- 1School of Biological Sciences, The University of Queensland, St Lucia, QLD, Australia
- 2Department of Biological Sciences, Florida International University, Miami, FL, USA
- 3Belle Baruch Institute, University of South Carolina, Columbia, SC, USA
The sediments of coastal wetlands contain large stores of carbon which are vulnerable to oxidation once disturbed, resulting in high levels of CO2 emissions that may be avoided if coastal ecosystems are conserved or restored. We used a simple model to estimate CO2 emissions from mangrove forests, seagrass beds, and tidal marshes based on known decomposition rates for organic matter in these ecosystems under either oxic or anoxic conditions combined with assumptions of the proportion of sediment carbon being deposited in either oxic or anoxic environments following a disturbance of the habitat. Our model found that over 40 years after disturbance the cumulative CO2 emitted from tidal marshes, mangrove forests, and seagrass beds were ~70–80% of the initial carbon stocks in the top meter of the sediment. Comparison of our estimates of CO2 emissions with empirical studies suggests that (1) assuming 50% of organic material moves to an oxic environment after disturbance gives rise to estimates that are similar to CO2 emissions reported for tidal marshes; (2) field measurements of CO2 emissions in disturbed mangrove forests were generally higher than our modeled emissions that assumed 50% of organic matter was deposited in oxic conditions, suggesting higher proportions of organic matter may be exposed to oxic conditions after disturbance in mangrove ecosystems; and (3) the generally low observed rates of CO2 emissions from disturbed seagrasses compared to our estimates, assuming removal of 50% of the organic matter to oxic environments, suggests that lower proportions may be exposed to oxic conditions in seagrass ecosystems. There are significant gaps in our knowledge of the fate of wetland sediment carbon in the marine environment after disturbance. Greater knowledge of the distribution, form, decomposition, and emission rates of wetland sediment carbon after disturbance would help to improve models.
Introduction
CO2 fluxes to the atmosphere from loss of blue carbon ecosystems are equivalent to 3–19% of the annual rates attributed to terrestrial land use—land cover change, and worth potentially US$ 6–42 billion (Pendleton et al., 2012). These values make fluxes from these ecosystems relevant at a global scale in climate change mitigation schemes (Pendleton et al., 2012), but rates of CO2 release need to be quantified to improve on Pendleton et al.'s order-of magnitude calculations and other recent estimates of CO2 emissions from land-use transitions (e.g., Thompson et al., 2014). Here we develop a simple model to support improved estimates of rates of CO2 fluxes from tidal marshes, mangrove forests and seagrass beds after habitat disturbances using published rates for decomposition of organic matter.
Coastal marine ecosystems contain large stocks of organic carbon stored in both biomass and sediment. Carbon stocks in the sediments of tidal marshes, mangrove forests and seagrass beds are especially large, often greatly exceeding carbon in live biomass (Morris et al., 2002; Donato et al., 2011; McLeod et al., 2011; Fourqurean et al., 2012). Under steady-state conditions, CO2 is lost from coastal marine systems due to respiration of the vegetation and through decomposition of deposited organic matter, but these losses tend to be smaller than the accumulation of organic carbon as a result of net autotrophy of these environments and sediment accumulation (Kennedy et al., 2010; Breithaupt et al., 2012; Alongi, 2014). Large carbon stocks are built up over thousands of years (Chmura et al., 2003; McKee et al., 2007) because of deposition of roots and other plant detritus (Mateo et al., 1997; McKee, 2011) and through inputs of mineral sediments and organic material that are trapped by the structure of the vegetation and incorporated into sediments (Baustian et al., 2012), and because anoxic conditions in saturated sediments slow decomposition processes (Moodley et al., 2005; Lallier-Vergès et al., 2008).
Disturbance of these ecosystems may lead to significant carbon losses and emissions of CO2 as carbon in the biomass and sediment is oxidized. Disturbances to tidal marsh, mangrove forests, and seagrass beds have been extensive (Valiela et al., 2001; Alongi, 2002; Gedan et al., 2009; Waycott et al., 2009). Losses of mangrove forests and seagrass beds are estimated at ~30% of original area (Alongi, 2002; Waycott et al., 2009). While there are no global estimates of tidal marsh losses, losses of tidal marshes has occurred over centuries, and within the world's 12 largest estuaries 67% of marshes have been lost due to land-use change (Gedan et al., 2009; Silliman et al., 2009). In mangrove forests, losses of forests have largely been due agriculture and aquaculture (shrimp and fish ponds; Alongi, 2002) where forests are cleared, wood burned, or otherwise used and sediments are drained and excavated, often increasing the exposure of sediments to air. In seagrass beds, losses have occurred due to direct disturbances such as dredging, as well as reductions in water quality associated with increased sediment supply and nutrient enrichment which reduces light reaching the benthos (Short and Wyllie-Echeverria, 1996; Waycott et al., 2009). Loss of seagrass vegetation increases the potential for erosion and sediment resuspension in the water column, which increases the exposure of seagrass sediment organic matter to oxic conditions, compared to conditions within intact sediments. Tidal marshes have been drained, converted to agricultural lands, used for peat extraction, salt works, and settlements (Gedan et al., 2009). Similar to disturbance of seagrass and mangrove forests, disturbance of tidal marshes is likely to expose sediments to conditions that enhance decomposition of organic matter leading to CO2 emissions.
Following a disturbance that destroys the aboveground vegetation, the loss of the primary production of the plant community likely leads to a switch to net heterotrophy, as decomposers continue to mineralize organic carbon stores; but rates of in-situ decomposition are likely to remain low if anoxic conditions prevail in the remaining sediments (Moodley et al., 2005). However, the loss of the vegetation in these ecosystems alters sediment properties, potentially exposing sediments to enhanced erosion, particularly at the margins of the vegetation, due to wave energy and tidal flows (Feagin et al., 2009; Anthony and Gratiot, 2011; Ganthy et al., 2011; Gedan et al., 2011; Coverdale et al., 2014). The rate of decomposition of the organic carbon in these eroded sediments could be much higher than in-situ rates if they are redeposited in oxic environments such as the surface of intertidal flats. The rapid release of carbon stores that have been built up over thousands of years has the potential to greatly surpass the annual rate of sequestration from undisturbed ecosystems; and these rapid flux rates are large enough to have high values in carbon mitigation schemes (Pendleton et al., 2012; Siikamäki et al., 2012; Thompson et al., 2014).
A wide range of factors are known to influence rates of decomposition of organic matter (Enríquez et al., 1993; Zonneveld et al., 2010; Bianchi, 2011). Decomposition rates of organic matter in coastal wetland ecosystems vary with the type of organic matter (e.g., whether leaves or roots, Middleton and McKee, 2001) and biological processes (Kristensen et al., 2008), but the environment in which decomposition occurs also strongly influences the rate of the process (Baldock et al., 2004; Blair and Aller, 2012). Fungi, especially the white-rot fungi, are the primary degraders of lignin in terrestrial systems (Sinsabaugh and Follstad Shah, 2012), while bacteria often dominate in anoxic aquatic systems (Benner et al., 1986) where decomposition is less efficient compared to carbon oxidation under oxic conditions (Kristensen et al., 1995; Baldock et al., 2004; Dai et al., 2009; Blair and Aller, 2012). Most fungi are obligate aerobes capable of degrading lignin to CO2, but are incapable of growing on lignin as a sole carbon and energy source (Griffin, 1994). Fungal dependence on oxygen partially explains the high accumulation rate of organic matter observed in anoxic sediments of blue carbon ecosystems. During disturbance of coastal wetlands one of the largest changes that can occur is the exposure of carbon previously in anoxic environments (buried) being exposed to conditions where oxidation of organic matter is enhanced (Moodley et al., 2005). Here we model CO2 emissions with variation in the quality of organic matter in different C pools (leaves, stems, sediment C) and the proportion of sediment carbon exposed to oxic conditions following disturbance in order to provide estimates, based on decomposition rates obtained from the published literature, of potential CO2 emissions with disturbance of coastal wetlands. We compare our model outputs with CO2 emissions which have been reported in the literature after ecosystem disturbance. Our goal was to assess whether modeling the proportion of organic matter exposed to oxic and anoxic conditions could provide reasonable estimates of CO2 emissions from degraded and damaged mangrove, seagrass, and tidal marsh ecosystems.
Rates of Decomposition of Organic Matter in Tidal Marsh, Mangroves, and Seagrass Ecosystems
In order to parameterize our CO2 emissions models we reviewed rates of decomposition of organic matter reported for blue carbon ecosystems. In our review we assessed decay rates for the major components of organic matter including leaf litter, and roots or rhizomes for all ecosystems and for wood in mangrove ecosystems. In addition, we also assessed decay rates for sediment organic matter. We identified decomposition rates for each component under both oxic and anoxic conditions. Although, many studies do not report oxygen availability during incubations when assessing rates of decomposition, we assumed that decomposition on the sediment surface was largely under oxic conditions while subsurface deployment of litter was under anoxic conditions.
For tidal marshes, differences in decay rates of organic matter among different Corg pools have been assessed. Morris and Lajtha (1986) fitted an empirical model with labile and refractory pools to the decay of leaf litter of four marsh species deployed in litter bags on the marsh surface and found decay constants of the labile fraction of between 0.0066 and 0.0093 d−1. Kirwan and Blum (2011) reported decay rates of 0.0041–0.0162 d−1 from surface litter bags of Spartina alterniflora. From a review by Hemminga and Buth (1991), decay rates of belowground material from a variety of species on the sediment surface ranged between 0.0012 and 0.0036 d−1. Kirwan et al. (2014) reported decay rates ranging from 0.003 to 0.0071 d−1 for roots and rhizomes deployed within sediments, presumably anoxic, while a review by Christian (1984) reported a range of rates averaging 0.0077 d−1. We conclude that rates of decay of leaf and root material in tidal marshes is greater under oxic conditions, probably by a factor of two or more, and that the decay of labile material is rapid with decay constants on the order of 0.003–0.0082 d−1 for material on the surface (oxic; Table 1).
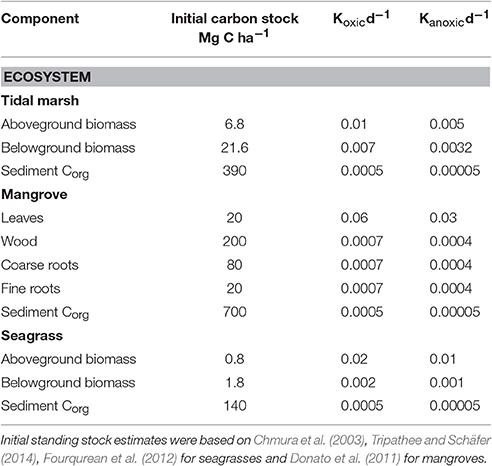
Table 1. Initial organic carbon density in different ecosystem components and the decomposition constants for each component under oxic and anoxic conditions, see text for rate justifications.
For mangrove ecosystems rates of decay of leaf litter was reviewed by Middleton and McKee (2001) who found a mean rate of 0.0064 d−1 for 17 studies. Poret et al. (2007) observed a mean decay rate of roots of ~0.002 d−1, but two-fold lower rates were observed under anoxic conditions (0.0015 d−1) compared to rates under oxic conditions (0.0028 d−1). Root decay rates reported by Poret et al. (2007) are similar to those reviewed in Middleton and McKee (2001) and Morrisey et al. (2010) which had a mean decay rate of 0.0035 d−1. No significant difference was observed for rates of decomposition among fine and coarse roots (Poret et al., 2007). However, in the study of Middleton and McKee (2001) fine roots (<2.5 mm diameter) decayed more slowly (0.001 d−1) than coarse roots (>2.5 mm diameter, 0.002 d-1) probably reflecting the high levels of aerenchyma in coarse roots compared to fine roots, which may enhance rates of decomposition. For mangrove wood, decay was more rapid on the sediment surface, approximately double compared to wood buried and therefore under more anoxic conditions (Romero et al., 2005). Labile components of wood were observed to decay relatively rapidly, while the slow decay components decayed at rates of ~0.0007 d−1, similar to those observed in Australia (Robertson and Daniel, 1989). From these studies we conclude that decay rates of all plant components would be approximately half the rate in anoxic conditions compared to oxic conditions (Table 1).
In an early review of seagrass decomposition rates (Harrison, 1989), the mean reported rate of seagrass leaf decomposition in situ was 0.02 d−1; and more recent measurements of decay rates of leaves fall in the same general range [e.g., 0.017 d−1 for Thalassia testudinum (Fourqurean and Schrlau, 2003), 0.0112–0.0152 d−1 for Zostera muelleri (Nicastro et al., 2012); and 0.011 d−1 for Thalassia hemprichii Chiu et al., 2013]. Given that decomposition rates of organic matter are faster when nitrogen and phosphorus content are higher (Enríquez et al., 1993) and that belowground plant parts generally have lower nutrient content than leaves, it is not surprising that decomposition of belowground parts is much slower than that of leaves (Holmer and Olsen, 2002; Fourqurean and Schrlau, 2003), but there are few reports of decay rates of belowground biomass. In Posidonia oceanica, rhizomes decayed at a rate of 0.0001 d−1 and below-ground leaf sheaths at a rate of 0.0002 d−1, which was two orders of magnitude slower than the decomposition of leaves at the same location (Romero et al., 1992). Similarly, decomposition rates of T. testudinum rhizomes ranged from 0.0006 d−1 (Kenworthy and Thayer, 1984) to 0.0032 d−1 (Fourqurean and Schrlau, 2003) which are 1–2 orders of magnitude slower than for leaf decomposition (0.006–0.045 d−1, Fourqurean and Schrlau, 2003; Morrisey et al., 2010); however the more metabolically-active roots decomposed faster than rhizomes (0.0065 d−1, Kenworthy and Thayer, 1984). When there is little difference in the nutrient content of leaves and belowground components, as in the small, fast-growing species Halophila decipiens, the belowground components decompose at rates similar to leaf tissue (Josselyn et al., 1986). Thus for this study, we have chosen to model the decomposition rates of belowground seagrass tissues as an order of magnitude slower than leaves of seagrass (Table 1).
The decomposition of refractory sediment organic matter has been rarely reported. Rybczyk et al. (1998) in saltmarsh reported rates of 0.0004 d−1 for surface sediment and 0.00011 d−1 for within the sediment (anoxic). These are higher rates than those assumed by Kirwan and Mudd (2012) for saltmarsh (0.00055 and 0.000027 d−1 for oxic and anoxic conditions, respectively). With few other data available we chose rates of 0.0005 d−1 under oxic, surface conditions, and 0.00005 d−1 for anoxic conditions for sediment organic matter in all three habitats (Table 1), although it may be an order of magnitude lower (Kirwan and Mudd, 2012).
Materials and Methods
We assume that we can allocate the total organic carbon stored (TCorg) in a coastal ecosystem into a number of component pools (Corg(i)) (Equation 1):
Where:
i = type of carbon (leaves, wood, stem, sediment)
For the herbaceous tidal marsh and seagrass ecosystems we used three component pools: aboveground biomass, belowground biomass, and sediment organic carbon. For mangroves we used five components: leaves, aboveground wood, coarse roots, fine roots, and sediment organic carbon. The size of the initial pools was estimated from the literature (Table 1). We also assumed that during disturbance of mangrove forests all the above ground biomass may be burned or otherwise converted to CO2 (IPCC, 2006).
For the belowground components and sediment Corg we assumed that during disturbance some fraction (α) of each component pool is deposited in two types of environments: either anoxic or oxic (Equation 2). We represented the fraction of each component deposited in oxic environments as the parameter α, with the fraction deposited in anoxic environments as 1- α. An α of 1 indicates all the component was deposited in the oxic, fast decomposing pool. An α of close to 0 would occur if Corg was deposited in an anoxic environment (e.g., intact, anoxic sediments, or deep in the ocean). In our models we vary α from 0 to 1 in order to assess the rates of decomposition of organic matter over a range of environmental conditions.
Where:
k1 is decomposition rate in oxic environments, k2 is decomposition in anoxic environments, t is time and α = fraction of Corg deposited in oxic environments. Decomposition constants for different components were obtained from the literature based on our review of decomposition rates, above (Table 1).
In our model, similar to the IPPC methods (IPCC, 2006) we assumed that disturbance alters the carbon balance of only the first meter of sediment. We assessed CO2 emissions for the first 3 years post disturbance and also assess potential emissions over a 40 year time frame, consistent with tier 1 and 2 methods of IPCC (2006) for organic sediments (IPCC, 2006).
Results
The fraction of the original carbon pool emitted in the first 3 years after disturbance was highly sensitive to α (the proportion of carbon deposited in an oxic environment). For seagrass and tidal marsh with α of 1 (all Corg exposed to oxic environments), about 40% of the original ecosystem carbon was lost in 3 years (Figure 1), while with an α of 0.5 only approximately 25% was lost in 3 years. Slightly more of the Corg stocks were lost from tidal marshes (Figure 1B) compared to seagrass meadows (Figure 1A), owing to the larger living, labile Corg pools in tidal marshes. For mangroves (Figure 2) with no biomass burning, Corg is lost at a slightly faster rate than from tidal marshes or seagrasses, such that about 30% of the original Corg is emitted after 3 years with α = 0.5 (Figure 2A). However, if we assume that the aboveground biomass is burned upon land clearing, with an α = 1, more than 50% of the original ecosystem carbon is lost in 3 years (Figure 2B). At α = 0.5, ~45% of original sediment Corg is lost in 3 years.
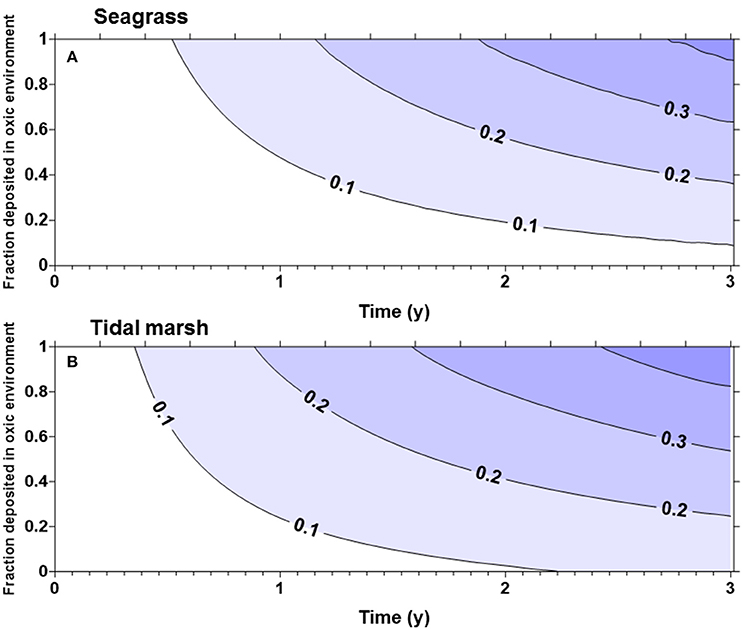
Figure 1. Fraction of the original organic carbon contained in the biomass and top meter of sediment in a seagrass bed (A) or tidal marsh (B), emitted as a function of time and α, the fraction deposited in oxic environments. More than 40% of the original organic matter will be remineralized in 3 years if all of the organic matter is deposited in oxic environments.
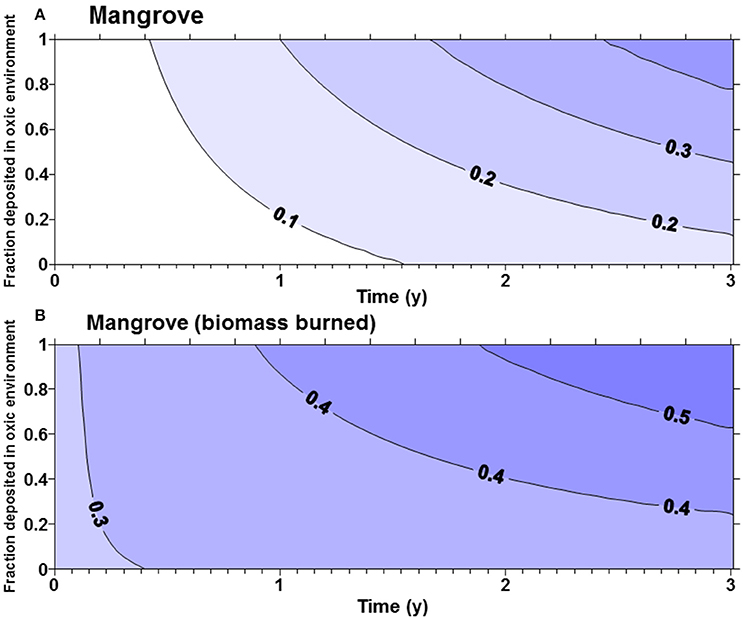
Figure 2. (A) Fraction of the original organic carbon contained in the biomass and top meter of sediment in a mangrove forest emitted as a function of time and α, the fraction deposited in oxic environments. More than 40% of the original organic matter will be mineralized in 3 years if all of the organic matter is deposited in oxic environments. (B) The fraction of the original organic carbon emitted if all aboveground biomass is burned upon land clearing of mangroves.
Using α = 0.5 we assessed trajectories of Corg remaining in the disturbed ecosystems and CO2 emissions over time. Because of the larger relative contribution of labile, living Corg stocks in mangrove ecosystems compared to tidal marshes or seagrass meadows, a smaller fraction of the original Corg stocks remain in disturbed mangrove ecosystems compared to the other ecosystems, which displayed a similar patterns in Corg remaining with time following disturbance (Figure 3). However, the 15-fold higher Corg in living biomass for an average tidal marsh compared to an average seagrass bed (Table 1), and the roughly 2x higher average Corg density in the soils underlying tidal marshes, leads to large differences in the modeled CO2 efflux from disturbed seagrass meadows and tidal marshes (Figure 4A). Our model indicates that CO2 emissions from seagrass beds are reduced from initial values of ~65 to 30 Mg CO2 ha−1y−1 after 3 years, with further reductions in emissions to 2–3 Mg CO2 ha−1y−1 from 30 to 40 years after disturbance (Figure 4A). For mangroves where no burning of biomass occurs, emissions are initially 685 Mg CO2 ha−1y−1 immediately after disturbance, decreasing to 266 Mg CO2 ha−1y−1 at 3 years and reaching rates between 10 and 15 Mg CO2 ha−1y−1 for the period 30–40 years after disturbance (Figure 4B). The combustion of aboveground biomass in mangroves greatly increases initial emissions, although lower rates are reached at ~10–20 years after forest loss.
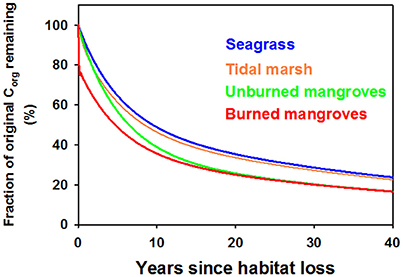
Figure 3. Model output of the fraction of the original organic carbon remaining in Blue Carbon ecosystems in the 40 years following disturbance, assuming that half of the organic carbon gets deposited in an oxic environment following disturbance (i.e., α = 0.5). Seagrasses in blue, tidal marshes in orange, mangroves where all above-ground biomass was burned (red) or the aboveground biomass was left to decompose in situ (green).
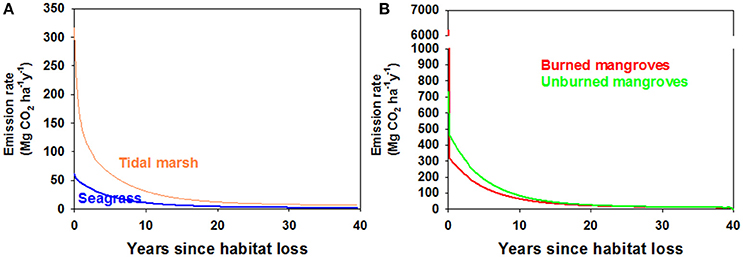
Figure 4. (A) Modeled CO2 emission rates from disturbed seagrass beds (blue) and tidal marshes (orange), with the assumption that half of the organic carbon was deposited in oxic environments (i.e., α = 0.5). (B) Modeled CO2 emission rates from disturbed mangroves where all above-ground biomass was burned (red) or the aboveground biomass was left to decompose in situ (green). The model was run with half of the sediment organic carbon deposited in an oxic environment (i.e., α = 0.5). Note the change of scale of the Y axis to accommodate very high initial CO2 emissions associated with burning of above-ground mangrove biomass.
Discussion
Our estimates of potential CO2 emissions from disturbance of tidal marsh, mangrove forests, and seagrass are within the range of observations of organic matter losses and CO2 emissions from ecosystems after disturbance. In Table 2 we summarize literature reporting CO2 emissions after disturbances based on observations of stock change and also, in mangroves, measures of gas fluxes. To facilitate comparison across studies of differing durations we have expressed CO2 emissions as a mean annual value and as a proportion of the loss of the C stock from the top 1 m of sediment per year over the duration of the study. For tidal marshes annual rates of CO2 emissions varied between 0.7 and 54 Mg CO2 ha−1 y−1 and were between 0.8 and 8% y−1 of the C stock to 1 m, with a higher rate observed in the shorter duration experiment of Macreadie et al. (2013). Our model, with α = 0.5 gave an average emission over 30 years of 10.1 Mg CO2 ha−1 y−1 or 4.3% per year of the original stock, similar to estimates of CO2 emissions from Macreadie et al. (2013) who assessed changes in sediment carbon in surface layers of the sediment. CO2 emissions were on the low end of the range of CO2 emissions estimated from (Coverdale et al., 2014) for marsh edge erosion which we would expect to have an α close to 1. CO2 emissions estimated from stock change reported in Bu et al. (2015) where marsh sediments were covered with fill (reclaimed), and which would be expected to be anoxic, were similar to the model values where α would approach zero.
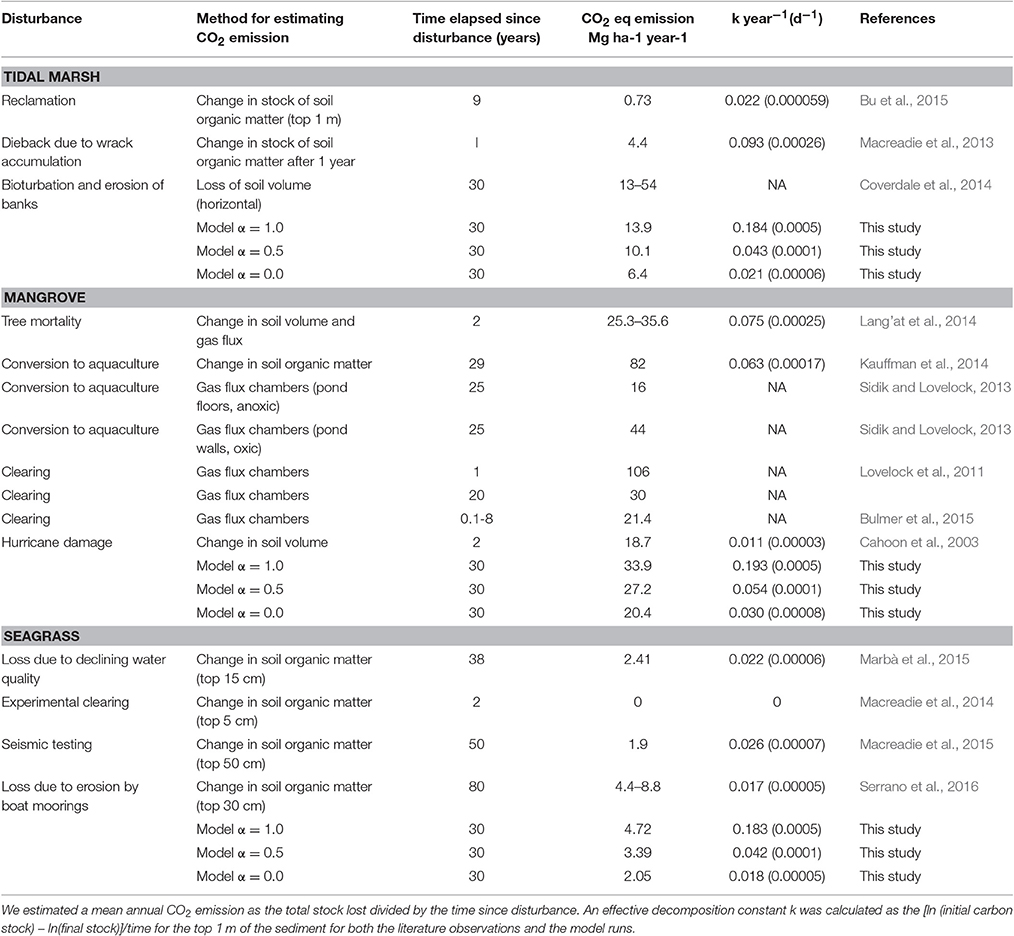
Table 2. CO2 emissions from degraded coastal wetlands reported from the literature compared to modeled results.
For mangroves, our model at α = 0.5 gave mean CO2 emissions over 30 years that were on the low end (27.2 Mg CO2 ha−1 y−1 or 5.4% per year of the original stock) of the observed range reported in the literature (16–106 Mg CO2 ha−1 y−1, Table 2). Although, high rates of CO2 emissions are expected when sediments are exposed to more oxic conditions as occurs with the construction of aquaculture ponds (Kauffman et al., 2014), forest mortality without sediment disturbance gave equivalently high estimates of CO2 emissions (e.g., Cahoon et al., 2003; Sidik and Lovelock, 2013; Lang'at et al., 2014) that often exceeded the model estimates even at α = 1. High rates of CO2 emissions in converted mangrove ecosystems may reflect the importance of additional factors that stimulate carbon remineralization under disturbed conditions (e.g., Bianchi, 2011, discussed below).
The loss of seagrass vegetation was observed to have resulted in emissions of 2.41 Mg CO2 ha−1 y−1 (0.67%y−1) within 38 years in south West Australia (Marbà et al., 2015). However, in an experimental study where small patches of seagrass were removed no losses in sedimentary C were observed (Macreadie et al., 2014). Our model predicted rates of CO2 emissions at α of 0–0.5 from seagrass that were broadly similar to those reported in the literature (i.e., 2–4 Mg CO2 ha−1 y−1, 2–4% y−1). Low levels of estimated CO2 emissions suggest that seagrass Corg may be less vulnerable to oxidation after disturbance than Corg within mangrove and tidal marsh ecosystems, as may be expected from their often lower stocks, their relative position in the intertidal zone and lower levels of exposure to air.
In our model CO2 emissions are highly sensitive to the parameter α (Figure 1), which specified the proportion of the carbon pool that is exposed to oxic conditions. In coastal wetlands the proportion of organic matter that is deposited in oxic environments is likely to vary with the type and extent of sediment disturbance and the habitat type. For example, drainage of peat deposits results in exposure of sediment organic matter to concentrations of oxygen sufficient to stimulate rapid decomposition (Couwenberg et al., 2010). The clearing of mangroves (without excavation) results in CO2 emissions from sediments probably due to changes in both the structure of sediments and microbial processes as roots die and redox conditions and nutrient availability changes (Lovelock et al., 2011; Lang'at et al., 2014). Excavation of mangrove and tidal marsh sediments to construct ponds for aquaculture may result in high initial emissions as a larger portion of the sediment carbon may be exposed to air (Kauffman et al., 2014). Additionally, the final fate of the excavated sediment can also vary giving rise to heterogeneous CO2 emissions (e.g., CO2 emissions from the walls of shrimp ponds were higher than those of pond floors, Sidik and Lovelock, 2013).
Temperature has a well-documented influence on decomposition rates of labile organic matter and release of CO2 to the atmosphere (Davidson and Janssens, 2006) and recent work in the temperate mangrove forests of New Zealand has measured an order-of-magnitude slower decomposition rate of mangrove leaves and roots than from some tropical locations, suggesting that climate can play a large role in determining the release of CO2 from disturbed mangrove forests (Morrisey et al., 2010; Gladstone-Gallagher et al., 2013). Similarly, decomposition rates and net C fluxes in seagrasses (e.g., Pedersen et al., 2011) and saltmarshes (e.g., Kirwan and Blum, 2011) are sensitive to temperature. Taken together, these observations suggest that temperature variation over latitude as well as future climate change could have a large effect on the rate of CO2 released from the labile carbon fraction of Blue Carbon ecosystems, disturbed or not. However, sensitivity of decomposition to variation in temperature may be mediated by other factors. For example, Philben et al. (2014, 2015) reported that peat formed at equatorial latitudes during the Holocene Climatic Optimum did not appear to be any more decomposed than peat formed at higher, cooler latitudes, or during subsequent cooler periods in more recent sections of the peat cores. They suggested that decomposition in peatlands was regulated primarily by oxygen-exposure time, and not by temperature.
In all three ecosystems, loss of vegetation and disturbance of sediments could lead to increases in particulate organic matter and dissolved organic matter within tidal waters which may influence CO2 emissions. Enhanced rates of C mineralization could occur due to increases the surface area of organic matter particles and thus exposure to physical (e.g., temperature, UV radiation) and biological (e.g., bacterial) agents of decomposition (Hargrave, 1972). If sediments are rich in calcium carbonate, as some seagrass sediments are (Mazarrasa et al., 2015), and calcium carbonate undergoes dissolution in the water column, then emissions may be lower since the dissolution of calcium carbonate consumes CO2 and produces bicarbonate, raising the alkalinity. Additionally, the binding of organic matter to the mineral fraction within sediments may also influence the probability of CO2 emissions (Miyajima et al., 2017).
We assumed in our model that mineralized sediment carbon is eventually emitted to the atmosphere, but this may not be the fate of all carbon that is eroded from disturbed wetlands and suspended in tidal waters. While a wide range of evidence suggests that a high proportion of the organic carbon that enters the marine environment is remineralized and that high concentration of CO2 in coastal waters, and associated degassing, is due to rapid mineralization of C derived from coastal plant communities, some proportion of this sediment carbon may be transported to deep water, offshore environments, and thus may be returned to anoxic conditions (Baldock et al., 2004; Cai, 2011; Blair and Aller, 2012; Miyajima et al., 2017). Additionally, re-fixation of CO2 by other primary producers (e.g., phytoplankton, macroalgae), which may depend on the level of nutrients and light available to support production, are also likely to be important factors determining CO2 emissions (Maher and Eyre, 2012). There are still many uncertainties as to the fate of carbon lost from disturbed wetland sediments, but evidence from the studies of terrestrial organic matter delivered to the marine environment suggest the majority of organic carbon is mineralized and emitted to the atmosphere with some proportion (25–50%) being buried in ocean sediments (Baldock et al., 2004; Cai, 2011; Blair and Aller, 2012). There are significant gaps in our knowledge of the processes that occur after disturbance of sediments and how wetland sediment carbon is distributed and decomposed over time in the marine environment. Increasing knowledge of these processes would help to constrain estimates of α and allow improved estimates of CO2 emissions from disturbing coastal wetlands.
The quality of organic matter is important to determining rates of decomposition. Fresh material (biomass) is rapidly decomposed (within days to weeks) leaving more recalcitrant compounds that decompose more slowly (Table 1; e.g., Zonneveld et al., 2010). Much of the sediment organic matter liberated during disturbance of wetlands is ancient and may be composed of mainly recalcitrant material. For example, C deposits beneath saltmarsh in Rhode Island are 2,000 years old (Donnolly and Bertness, 2001), those in Belizean mangroves up to 10,000 year old (McKee et al., 2007), and seagrass deposits in the Mediterranean up to 3,000 years old (Mateo et al., 1997). However, experiment evidence suggests that even though C stored in these sediments is old it may remain highly reactive when oxidized (Moodley et al., 2005). Thus, our use of decomposition constants for more recalcitrant carbon pools (Table 1) may underestimate rates of CO2 emissions after disturbance of wetland sediments comprised of old organic matter. Varying carbon concentrations of organic matter may also influence rates of decomposition (Enríquez et al., 1993; Romero et al., 2005) and give rise to varying potential levels of CO2 emissions after disturbance. In our model we have used mean global carbon concentration for each ecosystem, but ecosystems with high sediment carbon concentrations (e.g., Posidonia in the Mediterranean) may have higher rates of CO2 emissions after disturbance than those with mineral sediments (e.g., Halophila in the Pacific Ocean).
Our modeling approach may also under-estimate the rate of organic matter oxidation because we have assumed constant oxic conditions once sediment organic matter is disturbed. However, there is experimental evidence that oxic/anoxic transitions in muddy sediments that arise due to tidal flows may increase rates of organic matter decomposition (Abril et al., 2010). Additionally, decomposition is enhanced when higher plant material is combined with other more labile marine organic material (e.g., diatoms, Dai et al., 2009). Enhanced decomposition of organic matter after disturbance is possible where additional microbial pathways have been “primed” by altered conditions (Bianchi, 2011). The “priming” of sediment carbon decomposition pathways in the marine environment has been proposed to explain the low levels of terrestrial carbon found in marine sedimentary deposits (Bianchi, 2011). The importance of “priming” has yet to be assessed in the decomposition of coastal wetland sediment carbon in marine environments, but could increase organic matter decomposition and thus CO2 emissions in nutrient-enriched marine environments (where marine production is high). An enhanced understanding of factors that can prime decomposition of coastal wetland sediment organic carbon would also increase confidence in the decomposition rates used in our model and thus confidence in the CO2 emissions estimated for coastal wetland transitions.
Conclusions
Based on known decomposition rates of organic matter and a simple model we find that emissions of CO2 following disturbance of blue carbon ecosystems has the potential to be a large and valuable, particularly if biomass is burned (for mangroves) and if sediment organic matter is eroded and deposited in oxic environments. Our model for α = 0.5 in conjunction with documented blue carbon ecosystem loss rates over the last 20 years (McLeod et al., 2011) gives a potential global C emission of between 0.1 and 1.46 Pg C year−1, which is approximately four-fold higher than that estimated by Pendleton et al. (2012, 0.04–0.28 Pg C year−1), but similar to the shifts in the C balance documented for temperate peat lands (Armentano and Menges, 1986). CO2 emissions may be lower than estimated if organic matter is maintained in anoxic conditions (α ~ 0), if dissolution of carbonates occurs (as may occur in some seagrass sediments) or biological CO2 fixation occurs, and if organic matter is transported off-shore and reburied in anoxic environments, but if these processes are relatively limited, then CO2 emissions due to coastal wetland losses are a significant proportion of CO2 emissions associated with land-use change.
Author Contributions
All authors made substantial, direct and intellectual contribution to the work, and approved it for publication.
Conflict of Interest Statement
The authors declare that the research was conducted in the absence of any commercial or financial relationships that could be construed as a potential conflict of interest.
Acknowledgments
We thank the International Scientific Blue Carbon Working Group for their support and critical review and the Marine and Coastal Carbon Biogeochemistry Cluster, CSIRO for their support. We particularly thank Steve Crooks, Emily Pidgeon and staff of Conservation International. This is contribution #37 from the Marine Education and Research Center in the Institute for Water and Environment at Florida International University.
References
Abril, G., Commarieu, M.-V., Etcheber, H., Deborde, J., Deflandre, B., Živadinović, M. K., et al. (2010). In vitro simulation of oxic/suboxic diagenesis in an estuarine fluid mud subjected to redox oscillations. Estuar. Coast. Shelf Sci. 88, 279–291. doi: 10.1016/j.ecss.2010.04.003
Alongi, D. M. (2002). Present state and future of the world's mangrove forests. Environ. Conserv. 29, 331–349. doi: 10.1017/S0376892902000231
Alongi, D. M. (2014). Carbon cycling and storage in mangrove forests. Ann. Rev. Mar. Sci. 6, 195–219. doi: 10.1146/annurev-marine-010213-135020
Anthony, E. J., and Gratiot, N. (2011). Coastal engineering and large-scale mangrove destruction in Guyana, South America: averting an environmental catastrophe in the making. Ecol. Eng. 47, 268–273. doi: 10.1016/j.ecoleng.2012.07.005
Armentano, T. V., and Menges, E. S. (1986). Patterns of change in the carbon balance of organic soil-wetlands of the temperate zone. J. Ecol. 74, 755–774. doi: 10.2307/2260396
Baldock, J. A., Masiello, C. A., Gelinas, Y., and Hedges, J. I. (2004). Cycling and composition of organic matter in terrestrial and marine ecosystems Mar. Chem. 92, 39–64. doi: 10.1016/j.marchem.2004.06.016
Baustian, J. J., Mendelssohn, I. A., and Hester, M. W. (2012). Vegetation's importance in regulating surface elevation in a coastal salt marsh facing elevated rates of sea level rise. Glob. Change Biol. 18, 3377–3382. doi: 10.1111/j.1365-2486.2012.02792.x
Benner, R., Moran, M. A., and Hodson, R. E. (1986). Biogeochemical cycling of lingo-cellulosic carbon in marine and freshwater ecosystems: relative contributions of procaryotes and eucaryotes. Limnol. Oceanogr. 31, 89–100. doi: 10.4319/lo.1986.31.1.0089
Bianchi, T. S. (2011). The role of terrestrially derived organic carbon in the coastal ocean: a changing paradigm and the priming effect. Proc. Natl. Acad. Sci. U.S.A. 108, 19473–19481. doi: 10.1073/pnas.1017982108
Blair, N. E., and Aller, R. C. (2012). The fate of terrestrial organic carbon in the marine environment. Ann. Rev. Mar. Sci. 4, 401–423. doi: 10.1146/annurev-marine-120709-142717
Breithaupt, J. L., Smoak, J. M., Smith, T. J. III., Sanders, C. J., and Hoare, A. (2012). Organic carbon burial rates in mangrove sediments: Strengthening the global budget. Global Biogeochem. Cycles 26:GB301. doi: 10.1029/2012gb004375
Gedan, K. B., Silliman, B. R., and Bertness, M. D. (2009). Centuries of human-driven change in salt marsh ecosystems. Ann. Rev. Mar. Sci. 1, 117–141. doi: 10.1146/annurev.marine.010908.163930
Bu, N. S., Qu, J. F., Li, G., Zhao, B., Zhang, R. J., and Fang, C. M. (2015). Reclamation of coastal salt marshes promoted carbon loss from previously-sequestered soil carbon pool. Ecol. Eng. 81, 335–339. doi: 10.1016/j.ecoleng.2015.04.051
Bulmer, R. H., Lundquist, C. J., and Schwendenmann, L. (2015). Sediment properties and CO2 efflux from intact and cleared temperate mangrove forests. Biogeosciences 12, 6169–6180. doi: 10.5194/bg-12-6169-2015
Cahoon, D. R., Hensel, P., Rybczyk, J., McKee, K. L., Proffitt, E. D., and Perez, B. C. (2003). Mass tree mortality leads to mangrove peat collapse at Bay islands, Honduras after Hurricane Mitch. J. Ecol. 91, 1093–1105. doi: 10.1046/j.1365-2745.2003.00841.x
Cai, W. J. (2011). Estuarine and coastal ocean carbon paradox: CO2 sinks or sites of terrestrial carbon incineration? Ann. Rev. Mar. Sci. 3, 123–145. doi: 10.1146/annurev-marine-120709-142723
Chiu, S. H., Huang, Y. H., and Lin, H. J. (2013). Carbon budget of leaves of the tropical intertidal seagrass Thalassia hemprichii. Estuar. Coast. Shelf Sci. 125, 27–35. doi: 10.1016/j.ecss.2013.03.026
Chmura, G. L., Anisfeld, S. C., Cahoon, D. R., and Lynch, J. C. (2003). Global carbon sequestration in tidal, saline wetland sediments. Global Biogeochem. Cycles 17:1111. doi: 10.1029/2002GB001917
Christian, R. R. (1984). A life-table approach to decomposition studies. Ecology 65, 1693–1697. doi: 10.2307/1939150
Couwenberg, J., Dommain, R., and Joosten, H. (2010). Greenhouse gas fluxes from tropical peatlands in south-east Asia. Glob. Change Biol. 16, 1715–1732. doi: 10.1111/j.1365-2486.2009.02016.x
Coverdale, T. C., Brisson, C. P., Young, E. W., Yin, S. F., Donnelly, J. P., and Bertness, M. D. (2014). Indirect human impacts reverse centuries of carbon sequestration and salt marsh accretion. PLoS ONE 9:e93296. doi: 10.1371/journal.pone.0093296
Dai, J., Sun, M.-Y., Culp, R., and Noakes, J. (2009). A laboratory study on biochemical degradation and microbial utilization of organic matter comprising a marine diatom, land grass, and salt marsh plant in estuarine ecosystems. Aquat. Ecol. 43, 825–841. doi: 10.1007/s10452-008-9211-x
Davidson, E. A., and Janssens, I. A. (2006). Temperature sesativity of soil carbon decomposition and feedbacks to climate change. Nature 440, 165–173. doi: 10.1038/nature04514
Donato, D. C., Kauffman, J. B., Murdiyarso, D., Kurnianto, S., and Stidham, M. (2011). Mangroves among the most carbon-rich tropical forests and key in land-use carbon emissions. Nat. Geosci. 4, 293–297. doi: 10.1038/ngeo1123
Donnolly, J. P., and Bertness, M. D. (2001). Rapid shoreward encroachment of salt marsh cordgrass in response to accelerated sea-level rise. Proc. Natl. Acad. Sci. U.S.A. 98, 14218–14223. doi: 10.1073/pnas.251209298
Enríquez, S., Duarte, C. M., and Sand-Jensen, K. (1993). Patterns in decomposition rates among photosynthetic organisms: the importance of detritus C:N:P content. Oecologia 94, 457–471. doi: 10.1007/BF00566960
Feagin, R. A., Lozada-Bernard, S. M., Ravens, T. M., Möller, I., Yeager, K. M., and Baird, A. H. (2009). Does vegetation prevent wave erosion of salt marsh edges? Proc. Natl. Acad. Sci. U.S.A. 106, 10109–10113. doi: 10.1073/pnas.0901297106
Fourqurean, J. W., Duarte, C. M., Kennedy, H., Marbà, N., Holmer, M., Mateo, M. A., et al. (2012). Seagrass ecosystems as a globally significant carbon stock. Nat. Geosci. 5, 505–509. doi: 10.1038/ngeo1477
Fourqurean, J. W., and Schrlau, J. E. (2003). Changes in nutrient content and stable isotope ratios of C and N during decomposition of seagrasses and mangrove leaves along a nutrient availability gradient in Florida Bay, USA. Chem. Ecol. 19, 373–390. doi: 10.1080/02757540310001609370
Ganthy, F., Sottolichio, A., and Verney, R. (2011). Seasonal modification of tidal flat sediment dynamics by seagrass beds of Zostera noltii (Bassin d'Arcachon, France). J. Mar. Syst. 109–110, S233–S240. doi: 10.1016/j.jmarsys.2011.11.027.
Gedan, K., Kirwan, M., Wolanski, E., Barbier, E., and Silliman, B. (2011). The present and future role of coastal wetland vegetation in protecting shorelines: answering recent challenges to the paradigm. Clim. Change 106, 7–29. doi: 10.1007/s10584-010-0003-7
Gladstone-Gallagher, R. V., Lundquist, C. I., and Pilditch, C. A. (2013). Mangrove (Avicennia marina subsp. australasica) litter production and decomposition in a temperate estuary. New Zealand J. Mar. Freshwater Res. 48, 24–37. doi: 10.1080/00288330.2013.827124
Hargrave, B. T. (1972). Aerobic decomposition of sediment and detritus as a function of particle surface area and organic content. Limnol. Oceanogr. 17, 583–596. doi: 10.4319/lo.1972.17.4.0583
Harrison, P. G. (1989). Detrital processing in seagrass systems: a review of the factors affecting decay rates, remineralization, and herbivory. Aquat. Bot. 23, 263–288. doi: 10.1016/0304-3770(89)90002-8
Hemminga, M. A., and Buth, G. J. C. (1991). Decomposition in salt marsh ecosystems of the SW Netherlands: the effects of biotic and abiotic factors. Vegetatio 92, 73–83.
Holmer, M., and Olsen, A. B. (2002). Role of decomposition of mangrove and seagrass detritus in sediment carbon and nitrogen cycling in a tropical mangrove forest. Mar. Ecol. Prog. Ser. 230, 87–101. doi: 10.3354/meps.230087
IPCC (2006). Guidelines for National Greenhouse Gas Inventories. Prepared by the National Greenhouse Gas Inventories Programme, Edited by H. S. Eggleston, L. Buendia, K. Miwa, T. Ngara, K. Tanabe, IGES.
Josselyn, M., Fonseca, M., Niesen, T., and Larson, R. (1986). Biomass, production and decomposition of a deep-water seagrass, Halophila decipiens Ostenf. Aquat. Bot. 25, 47–61. doi: 10.1016/0304-3770(86)90039-2
Kauffman, J. B., Heider, C., Norfolk, J., and Payton, F. (2014). Carbon stocks of intact mangroves and carbon emissions arising from their conversion in the Dominican Republic. Ecol. Appl. 24, 518–527. doi: 10.1890/13-0640.1
Kennedy, H., Beggins, J., Duarte, C. M., Fourqurean, J. W., Holmer, M., Marbà, N., et al. (2010). Seagrass sediments as a global carbon sink: Isotopic constraints. Global Biogeochem. Cycles 24:GB4026. doi: 10.1029/2010GB003848
Kenworthy, W. J., and Thayer, G. W. (1984). Production and decomposition of the roots and rhizomes of seagrasses, Zostera marina and Thalassia testudinum, in temperate and subtropical marine ecosystems. Bull. Mar. Sci. 35, 364–379.
Kirwan, M. L., and Blum, L. K. (2011). Enhanced decomposition offsets enhanced productivity and soil carbon accumulation in coastal wetlands responding to climate change. Biogeosciences 8, 987–993. doi: 10.5194/bg-8-987-2011
Kirwan, M. L., Guntenspergen, G. R., and Langley, J. A. (2014). Temperature sensitivity of organic-matter decay in tidal marshes. Biogeosciences 11, 4801–4808. doi: 10.5194/bg-11-4801-2014
Kirwan, M. L., and Mudd, S. M. (2012). Response of salt-marsh carbon accumulation to climate change. Nature 489, 550–553. doi: 10.1038/nature11440
Kristensen, E., Ahmed, S. I., and Devol, A. H. (1995). Aerobic and anaerobic decomposition of organic matter in marine sediment: which is fastest? Limnol. Oceanogr. 40, 1430–1437. doi: 10.4319/lo.1995.40.8.1430
Kristensen, E., Bouillon, S., Dittmar, T., and Marchand, C. (2008). Organic carbon dynamics in mangrove ecosytems: a review. Aquat. Bot. 89, 201–219. doi: 10.1016/j.aquabot.2007.12.005
Lallier-Vergès, E., Marchand, C., Disnar, J.-R., and Lottier, N. (2008). Origin and diagenesis of lignin and carbohydrates in mangrove sediments of Guadeloupe (French West Indies): evidence for a two-step evolution of organic deposits. Chem. Geol. 255, 388–398. doi: 10.1016/j.chemgeo.2008.07.009
Lang'at, J. K., Kairo, J. G., Mencuccini, M., Bouillon, S., Skov, M. W., Waldron, S., et al. (2014). Rapid losses of surface elevation following tree girdling and cutting in tropical mangroves. PLoS ONE 9:e107868. doi: 10.1371/journal.pone.0107868
Lovelock, C. E., Ruess, R. W., and Feller, I. C. (2011). CO2 efflux from cleared mangrove peat. PLoS ONE 6:e21279. doi: 10.1371/journal.pone.0021279
Macreadie, P. I., Hughes, A. R., and Kimbro, D. L. (2013). Loss of ‘Blue Carbon’ from coastal salt marshes following habitat disturbance. PLoS ONE 8:e69244. doi: 10.1371/journal.pone.0069244
Macreadie, P. I., Trevathan-Tackett, S. M., Skilbeck, C. G., Sanderman, J., Curlevski, N., Jacobsen, G., et al. (2015). Losses and recovery of organic carbon from a seagrass ecosystem following disturbance. Proc. R. Soc. B 282:20151537. doi: 10.1098/rspb.2015.1537
Macreadie, P. I., York, P. H., Sherman, C. D., Keough, M. J., Ross, D. J., Ricart, A. M., et al. (2014). No detectable impact of small-scale disturbances on ‘blue carbon’ within seagrass beds. Mar. Biol. 161, 2939–2944. doi: 10.1007/s00227-014-2558-8
Maher, D. T., and Eyre, B. D. (2012). Carbon budgets for three autotrophic Australian estuaries: implications for global estimates of the coastal air-water CO2 flux. Global Biogeochem. Cycles 26:sGB1032. doi: 10.1029/2011GB004075
Marbà, N., Arias-Ortiz, A., Masqué, P., Kendrick, G. A., Mazarrasa, I., Bastyan, G. R., et al. (2015). Impact of seagrass loss and subsequent revegetation on carbon sequestration and stocks. J. Ecol. 103, 296–302. doi: 10.1111/1365-2745.12370
Mateo, M. A., Romero, J., Perez, M., Littler, M. M., and Littler, D. S. (1997). Dynamics of millenary organic deposits resulting from the growth of the Mediterranean seagrass Posidonia oceanica. Estuar. Coast. Shelf Sci. 44, 103–110. doi: 10.1006/ecss.1996.0116
Mazarrasa, I., Marbà, N., Lovelock, C. E., Serrano, O., Lavery, P. S., Fourqurean, J. W., et al. (2015). Seagrass meadows as a globally significant carbonate reservoir. Biogeosciences 12, 4993–5003. doi: 10.5194/bg-12-4993-2015
McKee, K. L. (2011). Biophysical controls on accretion and elevation change in Caribbean mangrove ecosystems. Estuar. Coast. Shelf Sci. 91, 475–483. doi: 10.1016/j.ecss.2010.05.001
McKee, K. L., Cahoon, D. R., and Feller, I. C. (2007). Caribbean mangroves adjust to rising sea level through biotic controls on change in sediment elevation. Glob. Ecol. Biogeogr. 16, 545–556. doi: 10.1111/j.1466-8238.2007.00317.x
McLeod, E., Chmura, G. L., Bouillon, S., Salm, R., Björk, M., Duarte, C. M., et al. (2011). A blueprint for blue carbon: toward an improved understanding of the role of vegetated coastal habitats in sequestering CO2. Front. Ecol. Environ. 9, 552–560. doi: 10.1890/110004
Middleton, B. A., and McKee, K. L. (2001). Degradation of mangrove tissues and implications for peat formation in Belizean island forests. J. Ecol. 89, 818–828. doi: 10.1046/j.0022-0477.2001.00602.x
Miyajima, T., Hori, M., Hamaguchi, M., Shimabukuro, H., and Yoshida, G. (2017). Geophysical constraints for organic carbon sequestration capacity of Zostera marina seagrass meadows and surrounding habitats. Limnol. Oceanogr. doi: 10.1002/lno.10478
Moodley, L., Middelburg, J. J., Herman, P. M. J., Soetaert, K., and de Lange, G. J. (2005). Oxygenation and organic-matter preservation in marine sediments: direct experimental evidence from ancient organic carbon–rich deposits. Geology 33, 889–892. doi: 10.1130/G21731.1
Morris, J. T., and Lajtha, K. (1986). Decomposition and nutrient dynamics of litter from four species of freshwater emergent macrophytes. Hydrobiologia 131, 215–223. doi: 10.1007/BF00008857
Morris, J. T., Sundareshwar, P. V., Nietch, C. T., Kjerfve, B., and Cahoon, D. R. (2002). Responses of coastal wetlands to rising sea level. Ecology 83, 2869–2877. doi: 10.1890/0012-9658(2002)083[2869:ROCWTR]2.0.CO;2
Morrisey, D. J., Swales, A., Dittmann, S., Morrison, M. A., Lovelock, C. E., and Beard, C. M. (2010). The ecology and management of temperate mangroves. Oceanogr. Mar. Biol. 48, 43–160. doi: 10.1201/EBK1439821169-c2
Nicastro, A., Onoda, Y., and Bishop, M. J. (2012). Direct and indirect effects of tidal elevation on eelgrass decomposition. Mar. Ecol. Prog. Ser. 456, 53–62. doi: 10.3354/meps09635
Pedersen, M. O., Serrano, O., Mateo, M. A., and Holmer, M. (2011). Temperature effects on decomposition of a Posidonia oceanica mat. Aquat. Microb. Ecol. 65, 169–182. doi: 10.3354/ame01543
Pendleton, L., Donato, D. C., Murray, B. C., Crooks, S., Jenkins, W. A., Sifleet, S., et al. (2012). Estimating global “Blue Carbon” emissions from conversion and degradation of vegetated coastal ecosystems. PLoS ONE 7:e43542. doi: 10.1371/journal.pone.0043542
Philben, M., Holmquist, J., MacDonald, G., Duan, D., Kaiser, K., and Benner, R. (2015). Temperature, oxygen, and vegetation controls on decomposition in a James Bay peatland. Global Biogeochem. Cycles 29, 729–743, doi: 10.1002/2014GB004989
Philben, M., Kaiser, K., and Benner, R. (2014). Does oxygen exposure time control the extent of organic matter decomposition in peatlands? J. Geophys. Res. Biogeosci. 119, 897–909. doi: 10.1002/2013jg002573
Poret, N., Twilley, R. R., Rivera-Monroy, V. H., and Coronado-Molina, C. (2007). Belowground decomposition of mangrove roots in Florida coastal everglades. Estuar. Coast. 30, 491–496. doi: 10.1007/BF02819395
Robertson, A. I., and Daniel, P. A. (1989). Decomposition and the annual flux of detritus from fallen timber in tropical mangrove forests. Limnol. Oceanogr. 34, 640–646. doi: 10.4319/lo.1989.34.3.0640
Romero, J., Pergent, G., Pergentmartini, C., Mateo, M. A., and Regnier, C. (1992). The detritic compartment in a Posidonia oceanica meadow. Litter features, decomposition rates and mineral stocks. Mar. Ecol. 13, 69–83.
Romero, L. M., Smith, T. J., and Fourqurean, J. W. (2005). Changes in mass and nutrient content of wood during decomposition in a south Florida mangrove forest. J. Ecol. 93, 618–631. doi: 10.1111/j.1365-2745.2005.00970.x
Rybczyk, J. M., Callaway, J. C., and Day, J. W. Jr. (1998). A relative elevation model for a subsiding coastal forested wetland receiving wastewater effluent. Ecol. Model. 112, 23–44. doi: 10.1016/S0304-3800(98)00125-2
Serrano, O., Ruhon, R., Lavery, P. S., Kendrick, G. A., Hickey, S., Masque, P., et al. (2016). Impact of mooring activities on carbon stocks in seagrass meadows. Sci. Rep. 6:23193. doi: 10.1038/srep23193
Short, F. T., and Wyllie-Echeverria, S. (1996). Natural and human-induced disturbance of seagrasses. Environ. Conserv. 23, 17–27. doi: 10.1017/S0376892900038212
Sidik, F., and Lovelock, C. E. (2013). CO2 efflux from shrimp ponds in Indonesia. PLoS ONE 8:e66329. doi: 10.1371/journal.pone.0066329
Siikamäki, J., Sanchirico, J. N., and Jardine, S. L. (2012). Global economic potential for reducing carbon dioxide emissions from mangrove loss. Proc. Natl. Acad. Sci. U.S.A. 109, 14369–14374. doi: 10.1073/pnas.1200519109
Silliman, B. R., Grosholz, T., and Bertness, M. D. (2009). Human Impacts on Salt Marshes: A Global Perspective. Berkeley, CA: University of California Press.
Sinsabaugh, R. L., and Follstad Shah, J. J. (2012). Ecoenzymatic stoichiometry and ecological theory. Annu. Rev. Ecol. Evol. Syst. 43, 313–343. doi: 10.1146/annurev-ecolsys-071112-124414
Thompson, B. S., Clubbe, C. P., Primavera, J. H., Curnick, D., and Koldewey, H. J. (2014). Locally assessing the economic viability of blue carbon: a case study from Panay Island, the Philippines. Ecosyst. Serv. 8, 128–140. doi: 10.1016/j.ecoser.2014.03.004
Tripathee, R., and Schäfer, K. V. R. (2014). Above-and belowground biomass allocation in four dominant salt marsh species of the Eastern United States. Wetlands 35, 21–30. doi: 10.1007/s13157-014-0589-z
Valiela, I., Bowen, J. L., and York, J. K. (2001). Mangrove forests: one of the world's threatened major tropical environments. Bioscience 51, 807–815. doi: 10.1641/0006-3568(2001)051[0807:MFOOTW]2.0.CO;2
Waycott, M., Duarte, C. M., Carruthers, T. J., Orth, R. J., Dennison, W. C., Olyarnik, S., et al. (2009). Accelerating loss of seagrasses across the globe threatens coastal ecosystems. Proc. Natl. Acad. Sci. U.S.A. 106, 12377–12381. doi: 10.1073/pnas.0905620106
Keywords: blue carbon, organic matter, sediment, soil, disturbance, decomposition, anoxia
Citation: Lovelock CE, Fourqurean JW and Morris JT (2017) Modeled CO2 Emissions from Coastal Wetland Transitions to Other Land Uses: Tidal Marshes, Mangrove Forests, and Seagrass Beds. Front. Mar. Sci. 4:143. doi: 10.3389/fmars.2017.00143
Received: 02 December 2016; Accepted: 27 April 2017;
Published: 15 May 2017.
Edited by:
Thomas K. Frazer, University of Florida, USAReviewed by:
Lise Lotte Sorensen, Aarhus University, DenmarkCarolyn J. Lundquist, National Institute of Water and Atmospheric Research, New Zealand
Copyright © 2017 Lovelock, Fourqurean and Morris. This is an open-access article distributed under the terms of the Creative Commons Attribution License (CC BY). The use, distribution or reproduction in other forums is permitted, provided the original author(s) or licensor are credited and that the original publication in this journal is cited, in accordance with accepted academic practice. No use, distribution or reproduction is permitted which does not comply with these terms.
*Correspondence: Catherine E. Lovelock, Yy5sb3ZlbG9ja0B1cS5lZHUuYXVs