- 1School of Marine and Atmospheric Sciences, Stony Brook University, Stony Brook, NY, USA
- 2220 McLean Laboratory, Geology and Geophysics, Woods Hole Oceanographic Institution, Woods Hole, Massachusetts, MA, USA
- 3Departamento de Oceanografía and Instituto Milenio de Oceanografia, Universidad de Concepción, Concepción, Chile
Little is still known of the impacts of protist grazing on bacterioplankton communities in the dark ocean. Furthermore, the accuracy of assessments of in situ microbial activities, including protist grazing, can be affected by sampling artifacts introduced during sample retrieval and downstream manipulations. Potential artifacts may be increased when working with deep-sea samples or samples from chemically unique water columns such as oxygen minimum zones (OMZs). OMZs are oxygen-depleted regions in the ocean, where oxygen concentrations can drop to <20 μM. These regions are typically located near eastern boundary upwelling systems and currently occur in waters occupying below about 8% of total ocean surface area, representing ~1% of the ocean's volume. OMZs have a profound impact not only on the distribution of marine Metazoa, but also on the composition and activities of microbial communities at the base of marine food webs. Here we present an overview of current knowledge of protist phagotrophy below the photic zone, emphasizing studies of oxygen-depleted waters and presenting results of the first attempt to implement new technology for conducting these incubation studies completely in situ (the Microbial Sampling- Submersible Incubation Device, MS-SID). We performed 24-h incubation experiments in the Eastern Tropical South Pacific (ETSP) OMZ. This preliminary study shows that up to 28% of bacterial biomass may be consumed by protists in waters where oxygen concentrations were down to ~4.8 μM and up to 13% at a station with nitrite accumulation where oxygen concentrations were undetectable. Results also show that shipboard measurements of grazing rates were lower than rates measured from the same water using the MS-SID, suggesting that in situ experiments help to minimize artifacts that may be introduced when conducting incubation studies using waters collected from below the photic zone, particularly from oxygen-depleted regions of the water column.
Introduction
Bacterioplankton are at the heart of marine biogeochemical cycles. Aside from bottom-up controls such as, temperature, competition, and nutrient availability, the primary top-down controls on marine bacterioplankton are viral lysis, and protist grazing (e.g., Cuevas and Morales, 2006; Chow et al., 2014). The relative contributions of viral lysis and protist phagotrophy remain debated (e.g., Pedrós Alió et al., 2000), and undoubtedly vary depending on physico-chemical conditions unique to each site, and the physiological state of individual populations within the microbial community. Either process can affect the phylogenetic diversity of bacterioplankton communities (through selective removal) and the specific activity of affected populations. For example, Detmer et al. (1993) found a significant impact of protist grazing on Synechococcus populations in Baltic Sea samples. Protist grazing and viral lysis are linked to heterotrophic bacterioplankton, in a “microbial loop” of complex interactions that drive carbon and other nutrient cycling in the world oceans (Taylor, 1982; Azam et al., 1983; and conceptually advanced over recent years, for example, in Chow et al., 2014). As much as a half or more of the total flux of matter and energy in marine food webs is thought to pass through heterotrophic bacterioplankton that utilize dissolved organic matter released from protist grazing, viral lysis, or upon cell death (Fuhrman, 1999). The mesopelagic (200–1,000 m) and bathypelagic (1,000–4,000 m) are realms of significant remineralization of organic matter, long-term carbon storage and burial (Fowler and Knauer, 1986). Organic carbon originating in the epipelagic from primary and secondary production is exported to depth through vertical fluxes of settling particles (particulate organic carbon, or POC), migration of plankton, and physical processes such as the movement of major water masses. As a result, deep ocean waters are the largest oceanic reservoir of dissolved organic carbon (Hansell and Carlson, 1998; Brenner, 2002). Up to one third of marine biological CO2 production is accounted for by respiration of this pool of carbon in dark pelagic layers (del Giorgio and Duarte, 2002; Arístegui et al., 2005). Quantifying accurately the impacts of protist grazing in the dark ocean at different locations is therefore critical to our understanding of the impacts of this component of the microbial loop and of the role of deep ocean waters in marine biogeochemical cycling.
Mesopelagic, and in some cases bathypelagic waters, are experiencing oxygen decline in many areas of the world oceans. Climate-change driven alterations in ocean thermohaline circulation, decreasing O2 storage capacity of warming waters, and imbalances between remineralization of sinking biogenic material and ventilation of subsurface waters all contribute to decreases in dissolved oxygen in marine waters. Oceanic oxygen minimum zones (OMZs) are typically located near eastern boundary upwelling systems. Some of the most prominent OMZs include the northern Indian Ocean and Arabian Sea, and the eastern North and South Pacific. Oxygen concentrations in offshore, subsurface waters have been declining over the last 50 years, and the vertical and horizontal extent of these oxygen-depleted regions has increased (Stramma et al., 2008). Coastal OMZs exist in locations where water circulation is reduced and/or oxygen-depleted conditions are exacerbated by anthropogenic additions of nutrients to coastal waters. OMZ oxygen concentrations range between ~20 μM and undetectable levels (Ulloa and Pantoja, 2009). OMZs cover about 8% of ocean surface area (Paulmier and Ruiz-Pino, 2009) and ~1% of the ocean volume (Ulloa and Pantoja, 2009). Recently developed sensor technology (Revsbech et al., 2009) has revealed that significant portions of major OMZs are anoxic (Thamdrup et al., 2012; Ulloa et al., 2012); here nitrite, a product of the anaerobic process of nitrate reduction, accumulates.
OMZs vary in intensity and duration, and have a profound impact not only on the distribution of marine Metazoa, but also on microbial nutrient cycling (e.g., carbon and nitrogen marine biogeochemical cycles) at the base of marine food webs. OMZs cause shifts in prokaryotic and eukaryotic microbial communities and biogeochemical processes favoring anaerobic respiratory and fermentative processes. Such metabolic shifts can lead to nitrogen loss by denitrification and anammox (e.g., Thamdrup et al., 2006; Lam et al., 2009). Most studies of biogeochemical cycling in OMZs have focused on prokaryotic (archaeal and bacterial) activities because large multicellular eukaryotes cannot permanently occupy anoxic marine waters (Parris et al., 2014). Nevertheless, a diverse community of microeukaryotes and even larger taxa (such as zooplankton) can potentially adapt to oxygen limitation and therefore play important roles in OMZ ecology. For example, vertically-migrating crustacean, zooplankton, chaetognaths and even fish can seek temporary refuge from predation in low-oxygen regions of OMZs (Wishner et al., 1998, 2013; Escribano et al., 2009). In addition, diverse communities of phagotrophic and parasitic protists are described from oxygen-depleted and anoxic water columns, many of which exhibit complex relationships with prokaryotes (Lin et al., 2007; Edgcomb et al., 2011; Orsi et al., 2011, 2012; Wright et al., 2012).
Knowledge of protistan dynamics in oxygen-depleted water columns is still poor. There is a lack of information on the impact of the protist grazing on prokaryotes due to the challenges in obtaining accurate assessments of in situ microbial activities and responses to environmental change (Taylor et al., 2015). Here we present an overview of the current state of knowledge on protist phagotrophy in the dark ocean (below the photic zone), including studies of oxygen-depleted waters. In addition, we present preliminary data on phagotroph activity in the Eastern Tropical South Pacific (ETSP) OMZ collected using recently-developed in situ incubation technology; the Microbial Sampling- Submersible Incubation Device (MS-SID). The motivation for development of this in situ incubation and sample preservation device was to minimize artifacts introduced by sample recovery from Niskin bottles. This was the first time completely in situ methods have been applied to studies of protist phagotrophy in OMZ waters. While these data are based on only a few samples due to technical challenges encountered during the first deployments of this instrument on a free-floating mooring (Bombar et al., 2015), they provide useful insights on the value of applying in situ approaches for researchers planning to conduct future studies of phagotrophy in the dark ocean.
Methodologies Used for Calculating Protist Grazing
In the last 30 years, several studies have been conducted to reveal the impact of protist grazing on bacterial communities in the ocean. Various methodologies used by different researchers, such as, the uptake of fluorescently labeled prey (FLP; Vazquez-Dominguez et al., 1999), the dilution method (Landry et al., 1995), the size-fractionation technique (Wright and Coffin, 1984), the use of metabolic inhibitors of protists (Sherr et al., 1986), or the radioactive labeling of prey (Hollibaugh et al., 1980). Consequently, results from most studies are not directly comparable. Nonetheless, general observations can be made about the impact of protists on prokaryotic populations in different locations and at different depths.
The most common method to determine protistan grazing on bacteria involves measuring the uptake of FLP (Vazquez-Dominguez et al., 1999). The use of FLP (often prepared from a commercially available cultured bacterium) allows for calculation of specific ingestion rates for particular classes of protists in short-term (~1 h) incubations by microscopic measurements of the accumulation of FLP inside food vacuoles (Jürgens and Massana, 2008). This approach also allows calculation of overall grazing rates for a whole community by measuring the disappearance of FLP in long-term (12–24 h) incubations (Marrasse et al., 1992; Vazquez-Dominguez et al., 1999). These long-term experiments are less labor-intensive, but also yield less specific information. The FLP approach has the potential to introduce different potential artifacts due to feeding biases against prey of particular sizes, morphologies or heat-killed prey (Marrasse et al., 1992; Vazquez-Dominguez et al., 1999; Fu et al., 2003). While the FLP technique has a number of drawbacks (e.g., noted in McManus and Okubo, 1991; Vaque et al., 1994; Fu et al., 2003) one of the major advantages is that the observed grazing effect can only be attributed to the predators examined, because the method is based on direct enumeration of the prey analogs (Oikonomou et al., 2014). Care must be taken however to avoid photo-bleaching of the fluorochrome in the FLP during the incubation study if it is conducted within the photic zone. As we found during shallow 13-m incubations (see below), this makes downstream analysis and data interpretation extremely difficult.
Another potential methodological bias of the FLP approach may occur due to shifts in total available prey concentrations once FLP are added. Generally, FLP are added at 10% of the indigenous prokaryotic concentrations (Vazquez-Dominguez et al., 1999). While the 10% target may be debated, it is generally accepted that if added FLP represent a higher percentage of the indigenous population, this significantly changes the microbial population size and thus, can impact the measured grazing rate. On the other hand, if the relative FLP concentration is too low, grazing may be undetectable over the time course of the experiment (Vazquez-Dominguez et al., 1999).
Studies of Protist Grazing in Normoxic Marine Water Column Samples Collected from Below the Photic Zone
Cho et al. (2000) used fluorescently labeled bacterial prey prepared from indigenous populations and short-term (0.5 h), on-board experiments to measure grazing rates of heterotrophic nanoflagellates (HNF) in water samples collected from 0 to 500 m depth in the East China Sea. These authors found that HNF ingestion rates were not statistically different between the mesopelagic and the epipelagic zones. However, HNF clearance rates were often higher in mesopelagic waters during several months of the study. They suggested that HNF could not only survive in the mesopelagic, but that they can be active consumers of bacteria and other particles in that realm. Additionally, mesopelagic HNF may adapt their physiological state to conditions found in the mesopelagic. That study suggests protist grazing could play an important role in carbon cycling in the mesopelagic environment.
Rocke et al. (2015) conducted on-board, long-term (24 h) incubation experiments using waters from the epipelagic, mesopelagic and bathypelagic North Atlantic collected via Niskin sampling, and FLP prepared from whole seawater samples from another location (Vineyard Sound, MA, USA). Estimation of grazing rates (prey consumed per eukaryote per hour) were not greatly different between the euphotic, 220 and 750 m. Similar percentages (up to 30%) of the standing stock of prokaryotes were consumed by eukaryotes in the mesopelagic (750 m) and at 2,500 m, despite lower eukaryote and prokaryote concentrations. Those authors emphasize the point that these results suggest a potentially important role of the protist community in biogeochemical cycling in deep water layers through their impact on carbon turnover and potentially through their control of key prokaryotic prey communities.
Pachiadaki et al. (2014) used a recently-designed instrument, the MS-SID, to estimate protist phagotrophy in euphotic, mesopelagic, and oxygen-depleted bathypelagic (discussed below) waters of the Mediterranean Sea. The MS-SID is an autonomous micro-laboratory that is simultaneously capable of conducting tracer incubation experiments in situ at user-chosen depths and preserving large volume microbial samples. The MS-SID consists of a 2-L syringe-like incubation chamber, a Fluidic Distribution Valve (FDV), a syringe pump-like Tracer Injector for accurate delivery of a specified volume of tracer (in this case FLP) into the sample to be incubated, and 48 Fixation Filter Units (FFUs Taylor et al., 2015) or flexible Restek® 5-layer (outer nylon layer, metalized aluminum, polyethylene, aluminum foil, inner polyethylene layer) bags containing preservative for collection and in situ preservation (Pachiadaki et al., 2014). In that study, total prokaryotic and protist abundances were measured and estimates of phagotrophy were made using samples that were fixed in situ following incubation studies employing FLP prepared from indigenous microbiota. This is the only previous study that has made use of the MS-SID for calculating grazing impacts. Results from mesopelagic incubations showed a decline in the prokaryotic turnover rate with depth, ranging from 31.37 ± 5.9% of prokaryotic cell abundance at 40 m to 0.57 ± 0.3% at 950 m, however the grazing effect showed an increase from 5,790 cell mL−1 h−1 at the euphotic zone to 13,600 cell mL−1 h−1 in the anoxic depth. That study indicated HNF appear to be the main grazers in the euphotic and in the mesopelagic zones, supporting the notion that in oligotrophic water columns flagellates have evolved adaptations to improve their methods for capturing bacteria prey.
Studies of Protist Grazing in Oxygen-Depleted Marine Water Column Samples Collected from below the Photic Zone
Studies have revealed difficulties associated with accurately assessing in situ microbial activities and their physiological responses in environments where oxygen is either very low or absent, because oxygen contamination is difficult to control when using Niskin bottles and transferring samples to incubation vessels (De Brabandere et al., 2012; Edgcomb et al., 2016). Small amounts of oxygen contamination and/or pressure changes can create stresses on a community from oxygen-depleted or anoxic water columns (Stewart et al., 2012).
In some cases, oxygen contamination may increase grazing activity, depending on the composition of the microbial consortium. For example, Detmer et al. (1993) measured the uptake rates of FLP by mesopelagic protists and estimated grazing effects in the Baltic Sea. Those authors found that grazing impacts in anoxic waters were at least 50% of prey production, and oxygen contamination may result in enhanced growth and grazing rates of facultative anaerobic organisms. As a result, grazing activities occurring in anoxic water columns may be underestimated in experiments where oxygen introduction may stimulates prey growth. Anderson et al. (2012) conducted on-board incubations using water collected at the oxic-anoxic interface of the Baltic Sea and measured the disappearance of FLP. They found that 50–80% of the prokaryotes were grazed daily.
Similar values were reported by Pachiadaki et al. (2014) from in situ incubation studies using the MS-SID in suboxic and anoxic waters in the Mediterranean Sea. They found that elevated concentrations of prokaryotes and heterotrophic nanoflagellates (HNF) within a bathypelagic oxycline water column above a deep hypersaline anoxic basin coincided with a high phagotrophic impact of the protistan community, reaching a grazing effect higher than that observed for the normoxic upper mesopelagic, and demonstrated protist grazing can be significant in oxygen-depleted water columns.
In contrast, using predator exclusion methods, a low impact of protist grazing was observed in water samples collected from the redoxcline of the Cariaco Basin at 400 m depth. It remains to be tested whether in situ approaches would have detected a greater grazing impact within the redoxcline of the Cariaco Basin. Those researchers did detect shifts in major phylogenetic groups upon removal of grazers, indicating selective grazing (Lin et al., 2007).
Cuevas and Morales (2006) conducted shipboard grazing experiments at both oxic and suboxic sites at two stations along the North coast of Chile using two different approaches [studies of food vacuolar content (FVC) and studies applying the Selective Inhibitor Technique (SIT)] to try to reduce disturbance of the organisms and to improve the maintenance of in situ oxygen conditions. Samples were collected from 10 to 12 depths using a Niskin rosette. The FVC studies quantified the content of cyanobacterial prey in food vacuoles of heterotrophic protists. The SIT approach focused on depths representing the oxic (~10 m) and suboxic layers (~60 m), immediately below the shallow oxycline, and utilized the eukaryotic inhibitor cycloheximide. At one sampling station bottle incubations were placed within an array and re-deployed at the depths from which the waters originated. In their studies the grazing impact on bacteria was higher in the suboxic layer (40–50 m) where removal of bacterial prey via grazing equaled the growth of the bacteria. In the surface oxic layer (15 m) net bacterial growth did occur, and grazing rates were negligible (<0.1 h−1). Grazing by HNF on cyanobacteria (Synechococcus) occurred at similar rates (0.2 h−1) in the oxic and the shallower suboxic layer (40 m) but was lower (<0.1 h−1) in the deeper suboxic layer (50 m). This may simply be a function of lower abundances of Synechococcus found at the deeper depths.
Oikonomou et al. (2014) quantified the protistan grazing effect in the sulfidic anoxic monimolimnion (at 22 m depth) of Lake Alatsee (Germany), and found that turnover rate in the anoxic bottom water of the lake was negligible (0.37% d−1), and bacterial loss was attributed principally to viruses. Along the oxycline (18 m), higher prokaryotes and protist abundances were observed, however the impact of grazing was only 2.13% d−1, suggesting that prokaryote communities were not controlled by top-down processes at this interface. Although this is a freshwater environment and methodologies are variable between studies, the anoxic conditions make an interesting comparison with results from anoxic marine water columns.
A Preliminary Study of Phagotrophy in the ETSP OMZ Using In situ Sampling and Incubation Approaches
Recently, we applied the MS-SID on a free-floating mooring system at two stations within the ETSP OMZ off the coast of Chile to examine impacts of protist grazing in an OMZ using in situ approaches. Initially, 48-h deployments were planned (two 24-h consecutive incubation studies at each depth). Due to technical difficulties associated with new software for the autonomous operation, only a limited number of in situ incubation studies could be completed. We therefore interpret these results with caution, and acknowledge additional future replicated experiments are necessary. However, these preliminary results are presented in this review as a means of introducing the potential value of utilizing in situ technologies for studies of protist grazing.
Methods
Site Description
The ETSP is considered one of the most productive regions in the world's oceans due to intense upwelling processes along the western coast of South America that introduce nutrient-rich water to the surface, promoting an increase in primary production (Stevens and Ulloa, 2008). Here, the water column is poorly ventilated below the surface and characterized by low levels of dissolved oxygen that can decline from 250 μM at the surface to undetectable levels, <10 nM at the OMZ core (Thamdrup et al., 2012; Ulloa et al., 2012), making it one of the most significant OMZ regions in the world (Ulloa et al., 2012). Our preliminary study using in situ approaches was conducted during the “Lowphox I” cruise on board the R/V Cabo de Hornos from Valparaiso to Iquique along the coast of northern Chile (Figure 1), between November 20th and December 14th, 2015. Two stations were chosen for conducting grazing experiments. One station, Station T3 (20° 06'007 S, 070° 25'215 W) was located ~20 kilometers offshore directly over the central portion of the OMZ, and exhibited nitrite accumulation and undetectable levels of oxygen at the core of the OMZ. The second station, OMZ Station L6 (29° 29'949, 71° 36'643) was located near the coast (where particle load measurements and microbial activity were high) ~1053 kilometers south of OMZ Station T3, away from the central portion of the OMZ. OMZ Station L6 exhibited no nitrite accumulation, and only a few micromoles per liter of oxygen (Figure 2).
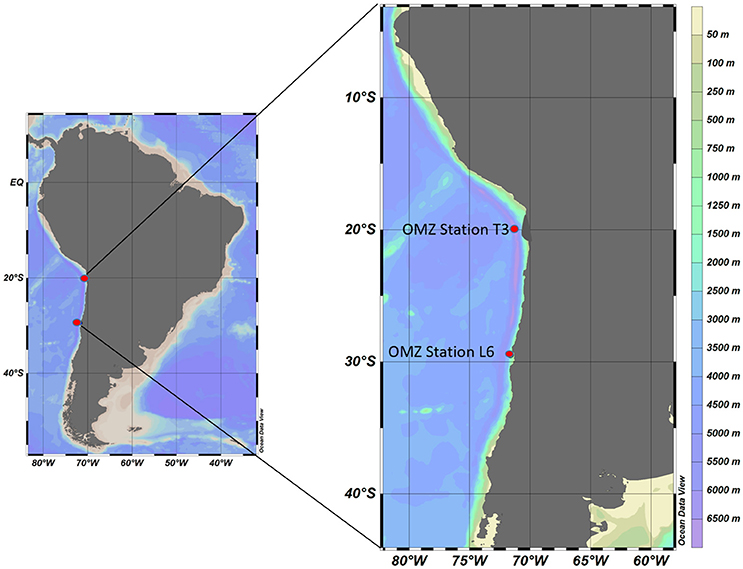
Figure 1. Location of sampling and MS-SID deployment sites in the ETSP OMZ off the northern coast of Chile.
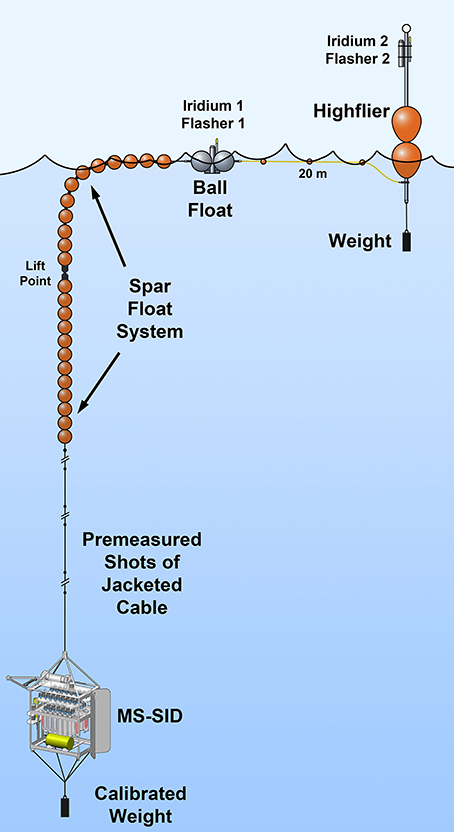
Figure 2. The MS-SID as deployed on a free-drifting spar float system used in this study of ETSP OMZ protist grazing.
Preparation of the Fluorescently-Labeled Prey (FLP)
FLP were prepared according to Pachiadaki et al. (2014), with minor modifications. Water samples were collected into Niskin bottles at each station from the depth of intended incubations (13 and 150 m). Two 5-ml replicates were taken from those samples and fixed with glutaraldehyde (1% final concentration) to count indigenous prokaryote cells using a flow cytometer on board. Twenty liter samples were pre-filtered through a 2.7 μm pore size glass fiber filters to exclude protists and larger eukaryotes, and prokaryotic cells were concentrated by tangential flow filtration (Pellicon System, Millipore Co. equipped with a Biomax 500 K polyethersulfone membrane). Concentrated cells were centrifuged at 12,000 rpm for 5 min and pelletized cells were re-suspended in Na2CO3/NaHCO3 buffer (pH: 9.5) before subsequent staining with the fluorescent stain 5-(4,6-dichlorotriazinyl) aminofluorescein (DTAF) at a final concentration of 0.8 mg mL−1. The mixture was incubated at 65°C for 2.5 h. Staining was followed by 3 washing steps, using Na2CO3/NaHCO3 buffer (pH: 9.5) to remove excess DTAF. Enumeration of prey analogs was performed via microscopic analyses.
Specification and Deployment of the MS-SID
Because two 24-h consecutive incubations would be conducted at each depth at each site, it was necessary to deploy the MS-SID on a free-drifting spar float system (Figure 2; modified from the version shown in Bombar et al., 2015) so that hydrocasting and other ship operations could proceed normally. This cruise was the first deployment of the MS-SID for autonomous tracer incubation studies. Each incubation study consisted of the following instrument routine: (1) Three pre-incubation flushes of the incubation chamber were performed by drawing ~100 mL of water from the environment and immediately expelling that back into the environment. Approximately 9 mL of the 100 mL flush volume remained after each flush. (2) After flushing, a 1-L sample was drawn into the incubation chamber and during this process a precise volume of the tracer (FLP cells) was injected via the Tracer Injector into the incoming sample, effectively mixing the tracer with the sample. (3) Once the chamber was filled to the desired volume, the incubation began, and subsamples (200 mL) were expelled from the chamber at T0 and then at 12-h intervals into sample bags containing glutaraldehyde (2% final concentration). (4) Upon completion of the incubation, remaining sample within the chamber was expelled into the environment and the chamber was flushed four times to remove remaining tracer.
A single 24-h near-surface incubation (13 m depth) and a single 24-h incubation at 150 m (depth of the turbidity maximum) were successfully executed in situ at OMZ Station T3. Due to photo-bleaching of the FLP during the un-shaded 13-m incubation and associated difficulties visualizing FLP in subsamples, results from those experiments will not be discussed further. Due to time constraints, an additional 24-h incubation using Niskin water from 150-m depth from OMZ Station T3 was conducted in the incubation chamber of the MS-SID immediately after returning the first 150-m MS-SID in situ incubation to the ship's deck. Niskin water was transferred to the MS-SID's incubation chamber on the ship's deck to be as consistent as possible between experiments intended to provide insight into potential biases associated with retrieving waters to the surface prior to incubations. Water was transferred by dispensing Niskin water through tubing into the bottom of a glass bottle pre-gassed with nitrogen, allowing the bottle to overflow for a couple of minutes, capping, and then inserting the input tubing for the MS-SID into the bottom of this bottle. This does not completely eliminate sources of potential oxygen intrusion, and is representative of typical approaches used to transfer water from Niskins to incubation bottles. The chamber was shaded during incubation, surrounded with ice packs and monitored to preserve water temperatures found at 150 m over the course of the experiment. Subsampling was conducted exactly the same as for in situ incubations. At OMZ Station L6 we conducted a pair of 24-h in situ incubations at 150 m (Table 1).
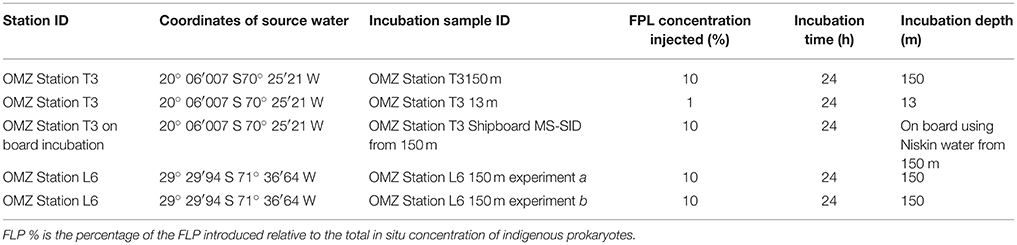
Table 1. Station information, and incubation experiment features for grazing studies conducted using waters collected from the ETSP OMZ.
After retrieval of the instrument when incubations were conducted in situ, or in the case of the on-deck incubation, following completion of the subsampling and fixation routine, the fixed subsamples (~200 mL) were collected from the 1L Restek® 5-layer bags and filtered onto 0.6 μm Millipore Isopore membrane filters for microscope estimations of total eukaryotic, prokaryotic and FLP abundances at all-time points. The equations from Salat and Marrase (1994) were used to calculate daily grazing (number of prokaryotes mL−1 day−1) by the total protistan community. The grazing effect (number of prokaryotes mL−1 h−1) was calculated by dividing the daily grazing by 24 h. The grazing rate (number of prokaryotes grazer−1 h−1) was calculated by dividing the grazing effect by the numbers of eukaryotes present in each sample.
Epifluorescence Microscopy
Initial and final abundances of FLP, eukaryotic, and prokaryotic populations from all incubations, were enumerated in triplicate using epifluorescence microscopy. A portion of each filter (~one quarter segment) was stained with 1 μg mL-1 DAPI (4′,6-diamidino-2-phenylindole, dihydrochloride) (Porter and Feig, 1980). The stained filters were mounted with immersion oil onto glass slides and observed with at 1,000x magnification. Microscopic eukaryotes and prokaryotes were counted using the DAPI filter set. FLP were counted using the FITC (fluorescein isothiocyanate) filter. For eukaryotes, prokaryotes and FLP 20 fields of view were counted on three different filter segments and results were averaged. For protists, numbers counted over 20 fields ranged from 44 to 101. The accuracy of DAPI counts for eukaryotes was validated with fluorescence in situ hybridization (FISH) using the general eukaryotic probe Euk1209 and methods according to Pernthaler et al. (2001).
Results
Hydrographic Conditions
Vertical profiles of temperature, salinity, dissolved oxygen and nitrite for both stations are presented in Figure 3. The water column at OMZ Station L6 was characterized by a steep thermocline from the surface (15.65°C) to 40 m (12.84°C), then temperature remained stable down to 190 m (12.60 ± 0.06°C), followed by a small decrease until 350 m. Salinity values, computed from the CTD conductivity using the practical salinity scale (PSU), did not vary significantly (mean 34.742 ± 0.1), but a halocline was observed between the surface (34.36) and 50 m (34.71), remaining stable down to 350 m. Station L6 was selected because it was away from the central portion of the OMZ and not anoxic, did not have measureable accumulated nitrite, yet had low oxygen concentrations with a steep vertical gradient. Oxygen values decreased from 280 μM at the surface to down to ~3 μM at the OMZ core, where nitrite concentrations were <0.1 μM.
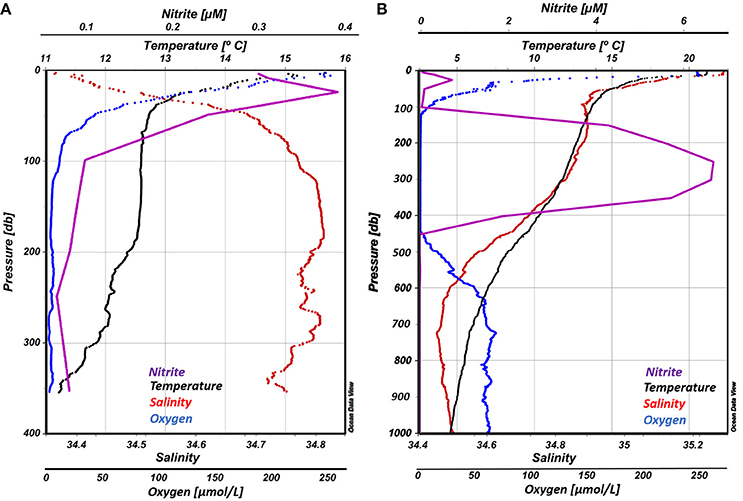
Figure 3. Vertical profiles of temperature, salinity dissolved oxygen and nitrite for both ETSP OMZ stations in this study: (A) OMZ Station L6, left panel; (B) OMZ Station T3, right panel.
Similar conditions were found at the OMZ Station T3. A well-mixed layer of 15 m depth occurred at the surface, followed by a steep thermocline, where temperature dropped from 21 to 14°C at 50 m, after which the temperature declined gradually to approximately 4°C at 1,000 m. In contrast to Station 1, salinity values were highest at the surface (35.29) decreasing rapidly until ~ 50 m (34.90). A minimum salinity of 34.45 was reached at 800 m, then gradually increased toward a maximum of 34.55 at 1,000 m. Below a well-oxygenated layer of ~15 m (248 μM), a steep oxycline was observed between 16 and ~100 m with oxygen concentrations decreasing from 240 to 52 μM. Within the OMZ core oxygen levels were undetectable and nitrite concentrations were > 6 μM. Below ~440 m depth, oxygen gradually increased, reaching a maximum of 69 μM at 725 m.
Prokaryotic and Eukaryotic Abundances and Estimated Phagotrophy
Bacterial abundances in water samples used for incubations from the upper mesopelagic zone (150 m) at OMZ station T3 (150 m) and at OMZ Station L6 (station L6 experiments a and b (150 m)) were 2.8 ± 1.0 × 105 cells mL−1, 2.3 ± 0.9 × 105 cells mL−1 and 2.6 ± 0.9 × 105 cells mL−1, respectively. A decrease in bacterial cell counts was observed from T0 to Tf (final incubation time, which was very close to 24 h) in all incubations (Table 2).
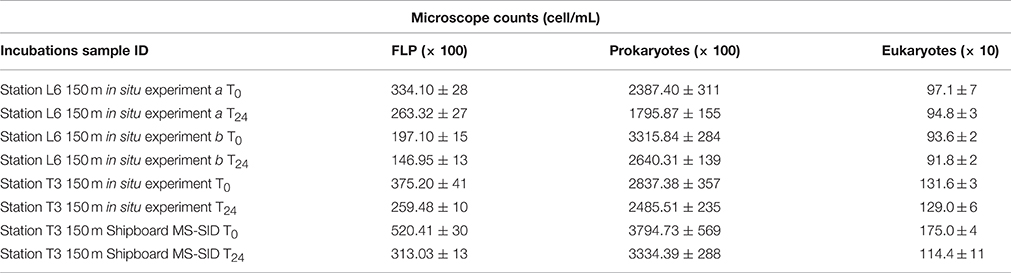
Table 2. Average microscope cell counts for Time 0 and Time final (Tf = 24 h) for in situ incubation studies of protist grazing in the ETSP OMZ using the MS-SID and for the shipboard study using Niskin bottle water from 150 m at Station T3 incubated in the MS-SID chamber.
Eukaryotic abundances (total ciliates and flagellates) were higher in samples collected at OMZ Station T3 (Table 2) than in any incubation at OMZ Station L6. Consistent with Cuevas and Morales (2006), who also studied oxygen depleted waters off Chile, heterotrophic nanoflagellates (2–20 μm) appeared to be the dominant protists. T0 counts ranged from 1,316 eukaryotic cells mL−1 recorded in the in situ incubation conducted at 150 m at OMZ Station T3 to 971 and 936 cells mL−1 recorded in the two consecutive replicate in situ incubation experiments a and b conducted at 150 m depth at OMZ Station L6. During the Station T3 Shipboard MS-SID incubation conducted using a water sample from 150 m depth collected using a Niskin bottle, a 35% decline in eukaryotic abundances was observed between T0 (1750 cells mL−1) and Tf (1144 cells mL−1). However, this decline was not observed over the course of the in situ incubation conducted at OMZ Station T3 at 150 m, where the eukaryotic abundances at T0 were 1,311 cells mL−1 and at Tf were 1,296 cells mL−1. This suggests the cumulative effects of retrieval of Niskin water to the surface and subsequent transfer to the incubation “bottle” (in this case, the MS-SID glass chamber, for consistency). As we could not replicate this comparative experiment during this cruise, these preliminary results must be interpreted with caution. Furthermore, while we believe microbial community composition at 150 m at this site is unlikely to be significantly different between the first 24-h in situ experiment and the start of the second 24-h shipboard experiment, the possible impacts of community shifts that may occur over this time period cannot be entirely ruled out. Nonetheless, these preliminary data warrant future confirmation, and we believe they are consistent with our view that minimizing sample manipulation through use of in situ approaches can only improve the accuracy of our estimates of protist phagotrophy in deep-ocean and/or low oxygen water columns.
Grazing estimates for the depths examined at both sites are presented in Table 3 and compared with results from previous studies of grazing conducted using water samples collected from below the euphotic zone in different regions of the world. We exclude from Table 3 the results for our 13-m depth in situ studies because photo-bleaching of FLP in the unshaded in situ experiment compromised the quality of resulting data. Future in situ incubation studies must protect fluorochromes from photobleaching during incubations conducted within the photic zone. For our ETSP studies, measured prokaryote turnover rates based on in situ studies were slightly higher in the 2 incubations conducted at OMZ Station L6 (28 and 20% d−1) than those measured during the in situ incubation conducted at OMZ Station T3 (13% d−1), (all studies conducted at 150 m).
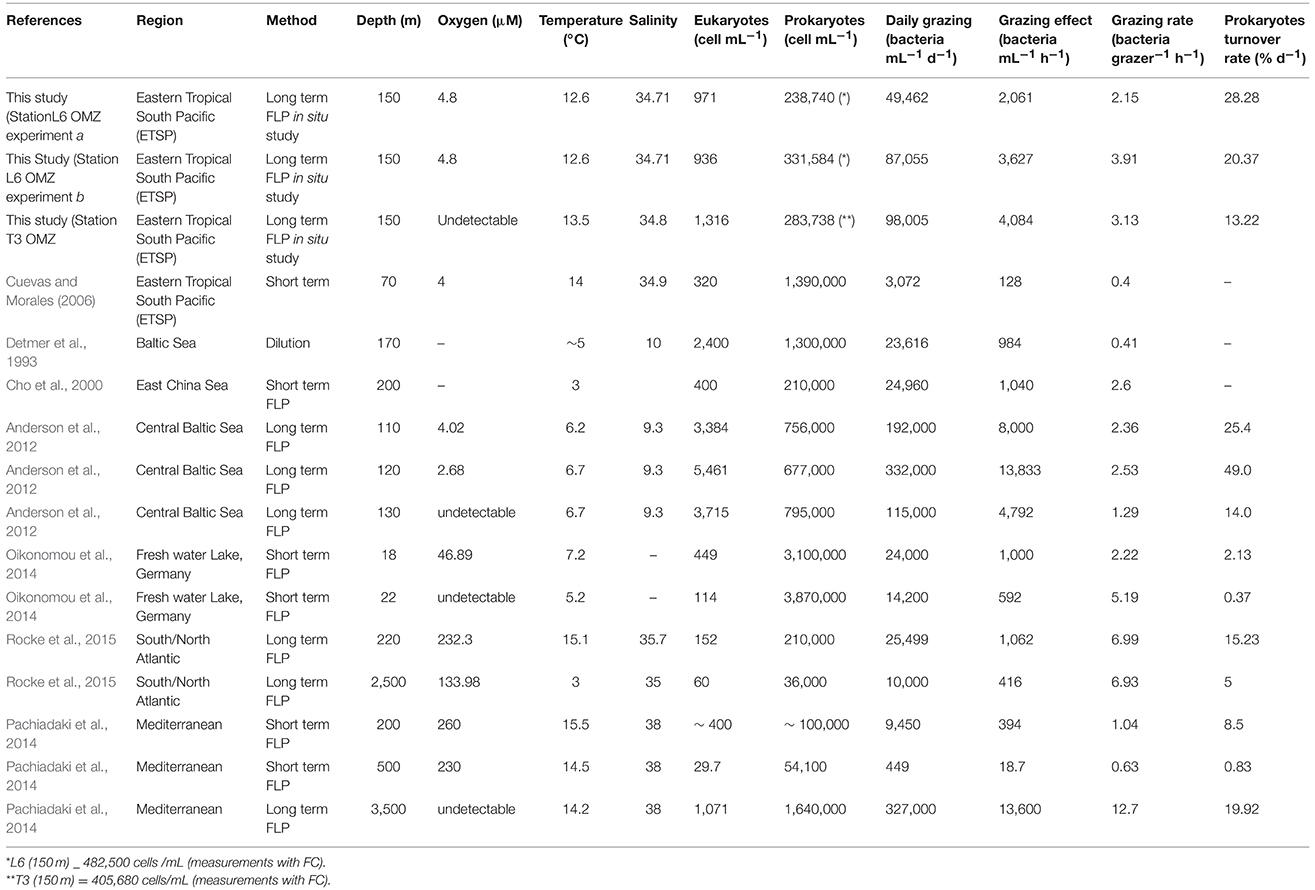
Table 3. Comparison of published data from studies of protist grazing conducted using waters collected from below the euphotic zone with our grazing study data from the ETSP OMZ.
Summary of Preliminary ETSP Experiments
In our experiments conducted at two stations in the ETSP, a decline in eukaryotic abundance (35% of the bulk population of eukaryotes) was observed in a shipboard incubation between T0 and Tf in water collected from 150 m depth using a Niskin bottle. A decline of this magnitude was not observed during an incubation conducted in situ at the same depth immediately prior to the shipboard incubation. The difference may be attributable to physiological changes in the predator population associated with recovery of water samples to the surface prior to initiation of experiments. This preliminary experiment suggests sample retrieval to the ocean surface and subsequent handling onboard the ship had an impact on the community (Table 2). Replication of these comparative studies in the future is warranted to assess reliability of these effects not only at these sites in the ETSP, but in other oceans and at other depths. Potential bottle effects during incubations typically include attachment of eukaryotes to container surfaces, negative responses to changing physicochemical conditions (e.g., temperature or oxygen) within the bottle, or preferential grazing and alteration of the food web (Marrasse et al., 1992; Oikonomou et al., 2014; Rocke et al., 2015). In situ incubations will not eliminate all potential sources of artifacts, but can minimize physico-chemical alterations associated with sample depressurization and handling prior to initiation of experiments.
Estimates of grazing from our ETSP experiments are within the range found in studies of several other oxygen-depleted waters around the world (Table 3), showing that up to 28.28% of the bulk population of bacteria could be consumed by protists at 150 m where oxygen concentrations were down to ~4.8 μM (Station L6) and up to 13.22% at 150 m where oxygen was undetectable (OMZ Station T3). Abundances of prokaryotes and eukaryotes measured in our ETSP samples are comparable to values reported by Lin et al. (2007) in the Cariaco Basin, Anderson et al. (2012) in the central Baltic Sea, Pachiadaki et al. (2014) in the Mediterranean Sea, and Rocke et al. (2015) in the western Atlantic. Cuevas and Morales (2006) also reported similar values of prokaryotic (0.6 ± 0.04 × 106 cells mL−1) and eukaryotic (0.10 ± 3.84 × 103 cells mL−−1) abundances for the north coast of Chile over the OMZ at 100 m. We observed a decline in concentration of both prokaryotes and protist communities with depth. In the absence of seasonal or episodic phenomena (e.g., turnover events, storms), results from different studies support a general decline in abundances of bacterial and protistan microbiota with depth in normoxic water columns. However, protist abundances can shift drastically within a short vertical distance within redox gradients found within stratified water columns (e.g., Anderson et al. (2012). As a result, we cannot rule out the possibility that higher resolution grazing studies (conducted at a greater number of depths in the ETSP OMZ) may have detected higher protist numbers at another depth along the redoxcline.
Specific Challenges Associated with Studies of Protist Phagotrophy in Anoxic and Deep Waters
Much remains to be learned about microbial activities and their role in biogeochemical cycling in the mesopelagic ocean and below, as well as in seasonally- or permanently-stratified water columns below the photic zone. In particular, the impact of protist grazing in these realms on carbon turnover and on prey communities integral to particular biogeochemical cycles is still poorly understood. Most studies of protist grazing that have been conducted using waters collected from below the photic zone suggest that protist grazing can have a significant impact on carbon turnover and on particular prey populations.
Collecting water samples and returning them to the surface using Niskin bottles, is a practice that can affect survival of protists from the deep sea or from deep low oxygen/anoxic waters, and can significantly alter the activities of both predator and prey populations retained in those samples (Edgcomb et al., 2011, 2016). When conducting studies of protist phagotrophy on deck of a ship the experimental outcome can be affected by artifacts associated with sample handling (e.g., changes in pressure, temperature, oxygen) that may compromise microbial functioning, and in some cases cause cell lysis (Edgcomb et al., 2011; Anderson et al., 2012; Pachiadaki et al., 2014). Longer intervals between sample collection and initiation of shipboard experiments and increased pressure changes for samples coming from greater depths can potentially exacerbate experimental artifacts. Evidence to date suggests that for more accurate assessments of protist phagotrophy the use of methodologies that allow in situ sample collection and experimentation prior to retrieval to the ocean surface should be considered, particularly for studies of waters from below the epipelagic.
Regardless of experimental design and whether in situ approaches are utilized or not, if ingestion of prey or disappearance of prey is used to gauge protist phagotrophy, the choice of prey should be considered carefully. Tracer techniques that follow fluorescently-labeled prey (FLP) surrogates into protist food vacuoles and either measure the ingestion of FLP by examining food vacuoles or by calculating the removal of FLP from the aqueous phase (or both) are considered to be relatively robust for providing specific ingestion rates for different protists morphotypes in marine samples (Jürgens and Massana, 2008). Potential artifacts associated with this approach include egestion of vacuole contents during the addition of fixatives following incubations (Pace and Baliff, 1987), prey selectivity (e.g., Landry et al., 1991) and times often required for obtaining reliable counts of ingested FLPs, particularly at low grazer densities, which may exacerbate known bottle effects (grazing of smaller protist by larger ones during the experiment, attachment of prey or predators to bottle walls, deleterious effects of altered chemistry due to confinement in bottles) (McManus and Okubo, 1991). For oligotrophic systems with low grazer concentrations one can follow the disappearance of added FLPs in longer-term (12–48 h) incubations (Marrasse et al., 1992). These long-term experiments are less labor-intensive, but can only provide an estimate of phagotrophy for the whole protistan community (Jürgens and Massana, 2008). Results of short- and long-term incubation experiments for deep-sea (3,000 m) samples were found to be consistent (Pachiadaki et al., 2014). Additionally, autofluorescence of phototrophic prey can interfere with fluorescence detection of labeled prey (ingested or not) or with attempts to apply cell sorting of protists based on ingestion of FLP. The application of protist inhibitors to gauge protist grazing impacts by monitoring bacterioplankton responses requires verification that the inhibitor does not have undesired effects on the natural bacterioplankton community. It stands to reason that if one can prepare labeled prey using indigenous bacterioplankton from the depths targeted for grazing studies, this will minimize potential artifacts due to prey selectivity that may occur. In the future, advances in cell sorting sensitivities as well as increasing availability of instruments allowing sample collection and replicated experimentation in situ are likely to enable better estimates of protist grazing impacts on deep sea bacterioplankton communities and on communities in oxygen-depleted water columns.
Author Contributions
LM, CT, VE, MP, and OU contributed to experimental design. CT, LM, and CH conducted all shipboard experiments. LM took the lead on data analysis and manuscript preparation with contributions to both from MP, CT, VE, and OU. OU was Chief Scientist on the cruise.
Conflict of Interest Statement
The authors declare that the research was conducted in the absence of any commercial or financial relationships that could be construed as a potential conflict of interest.
Acknowledgments
We would like to thank the captain and crew of the R/V Cabo de Hornos for their hard work that helped to make this work possible, John N. Kemp and team, Woods Hole Oceanographic Institution, for the design and fabrication of the surface float system used in this study, and Nadín Ramírez and Eduardo Navarro, Millennium Institute of Oceanography, for help with the MS-SID deployment and recovery at sea. This work was funded by the Agouron Institute, grant AI-M010.16.1 WHO to OU, M. Sullivan, and VE, and the Millenium Science Initiative, grant IC 120019. Ship time was provided the Chilean National Commission for Scientific and Technological Research (CONICYT) grant AUB 150006/12806.
References
Anderson, R., Winter, C., and Jürgens, K. (2012). Protist grazing and viral lysis as prokaryotic mortality factors at Baltic Sea oxica-noxic interfaces. Mar. Ecol. Prog. Ser. 467, 1–14. doi: 10.3354/meps10001
Arístegui, J., Duarte, C. M., Gasol, J. M., and Alonso-Saez, L. (2005). Active mesopelagic prokaryotes support high respiration oin the subtropical northeast Atlantic Ocean. Geophys. Res. Lett. 32, 1–4. doi: 10.1029/2004GL021863
Azam, F., Fenchel, T., Field, J., Gray, J., Meyer-Reil, L., and Thingstad, F. (1983). The ecological role of water-column microbes in the sea. Mar. Ecol. Prog. Ser. 10, 257–263. doi: 10.3354/meps010257
Bombar, D., Taylor, C. D., Wilson, S. T., Robidart, J. C., Rabines, A., Turk-Kubo, K. A., et al. (2015). Measurements of nitrogen fixation in the oligotrophic North Pacific subtropical gyre using a free-drifting submersible incubation device. J. Plankton Res. 37, 727–739. doi: 10.1093/plankt/fbv049
Brenner, R. (2002). “Chemical composition and reactivity,” in Biogeochemistry of Marine Dissolved Organic Matter, eds D. Hansell and C. Carlson (London: Elsevier Science), 59–85.
Cho, B. C., Na, S. C., and Choi, D. H. (2000). Active ingestion of fluorescently labeled bacteria by mesopelagic heterotrophic nanoflagellates in the East Sea, Korea. Mar. Ecol. Prog. Ser. 206, 23–32. doi: 10.3354/meps206023
Chow, C.-E., Kim, D. Y., Sachdeva, R., Caron, D. A., and Fuhrman, J. A. (2014). Top-down controls on bacterial community structure: microbial network analysis of bacteria, T4-like viruses and protists. ISME J. 8, 816–829. doi: 10.1038/ismej.2013.199
Cuevas, L. A., and Morales, C. E. (2006). Nanoheterotroph grazing on bacteria and cyanobacteria in oxic and suboxic waters in coastal upwelling areas of northern Chile. J. Plankton Res. 28, 385–397. doi: 10.1093/plankt/fbi124
De Brabandere, L., Thamdrup, B., Revsbech, N. P., and Foadi, R. (2012). A critical assessment of the occurrence and extend of oxygen contamination during anaerobic incubations utilizing commercially available vials. J. Microbiol. Methods 88, 147–154. doi: 10.1016/j.mimet.2011.11.001
del Giorgio, P. A., and Duarte, C. M. (2002). Respiration in the open ocean. Nature 420, 379–384. doi: 10.1038/nature01165
Detmer, A., Giesenhagen, H., Trenkel, V., Aui dem Venne, H., and Jochem, F. (1993). Phototrophic and heterotrophic pico- and nanoplankton in anoxic depths of the central Baltic Sea. Mar. Ecol. Prog. Ser. 99, 197–203. doi: 10.3354/meps099197
Edgcomb, V. P., Taylor, C., Pachiadaki, M. G., Honjo, S., Engstrom, I., and Yakimov, M. (2016). Comparison of Niskin vs. in situ approaches for analysis of gene expression in deep Mediterranean Sea water samples. Deep. Res. Part II 129, 213–222. doi: 10.1016/j.dsr2.2014.10.020
Edgcomb, V., Orsi, W., Taylor, G. T., Vdacny, P., Taylor, C., Suarez, P., et al. (2011). Accessing marine protists from the anoxic Cariaco basin. ISME J. 5, 1237–1241. doi: 10.1038/ismej.2011.10
Escribano, R., Hidalgo, P., and Krautz, M. C. (2009). Zooplankton associated with the oxygen minimum zone system in the northern upwelling region of Chile during March 2000. Deep Sea Res. Part II Top. Stud. Oceanogr. 56, 1083–1094. doi: 10.1016/j.dsr2.2008.09.009
Fowler, S. W., and Knauer, G. A. (1986). Role of large particles in the transport of elements and organic compounds through the oceanic water column. Prog. Oceanogr. 16, 147–194. doi: 10.1016/0079-6611(86)90032-7
Fu, Y., O'Kelly, C., Sieracki, M., Daniel, L., and Distel, D. L. (2003). Protistan grazing analysis by flow cytometry using prey labeled by in vivo expression of fluorescent proteins protistan grazing analysis by flow cytometry using prey labeled by in vivo expression of fluorescent proteins. Appl. Environ. Microbiol. 69, 6848–6855. doi: 10.1128/AEM.69.11.6848-6855.2003
Fuhrman, J. A. (1999). Marine viruses and their biogeochemical and ecological effects. Nature 399, 541–548. doi: 10.1038/21119
Hansell, D. A., and Carlson, C. A. (1998). Deep-ocean gradients in the concentration of dissolved organic carbon. Nature 395, 263–266. doi: 10.1038/26200
Hollibaugh, J. T., Fuhrman, J. A., and Azam, F. (1980). Radioactively labeling of natural assemblages of bacterioplankton for use in trophic studies. Limnol. Oceanogr. 25, 172–181. doi: 10.4319/lo.1980.25.1.0172
Jürgens, K., and Massana, R. (2008). “Protistan grazing on marine bacterioplankton,” in Microbial Ecology of the Oceans, 2nd Edn, ed D. L. Kirchman (New York, NY: Wiley-Liss), 383–441. doi: 10.1002/9780470281840.ch11
Lam, P., Lavik, G., Jensen, M. M., van de Vossenberg, J., Schmid, M., Woebken, D., et al. (2009). Rivising the nitrogen cycle in the Peruvian oxygen minimum zone. Proc. Natl. Acad. Sci. U.S.A. 106, 4752–4757. doi: 10.1073/pnas.0812444106
Landry, M. R., Kirshtein, J., and Constantinou, J. (1995). A refined dilution technique for measuring the community grazing impact of microzooplankton, with experimental tests in the central equatorial Pacific. Mar. Ecol. Prog. Ser. 120, 53–64. doi: 10.3354/meps120053
Landry, M. R., Lehner-Fournier, J. M., Sundstrom, J. A., Fagerness, V. L., and Selph, K. E. (1991). Discrimination between living and heat-killed prey by a marine zooflagellate, Paraphysomonas vestita (Stokes). J. Exp. Mar. Biol. Ecol. 146, 139–151. doi: 10.1016/0022-0981(91)90021-N
Lin, X., Scranton, M. I., Varela, R., Chistoserdov, A., and Taylor, G. T. (2007). Compositional responses of bacterial communities to redox gradients and grazing in the anoxic Cariaco basin. Aquat. Microb. Ecol. 47, 57–72. doi: 10.3354/ame047057
Marrasse, C., Lim, E. L., and Caron, D. A. (1992). Seasonal and daily changes in bacterivory in a coastal plankton community. Mar. Ecol. Prog. Ser. 82, 281–289. doi: 10.3354/meps082281
McManus, G. B., and Okubo, A. (1991). On the use of surrogate food particles measure protistan ingestion. Limnol. Oceanogr. 36, 613–617. doi: 10.4319/lo.1991.36.3.0613
Oikonomou, A., Pachiadaki, M., and Stoeck, T. (2014). Protistan grazing in a meromictic freshwater lake with anoxic bottom water. FEMS Microbiol. Ecol. 87, 691–703. doi: 10.1111/1574-6941.12257
Orsi, W., Edgcomb, V., Jeon, S., Leslin, C., Bunge, J., Taylor, G., et al. (2011). Protistan microbial observatory in the Cariaco basin, Caribbean. II. Habitat specialization. ISME J. 5, 1344–1356. doi: 10.1038/ismej.2011.7
Orsi, W., Song, Y. C., Hallam, S., and Edgcomb, V. (2012). Effect of oxygen minimum zone formation on communities of marine protists. ISME J. 6, 1586–1601. doi: 10.1038/ismej.2012.7
Pace, M. L., and Baliff, M. D. (1987). Evaluation of a fluorescent microsphere technique for measuring grazing rates of phagotrophic microorganisms. Mar. Ecol. Prog. Ser. 40, 185–193. doi: 10.3354/meps040185
Pachiadaki, M. G., Taylor, C., Oikonomou, A., Yakimov, M. M., Stoeck, T., and Edgcomb, V. (2014). In situ grazing experiments apply new technology to gain insights into deep-sea microbial food webs. Deep. Res. Part II Top. Stud. Oceanogr. 129, 223–231. doi: 10.1016/j.dsr2.2014.10.019
Parris, D. J., Ganesh, S., Edgcomb, V. P., DeLong, E. F., and Stewart, F. J. (2014). Microbial eukaryote diversity in the marine oxygen minimum zone off northern Chile. Front. Microbiol. 5:543. doi: 10.3389/fmicb.2014.00543
Paulmier, A., and Ruiz-Pino, D. (2009). Oxygen minimum zones (OMZs) in the modern ocean. Prog. Oceanogr. 80, 113–128. doi: 10.1016/j.pocean.2008.08.001
Pedrós Alió, C., Calderon-Paz, J. I., and Gason, J. M. (2000). Comparative analysis shows that bacterivory, not viral lysis, controls the abundance of heterotrophic prokaryotic plankton. FEMS Microbiol. Ecol. 32, 157–165. doi: 10.1016/S0168-6496(00)00026-X
Pernthaler, J., Glöckner, F. O., Schönhuber, W., and Amann, R. (2001). Fluorescence in situ hybridization with rRNA-targeted oligonucleotide probes. Methods Microbiol. 30, 207–226. doi: 10.1016/S0580-9517(01)30046-6
Porter, K. G., and Feig, Y. S. (1980). The use of dapi for identifying and counting aquatic microflora. Limnol. Oceanogr. 25, 943–948. doi: 10.4319/lo.1980.25.5.0943
Revsbech, N. P., Larsen, L. H., Gundersen, J., Dalsgaard, T., Ulloa, O., and Thamdrup, B. (2009). Determination of ultra-low oxygen concentrations in oxygen minimum zones by the STOX sensor. Limnol. Oceanogr. Methods 7, 371–381. doi: 10.4319/lom.2009.7.371
Rocke, E., Pachiadaki, M. G., Cobban, A., Kujawinski, E. B., and Edgcomb, V. P. (2015). Protist community grazing on prokaryotic prey in deep ocean water masses. PLoS ONE 10:e0124505. doi: 10.1371/journal.pone.0124505
Salat, J., and Marrase, C. (1994). Exponential and linear estimations of grazing on bacteria: effects of changes in the proportion of marked cells. Mar. Ecol. Prog. Ser. 104, 205–209. doi: 10.3354/meps104205
Sherr, B. F., Sherr, E. B., Andrew, T. L., Fallon, R. D., and Newell, S. Y. (1986). Trophic interactions between heterotrophic Protozoa and bacterioplankton in estuarine water analyzed with selective metabolic inhibitors. Mar. Ecol. Prog. Ser. 32, 169–180. doi: 10.3354/meps032169
Stevens, H., and Ulloa, O. (2008). Bacterial diversity in the oxygen minimum zone of the eastern tropical South Pacific. Environ. Microbiol. 10, 1244–1259. doi: 10.1111/j.1462-2920.2007.01539.x
Stewart, F. J., Ulloa, O., and Delong, E. F. (2012). Microbial metatranscriptomics in a permanent marine oxygen minimum zone. Environ. Microbiol. 14, 23–40. doi: 10.1111/j.1462-2920.2010.02400.x
Stramma, L., Johnson, G. C., Sprintall, J., and Mohrholz, V. (2008). Expanding oxygen-minimum zones in the tropical oceans. Science 320, 655–658. doi: 10.1126/science.1153847
Taylor, C. D., Edgcomb, V. P., Doherty, K. W., Engstrom, I., Shanahan, T., Pachiadaki, M. G., et al. (2015). Fixation filter, device for the rapid in situ preservation of particulate samples. Deep. Res. Part I Oceanogr. Res. Pap. 96, 69–79. doi: 10.1016/j.dsr.2014.09.006
Taylor, G. T. (1982). The role of pelagic heterotrophic protozoa in nutrient cycling: a review. Ann. Inst. Oceanogr. 58(Suppl.), 227–241.
Thamdrup, B., Dalsgaard, T., and Peter, N. (2012). Deep-sea research I Widespread functional anoxia in the oxygen minimum zone of the Eastern South Pacific. Deep. Res. Part I 65, 36–45. doi: 10.1016/j.dsr.2012.03.001
Thamdrup, B., Dalsgaard, T., Jensen, M. M., Ulloa, O., Farias, L., and Escribano, R. (2006). Anaerobic ammonium oxidation in the oxygen-deficient waters off northern Chile. Limnol. Oceanogr. 51, 2145–2156. doi: 10.4319/lo.2006.51.5.2145
Ulloa, O., and Pantoja, S. (2009). The oxygen minimum zone of the eastern South Pacific. Deep Sea Res. Part II Top. Stud. Oceanogr. 56, 987–991. doi: 10.1016/j.dsr2.2008.12.004
Ulloa, O., Canfield, D. E., DeLong, E. F., Letelier, R. M., and Stewart, F. J. (2012). Microbial oceanography of anoxic oxygen minimum zones. Proc. Natl. Acad. Sci. U.S.A. 109, 15996–16003. doi: 10.1073/pnas.1205009109
Vaque, D., Gasol, J. M., and Marrase, C. (1994). Grazing rates on bacteria - The significance of methodology and ecological factors. Mar. Ecol. Prog. Ser. 109, 263–274. doi: 10.3354/meps109263
Vazquez-Dominguez, E., Peters, F., Gasol, J. M., and Vaqué, D. (1999). Measuring the grazing losses of picoplankton: methodological improvements in the use of fluorescently labeled tracers combined with flow cytometry. Aquat. Microb. Ecol. 20, 119–128. doi: 10.3354/ame020119
Wishner, K. F., Gowing, M. M., and Gelfman, C. (1998). Mesozooplankton biomass in the upper 1000 m in the Arabian Sea: overall seasonal and geographic patterns, and relationship to oxygen gradients. Deep. Res. Part II Top. Stud. Oceanogr. 45, 2405–2432. doi: 10.1016/S0967-0645(98)00078-2
Wishner, K. F., Outram, D. M., Seibel, B. A., Daly, K. L., and Williams, R. L. (2013). Zooplankton in the eastern tropical north Pacific: boundary effects of oxygen minimum zone expansion. Deep Sea Res. Part I Oceanogr. Res. Pap. 79, 122–140. doi: 10.1016/j.dsr.2013.05.012
Wright, J. J., Konwar, K. M., and Hallam, S. J. (2012). Microbial ecology of expanding oxygen minimum zones. Nat. Rev. Microbiol. 10, 381–394. doi: 10.1038/nrmicro2778
Keywords: OMZ, phagotrophy, in situ technology, incubation studies, ETSP, Eastern Tropical South Pacific OMZ
Citation: Medina LE, Taylor CD, Pachiadaki MG, Henríquez-Castillo C, Ulloa O and Edgcomb VP (2017) A Review of Protist Grazing Below the Photic Zone Emphasizing Studies of Oxygen-Depleted Water Columns and Recent Applications of In situ Approaches. Front. Mar. Sci. 4:105. doi: 10.3389/fmars.2017.00105
Received: 03 November 2016; Accepted: 29 March 2017;
Published: 26 April 2017.
Edited by:
Hongbin Liu, Hong Kong University of Science and Technology, Hong KongReviewed by:
Il-Nam Kim, Incheon National University, South KoreaRuth Anderson, University of Copenhagen, Denmark
Copyright © 2017 Medina, Taylor, Pachiadaki, Henríquez-Castillo, Ulloa and Edgcomb. This is an open-access article distributed under the terms of the Creative Commons Attribution License (CC BY). The use, distribution or reproduction in other forums is permitted, provided the original author(s) or licensor are credited and that the original publication in this journal is cited, in accordance with accepted academic practice. No use, distribution or reproduction is permitted which does not comply with these terms.
*Correspondence: Virginia P. Edgcomb, dmVkZ2NvbWJAd2hvaS5lZHU=
†Present Address: Maria G. Pachiadaki, Bigelow Laboratories, East Boothbay, ME, USA