- 1Integrative Oceanography Division, Scripps Institution of Oceanography, La Jolla, CA, United States
- 2Department of Biological Sciences and School of the Earth, Ocean, and Environment, University of South Carolina, Columbia, SC, United States
- 3Department of Polar Biological Oceanography, Alfred Wegener Institute, Bremerhaven, Germany
Carbon flow through pelagic food webs is an expression of the composition, biomass and activity of phytoplankton as primary producers. In the near future, severe environmental changes in the Arctic Ocean are expected to lead to modifications of phytoplankton communities. Here, we used a combination of linear inverse modeling and ecological network analysis to study changes in food webs before, during, and after an anomalous warm water event in the eastern Fram Strait of the West Spitsbergen Current (WSC) that resulted in a shift from diatoms to flagellates during the summer (June–July). The model predicts substantial differences in the pathways of carbon flow in diatom- vs. Phaeocystis/nanoflagellate-dominated phytoplankton communities, but relatively small differences in carbon export. The model suggests a change in the zooplankton community and activity through increasing microzooplankton abundance and the switching of meso- and macrozooplankton feeding from strict herbivory to omnivory, detritivory and coprophagy. When small cells and flagellates dominated, the phytoplankton carbon pathway through the food web was longer and the microbial loop more active. Furthermore, one step was added in the flow from phytoplankton to mesozooplankton, and phytoplankton carbon to higher trophic levels is available via detritus or microzooplankton. Model results highlight how specific changes in phytoplankton community composition, as expected in a climate change scenario, do not necessarily lead to a reduction in carbon export.
Introduction
The Arctic Ocean is one region where climate change is most pronounced, impacting the pelagic environment with observed effects on stratification, pH and currents. The consequences of these effects on phytoplankton are complex. Spatial shifts in latitude as well as timing of biological events affect phytoplankton bloom phenology, microalgal species distribution and trophic interactions (Aberle et al., 2012). A third major effect is the decrease in cell size distribution (Peter and Sommer, 2012), the focus of this study. A decrease in cell size can come about by direct effects of the environment on phytoplankton, e.g., higher temperature increasing metabolism, or indirectly, where environmental conditions alter grazing pressure on phytoplankton abundance, composition and cell size (Winder and Sommer, 2012). In the Arctic, warmer climate increases stratification, with warmer and less saline mixed layers, lower nitrate concentrations and higher picoplankton abundance (Li et al., 2009). Similarly, reduced sea ice cover in Lake Erie has been associated with smaller-sized cells that attain lower total biomass than during periods of ice cover that instead promotes chain-forming diatoms (Beall et al., 2016). Temperature could affect cell size of a given species or may facilitate larger vs. small species abundance, or both. However, this is not a given (Rüger and Sommer, 2012). Alternatively, it has been proposed that at higher temperatures grazing could intensify in a size-selective mode, affecting phytoplankton cell size distribution by top-down controls. Results are variable, with no cell size changes observed at higher temperatures (Rüger and Sommer, 2012 but see Daufresne et al., 2009) or grazing causing a reduction in cell size (Peter and Sommer, 2012). Although the importance of the grazers in the food chain is considered key to sedimentation (e.g., Reigstad et al., 2011) there is no large-scale consensus that small cells contribute substantially to sedimentation (but see Richardson and Jackson, 2007). Large zooplankton (e.g., Calanus spp.) feeding on the phytoplankton spring bloom, usually dominated by large cells, is known to produce a pulse of sedimentation through fecal pellet formation (Forest et al., 2010). Within this paradigm, it is expected that an absence of large cells, i.e., diatoms, will decrease the flux of material to the sediments (Wohlers et al., 2009).
In the Arctic, Atlantic water coming from the south becomes the West Spitsbergen Current (WSC); west of Svalbard, this current has a subsurface core at about 250 m depth and a surface expression. The current brings 6.6–8.5 Sv (or 106 m3 s−1) with a northward flow (Beszczynska-Möller et al., 2011). A cooling occurs as the water moves north, losing heat at the surface in contact with the atmosphere as well as sub-surface cross-front exchange with fresher and colder water from sea ice and/or glacial melting (Rudels et al., 2005). The Atlantic water cooling and freshening as it is transported north has a 5-to-6-year cycle in its salinity and temperature properties. Temperatures >2°C, with a mean temperature in the WSC of 3.1 ± 0.1°C characterize the Atlantic water at these latitudes (Beszczynska-Moller et al., 2012). Only one third of the heat carried by the WSC is transported into the Arctic Ocean, the rest is lost in westward transport and sea surface cooling (Kawasaki and Hasumi, 2016). In the 1997–2010 period, the trend is one of increased temperature but no significant change in volume transport (Beszczynska-Moller et al., 2012). The Warm Water Anomaly in 2005–2007 was defined as a northward advance of Atlantic water, a warm tongue more than 350 km north, reaching the Fram Strait northwest of Svalbard with waters 1°C higher than average (Walczowski et al., 2012).
The observed biological changes in eastern Fram Strait, and their implication for the Central Arctic Ocean, were tightly coupled with changes in the hydrography. Although the WSC shows pronounced inter-annual variability in primary productivity, phytoplankton and zooplankton abundance and composition (Wassmann et al., 2010; Carstensen et al., 2012; Kwasniewski et al., 2012), large changes in phytoplankton and zooplankton were associated with the warm water anomaly from 2005 to 2007 (Beszczynska-Moller et al., 2012; Nöthig et al., 2015; Soltwedel et al., 2016). This is best reflected in the long-term data set of the HAUSGARTEN observatory at 79°N, 4°E (Long-Term Ecological Research in the deep Arctic Ocean) that demonstrated a shift in phytoplankton community structure and in the composition of the sedimenting particulate carbon (Alcaraz et al., 2010; Lalande et al., 2013). The main diatoms found in the Atlantic waters of the WSC before the warm water event were large centric or chain-forming species, including Thalassiosira spp., Chaetoceros spp. (very often Chaetoceros socialis), chains of pennate diatoms of the genus Fragilariopsis spp., Navicula spp., Achnanthes taeniata and Fossula arctica in different proportions. Sometimes a few Rhizosolenia spp, Nitzschia/Pseudonitzscha sp., or Cylindrotheca sp., were observed (Degerlund and Eilertsen, 2010). At the time of the warm water pulse, higher phytoplankton biomass was observed in the water column, protistan plankton >3 μm changed in composition, and diatoms that dominated the period before the warm event switched to a dominance by coccolithophores in 2004, followed by Phaeocystis pouchetii dominance in 2006 (Nöthig et al., 2015). Several of these changes remained after the warm-water event, with Phaeocystis sp., still being prominent in the community (Metfies et al., 2016), although there has been a decrease in water temperature and in Phaeocystis sp. abundance from 97 to 48% from 2007 to 2011 (Soltwedel et al., 2016) whereas diatom concentration remained low and nanoflagellates increased to 43% (Nöthig et al., 2015). The ecosystem responded to the observed pelagic changes: there was an increase in food availability to the benthos in 2006–2007 when Phaeocystis sp., and flagellates dominated the overlying plankton community, which altered the abundance and community structure of the benthic bacteria and meiofauna, while macrofauna response lagged by a year (Jacob, 2014; Soltwedel et al., 2016).
Biological changes in the Fram Strait might foreshadow expected future changes in the Central Arctic, as this is the largest sub-Arctic water feeding the Arctic Ocean. In fact, what was observed in the Fram Strait during the warm period is seen throughout the Arctic Ocean and Arctic Seas: an increase of 20% in phytoplankton productivity due to more ice-free days during the growth season (e.g., Arrigo and van Dijken, 2011), a decrease in phytoplankton cell size associated with freshening and nitrate depletion in the mixed layer (Li et al., 2009), and changes in bloom phenology, both by an early sea ice retreat and late summer blooms (Kahru et al., 2011; Harrison et al., 2013; Ji et al., 2013; Ardyna et al., 2014).
In this study, we used a combination of linear inverse modeling and ecological network analysis to characterize and quantify the pathways of carbon flow through pelagic food webs of the eastern Fram Strait. We were particularly interested in how variations in phytoplankton community composition and in cell size before, during, and after the anomalously warm period of 2005–2007 affected the ecosystem trophic dynamics, including the transfer of carbon to higher trophic levels (planktivorous fish and cod) and export of carbon out of surface waters.
Methods
Model Construction
We constructed food webs for the WSC region of the eastern Fram Strait (Figure 1) using published data for the late spring/summer of 2003 (before the warming event), 2006 (during), and 2010 (after), or as close as possible to the time period (but always within 1 year). The same model structure was used for each time period (Figure 2). Each web comprised 42 flows that represented carbon flows between two compartments, or from one compartment to a sink (Table 1). The structure of the food webs was based on the assumption that sizes of the producers and consumers were major determinants of the trophic dynamics of these systems, i.e., small grazers are restricted to small algae. Choices of compartments and trophic relationships were a compromise between achieving biological reality and keeping the total number of flows in the system reasonable. The living components included two phytoplankton compartments, three zooplankton compartments, one compartment for small planktivorous fish, one for cod, and one compartment for heterotrophic bacteria. The phytoplankton were divided into “small” (0.2 to ~10 μm; assumed to be mainly picophytoplankton, coccolithophores, Phaeocystis sp., and small autotrophic flagellates) and “large” (> 10 μm; mainly diatoms and larger dinoflagellates; Kilias et al., 2014; Nöthig et al., 2015). Zooplankton size classes were the microzooplankton (20–200 μm; ciliates and flagellates), the mesozooplankton (200 to ~1,000 μm; mainly small copepods) and macrozooplankton (chaetognaths, euphausiids, and Calanus copepods >1,000 μm; Bamstedt et al., 1991; Hop et al., 2006; Blachowiak-Samolyk et al., 2007; Calbet, 2008; Pasternak et al., 2008; De Laender et al., 2010; Svensen et al., 2011; Monti and Minocci, 2013). Small planktivorous fish were assumed to be mainly capelin and herring but this compartment also includes carnivorous zooplankton, such as amphipods (Wassmann et al., 2006; Dalpadado et al., 2016). The top predator in the system was cod (Wassmann et al., 2015)
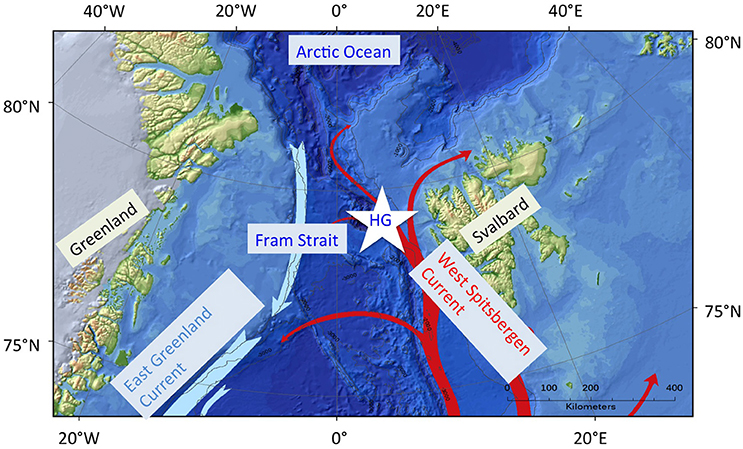
Figure 1. Map of the Fram Strait region with the northward moving, warm Atlantic water carrying West Spitsbergen Current (WSC) in the eastern Fram Strait (red arrows); blue arrows indicate the outflow of polar water in the East Greenland Current in the western Fram Strait. The center of the star is at 79°N and 4°E indicating the source of the data used in this study, HG is HAUSGARTEN observatory. (Map was produced with ArcGIS 10.3 using GEBCO 08, modified by Laura Hehemann from the Alfred Wegener Institute, Germany).
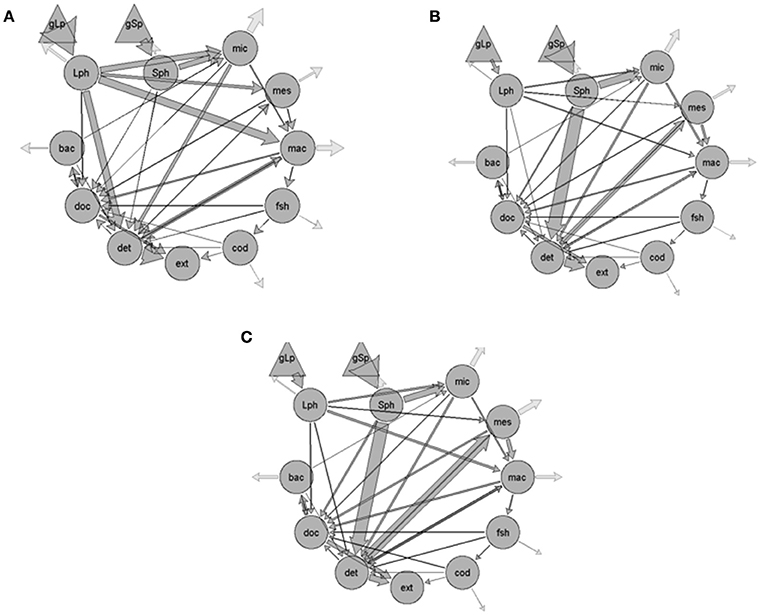
Figure 2. Flows of carbon (mgC m−2 d−1) through the food web of the West Spitzbergen Current in the eastern Fram Strait (A) before, (B) during, and (C) after the warm water anomaly of 2005–2007. Primary productivity was dominated by the large phytoplankton (Lph), primarily diatoms, before the warm event and by small phytoplankton (Sph; primarily Phaeocystis) during and afterwards. Phytoplankton were grazed by microzooplankton (mic), mesozooplankton (mes), and macrozooplankton (mac). Planktivorous fish (fsh) and cod (cod) generally consumed the larger grazers. Carbon could flow to detritus (det) as particulate material, or be remineralized as dissolved organic carbon (doc) to fuel bacterial productivity (bac). Gray arrows to nowhere represent respiration losses. gLp, gross primary productivity of the Lph; gSp, gross primary productivity of the Sph.
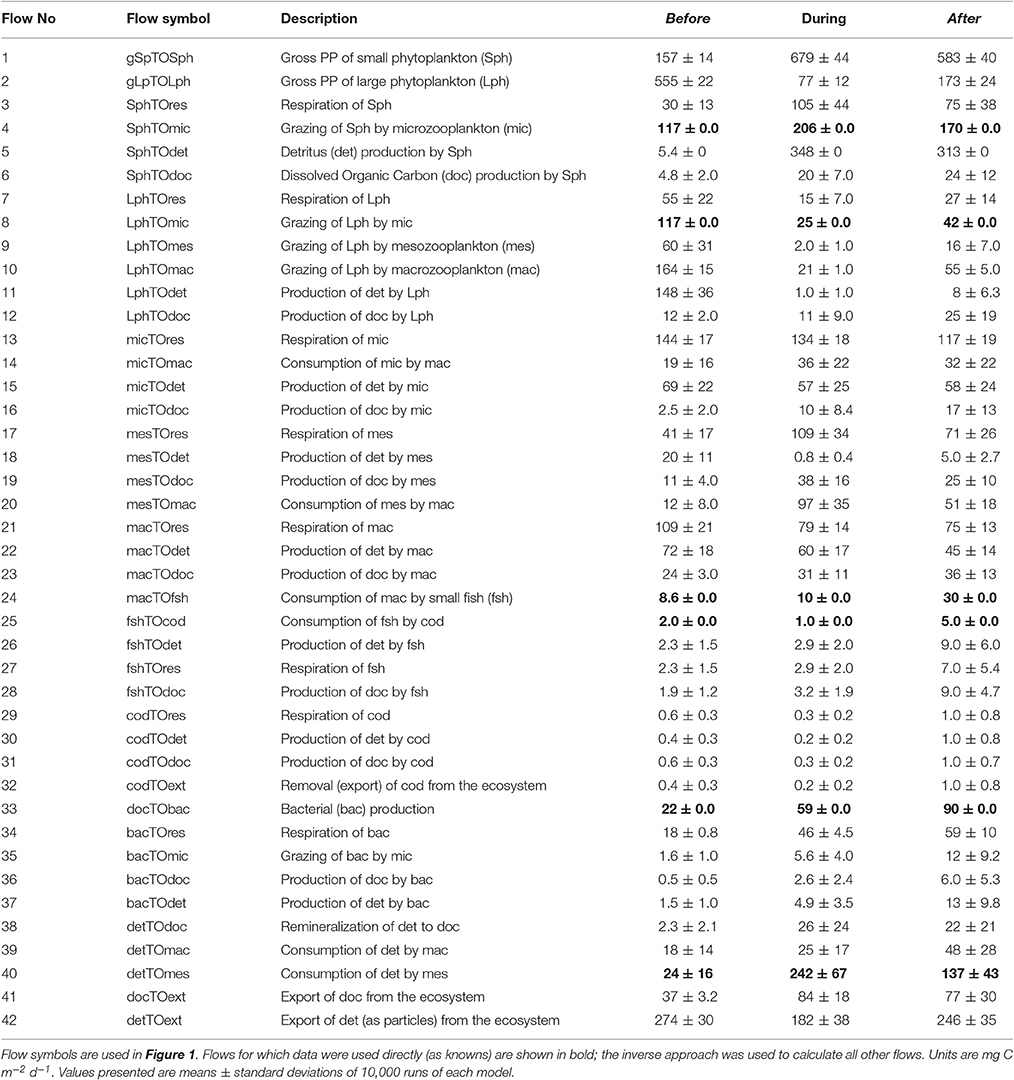
Table 1. Carbon flows in food webs constructed for before, during and after anomalously warm waters in the eastern Fram Strait.
All living compartments contributed to a labile dissolved organic carbon (DOC) pool through excretion and to the detrital pool through mortality or defecation. Sloppy feeding was implicitly included as excretion to DOC. Detritus was transformed to DOC by chemically- or bacterially-mediated dissolution (Jumars et al., 1989). All living compartments lost carbon by respiration. Other forms of mortality (e.g., viral lysis or natural cell mortality) were implicitly included in flows to detritus and DOC. All non-respiratory losses from the system were represented by flows to an “external” compartment that served as a mathematical closure term. These losses included particulate organic carbon (POC) export by detrital settling, DOC loss by advection, and removal of cod through the fishery or via consumption by higher trophic levels.
Data
We used published data from Fram Strait to calculate input (“known”) values for 7 of the 42 carbon flows: small and large phytoplankton primary productivity, bacterial productivity, microzooplankton grazing on small phytoplankton and flagellates, microzooplankton grazing on large phytoplankton, ingestion rates for the small fish and ingestion rates for cod (Table 2). Total primary productivity rates were taken from remote sensing estimates by Arrigo et al. (2008), Arrigo and van Dijken (2011, 2015) and daily production was estimated assuming annual primary production was evenly distributed over the period of open water. Contributions of flagellates vs. large phytoplankton to total primary productivity were assumed to be proportional to their size-specific contributions to biomass and were calculated from chlorophyll a measurements and phytoplankton community composition data of Nöthig et al. (2015, their Table 2). Accordingly, small phytoplankton and flagellates constituted 20% of the total phytoplankton biomass for the before models and 80% were considered large phytoplankton; Phaeocystis sp. and other flagellates accounted for 97 and ~50% of the small phytoplankton biomass for the during and after models, respectively.
Conversions from chlorophyll a to carbon units were done using an average C:chl ratio of 53 (g:g) to avoid seasonal biases (Svensen et al., 2011). The C:chl ratio of 53 for phytoplankton was chosen as a compromise between spring and summer values and those for small and large phytoplankton cells as shown by mesocosm experiments in which C:chl ratio of diatoms, dinoflagellate and mixed composition were 95, 45, and 60, respectively (Svensen et al., 2011; Spilling et al., 2014). Similarly, phytoplankton in the Fram Strait in May and August 2014 had a C:chl ratio of 41 (Marit Reigstad, personal communication). The ratio of 53 was used when converting from chl a estimates in the field (in mg chla m−2) to phytoplankton carbon (mg C m−2). A different C:chl ratio would either increase or decrease phytoplankton biomass in the model constraints but has no effect where phytoplankton biomass is an unknown.
There is difficulty in obtaining reliable and consistent data in high latitude environments due to the effort and cost of such studies. The data from Nöthig et al. (2015) as well as other field campaigns are based on cruises of a few weeks length, in the June and July time period, with the exception of estimates of fish abundance, provided by year. In this way, it is possible to compare year-to-year summer variability. Data from cruises from other times of the year (either April–May or August–September) were not included in this study.
Bacterial productivity values were calculated by multiplying bacterial abundance data by a C-specific production of 0.109 ± 0.89 d−1 (L. Seuthe, personal communication) an integrated over 0–45 m during cruises to NW Spitsbergen in 2014 (n = 7), and a biomass of 10 fg C cell−1 (Fukuda et al., 1998). Microzooplankton grazing rates were estimated from Verity et al. (1999, 2002) in the Barents Sea and Calbet et al. (2011) in the Fram Strait. For the before model, we assumed that microzooplankton grazing would be higher on small phytoplankton and flagellates (0.2 d−1) than on the larger phytoplankton (0.05 – 0.1 d−1) based on Strom et al. (2001). In contrast, for the models of during and after the warm period, when Phaeocystis sp. dominated the phytoplankton community, we assumed that microzooplankton grazing rates on small phytoplankton and Phaeocystis sp. were also low (0.05 d−1 for during and 0.1 d−1 for after) and no more than 8% of the phytoplankton standing stock based on previous studies (see also Caron et al., 2000; Calbet et al., 2011). After the warm period, grazing of microzooplankton on non-Phaeocystis sp. was 0.2 d−1. Ingestion rates for the small fish and cod were calculated from annual fish biomass in ICES ASWG 2014 and a conservative C-specific ingestion rate of 0.017 d−1 for cod (range of 0.017–0.057 d−1, De Laender et al., 2010) and an average C-specific ingestion rate of 0.04 d−1 for capelin and herring (range of 0.01–0.1 d−1, Ajiad and Pushchaeva, 1992; Megrey et al., 2007).
Sources of biomass for compartments are detailed in Table 3; these data are used to formulate the constraints used to set bounds on the flows predicted by the model (see Section Inverse Analysis below) (Table 4). The conversion factor of 0.132 was used to estimate carbon from wet weight in fishes (Sakshaug et al., 1994). Microzooplankton biomass was estimated from cell counts by the conversion factors of Verity and Lagdon (1984) and Menden-Deuer and Lessard (2000).
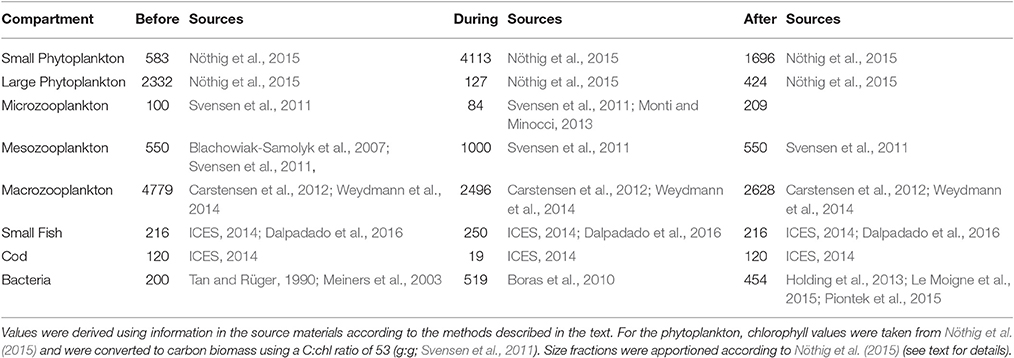
Table 3. Biomass values (mg C m−2) used for the formulation of constraint equations for the inverse analysis.
Inverse Analysis
The linear inverse modeling approach of Vézina and Platt (1988) was used in conjunction with the Monte Carlo solutions approach of Donali et al. (1999) for estimating the range of values for all flows in our constructed food webs. Model code was run in Matlab R2011b and was kindly provided by Dr. Nathalie Niquil (Centre National de la Recherche Scientifique, Caen, France). The approach taken assumes that biomass in any compartment is in steady state, i.e., the total flows entering any compartment are equal to the flows leaving it without any accumulation or decrease (with the exception of the “external” compartment), although modifications to the approach can be made to accommodate non-steady state scenarios by allowing residual flows to balance the system (e.g., Richardson et al., 2003).
As described above, data from the scientific literature were used to formulate 7 input equations. Combined with the 10 mass balance equations (one for each compartment; see Table 5), there were 17 equations available to describe the system with 42 flows. We reduced the number of possible solutions for this underdetermined system by applying a set of biological constraints (provided in Table 4). Allometric constraints based on published relationships incorporated available biomass data and provided upper and lower bounds on the rates and efficiencies of biological processes. For example, the respiration of all phytoplankton was constrained to be at least 5% but no more than 30% of the gross primary productivity (GPP) (Vézina and Platt, 1988). Growth efficiencies were assumed to be 25–50% of ingestion for the zooplankton groups (Straile, 1997). Bounds on assimilation efficiencies for all grazers were 50–90% of ingestion for the microzooplankton (Vézina and Platt, 1988; Straile, 1997) and 50–80% for the macrozooplankton (Straile, 1997). We also set a lower bound on the macrozooplankton grazing such that they consumed at least 30% of the large phytoplankton productivity (based on Wassmann et al., 2006). Other constraints are detailed in Table 4. We used temperatures of 3.5, 5, and 4°C for the before, during and after models, respectively (Beszczynska-Moller et al., 2012).
Application of constraints reduces the range of possible solutions, but does not provide a unique solution. The Monte Carlo approach of Donali et al. (1999) (see also the review by Niquil et al., 2012) calculates 10,000 possible solutions for each set of flows, thus we were able to calculate both an average and a standard deviation for each flow in the food web.
Econetwork Analysis of Inverse Solutions
After food webs were constructed for the before, during and after warm water event, the structure and function of each web was assessed using EcoNetwork analysis software (available at https://www.cbl.umces.edu/~ulan/ntwk/network.html; see also Ulanowicz and Kay, 1991; Ulanowicz, 2004). Michaels and Silver (1988), Ducklow et al. (1989), and McManus (1991) used earlier versions of this program to examine flows of nitrogen and energy, respectively, through microbial food webs to higher trophic levels in planktonic systems. We chose a key index from the output, input/export vectors, to calculate how much of each input flow (i.e., primary production of the two phytoplankton groups) eventually was exported through the three possible routes of export (via cod, detritus, or DOC). This calculation allowed us to break down the export flows into the relative contributions by the flagellate (small) vs. diatom (large) phytoplankton.
Results
Food webs constructed for before, during, and after the warm anomaly (Figure 2) differ substantially with respect to the input flows (contributions by the small phytoplankton and flagellates vs. large phytoplankton), trophic transformations, and predicted export pathways (Figure 2, Table 1). While diatoms (or large phytoplankton) dominated primary productivity before the warm anomaly in the WSC, Phaeocystis sp. accounted for 97% of the primary productivity during the warm years, and small phytoplankton (Phaeocystis sp. and flagellates) continued to dominate for almost 4 years after the peak in water temperature. The dominance of Phaeocystis sp. and low grazing of this material by the microzooplankton during the warm water event (see also Calbet et al., 2011) resulted in the model prediction of more carbon from flagellates (or small phytoplankton) going to detritus, which then became an important source of food for mesozooplankton or macrozooplankton (Figure 3).
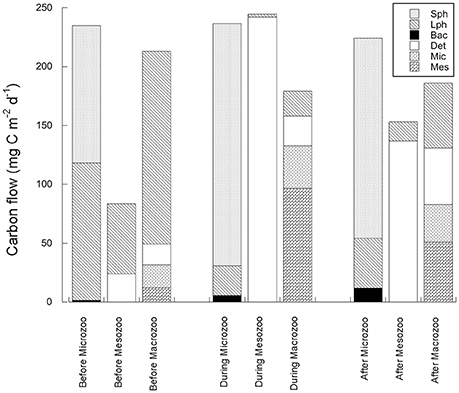
Figure 3. Carbon flow, predicted by the inverse modeling, through the zooplankton compartments (microzooplankton <200 μm, mesozooplankton, 200–100 μm and macrozooplankton (>1,000 μm) during three time periods in the Fram Strait, before, during and after the warm water event of 2005–2007. Compartment identification as in Figure 2.
In general, the zooplankton diet in the model reflected the dominant phytoplankton community composition (Figure 3). When large phytoplankton dominated before the warm water event, the microzooplankton fed equally on small and large phytoplankton, but consumed mostly carbon originating from small cells and flagellates in the during and after periods. The mesozooplankton diet changed during and after the warm anomaly to rely more heavily on detritus than on the large phytoplankton, while the carbon flow increased from <100 mg C m−2 d−1 to >150 mg Cm−2 d−1. In contrast, macrozooplankton carbon flow was predicted to remain somewhat constant through the three periods albeit important changes in the quality of diet, from mostly feeding on large phytoplankton, to a mixed diet where 50% of the carbon originated from the abundant mesozooplankton, and an even more mixed diet after the warm water event, with approximately equal consumption of large phytoplankton, detritus, microzooplankton and mesozooplankton (Table 1, Figures 2, 3).
Carbon in the form of detritus dominated the export fluxes, and was generally higher in the before period than during or after the warm event (Figure 4, Table 1). Carbon that originated from the diatoms dominated detrital export before the warm anomaly (86% of the total detritus export flux vs. 14% from the flagellates), but carbon from flagellates comprised the majority of the carbon exported as detritus during (96% small, 4% large) and after (89% small, 11% large) the warm water anomaly (Figures 4, 5).
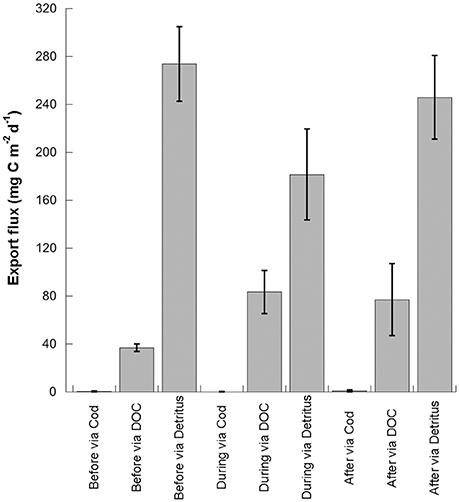
Figure 4. Main pathways of carbon export, predicted by the inverse modeling, during time three periods in the Fram Strait, before, during, and after the warm water event of 2005–2007: via cod, top predator, via dissolved organic carbon (DOC) and via particulate organic carbon (POC) or detritus. Error bars indicate one standard deviation from the mean.
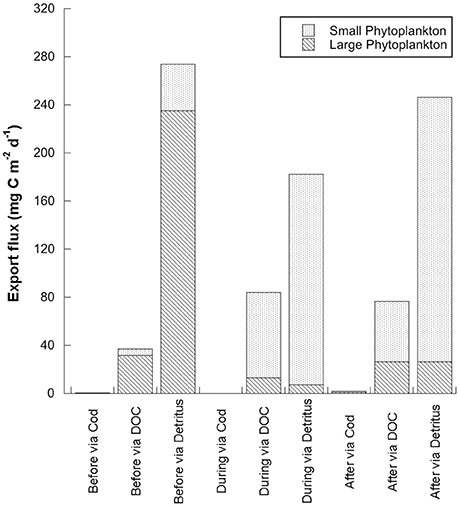
Figure 5. Pathways of small (including Phaeocystis sp.) and large (diatom) phytoplankton carbon export: via cod, via dissolved organic carbon (DOC) and via particulate organic carbon (POC) or detritus.
Calculated rates of respiration were dominated overall by the small phytoplankton and the microzooplankton (Figure 6). Microzooplankton and macrozooplankton respiration were maximum before, and mesozooplankton respiration rates were highest in during the warm water event. Only planktivorous fish and bacteria showed maximum respiration in after period. The largest changes were from before to during in small phytoplankton and mesozooplankton.
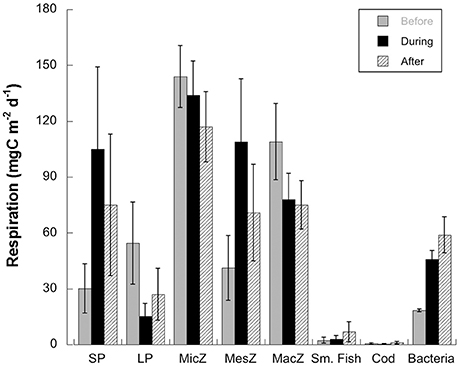
Figure 6. Respiration rates of the main food web compartments, predicted by the inverse modeling, during three time periods in the Fram Strait, before, during, and after the warm water event of 2005–2007. Compartment identification as in Figure 2.
Discussion
Carbon flow through pelagic food webs is impacted by the composition and biomass of the primary producers, the phytoplankton. It is expected that Arctic environmental change in response to climate warming will lead to modifications in phytoplankton species composition, cell size and biomass (Daufresne et al., 2009; Winder and Sommer, 2012). Consequences of these changes must be elucidated via field studies and modeling. We selected a modeling approach to study possible consequences in carbon cycling in the WSC, Fram Strait ecosystem from observed phytoplankton changes (Nöthig et al., 2015). Inverse modeling can synthesize and test current understanding in a system as well as provide a first approximation of carbon flows for which data are sparse. It has been applied in high latitude waters, mainly the Barents Sea (De Laender et al., 2010), the Amundsen Bay in the Canadian High Arctic (Forest et al., 2010), and in the western Antarctic Peninsula (Daniels et al., 2006; Sailley et al., 2013). The results from the model presented here are considered hypotheses about how the phytoplankton carbon could cycle in Arctic regions subject to shifts in phytoplankton composition.
The changes in carbon flow predicted by the inverse model from before to after warm water conditions parallel the changes modeled by Rivkin et al. (1996) in food webs from the Gulf of St. Lawrence. There, the structure of the food web changed from the colder spring bloom period, when large phytoplankton and herbivory by mesozooplankton dominated, to a warmer summer, microbial-dominated food web, but the amount of carbon exported in the cold vs. the warm periods was not substantially different. In summer, the mesozooplankton switched from eating large phytoplankton to consuming mainly microzooplankton. Thus, while the pathway of carbon through the system changed, the POC export flux did not. The Fram Strait system responded similarly; grazing was replaced by omnivory during warm water periods in the absence of large diatoms (Figure 3). The generality of the response is more striking as the post-bloom condition in the Gulf of St. Lawrence and the eastern Fram Strait were different: the first one was dominated by dinoflagellates where in the latter, diatoms were replaced by Phaeocystis sp. and nanoflagellates.
The inverse model suggested a scenario predicted already by Weisse et al. (1994) for the North Sea Phaeocystis sp. blooms. This author speculated that mesozooplankton, especially small copepods like Acartia and Temora, may indirectly benefit from Phaeocystis sp. blooms by feeding on detrital particles or microzooplankton. Vast amounts of detritus appear in the form of marine snow during and after Phaeocystis sp. blooms that, when coated with bacteria and microheterotrophs, is considered nutritious (Heinle et al., 1977). This new scenario of trophic pathways is not commonly found in the literature, as very little is known about pelagic detritivory. It has been proposed that zooplankton ingestion of detritus breaks up marine snow particles, facilitating bacterial degradation that, in turn, increases the nutritional value of the organic matter (Mayor et al., 2014). Although these authors focus their hypothesis on the water column below the euphotic zone, similar processes could occur in the mixed layer.
Response of Phytoplankton to the Warming Event in Eastern Fram Strait
The prediction of the proliferation of small cells in a future warmer ocean is usually associated with flagellates, whereas larger cells are presumed to be diatoms (Li et al., 2009, but see Wright et al., 2010). In the WSC, the transition of phytoplankton communities exposed to elevated temperatures can be more complex: the prymnesiophyte Phaeocystis sp. is a flagellate that can form both large colonies as well as single cells (Rousseau et al., 2000). In the WSC, the warming event was associated with a proliferation of this microalga from the summer of 2005 onwards, i.e., different clades of Phaeocystis sp. were dominant during and after the warm water event from 2005 to 2007 (Nöthig et al., 2015). This species is not new to the Fram Strait, commonly found in the region in spring and summer (Smith, 1987; Hegseth and Tverberg, 2013; Saiz et al., 2013). P. pouchetti slowly decreased in concentration after 2007 with an average 45% concentration after the warm water event. A major consequence of this shift from diatoms to Phaeocystis sp. is the change in grazing pressure; Phaeocystis sp. experiences less grazing than other flagellates (Caron et al., 2000; Strom et al., 2001; Calbet, 2008; Calbet et al., 2011).
Blooms of single-cell Phaeocystis sp. are of widespread distribution, with individual cells of 3–7 μm (Vernet et al., 1996; Kozlowski et al., 2011; Metfies et al., 2016) although it is generally considered that Phaeocystis sp. blooms in its colonial form, with colonies in excess of 100 μm and up to 1000 μm (Schoemann et al., 2005; Lasternas and Agusti, 2010). Single-cell Phaeocystis sp. in the eastern Fram Strait was observed in 2012 after the warm event in the <3 μm phytoplankton size fraction (Metfies et al., 2016). The proportion of P. pouchetii in colonial or in single-cell form at the WSC in 2005–2007 is unknown; both forms were modeled in this study (Stelfox-Widdicombe et al., 2004).
The model provides realistic estimates of phytoplankton growth rates, approximated from C-specific primary production. Flagellates grew at an average 0.2, 0.13, and 0.28 d−1 and large cells at an average 0.21, 0.39, and 0.28 d−1 within the surface layer before, during and after the warm water event, respectively. These rates were not measured, the biomass originated from the field (Table 3) and primary production from the model output (Table 1), which carry the inherent approximation that both phytoplankton size fractions have equal photosynthetic efficiency.
Trophic Pathways during a Shift in Phytoplankton Composition
When diatoms dominated (i.e., cells > 10 μm), the model predicted that phytoplankton were consumed by meso- and macrozooplankton herbivores, with a major carbon flow from fecal pellets to detritus and eventual export (i.e., sedimentation, Figure 2). Carbon also flowed to detritus and microzooplankton, accounting for the rest of the large cells, but the contribution was minor based on the small grazing pressure of microzooplankton on diatom blooms (Figure 3, Sherr and Sherr, 2009). The inverse model suggested that when Phaeocystis sp. dominated pelagic photosynthetic communities, e.g., by contributing up to 97% of the autotrophic community, phytoplankton carbon goes directly to detritus, and to a lesser extent to microzooplankton and DOC production (Figure 3). Mesozooplankton fed mostly on the detrital carbon, originating from phytoplankton sinking and fecal pellets, while a large proportion of the detritus was also exported. In this way, the mesozooplankton role in the food web increased when flagellates dominated but macrozooplankton role stayed rather constant, as in the case of microzooplankton. Total export out of the system decreased by 15%, due to a 35% diminution in POC export while DOC export increased (Figure 4). When the phytoplankton community bounced back to more diatoms, but still with ~45% Phaeocystis sp., overall export of particulate carbon also recovered while DOC contribution remained high (Figure 4). Additionally, the increase in microzooplankton and bacterial abundance during and after the warm water period indicate an increase in substrate, as expected from Kirchman et al. (2009a,b). The modeled sedimentation flux, where the mixed phytoplankton community composed of diatoms, Phaeocystis sp. and nanoflagellates exported as much carbon as diatoms alone, was also expected from the sediment trap data of Lalande et al. (2013) that showed that fluxes remained the same and only the quality changed. However, these model predictions are novel and will be discussed further.
Detritus Formation
The results of the inverse model suggest that when Phaeocystis sp. dominated the phytoplankton community a large proportion of the biomass was not consumed by grazers and was lost to other processes, mostly routed through detritus (e.g., marine snow), DOC production and respiration. The model did not predict high DOC production (Table 1, see below for further discussion) and the respiration changed based mostly on the amount of carbon cycling each through each compartment (compare Figures 2, 3 with Figure 6) and to lesser extent to higher ambient temperature; the remaining possibility was for the carbon to flow to detritus as marine snow. Most of the detritus originated from phytoplankton sinking or coagulating, from 148 mg C m−2 d−1 from diatoms in the before conditions to 313–348 mg C m−2 d−1 in the during and after conditions, mostly from Phaeocystis sp. (Table 1). Due to lack of data, the model does not have rigid constraints for this flow that at its highest reached 50% of the Phaeocystis sp. primary production in the during and after time periods (compare flows 5 and 1 in Table 1), whereas 26% of the diatom primary production was converted to detritus in the before conditions. These results suggest a doubling of the phytoplankton-detritus flow during the warm water event compared to the before (diatom) conditions, similar to observations of high concentration of marine snow in the North Sea during and after Phaeocystis sp. blooms (Lancelot and Mathot, 1987; Riebesell et al., 1993).
Microzooplankton Grazing
High microzooplankton grazing in summer/post-bloom/during conditions rich in flagellates has been well documented in the field (Vernet, 1991; Verity et al., 2002; Calbet and Saiz, 2005) and grazing efficiency of the microzooplankton can be lower when feeding on large phytoplankton cells (Strom et al., 2001). In the model, microzooplankton grazed on equal amounts of diatoms and small cells before 2003. During and after the warm water event, this compartment grazed mainly on Phaeocystis sp., maintaining their overall carbon intake (flow 4, Table 1). Microzooplankton consumed 235, 236, and 224 mg C m−2 d−1 in the form of large and small phytoplankton and bacteria in the before, during, and after conditions (Table 1), corresponding to a grazing rate of ~0.08, ~0.055, and ~0.1 d−1. These grazing rates are lower than what was observed in the Barents Sea for non-Phaeocystis phytoplankton (0.24 ± 0.1, 0.29 ± 0.13, 0.33 ± 0.11 d−1, Verity et al., 2002), and within the median value of Calbet et al. (2011) during a Phaeocystis sp. bloom (observed range of −0.04–0.14 d−1) in the Fram Strait. The explicit grazing inhibition by Phaeocystis sp. in the model (see Methods) is found not only in the Fram Strait but also in Antarctica (Caron et al., 2000) and elsewhere (Strom et al., 2001). In their review, Nejstgaard et al. (2007, Table 4) report grazing rates of 0.0–0.36 d−1 on solitary Phaeocystis sp. cells (3–8 μm). For field observations, the same authors report microzooplankton grazing was positive in April 2003 (0.21 ± 0.3 d−1) and negative in May 2004 (−0.23 ± 0.34 d−1). Without detailed knowledge of the factors affecting Phaeocystis sp. grazing in the WSC after 2004, the rates in the model are in the middle of the range found in the literature, and thus considered conservative. Further estimates of microzooplankton grazing, in particular in large phytoplankton and during Phaeocystis sp. blooms in the Arctic, are needed in order to improve our model parameterizations and our understanding of the fate of Phaeocystis sp. carbon through this compartment in the food web.
Inhibition of microzooplankton grazing by Phaeocystis sp. is similar to observations on other Ecosystem Disruptive Algal Blooms and Harmful Algal Blooms (EDABs and HABs). Acrylic acid, released by Phaeocystis sp. in the conversion from dimethylsulfoniopropionate (DMSP) to dimethylsulfide (DMS), is considered an antibiotic (Sieburth, 1960) and other growth and grazing inhibitors could be released by this species as well (Nejstgaard et al., 2007, but see Turner, 2015). The production of toxins by phytoplankton has lethal or sub-lethal effects on the microzooplankton, both for ciliates or tintinnids (Verity and Stoecker, 1982; Carlsson et al., 1990; Hansen, 1995). Rosetta and McManus (2003) concluded that ciliates may exert grazing pressure on HAB species early on, potentially contributing to the suppression and decline of Prymnesium minimum and P. parvum before they bloomed, but that ciliate grazing would be relatively ineffective once blooms (and toxicity) developed fully. In mixed diets, as long as non-toxic cells were available, ciliates survived and sometimes grew well at concentrations that otherwise would have killed them. Similar for rotifers, when exposed to a mixed diet of toxic and non-toxic phytoplankton species, these protists would tolerate and even acclimate to a toxic species (e.g., Karenia brevis), supporting the notion of low but positive grazing rates when Phaeocystis sp. was dominant (Table 2).
Grazing by Copepods
Grazing of meso- and macrozooplankton on Phaeocystis sp. depends on multiple environmental factors and it is not predictable. Nejstgaard et al. (2007) conclude in their review on grazing impacts on Phaeocystis sp. that small copepods cannot feed on colonies whereas macrozooplankton can. It has been observed that Arctic copepods do not avoid surface waters during Phaeocystis sp. blooms (Norrbin et al., 2009). However, Saiz et al. (2013) reported that under these conditions the copepod ingestion rate was low in spite of positive grazing rates, making a low impact on phytoplankton standing stocks. In this way, same as with microzooplankton grazing, Phaeocystis sp. seems to deter herbivory of larger zooplankton.
Mesozooplankton grazing
Grazing by mesozooplankton on diatoms, flagellates and detritus was set by the model within the constraints in this compartment on assimilation efficiency, respiration, excretion and growth gross efficiency (Table 4). Mesozooplankton consumed diatoms and detritus in the before conditions; in the absence of diatoms this compartment could decrease or consume more detritus. The model predicted detritivory, with an overall increase in mesozooplankton abundance (Figure 3, Table 1). Overestimation of detritivory with respect to other mesozooplankton feeding behavior by the model is possible due to the lack of constraints on this flow. As an alternative, mesozooplankton could consume more microzooplankton (Stoecker and Capuzzo, 1990; Rivkin et al., 1996). This pathway was not explicit in the model (Figure 1) as flows were limited to those identified as most important in the Fram Strait literature, where small copepods are considered of minor importance (Falk-Petersen et al., 2009; Nöthig et al., 2015). However, they might play a major role during the summer (Svensen et al., 2011). Results from the inverse model suggest that mesozooplankton could be an important carbon compartment in this region's food web and their role deserves further study and experimentation.
Macrozooplankton grazing
Macrozooplankton did not change ingestion on microzooplankton or detritus when Phaeocystis sp. was abundant, rather they increased predation on mesozooplankton. These results contrast with those of De Laender et al. (2010) that predicted higher trophic levels in food webs in the southern Barents Sea, flooded by Atlantic waters from another branch of the Norwegian Atlantic Current, could rely on the microbial loop as a source of carbon, with a doubling of microzooplankton as food source for Calanus spp. copepods. These authors argue that when small zooplankton is dominant during warmer periods, their feeding strategies are more suited to ciliate predation (e.g., Svensen and Vernet, 2016). In the Fram Strait model, macrozooplankton consumed 213, 179, and 196 mg C m−2 d−1 before, during and after the warm water event from diatoms, microzooplankton, mesozooplankton and detritus (Table 1, Figure 3). In the absence of diatoms, large zooplankton switched their intake to 8x more mesozooplankton, 2x more microzooplankton, but remained rather constant on detritus consumption.
Results from grazing experiments do not present a clear picture on Phaeocystis-zooplankton interactions. In their review, Nejstgaard et al. (2007) found a large variability in grazing rates within the literature, attributed to differences in P. globosa and P. pouchetii strains, cell types, physiological state, etc. In addition to grazing, macrozooplankton has the ability to break up large marine snow aggregates into smaller ones, facilitating their decomposition and increasing their nutrition (Dilling and Alldredge, 2000). The grazing estimates in the inverse model compare well with recent experimental results in the Fram Strait: Hildebrandt (2014) reports an average concentration of 26.6 Calanus finmarchicus per m3 with a grazing rate of 0.0028–0.014 μg chla h−1 for the summer of 2012; in a 45-m upper layer and assuming 24-h feeding during boreal summer the copepods could consume up to 24 mg C m−2 d−1. Similarly, average macrozooplankton grazing rates of 0.089, 0.205, and 0.137 d−1 for Calanus glacialis, C. hyperboreus, and C. finmarchicus, respectively, with an average rate of 0.15 d−1, were reported by Weydmann et al. (2014); these copepods could consume 349, 19, and 63 mg C m−2 d−1 before, during and after the warm water event (based on phytoplankton biomass from Table 3). These calculations based on Fram Strait experiments and field data agree with results in the North Sea where of P. globosa was not considered a good food source for copepods (Gasparini et al., 2000). In spite of selecting for diatoms and microzooplankton, copepods suffered during a Phaeocystis sp. bloom; copepods consumed 27–50% of the copepod carbon weight per day during diatom dominance that decreased to 7–17% during the Phaeocystis sp. bloom and to 14–21% after the bloom.
Detritivorous copepods
Detritivorous copepods are usually considered to feed below the euphotic zone. Jackson (1993) suggested that this process could explain the decreased in POC sedimentation in the ocean where only a few percentage of primary production reaches the sediments. “Flux feeding” was proposed as a major carbon flow to complement bacterial degradation of sinking organic matter that could not explain all the carbon reduction with depth. Similarly, Reigstad and Wassmann (2007) measuring recycling of Phaeocystis sp. phytodetritus found that between 7 and 11% of Phaeocystis sp. biomass reaches 40 m depth and only 3 ± 2% reaches 100 m. Assimilation efficiency of zooplankton feeding marine snow in the California Current were 64–83% (Dilling et al., 1998). Similarly, 70% retention of copepod and euphausiid fecal pellet carbon was established in the mixed layers of the Barents Sea thru flux feeding (Wexels Riser et al., 2002), but no studies exist of Arctic copepods and other planktonic organisms consuming sinking phytodetritus (Turner, 2015). For the California Current, Graham et al. (2000) explained diel variability in marine snow concentration in the upper water column to nighttime consumption by vertically migrating zooplankton. There is evidence of high mesozooplankton abundance during periods of Phaeocystis sp. blooms in the North Sea (Fransz and Gieskes, 1984; Weisse et al., 1986). In the Arctic, there is an increasing awareness that small copepods have been undersampled due to large mesh sizes in zooplankton nets. Svensen et al. (2011) argue the best method to sample small copepods quantitatively is with water bottles (e.g., 30-L Niskin). Small copepods have a high growth rate and reproduce year around, are not restricted in their reproduction to the spring bloom as are large copepods and thus can be very abundant year around (Svensen et al., 2011). The model results highlight the possibility that if Phaeocystis sp. is not consumed at a high rate, the mesozooplankton could benefit (as predicted by Weisse et al., 1994). Potential detritivory and the role of mesozooplankton during periods of Phaeocystis sp. dominance are ideas that deserve further study.
Bacteria
The model predicts a higher bacterial abundance and activity in warmer periods in the Arctic, when the phytoplankton community is dominated by flagellates, resulting in a more active microbial food web (Table 1, Figure 6). The bacterial activation occurs parallel to detritus formation, during and after the warm water anomaly. Bacteria decomposition of this detrital material is accounted for in the model (flow 36 in Table 1), so bacteria can either benefit from phytoplankton excretion or lysis as DOC, other sources of DOC production, or particulate matter degradation (Figure 2). Bacterial production, based on abundance and a C-specific production of 0.1 ± 0.98 d−1 (Seuthe, pers. commun.), is predicted at 22, 59, and 90 mg C m−2 d−1 before, during, after the warm water event (flow 33 in Table 1). These estimates are within those observed in the region. In the productive waters of Kongsfjorden, a fjord in the western coast of Spitsbergen, Iversen and Seuthe (2011) reported that for 2006, integrated over 0–50 m depth, bacterial production was 105 mg mg C m−2 d−1 and bacterial respiration 56 mg C m−2 d−1. For open waters close to the HAUSGARTEN station, surface bacterial production is highly variable, and was estimated at 2 mg C m−3 d−1 (or 90 mg C m−2 d−1) between 25 June and 20 July 2011 (Piontek et al., 2014, 2015).
DOC Production
In the model, DOC is produced by all living compartments via phytoplankton excretion, by bacterial activity (including detritus) and by zooplankton sloppy feeding (Figure 1, Table 1). DOC production increased during the flagellate periods, with more DOC produced in the during and after scenarios: from 57.3 to 116.1 mg C m−2 d−1 and 143 mg C m−2 d−1 as a result of bacterial activity (flow 36), of grazing by microzooplankton (flow 16), by mesozooplankton (flow 19) and by macrozooplankton (flow 23) (Table 1, Figures 3, 6). Due to restrictions in the number of flows (see Methods) viruses and fungi, another potential source of DOC production, were not included explicitly as part of the microbial loop, but their activity was implicit in phytoplankton DOC production. The constraints for this compartment were chosen to allow for “excess” (beyond normal excretion) DOC production, up to 55% of the primary production (Table 4). The DOC rates from phytoplankton predicted by the model are toward the low end of this range, 2.1% to 14% for large phytoplankton, and 3–4.1% for Phaeocystis sp. (Table 1). These estimates are close to the 10% universal estimate on phytoplankton excretion even in the presence of mucilaginous colonies (Veldhuis et al., 1986), and lower than field measurements in the Arctic of up to 39% (Vernet et al., 1998; Matrai et al., 2007; Poulton et al., 2016). The extent of DOC production by viral lysis in the Arctic is not well characterized. In the North Atlantic, Mojica et al. (2016) reported elevated rates of DOC production from phytoplankton viral lysis, with a “striking reduction” toward high latitudes, where the ratio of viral lysis to grazing decreased by up to two orders of magnitude in comparison to lower latitudes. Fungi are reported to be abundant in sea ice, but have not been found in any quantity in seawater (Hassett and Gradinger, 2016). Metfies et al. (2016), analyzing data for this region, did not detect many fungi (OTUs) in the water column; they were found only occasionally in waters dominated by diatoms. Fungi seem to be mainly associated with marine snow (Bochdansky et al., 2017) and in sediment-trap material (Metfies, in prep.). Increasing the lower limit of DOC production in the model can force more carbon through the DOC compartment which might decrease other loss terms, such as detritus production during and after the warm water event, presumably channeling more carbon through the microbial loop. The complexity of the microbial food web in Arctic waters, including viruses and fungi, requires further experimentation. The model for this study (Figure 2) was structured to maximize all the pathways that contribute to carbon sedimentation out of surface waters in the WSC and is thus not the best vehicle to represent a complete picture of microbial processes in this region, which would probably require a model restricted to lower trophic levels only.
Carbon Export
What the inverse model in this study provides is a picture of possible carbon pathways within Arctic food webs that could explain how to maintain an important contribution of phytoplankton carbon to deep water in the absence of a diatom bloom. Export of carbon originating from non-diatoms is not surprising (Figure 4). Picoplankton and flagellates have been observed in sedimenting matter for the last 30 years: Synechococcus was a major contributor to fluxes to the deep sea (Lochte and Turley, 1988) and has been detected in the South Pacific (Waite et al., 2000), tropical Pacific (Stukel et al., 2013) and in equatorial Pacific, Atlantic and Indian Oceans (e.g., Lampitt et al., 1993). High abundance of Synechococcus has been recently reported for the eastern Fram Strait, included here as cells <10 μm (Paulsen et al., 2016). Similarly, the ubiquitous Micromonas sp. in Arctic waters has been detected in this region by sediment traps, and associated with increased 234Thorium adsorption in the Central Arctic (Charles Bachy, pers. commun., Roca-Marti et al., 2016). Great quantities of Phaeocystis sp. were observed at the ocean bottom in the Ross Sea, at >500 m depth (DiTullio et al., 2000). In the Fram Strait, highest export was observed during a Phaeocystis sp. bloom, equivalent to the export efficiency, or % of primary production that sediments, by diatoms (Le Moigne et al., 2015). In the Gulf of St. Lawrence, the export efficiency increased from 10% during the diatom bloom to 10–5% in post-bloom conditions (Rivkin et al., 1996). In the Canadian Arctic, flagellates were associated with high export ratios of 0.38–0.69 (Lapoussiere et al., 2013). Similarly, the inverse model in the Fram Strait predicts an export efficiency of 51, 44, and 53.5% before, during and after the warm water event (Table 1). In this way, Phaeocystis sp. and flagellates can fuel the biological pump, transferring an important proportion of surface primary production to depth.
Carbon export in the model originated from either diatoms or detritus that during the warm period is overwhelmingly dominated by Phaeocystis sp. carbon (Figure 5). By which processes can flagellates contribute to export out of the surface layer? High biomass, stickiness, and presence of ballast all correlate with increased phytoplankton sedimentation by coagulation (e.g., Passow and Alldredge, 1999; Jouandet et al., 2014). In general, diatoms and coccolithophores are considered to sink faster than other phytoplankton and their silicon frustule (opal) or carbonate coccoliths are assumed to act as ballast for phytoplankton sinking and zooplankton fecal pellets, activating the biological pump (Armstrong et al., 2001; Klaas and Archer, 2002; Ploug et al., 2008). Ballast for phytoplankton could originate also from intracellular carbohydrates, minerals or carbonate precipitated within sea ice (Richardson and Cullen, 1995; Iversen and Ploug, 2010). Phaeocystis sp. blooms are reported to have very high sinking rates (Wassmann et al., 1990; DiTullio et al., 2000 but see Schoemann et al., 2005). DMSP, known to be elevated in Phaeocystis sp., has recently been suggested as ballast for this species (Lavoie et al., 2015, but see Boyd and Gradmann, 2002). Coagulation of cells in turbulent environments, in particular species with a sticky surface as observed in senescent Phaeocystis sp. blooms, generates marine snow; this process is considered a widespread venue of removing cells from the upper ocean (Passow and Wassmann, 1994; Logan et al., 1995). Mucus webs of pteropods are also known to be an efficient transport vehicle for pico-plankton particles (Noji et al., 1997). High stickiness in Arctic phytoplankton is expected; diatoms excrete large amounts of polysaccharides (Myklestad, 1995) and Arctic phytoplankton, both diatoms and flagellates, can excrete as much as 70% of their daily primary production as DOC (Vernet et al., 1998; Matrai et al., 2007; Poulton et al., 2016), which can be considered a source of stickiness (Schoemann et al., 2005). Marine snow is part of the detrital carbon, and is difficult to detect and quantify. TEP (transparent exo-polymers) is believed to comprise most of the marine snow and its sinking speed is also related to size, porosity and ballast usually provided by its constituents (Passow, 2002; Bach et al., 2016). For example, porosity of marine snow is lower when flagellates dominate in comparison with diatom-rich aggregates, thus providing another mechanism by which non-diatom aggregates can export carbon (Bach et al., 2016).
The changes in carbon export predicted by the model when Phaeocystis sp. dominated agree in large extent to the observations from sediment traps in the eastern Fram Strait. Flux of (POC) at 179–280 m depth from 2002 to 2008, with 20 sampling cups per year collecting material from 59 days in winter and 7 days in summer, showed POC sedimentation associated with biogenic silica (bSi) pulses (Lalande et al., 2013). Before the fall of 2004, these pulses occurred in spring (April to June), sometimes associated with the ice edge and in the late summer (August to October) due to atmospheric heating of the upper water column. Before 2004 the pulses ranged from 30 to 50 mg C m−2 d−1 and 10–30 mg bSi m−2 d−1. From late 2004 to the summer of 2008, during the warm water event, the consistency of the spring and late summer bSi pulses disappeared, with a few peaks in sedimentation in either May or August (~10 mg bSi m−2 d−1) remaining and the rest of the time sedimentation was <5 mg bSi m−2 d−1 (Figure 2f, Lalande et al., 2013). The pulses of POC remained unchanged throughout this period, both in magnitude and time of the year (Figure 2e, Lalande et al., 2013).
Any differences between model predictions and sediment trap data on sedimentation rates are expected, as export in the model represents carbon loss out of the surface layer (<100 m) while the sediment traps were deployed at ~250 m depth. In the field, the changes in flux of bSi correlated with other important changes in the nature and quality of the sedimenting matter: lower fecal pellet carbon and an increase in the amount of small fecal pellets, attributed to dominance of smaller zooplankton (Lalande et al., 2013). The inverse model predicts the change in quality of sedimenting matter can be attributed to the dominant phytoplankton community, diatoms vs. Phaeocystis sp. and to the changes in trophic pathways in the food web (Figure 5, see Section Discussion. for a detailed discussion on carbon flow through the modeled food web). The predicted changes in the biomass of the different compartments are reflected in higher respiration during and after the warm water event for small phytoplankton, mesozooplankton (small copepods) and bacteria and lower respiration from large phytoplankton (Figure 6).
Our model results present an alternative hypothesis that warming and flagellates could bring increased heterotrophy to the Arctic, expressed as the ratio of respiration to primary production (Table 1, Figure 6). The paradigm that flagellates will decrease sedimentation corresponds to a scenario of higher retention of organic carbon in surface waters and higher respiratory losses (Forest et al., 2010; Vaquer-Sunyer et al., 2010). Similarly, predictions of lower sedimentation are associated with an activation of the microbial loop (Kirchman et al., 2009b). High export in absence of diatoms in the Arctic is also possible as shown in this study, or when dinoflagellates replace diatoms (Rivkin et al., 1996); high sedimentation by flagellates has been observed in the field even when diatoms are abundant in surface waters (Amacher et al., 2013). A high export is possible if a link between the classical and microbial food webs develops through the consumption of microzooplankton and detritus by copepods.
Conclusions
1. In conclusion, more proliferation of flagellates, such as Phaeocystis sp. in Arctic waters as a response to warming, presumably increasing stratification and reducing nitrate availability through the halocline, is predicted by the inverse model to alter the amount of carbon sedimentation by <20%, and thus the biological pump remains effective. The consumers in the Arctic food web can adjust to the change from diatoms to flagellates by increasing microzooplankton abundance, by switching meso- and macrozooplankton feeding from herbivory to omnivory, detritivory and coprophagy. When Phaeocystis sp. dominates, the pathway of carbon through the food web is longer, at least one step is added in the flow from phytoplankton to mesozooplankton. Phytoplankton carbon to higher trophic levels is available either as detritus or as microzooplankton biomass.
2. Results from the inverse model provide several important hypotheses in relation to the carbon cycling in Arctic food webs subject to warming and presumable to a decrease in diatoms and an increase in flagellates, including Phaeocystis sp. The hypotheses in relation to the role of grazing by microzooplankton and small copepods, the role of detritivore copepods in consuming marine snow and other detrital carbon and the relative importance of the microbial vs. the classical food web need to be tested experimentally, both in the laboratory and in the field.
3. Inverse modeling provides a snapshot of conditions over short timescales, here during summer cruises to the eastern Fram Strait. The emphasis is on studies of trophic interactions. The quality of the results is based on previous knowledge of the food web and trophic interactions as well as the availability of rate processes at critical times through the growth season (April to September). Technological advances will help provide a better understanding of inter-annual and intra-annual variability in Arctic systems. As more data becomes available the quality of model predictions, as well as their accuracy, will increase.
Author Contributions
MV organized the study, was responsible for searching the majority of data used in the model, participated in model interpretation and was in charge of the writing. TR was in charge of the model development, participated in interpretation of results and writing the manuscript. IP, EN, and KM provided data for the model and participated in food web construction, data interpretation and writing.
Conflict of Interest Statement
The authors declare that the research was conducted in the absence of any commercial or financial relationships that could be construed as a potential conflict of interest.
Acknowledgments
This work was made possible through a fellowship from Hanse-Wissenchaftskolleg, Delmenhorst, Germany to MV and we thank them for their hospitality. We thank Natalie Niquil for sharing inverse modeling code and Marit Reigstad and Lena Seuthe for data on POC, chla and bacterial abundance and production. We also thank Marit Reigstad, Lena Seuthe, Camilla Svensen and Elisabeth Halverson for discussions on Arctic food webs, Anya Waite and George Jackson for discussions on phytoplankton sedimentation and Alexandra Kraberg for sharing her knowledge on C:Chla rations in the Arctic. The Polar Biological Oceanography Group of the Alfred Wegener Institute, Helmholtz Centre for Polar and Marine Research within Polar Regions and Coasts in the changing Earth System (PACES I and II) provided support for EN and IP in the Plankton Ecology and Biogeochemistry in a Changing Arctic Ocean (PEBCAO) group. A United States National Science Foundation grant PLR-1443705 and the Carbon Bridge project No. 226415, Polar Program under the Research Council of Norway, provided partial funding for MV.
References
Aberle, N., Bauer, B., Lewandowska, A., Gaedke, U., and Sommer, U. (2012). Warming induces shifts in microzooplankton phenology and reduces time-lags between phytoplankton and protozoan production. Mar. Biol. 159, 2441–2453. doi: 10.1007/s00227-012-1947-0
Ajiad, A. M., and Pushchaeva, T. Y. (1992). The Daily Feeding Dynamics in Various Lenght Groups of the Barents Sea Capelin during the Feeding Period. ICES CM Documents 1991/H:16.
Alcaraz, M., Almeda, R., Calbet, A., Saiz, E., Duarte, C. M., Lasternas, S., et al. (2010). The role of arctic zooplankton in biogeochemical cycles: respiration and excretion of ammonia and phosphate during summer. Polar Biol. 33, 1719–1731. doi: 10.1007/s00300-010-0789-9
Amacher, J., Neuer, S., and Lomas, M. (2013). DNA-based molecular fingerprinting of eukaryotic protists and cyanobacteria contributing to sinking particle flux at the Bermuda Atlantic time-series study. Deep Sea Res. II Top. Stud. Oceanogr. 93, 71–83. doi: 10.1016/j.dsr2.2013.01.001
Ardyna, M., Babin, M., Gosselin, M., Devred, E., Rainville, L., and Tremblay, J. -É. (2014). Recent Arctic Ocean sea ice loss triggers novel fall phytoplankton blooms. Geophys. Res. Lett. 41, 6207–6212. doi: 10.1002/2014GL061047
Armstrong, R. A., Lee, C., Hedges, J. I., Honjo, S., and Wakeham, S. G. (2001). A new, mechanistic model for organic carbon fluxes in the ocean based on the quantitative association of POC with ballast minerals. Deep Sea Res. II Top. Stud. Oceanogr. 49, 219–236. doi: 10.1016/S0967-0645(01)00101-1
Arrigo, K. R., and van Dijken, G. L. (2011). Secular trends in Arctic Ocean net primary production. J. Geophys. Res. Oceans 116, 1–15. doi: 10.1029/2011jc007151
Arrigo, K. R., and van Dijken, G. L. (2015). Continued increases in Arctic Ocean primary production. Prog. Oceanogr. 136, 60–70. doi: 10.1016/j.pocean.2015.05.002
Arrigo, K. R., van Dijken, G., and Pabi, S. (2008). Impact of a shrinking Arctic ice cover on marine primary production. Geophys. Res. Lett. 35, L19603. doi: 10.1029/2008GL035028
Bach, L. T., Boxhammer, T., Larsen, A., Hildebrandt, N., Schulz, K. G., and Riebesell, U. (2016). Influence of plankton community structure on the sinking velocity of marine aggregates. Global Biogeochem. Cycles 30, 1145–1165. doi: 10.1002/2016GB005372
Baines, S. B., and Pace, M. L. (1991). The production of dissolved organic matter by phytoplankton and its importance to bacteria: patterns across marine and freshwater systems. Limnol. Oceanogr. 36, 1078–1090. doi: 10.4319/lo.1991.36.6.1078
Bamstedt, U., Eilertsen, H. C., Tande, K. S., Slagstad, D., and Skjoldal, H. R. (1991). Copepod grazing and its potential impact on the phytoplankton development in the Barents Sea. Polar Res. 10, 339–353. doi: 10.1111/j.1751-8369.1991.tb00658.x
Beall, B. F., Twiss, M. R., Smith, D. E., Oyserman, B. O., Rozmarynowycz, M. J., Binding, C. E., et al. (2016). Ice cover extent drives phytoplankton and bacterial community structure in a large north-temperate lake: implications for a warming climate. Environ. Microbiol. 18, 1704–1719. doi: 10.1111/1462-2920.12819
Beszczynska-Moller, A., Fahrbach, E., Schauer, U., and Hansen, E. (2012). Variability in Atlantic water temperature and transport at the entrance to the Arctic Ocean, 1997-2010. ICES J. Mar. Sci. 69, 852–863. doi: 10.1093/icesjms/fss056
Beszczynska-Möller, A., Woodgate, R., Lee, C., Melling, H., and Karcher, M. (2011). A synthesis of exchanges through the main oceanic gateways to the Arctic Ocean. Oceanography 24, 82–99. doi: 10.5670/oceanog.2011.59
Blachowiak-Samolyk, K., Kwasniewski, S., Dmoch, K., Hop, H., and Falk-Petersen, S. (2007). Trophic structure of zooplankton in the Fram Strait in spring and autumn 2003. Deep Sea Res. II Top. Stud. Oceanogr. 54, 2716–2728. doi: 10.1016/j.dsr2.2007.08.004
Bochdansky, A. B., Clouse, M. A., and Herndl, G. J. (2017). Eukaryotic microbes, principally fungi and labyrinthulomycetes, dominate biomass on bathypelagic marine snow. ISME J. 11, 362–373. doi: 10.1038/ismej.2016.113
Boras, J. A., Sala, M. M., Arrieta, J. M., Sa, E. L., Felipe, J., Agusti, S., et al. (2010). Effect of ice melting on bacterial carbon fluxes channelled by viruses and protists in the Arctic Ocean. Polar Biol. 33, 1695–1707. doi: 10.1007/s00300-010-0798-8
Boyd, C., and Gradmann, D. (2002). Impact of osmolytes on buoyancy of marine phytoplankton. Mar. Biol. 141, 605–618. doi: 10.1007/s00227-002-0872-z
Calbet, A. (2008). The trophic roles of microzooplankton in marine systems. ICES J. Mar. Sci. 65, 325–331. doi: 10.1093/icesjms/fsn013
Calbet, A., and Saiz, E. (2005). The ciliate-copepod link in marine ecosystems. Aquat. Microb. Ecol. 38, 157–167. doi: 10.3354/ame038157
Calbet, A., Saiz, E., Almeda, R., Movilla, J. I., and Alcaraz, M. (2011). Low microzooplankton grazing rates in the Arctic Ocean during a Phaeocystis pouchetii bloom (Summer 2007): fact or artifact of the dilution technique? J. Plankton Res. 33, 687–701. doi: 10.1093/plankt/fbq142
Carlsson, P., Graneli, E., and Olsson, P. (1990). “Grazer elimination through poisoning-one of the mechanisms behind Chrysochromulina-polylepis blooms” in 4th International Conference on Toxic Marine Phytoplankton (Lund), 116–122.
Caron, D. A., Dennett, M. R., Lonsdale, D. J., Moran, D. M., and Shalapyonok, L. (2000). Microzooplankton herbivory in the Ross Sea, Antarctica. Deep Sea Res. II Top. Stud. Oceanogr. 47, 3249–3272. doi: 10.1016/S0967-0645(00)00067-9
Carstensen, J., Weydmann, A., Olszewska, A., and Kwasniewski, S. (2012). Effects of environmental conditions on the biomass of Calanus spp. in the Nordic Seas. J. Plankton Res. 34, 951–966. doi: 10.1093/plankt/fbs059
Dalpadado, P., Hop, H., Ronning, J., Pavlov, V., Sperfeld, E., Buchholz, F., et al. (2016). Distribution and abundance of euphausiids and pelagic amphipods in Kongsfjorden, Isfjorden and Rijpfjorden (Svalbard) and changes in their relative importance as key prey in a warming marine ecosystem. Polar Biol. 39, 1765–1784. doi: 10.1007/s00300-015-1874-x
Daniels, R. M., Richardson, T. L., and Ducklow, H. W. (2006). Food web structure and biogeochemical processes during oceanic phytoplankton blooms: an inverse model analysis. Deep Sea Res. II Top. Stud. Oceanogr. 53, 532–554. doi: 10.1016/j.dsr2.2006.01.016
Daufresne, M., Lengfellner, K., and Sommer, U. (2009). Global warming benefits the small in aquatic ecosystems. Proc. Natl. Acad. Sci. U.S.A. 106, 12788–12793. doi: 10.1073/pnas.0902080106
Degerlund, M., and Eilertsen, H. C. (2010). Main species characteristics of phytoplankton spring blooms in NE Atlantic and Arctic waters (68–80° N). Estuaries Coasts 33, 242–269. doi: 10.1007/s12237-009-9167-7
De Laender, F., Van Oevelen, D., Soetaert, K., and Middelburg, J. J. (2010). Carbon transfer in herbivore-and microbial loop-dominated pelagic food webs in the southern Barents Sea during spring and summer. Mar. Ecol. Prog. Ser. 398, 93–107. doi: 10.3354/meps08335
Dilling, L., and Alldredge, A. L. (2000). Fragmentation of marine snow by swimming macrozooplankton: a new process impacting carbon cycling in the sea. Deep Sea Res. I Oceanogr. Res. Papers 47, 1227–1245. doi: 10.1016/S0967-0637(99)00105-3
Dilling, L., Wilson, J., Steinberg, D., and Alldredge, A. L. (1998). Feeding by the euphausiid Euphausia pacifica and the copepod Calanus pacifica on marine snow. Mar. Ecol. Progress 170, 189–201. doi: 10.3354/meps170189
DiTullio, G. R., Grebmeier, J. M., Arrigo, K. R., Lizotte, M. P., Robinson, D. H., Leventer, A., et al. (2000). Rapid and early export of Phaeocystis antarctica blooms in the Ross Sea, Antarctica. Nature 404, 595–598. doi: 10.1038/35007061
Donali, E., Olli, K., Heiskanen, A. S., and Andersen, T. (1999). Carbon flow patterns in the planktonic food web of the Gulf of Riga, the Baltic Sea: a reconstruction by the inverse method. J. Mar. Syst. 23, 251–268. doi: 10.1016/S0924-7963(99)00061-5
Ducklow, H. W., Fasham, M. J. R., and Vézina, A. F. (1989). Derivation and analysis of flow networks for open ocean plankton systems. Netw. Anal. Mar. Ecol. 32, 159–205. doi: 10.1007/978-3-642-75017-5_8
Falk-Petersen, S., Mayzaud, P., Kattner, G., and Sargent, J. R. (2009). Lipids and life strategy of ArcticCalanus. Mar. Biol. Res. 5, 18–39. doi: 10.1080/17451000802512267
Forest, A., Wassmann, P., Slagstad, D., Bauerfeind, E., Nöthig, E. M., and Klages, M. (2010). Relationships between primary production and vertical particle export at the Atlantic-Arctic boundary (Fram Strait, HAUSGARTEN). Polar Biol. 33, 1733–1746. doi: 10.1007/s00300-010-0855-3
Fransz, H., and Gieskes, W. W. C. (1984). The unbalance of phytoplankton and copepods in the North Sea. Rapp. P.-v. Réun. Cons. Int. Explor. Mer. 183, 218–225.
Fukuda, R., Ogawa, H., Nagata, T., and Koike, I. (1998). Direct determination of carbon and nitrogen contents of natural bacterial assemblages in marine environments. Appl. Environ. Microbiol. 64, 3352–3358.
Gasparini, S., Daro, M. H., Antajan, E., Tackx, M., Rousseau, V., Parent, J. Y., et al. (2000). Mesozooplankton grazing during the Phaeocystis globosa bloom in the southern bight of the North Sea. J. Sea Res. 43, 345–356. doi: 10.1016/S1385-1101(00)00016-2
Graham, M. W., MacIntyre, S., and Alldredge, A. L. (2000). Diel variations of marine snow concentrations in surface waters and implications for particle flux in the sea. Deep Sea Res. I 47, 367–395. doi: 10.1016/S0967-0637(99)00063-1
Hansen, F. C. (1995). Trophic interactions between zooplankton and phaeocystis cf globosa. Helgolander Meeresuntersuchungen 49, 283–293. doi: 10.1007/BF02368356
Harrison, W. G., Borsheim, K. Y., Li, W. K. W., Maillet, G. L., Pepin, P., Sakshaug, E., et al. (2013). Phytoplankton production and growth regulation in the Subarctic North Atlantic: a comparative study of the Labrador Sea-Labrador/Newfoundland shelves and Barents/Norwegian/Greenland seas and shelves. Prog. Oceanogr. 114, 26–45. doi: 10.1016/j.pocean.2013.05.003
Hassett, B. T., and Gradinger, R. (2016). Chytrids dominate arctic marine fungal communities. Environ. Microbiol. 18, 2001–2009. doi: 10.1111/1462-2920.13216
Hegseth, E. N., and Tverberg, V. (2013). Effect of Atlantic water inflow on timing of the phytoplankton spring bloom in a high Arctic fjord (Kongsfjorden, Svalbard). J. Mar. Syst. 113–114, 94–105. doi: 10.1016/j.jmarsys.2013.01.003
Heinle, D. R., Harris, R. P., Ustach, J. F., and Flemer, D. A. (1977). Detritus as food for estuarine copepods. Mar. Biol. 40, 341–353. doi: 10.1007/BF00395727
Hildebrandt, N. (2014). The Response of Three Dominant Arctic Copepod Species to Elevated CO2 Concentrations and Water Temperatures, Staats-und Universitätsbibliothek Bremen. PhD thesis, Available online at: http://elib.suub.uni-bremen.de/edocs/00103786-1.pdf
Holding, J. M., Duarte, C. M., Arrieta, J. M., Vaquer-Sunyer, R., Coello-Camba, A., Wassmann, P., et al. (2013). Experimentally determined temperature thresholds for Arctic plankton community metabolism. Biogeosciences 10, 357–370. doi: 10.5194/bg-10-357-2013
Holdway, D. A., and Beamish, F. W. H. (1984). Specific growth rate and proximate body composition of Atlantic cod (Gadus morhua L.). J. Exp. Mar. Biol. Ecol. 81, 147–170. doi: 10.1016/0022-0981(84)90003-0
Hop, H., Falk-Petersen, S., Svendsen, H., Kwasniewski, S., Pavlov, V., Pavlova, O., et al. (2006). Physical and biological characteristics of the pelagic system across Fram Strait to Kongsfjorden. Prog. Oceanogr. 71, 182–231. doi: 10.1016/j.pocean.2006.09.007
Iversen, M. H., and Ploug, H. (2010). Ballast minerals and the sinking carbon flux in the ocean: carbon-specific respiration rates and sinking velocity of marine snow aggregates. Biogeosciences 7, 2613–2624. doi: 10.5194/bg-7-2613-2010
Iversen, K. R., and Seuthe, L. (2011). Seasonal microbial processes in a high-latitude fjord (Kongsfjorden, Svalbard): I. Heterotrophic bacteria, picoplankton and nanoflagellates. Polar Biol. 34, 731–749. doi: 10.1007/s00300-010-0929-2
Jackson, G. A. (1993). Flux feeding as a mechanism for zooplankton grazing and its implications for vertical particulate flux 1. Limnol. Oceanogr. 38, 1328–1331. doi: 10.4319/lo.1993.38.6.1328
Jacob, M. (2014). Influence of Global Change on Microbial Communities in Arctic Sediments. Ph, D., thesis, Universität Bremen, Alfred-Wegener-Institut.
Ji, R. B., Jin, M. B., and Varpe, O. (2013). Sea ice phenology and timing of primary production pulses in the Arctic Ocean. Glob. Chang. Biol. 19, 734–741. doi: 10.1111/gcb.12074
Jouandet, M. P., Jackson, G. A., Carlotti, F., Picheral, M., Stemmann, L., and Blain, S. (2014). Rapid formation of large aggregates during the spring bloom of Kerguelen Island: observations and model comparisons. Biogeosciences 11, 4393–4406. doi: 10.5194/bg-11-4393-2014
Jumars, P. A., Penry, D. L., Baross, J. A., Perry, M. J., and Frost, B. W. (1989). Closing the microbial loop-dissolved carbon pathway to heterotrophic bacteria from incomplete ingestion, digestion and absorption in animals. Deep Sea Res. A Oceanogr. Res. Papers 36, 483–495. doi: 10.1016/0198-0149(89)90001-0
Kahru, M., Brotas, V., Manzano-Sarabia, M., and Mitchell, B. G. (2011). Are phytoplankton blooms occurring earlier in the Arctic? Glob. Chang. Biol. 17, 1733–1739. doi: 10.1111/j.1365-2486.2010.02312.x
Kawasaki, N., Sohrin, R., Ogawa, H., Nagata, T., and Benner, R. (2011). Bacterial carbon content and the living and detrital bacterial contributions to suspended particulate organic carbon in the North Pacific Ocean. Aquat. Microb. Ecol. 62, 165–176. doi: 10.3354/ame01462
Kawasaki, T., and Hasumi, H. (2016). The inflow of Atlantic water at the Fram Strait and its interannual variability. J. Geophys. Res. Oceans 121, 502–519. doi: 10.1002/2015JC011375
Kilias, E. S., Nöthig, E. M., Wolf, C., and Metfies, K. (2014). Picoeukaryote plankton composition off west spitsbergen at the entrance to the Arctic Ocean. J. Eukaryot. Microbiol. 61, 569–579. doi: 10.1111/jeu.12134
Kirchman, D. L., Hill, V., Cottrell, M. T., Gradinger, R., Malmstrom, R. R., and Parker, A. (2009a). Standing stocks, production, and respiration of phytoplankton and heterotrophic bacteria in the western Arctic Ocean. Deep Sea Res. II Top. Stud. Oceanogr. 56, 1237–1248. doi: 10.1016/j.dsr2.2008.10.018
Kirchman, D. L., Moran, X. A., and Ducklow, H. (2009b). Microbial growth in the polar oceans-role of temperature and potential impact of climate change. Nat. Rev. Microbiol. 7, 451–459. doi: 10.1038/nrmicro2115
Klaas, C., and Archer, D. E. (2002). Association of sinking organic matter with various types of mineral ballast in the deep sea: implications for the rain ratio. Global Biogeochem. Cycles 16, 63-1–63-14. doi: 10.1029/2001GB001765
Klumpp, D. W., and von Westernhagen, H. (1986). Nitrogen balance in marine fish larvae: influence of developmental stage and prey density. Mar. Biol. 93, 189–199. doi: 10.1007/BF00508256
Kozlowski, W. A., Deutschman, D., Garibotti, I., Trees, C., and Vernet, M. (2011). An evaluation of the application of CHEMTAX to Antarctic coastal pigment data. Deep Sea Res. I Oceanogr. Res. Papers 58, 350–364. doi: 10.1016/j.dsr.2011.01.008
Kwasniewski, S., Gluchowska, M., Walkusz, W., Karnovsky, N. J., Jakubas, D., Wojczulanis-Jakubas, K., et al. (2012). Interannual changes in zooplankton on the West Spitsbergen Shelf in relation to hydrography and their consequences for the diet of planktivorous seabirds. ICES J. Mar. Sci. 69, 890–901. doi: 10.1093/icesjms/fss076
Lalande, C., Bauerfeind, E., Nöthig, E. M., and Beszczynska-Moller, A. (2013). Impact of a warm anomaly on export fluxes of biogenic matter in the eastern Fram Strait. Prog. Oceanogr. 109, 70–77. doi: 10.1016/j.pocean.2012.09.006
Lampitt, R. S., Wishner, K. F., Turley, C. M., and Angel, M. V. (1993). Marine snow studies in the Northeast Atlantic Ocean: distribution, composition and role as a food source for migrating plankton. Mar. Biol. 116, 689–702. doi: 10.1007/BF00355486
Lancelot, C., and Mathot, S. (1987). Dynamics of a Phaeocystis-dominated spring bloom in Belgian coastal waters. I. Phytoplanktonic activities and related parameters. Mar. Ecol. Prog. Ser. 37, 239–248. doi: 10.3354/meps037239
Lapoussiere, A., Michel, C., Gosselin, M., Poulin, M., Martin, J., and Tremblay, J. E. (2013). Primary production and sinking export during fall in the Hudson Bay system, Canada. Cont. Shelf Res. 52, 62–72. doi: 10.1016/j.csr.2012.10.013
Lasternas, S., and Agusti, S. (2010). Phytoplankton community structure during the record Arctic ice-melting of summer 2007. Polar Biol. 33, 1709–1717. doi: 10.1007/s00300-010-0877-x
Lavoie, M., Levasseur, M., and Babin, M. (2015). Testing the potential ballast role for dimethylsulfoniopropionate in marine phytoplankton: a modeling study. J. Plankton Res. 37, 699–711. doi: 10.1093/plankt/fbv050
Le Moigne, F. A. C., Poulton, A. J., Henson, S. A., Daniels, C. J., Fragoso, G. M., Mitchell, E., et al. (2015). Carbon export efficiency and phytoplankton community composition in the Atlantic sector of the Arctic Ocean. J. Geophys. Res. Oceans 120, 3896–3912. doi: 10.1002/2015JC010700
Li, W. K. W., McLaughlin, F. A., Lovejoy, C., and Carmack, E. C. (2009). Smallest algae thrive as the Arctic Ocean freshens. Science 326, 539–539. doi: 10.1126/science.1179798
Lochte, K., and Turley, C. M. (1988). Bacteria and Cyanobacteria associated with phytodetritus in the Deep-Sea. Nature 333, 67–69. doi: 10.1038/333067a0
Logan, B. E., Passow, U., Alldredge, A. L., Grossartt, H.-P., and Simont, M. (1995). Rapid formation and sedimentation of large aggregates is predictable from coagulation rates (half-lives) of transparent exopolymer particles (TEP). Deep Sea Res. II Top. Stud. Oceanogr. 42, 203–214. doi: 10.1016/0967-0645(95)00012-F
Matrai, P., Vernet, M., and Wassmann, P. (2007). Relating temporal and spatial patterns of DMSP in the Barents Sea to phytoplankton biomass and productivity. J. Mar. Syst. 67, 83–101. doi: 10.1016/j.jmarsys.2006.10.001
Mayor, D. J., Sanders, R., Giering, S. L., and Anderson, T. R. (2014). Microbial gardening in the ocean's twilight zone: detritivorous metazoans benefit from fragmenting, rather than ingesting, sinking detritus: fragmentation of refractory detritus by zooplankton beneath the euphotic zone stimulates the harvestable production of labile and nutritious microbial biomass. Bioessays 36, 1132–1137. doi: 10.1002/bies.201400100
McManus, G. B. (1991). Flow analysis of a planktonic microbial food web model. Mar. Microb. Food Webs 5, 145–160.
Megrey, B. A., Rose, K. A., Klumb, R. A., Hay, D. E., Werner, F. E., Eslinger, D. L., et al. (2007). A bioenergetlics-based population dynamics model of Pacific herring (Clupea harengus pallasi) coupled to a lower trophic level nutrient-phytoplankton-zooplankton model: description, calibration, and sensitivity analysis. Ecol. Modell. 202, 144–164. doi: 10.1016/j.ecolmodel.2006.08.020
Meiners, K., Gradinger, R., Fehling, J., Civitarese, G., and Spindler, M. (2003). Vertical distribution of exopolymer particles in sea ice of the Fram Strait (Arctic) during autumn. Mar. Ecol. Prog. Ser. 248, 1–13. doi: 10.3354/meps248001
Menden-Deuer, S., and Lessard, E. J. (2000). Carbon to volume relationships for dinoflagellates, diatoms, and other protist plankton. Limnol. Oceanogr. 45, 569–579. doi: 10.4319/lo.2000.45.3.0569
Metfies, K., von Appen, W. J., Kilias, E., Nicolaus, A., and Nothig, E. M. (2016). Biogeography and photosynthetic biomass of arctic marine pico-eukaroytes during summer of the record sea ice minimum 2012. PLoS ONE 11:e0148512. doi: 10.1371/journal.pone.0148512
Michaels, A. F., and Silver, M. W. (1988). Primary production, sinking fluxes and the microbial food web. Deep Sea Res. A 35, 473–490. doi: 10.1016/0198-0149(88)90126-4
Mojica, K. D., Huisman, J., Wilhelm, S. W., and Brussaard, C. P. (2016). Latitudinal variation in virus-induced mortality of phytoplankton across the North Atlantic Ocean. ISME J. 10, 500–513. doi: 10.1038/ismej.2015.130
Moloney, C. L., and Field, J. G. (1989). General allometric equations for rates of nutrient uptake, ingestion, and respiration in plankton organisms. Limnol. Oceanogr. 34, 1290–1299. doi: 10.4319/lo.1989.34.7.1290
Monti, M., and Minocci, M. (2013). Microzooplankton along a transect from northern continental Norway to Svalbard. Polar Res. 32, 1–9. doi: 10.3402/polar.v32i0.19306
Myklestad, S. M. (1995). Release of extracellular products by phytoplankton with special emphasis on polysaccharides. Sci. Total Environ. 165, 155–164. doi: 10.1016/0048-9697(95)04549-G
Nejstgaard, J. C., Tang, K. W., Steinke, M., Dutz, J., Koski, M., Antajan, E., et al. (2007). Zooplankton grazing on Phaeocystis: a quantitative review and future challenges. Biogeochemistry 83, 147–172. doi: 10.1007/s10533-007-9098-y
Niquil, N., Chaumillon, E., Johnson, G. A., Bertin, X., Grami, B., David, V., et al. (2012). The effect of physical drivers on ecosystem indices derived from ecological network analysis: comparison across estuarine ecosystems. Estuar. Coast. Shelf Sci. 108, 132–143. doi: 10.1016/j.ecss.2011.12.031
Niquil, N., Saint-Béat, B., Johnson, G. A., Soetaert, K., van Oevelen, D., Bacher, C., et al. (2011). “Inverse modeling in modern ecology and application to coastal ecosystems,” in Estuarine and Coastal Ecosystem Modelling, Vol. 9.07, Treatise on Estuarine and Coastal Science, eds E. Wolanski and D. McClusky (Elsevier), 115–133. doi: 10.1016/B978-0-12-374711-2.00906-2
Noji, T. T., Bathmann, U. V., von Bodungen, B., Voss, M., Antia, A., Krumbholz, M., et al. (1997). Clearance of picoplankton-sized partides and formation of rapidly sinking aggregates by the pteropod, Limacina reiroversa. J. Plankton Res. 19, 863–875. doi: 10.1093/plankt/19.7.863
Norrbin, F., Eilertsen, H. C., and Degerlund, M. (2009). Vertical distribution of primary producers and zooplankton grazers during different phases of the Arctic spring bloom. Deep Sea Res. II Top. Stud. Oceanogr. 56, 1945–1958. doi: 10.1016/j.dsr2.2008.11.006
Nöthig, E. M., Bracher, A., Engel, A., Metfies, K., Niehoff, B., Peeken, I., et al. (2015). Summertime plankton ecology in Fram Strait-a compilation of long- and short-term observations. Polar Res. 34:23349. doi: 10.3402/polar.v34.23349
Passow, U. (2002). Transparent exopolymer particles (TEP) in aquatic environments. Prog. Oceanogr. 55, 287–333. doi: 10.1016/S0079-6611(02)00138-6
Passow, U., and Alldredge, A. L. (1999). Do transparent exopolymer particles (TEP) inhibit grazing by the euphausiid Euphausia pacifica? J. Plankton Res. 21, 2203–2217. doi: 10.1093/plankt/21.11.2203
Passow, U., and Wassmann, P. (1994). On the trophic fate of Phaeocystis pouchetii (Hariot): IV. The formation of marine snow by P. pouchetii. Mar. Ecol. Prog. Ser. 104, 153–161. doi: 10.3354/meps104153
Pasternak, A., Arashkevich, E., Reigstad, M., Wassmann, P., and Falk-Petersen, S. (2008). Dividing mesozooplankton into upper and lower size groups: applications to the grazing impact in the Marginal Ice Zone of the Barents Sea. Deep Sea Res. II Top. Stud. Oceanogr. 55, 2245–2256. doi: 10.1016/j.dsr2.2008.05.002
Paulsen, M. L., Doré, H., Garczarek, L., Seuthe, L., Müller, O., Sandaa, R.-A., et al. (2016). Synechococcus in the Atlantic Gateway to the Arctic Ocean. Front. Mar. Sci. 3:191. doi: 10.3389/fmars.2016.00191
Peter, K. H., and Sommer, U. (2012). Phytoplankton cell size: intra- and interspecific effects of warming and grazing. PLoS ONE 7:e49632. doi: 10.1371/journal.pone.0049632
Piontek, J., Sperling, M., Nöthig, E. M., and Engel, A. (2015). Multiple environmental changes induce interactive effects on bacterial degradation activity in the Arctic Ocean. Limnol. Oceanogr. 60, 1392–1410. doi: 10.1002/lno.10112
Piontek, J., Sperling, M., Nöthig, E.-M., and Engel, A. (2014). Regulation of bacterioplankton activity in Fram Strait (Arctic Ocean) during early summer: the role of organic matter supply and temperature. J. Mar. Syst. 132, 83–94. doi: 10.1016/j.jmarsys.2014.01.003
Ploug, H., Iversen, M. H., and Fischer, G. (2008). Ballast, sinking velocity, and apparent diffusivity within marine snow and zooplankton fecal pellets: implications for substrate turnover by attached bacteria. Limnol. Oceanogr. 53, 1878–1886. doi: 10.4319/lo.2008.53.5.1878
Poulton, A. J., Daniels, C. J., Esposito, M., Humphreys, M. P., Mitchell, E., Ribas-Ribas, M., et al. (2016). Production of dissolved organic carbon by Arctic plankton communities: responses to elevated carbon dioxide and the availability of light and nutrients. Deep Sea Res. II Top. Stud. Oceanogr. 127, 60–74. doi: 10.1016/j.dsr2.2016.01.002
Reigstad, M., and Wassmann, P., (2007). Does Phaeocystis spp. contribute significantly to vertical export of organic carbon? Biogeochemistry 83, 217–234. doi: 10.1007/978-1-4020-6214-8_16
Reigstad, M., Carroll, J., Slagstad, D., Ellingsen, I., and Wassmann, P. (2011). Intra-regional comparison of productivity, carbon flux and ecosystem composition within the northern Barents Sea. Prog. Oceanogr. 90, 33–46. doi: 10.1016/j.pocean.2011.02.005
Richardson, T. L., and Cullen, J. J. (1995). Changes in buoyancy and chemical composition during growth of a coastal marine diatom: ecological and biogeochemical consequences. Mar. Ecol. Prog. Ser. 128, 77–90. doi: 10.3354/meps128077
Richardson, T. L., and Jackson, G. A. (2007). Small phytoplankton and carbon export from the surface ocean. Science 315, 838–840. doi: 10.1126/science.1133471
Richardson, T. L., Jackson, G. A., and Burd, A. B. (2003). Planktonic food web dynamics in two contrasting regions of Florida Bay, US. Bull. Mar. Sci. 73, 569–591.
Riebesell, U., Wolf-Gladrow, D. A., and Smetacek, V. (1993). Carbon dioxide limitation of marine phytoplankton growth rates. Nature 361, 249–251. doi: 10.1038/361249a0
Riser, C. W., Wassmann, P., Olli, K., Pasternak, A., and Arashkevich, E. (2002). Seasonal variation in production, retention and export of zooplankton faecal pellets in the marginal ice zone and central Barents Sea. J. Mar. Syst. 38, 175–188. doi: 10.1016/S0924-7963(02)00176-8
Rivkin, R. B., Legendre, L., Deibel, D., Tremblay, J. E., Klein, B., Crocker, K., et al. (1996). Vertical flux of biogenic carbon in the ocean: is there food web control? Science 272, 1163–1166. doi: 10.1126/science.272.5265.1163
Roca-Marti, M., Puigcorbé, V., van der Loeff, M. M. R., Katlein, C., Fernández-Méndez, M., Peeken, I., et al. (2016). Carbon export fluxes and export efficiency in the central Arctic during the record sea-ice minimum in 2012: a joint 234Th/238U and 210Po/210Pb study. J. Geophys. Res. Oceans 121, 5030–5049. doi: 10.1002/2016JC011816
Rosetta, C. H., and McManus, G. B. (2003). Feeding by ciliates on two harmful algal bloom species, Prymnesium parvum and Prorocentrum minimum. Harmful Algae 2, 109–126. doi: 10.1016/S1568-9883(03)00019-2
Rousseau, V., Becquevort, S., Parent, J. Y., Gasparini, S., Daro, M. H., Tackx, M., et al. (2000). Trophic efficiency of the planktonic food web in a coastal ecosystem dominated by Phaeocystis colonies. J. Sea Res. 43, 357–372. doi: 10.1016/S1385-1101(00)00018-6
Rudels, B., Bjork, G., Nilsson, J., Winsor, P., Lake, I., and Nohr, C. (2005). The interaction between waters from the Arctic Ocean and the Nordic Seas north of Fram Strait and along the East Greenland Current: results from the Arctic Ocean-02 Oden expedition. J. Mar. Syst. 55, 1–30. doi: 10.1016/j.jmarsys.2004.06.008
Rüger, T., and Sommer, U. (2012). Warming does not always benefit the small–Results from a plankton experiment. Aquat. Bot. 97, 64–68. doi: 10.1016/j.aquabot.2011.12.001
Sailley, S. F., Vogt, M., Doney, S. C., Aita, M. N., Bopp, L., Buitenhuis, E. T., et al. (2013). Comparing food web structures and dynamics across a suite of global marine ecosystem models. Ecol. Modell. 261–262, 43–57. doi: 10.1016/j.ecolmodel.2013.04.006
Saiz, E., Calbet, A., Isari, S., Anto, M., Velasco, E. M., Almeda, R., et al. (2013). Zooplankton distribution and feeding in the Arctic Ocean during a Phaeocystis pouchetii bloom. Deep Sea Res. I Oceanogr. Res. Papers 72, 17–33. doi: 10.1016/j.dsr.2012.10.003
Sakshaug, E., Bjorge, A., Gulliksen, B., Loeng, H., and Mehlum, F. (1994). Structure, biomass distribution, and energetics of the pelagic ecosystem in the Barents Sea-a synopsis. Polar Biol. 14, 405–411. doi: 10.1007/BF00240261
Schoemann, V., Becquevort, S., Stefels, J., Rousseau, W., and Lancelot, C. (2005). Phaeocystis blooms in the global ocean and their controlling mechanisms: a review. J. Sea Res. 53, 43–66. doi: 10.1016/j.seares.2004.01.008
Sherr, E. B., and Sherr, B. F. (2009). Capacity of herbivorous protists to control initiation and development of mass phytoplankton blooms. Aquat. Microb. Ecol. 57, 253–262. doi: 10.3354/ame01358
Sieburth, J. M. (1960). Acrylic acid, an antibiotic principle in phaeocystis blooms in antarctic waters. Science 132, 676–677. doi: 10.1126/science.132.3428.676
Smith, W. O. Jr. (1987). Phytoplankton dynamics in marginal ice zones. Oceanogr. Mar. Biol. 25, 11–38.
Soltwedel, T., Bauerfeind, E., Bergmann, M., Bracher, A., Budaeva, N., Busch, K., et al. (2016). Natural variability or anthropogenically-induced variation? Insights from 15 years of multidisciplinary observations at the arctic marine LTER site HAUSGARTEN. Ecol. Indic. 65, 89–102. doi: 10.1016/j.ecolind.2015.10.001
Spilling, K., Kremp, A., Klais, R., Olli, K., and Tamminen, T. (2014). Spring bloom community change modifies carbon pathways and C: N: P: Chl a stoichiometry of coastal material fluxes. Biogeosciences 11, 7275–7289. doi: 10.5194/bg-11-7275-2014
Stelfox-Widdicombe, C. E., Archer, S. D., Burkill, P. H., and Stefels, J. (2004). Microzooplankton grazing in Phaeocystis and diatom-dominated waters in the southern North Sea in spring. J. Sea Res. 51, 37–51. doi: 10.1016/j.seares.2003.04.004
Stoecker, D. K., and Capuzzo, J. M. (1990). Predation on protozoa: its importance to zooplankton. J. Plankton Res. 12, 891–908. doi: 10.1093/plankt/12.5.891
Straile, D. (1997). Gross growth efficiencies of protozoan and metazoan zooplankton and their dependence on food concentration, predator-prey weight ratio, and taxonomic group. Limnol. Oceanogr. 42, 1375–1385. doi: 10.4319/lo.1997.42.6.1375
Strom, S. L., Brainard, M. A., Holmes, J. L., and Olson, M. B. (2001). Phytoplankton blooms are strongly impacted by microzooplankton grazing in coastal North Pacific waters. Mar. Biol. 138, 355–368. doi: 10.1007/s002270000461
Stukel, M. R., Decima, M., Selph, K. E., Taniguchi, D. A. A., and Landry, M. R. (2013). The role of Synechococcus in vertical flux in the Costa Rica upwelling dome. Prog. Oceanogr. 112, 49–59. doi: 10.1016/j.pocean.2013.04.003
Svensen, C., Seuthe, L., Vasilyeva, Y., Pasternak, A., and Hansen, E. (2011). Zooplankton distribution across Fram Strait in autumn: are small copepods and protozooplankton important? Prog. Oceanogr. 91, 534–544. doi: 10.1016/j.pocean.2011.08.001
Svensen, C., and Vernet, M. (2016). Production of dissolved organic carbon by Oithona nana (Copepoda: Cyclopoida) grazing on two species of dinoflagellates. Mar. Biol. 163, 1–8. doi: 10.1007/s00227-016-3005-9
Tan, T. L., and Rüger, H. J. (1990). “Biomass and nutritional requirements of psychrotrophic bacterial communities in Fram Strait and Western Greenland Sea,” in Proceedings of the Fourth European Marine Micobiology Symposium (Kieler).
Turner, J. T. (2015). Zooplankton fecal pellets, marine snow, phytodetritus and the ocean's biological pump. Prog. Oceanogr. 130, 205–248. doi: 10.1016/j.pocean.2014.08.005
Ulanowicz, R. E. (2004). Quantitative methods for ecological network analysis. Comput. Biol. Chem. 28, 321–339. doi: 10.1016/j.compbiolchem.2004.09.001
Ulanowicz, R. E., and Kay, J. J. (1991). A package for the analysis of ecosystem flow networks. Environ. Softw. 6, 131–142. doi: 10.1016/0266-9838(91)90024-K
Vaquer-Sunyer, R., Duarte, C. M., Santiago, R., Wassmann, P., and Reigstad, M. (2010). Experimental evaluation of planktonic respiration response to warming in the European Arctic Sector. Polar Biol. 33, 1661–1671. doi: 10.1007/s00300-010-0788-x
Veldhuis, M. J. W., Colijn, F., and Venekamp, L. A. H. (1986). The spring bloom of phaeocystis pouchetii (haptophyceae) in Dutch coastal waters. Neth. J. Sea Res. 20, 37–48. doi: 10.1016/0077-7579(86)90059-1
Verity, P. G., and Lagdon, C. (1984). Relationships between lorica volume, carbon, nitrogen, and ATP content of tintinnids in Narragansett Bay. J. Plankton Res. 6, 859–868. doi: 10.1093/plankt/6.5.859
Verity, P. G., and Stoecker, D. (1982). Effects of Olisthodiscus-luteus on the growth and abundance of tintinnids. Mar. Biol. 72, 79–87. doi: 10.1007/BF00393951
Verity, P. G., Wassmann, P., Frischer, M. E., Howard-Jones, M. H., and Allen, A. E. (2002). Grazing of phytoplankton by microzooplankton in the Barents Sea during early summer. J. Mar. Syst. 38, 109–123. doi: 10.1016/S0924-7963(02)00172-0
Verity, P. G., Wassmann, P., Ratkova, T. N., Andreassen, I. J., and Nordby, E. (1999). Seasonal patterns in composition and biomass of autotrophic and heterotrophic nano- and microplankton communities on the north Norwegian shelf. Sarsia 84, 265–277. doi: 10.1080/00364827.1999.10420431
Vernet, M. (1991). Phytoplankton dynamics in the Barents Sea estimated from chlorophyll budget models. Polar Res. 10, 129–145. doi: 10.3402/polar.v10i1.6733
Vernet, M., Matrai, P. A., and Andreassen, I. (1998). Synthesis of particulate and extracellular carbon by phytoplankton at the marginal ice zone in the Barents Sea. J. Geophys. Res. Oceans 103, 1023–1037. doi: 10.1029/97JC02288
Vernet, M., Mitchell, B. G., Sakshaug, E., Johnsen, G., Iturriaga, R., and Wassmann, P. (1996). Evidence for a novel pigment with in vivo absorption maximum at 708 nm associated with Phaeocystis cf pouchetii blooms. Mar. Ecol. Prog. Ser. 133, 253–262. doi: 10.3354/meps133253
Vézina, A. F., and Pace, M. L. (1994). An inverse model analysis of planktonic food webs in experimental lakes. Can. J. Fish. Aquat. Sci. 51, 2034–2044. doi: 10.1139/f94-206
Vézina, A. F., and Platt, T. (1988). Food web dynamics in the ocean. 1. Best-estimates of flow networks using inverse methods. Mar. Ecol. Prog. Ser. 42, 269–287. doi: 10.3354/meps042269
Vézina, A. F., Savenkoff, C., Roy, S., Klein, B., Rivkin, R., Therriault, J. C., et al. (2000). Export of biogenic carbon and structure and dynamics of the pelagic food web in the Gulf of St. Lawrence Part 2. Inverse analysis. Deep Sea Res. II Top. Stud. Oceanogr. 47, 609–635. doi: 10.1016/S0967-0645(99)00120-4
Waite, A. M., Safi, K. A., Hall, J. A., and Nodder, S. D. (2000). Mass sedimentation of picoplankton embedded in organic aggregates. Limnol. Oceanogr. 45, 87–97. doi: 10.4319/lo.2000.45.1.0087
Walczowski, W., Piechura, J., Goszczko, I., and Wieczorek, P. (2012). Changes in Atlantic water properties: an important factor in the European Arctic marine climate. ICES J. Mar. Sci. 69, 864–869. doi: 10.1093/icesjms/fss068
Wassmann, P., Kosobokova, K. N., Slagstad, D., Drinkwater, K. F., Hopcroft, R. R., Moore, S. E., et al. (2015). The contiguous domains of Arctic Ocean advection: trails of life and death. Prog. Oceanogr. 139, 42–65. doi: 10.1016/j.pocean.2015.06.011
Wassmann, P., Reigstad, M., Haug, T., Rudels, B., Carroll, M. L., Hop, H., et al. (2006). Food webs and carbon flux in the Barents Sea. Prog. Oceanogr. 71, 232–287. doi: 10.1016/j.pocean.2006.10.003
Wassmann, P., Slagstad, D., and Ellingsen, I. (2010). Primary production and climatic variability in the European sector of the Arctic Ocean prior to 2007: preliminary results. Polar Biol. 33, 1641–1650. doi: 10.1007/s00300-010-0839-3
Wassmann, P., Vernet, M., Mitchell, B. G., and Rey, F. (1990). Mass sedimentation of Phaeocystis pouchetii in the Barents Sea. Mar. Ecol. Prog. Ser. 66, 183–195. doi: 10.3354/meps066183
Weisse, T., Grimm, N., Hickel, W., and Martens, P. (1986). Dynamics of Phaeocystis pouchetii blooms in the Wadden Sea of Sylt (German Bight, North Sea). Estuar. Coast. Shelf Sci. 23, 171–182. doi: 10.1016/0272-7714(86)90052-1
Weisse, T., Tande, K., Verity, P., Hansen, F., and Gieskes, W. (1994). The trophic significance of Phaeocystis blooms. J. Mar. Syst. 5, 67–79. doi: 10.1016/0924-7963(94)90017-5
Weydmann, A., Carstensen, J., Goszczko, I., Dmoch, K., Olszewska, A., and Kwasniewski, S. (2014). Shift towards the dominance of boreal species in the Arctic: inter-annual and spatial zooplankton variability in the West Spitsbergen Current. Mar. Ecol. Prog. Ser. 501, 41–52. doi: 10.3354/meps10694
Winder, M., and Sommer, U. (2012). Phytoplankton response to a changing climate. Hydrobiologia 698, 5–16. doi: 10.1007/s10750-012-1149-2
Wohlers, J., Engel, A., Zollner, E., Breithaupt, P., Jurgens, K., Hoppe, H. G., et al. (2009). Changes in biogenic carbon flow in response to sea surface warming. Proc. Natl. Acad. Sci. U.S.A. 106, 7067–7072. doi: 10.1073/pnas.0812743106
Wright, S. W., van den Enden, R. L., Pearce, I., Davidson, A. T., Scott, F. J., and Westwood, K. J. (2010). Phytoplankton community structure and stocks in the Southern Ocean (30–80°E) determined by CHEMTAX analysis of HPLC pigment signatures. Deep Sea Res. II Top. Stud. Oceanogr. 57, 758–778. doi: 10.1016/j.dsr2.2009.06.015
Keywords: phytoplankton, flagellates, food web, carbon cycling, inverse model
Citation: Vernet M, Richardson TL, Metfies K, Nöthig E-M and Peeken I (2017) Models of Plankton Community Changes during a Warm Water Anomaly in Arctic Waters Show Altered Trophic Pathways with Minimal Changes in Carbon Export. Front. Mar. Sci. 4:160. doi: 10.3389/fmars.2017.00160
Received: 22 November 2016; Accepted: 12 May 2017;
Published: 31 May 2017.
Edited by:
Connie Lovejoy, Laval University, CanadaReviewed by:
Robert McKay, Bowling Green State University, United StatesDouwe Maat, Royal Netherlands Institute for Sea Research (NWO), Netherlands
Copyright © 2017 Vernet, Richardson, Metfies, Nöthig and Peeken. This is an open-access article distributed under the terms of the Creative Commons Attribution License (CC BY). The use, distribution or reproduction in other forums is permitted, provided the original author(s) or licensor are credited and that the original publication in this journal is cited, in accordance with accepted academic practice. No use, distribution or reproduction is permitted which does not comply with these terms.
*Correspondence: Maria Vernet, bXZlcm5ldEB1Y3NkLmVkdQ==