulfate esters and calcium provide the cell adhesion required for the emergence of early metazoans. J. Biol. Chem. 291, 9425–9437. doi: 10.1074/jbc.M115.708958
Keywords: fucoidans, microarray profiling, structural polysaccharides, anti-glycan antibodies, immunolocalisation, sponges, Cliona celata, marine fauna
Citation: Salmeán AA, Hervé C, Jørgensen B, Willats WGT and Mravec J (2017) Microarray Glycan Profiling Reveals Algal Fucoidan Epitopes in Diverse Marine Metazoans. Front. Mar. Sci. 4:293. doi: 10.3389/fmars.2017.00293
Received: 04 June 2017; Accepted: 28 August 2017;
Published: 12 September 2017.
Edited by:
Antonio Trincone, Consiglio Nazionale Delle Ricerche (CNR), ItalyReviewed by:
Christopher James Day, Griffith University, AustraliaShishir P. S. Chundawat, Rutgers University, The State University of New Jersey, United States
Jan-Hendrik Hehemann, University of Victoria, Canada
Copyright © 2017 Salmeán, Hervé, Jørgensen, Willats and Mravec. This is an open-access article distributed under the terms of the Creative Commons Attribution License (CC BY). The use, distribution or reproduction in other forums is permitted, provided the original author(s) or licensor are credited and that the original publication in this journal is cited, in accordance with accepted academic practice. No use, distribution or reproduction is permitted which does not comply with these terms.
*Correspondence: Jozef Mravec, bXJhdmVjQHBsZW4ua3UuZGs=