- 1Department of Earth, Ocean, and Atmospheric Sciences, Florida State University, Tallahassee, FL, USA
- 2Arctic Research Centre, Aarhus University, Aarhus, Denmark
- 3Consiglio Nazionale delle Ricerche, Istituto di Scienze Marine, La Spezia, Italy
- 4Centro Oceanográfico de Baleares, Instituto Español de Oceanografía, Palma de Mallorca, Spain
- 5Department of Computer Science, Jerusalem College of Technology, Jerusalem, Israel
- 6National Institute of Oceanography and Experimental Geophysics, Trieste, Italy
- 7Italian National Institute for Environmental Protection and Research, Chioggia, Italy
- 8DiSVA, Università Politecnica delle Marche, Ancona, Italy
- 9National Institute of Aquatic Resources, Technical University of Denmark Aqua, Hirtshals, Denmark
Visual ship transect surveys provide crucial information about the density, and spatial distribution of floating anthropogenic litter in a basin. However, such observations provide a ‘snapshot’ of local conditions at a given time and cannot be used to deduce the provenance of the litter or to predict its fate, crucial information for management and mitigation policies. Particle tracking techniques have seen extensive use in these roles, however, most previous studies have used simplistic initial conditions based on bulk average inputs of debris to the system. Here, observations of floating anthropogenic macro debris in the Adriatic Sea are used to define initial conditions (number of particles, location, and time) in a Lagrangian particle tracking model. Particles are advected backward and forward in time for 60 days (120 days total) using surface velocities from an operational regional ocean model. Sources and sinks for debris observed in the central and southern Adriatic in May 2013 and March 2015 included the Italian coastline from Pescara to Brindisi, the Croatian island of Mljet, and the coastline from Dubrovnik through Montenegro to Albania. Debris observed in the northern Adriatic originated from the Istrian peninsula to the Italian city of Termoli, as well as the Croatian island of Cres and the Kornati archipelago. Particles spent a total of roughly 47 days afloat. Coastal currents, notably the eastern and western Adriatic currents, resulted in large alongshore displacements. Our results indicate that anthropogenic macro debris originates largely from coastal sources near population centers and is advected by the cyclonic surface circulation until it strands on the southwest (Italian) coast, exits the Adriatic, or recirculates in the southern gyre.
1. Introduction
Advancing the study and management of anthropogenic marine debris (AMD) requires accurate identification of source regions (Critchell and Lambrechts, 2016) and quantification of temporal variability of injection rates. Identifying sources and sinks of AMD can assist resource managers maximize the effectiveness of prevention and response efforts by providing scientific support to the implementation of public policies. While progress has been made, most relevant studies either relied on observations of beached AMD (Yoon et al., 2010; Kako et al., 2011, 2014; Neumann et al., 2014), made assumptions about the amount of AMD as well as its temporal and/or spatial distributions (Lebreton et al., 2012; Critchell et al., 2015; Mansui et al., 2015; Liubartseva et al., 2016), or used coarse resolution velocity data and/or idealized surface currents (Aliani and Molcard, 2003; Maximenko et al., 2012; van Sebille et al., 2012; Reisser et al., 2013; Isobe et al., 2014). Such studies have identified the physical processes relevant to the transport and accumulation of debris, however, the efficacy of models in aiding management efforts depends strongly on the assumptions applied in the particle tracking scheme (Critchell and Lambrechts, 2016) as well as the resolution and accuracy of the underlying velocity field (Putman and He, 2013). Beaching, for example, is a complex process that is not properly represented in most particle tracking models (Lebreton et al., 2012) as ocean model domains typically extend to some minimum depth and, therefore, do not resolve the shoreline. Furthermore, even relatively high-resolution regional models, like the one employed here, cannot resolve complex shoreline topography. Additionally, a resource manager coordinating cleanup efforts is not faced with the mean state, which is most commonly reported by modeling studies, but instead must respond to debris loads that can vary in space and time (Kako et al., 2010, 2011) in response to numerous environmental (Critchell and Lambrechts, 2016) and human factors (Slavin et al., 2012; Munari et al., 2016).
Here, observed position, time, and abundance of floating macro AMD are used to specify initial conditions in a Lagrangian particle tracking model, thereby removing assumptions about debris injection rates and locations. Backward-in-time and forward-in-time particle trajectory computations identify potential coastal sources and sinks, respectively, for the observed AMD. Furthermore, only AMD observations within the model domain and at least 4 km from a boundary were used to initialize the particle tracking model. The particle tracking model brings the “snapshot” AMD surveys to life while, at the same time, realistic initial conditions based on the AMD surveys can arguably lead to identification of debris “hot spots” (Galgani, 2015). In the remainder of the manuscript we describe the methodology, apply it to the Adriatic Sea, discuss strengths and weaknesses of the proposed methodology, and suggest improvements to both our method, and AMD modeling in general.
2. Methods
2.1. Study Area
Twenty five years of surface drifter studies have effectively characterized the basin-scale and mesoscale surface currents of the Adriatic Sea, as well as their variability (Poulain, 1999, 2001; Ursella et al., 2006; Carlson et al., 2016). The surface Lagrangian pathways relevant to the transport of AMD at synoptic to seasonal time scales are the cyclonic coastal currents and the northern, central, and southern cyclonic sub-gyres (Poulain, 2001). The east Adriatic current (EAC) flows northwestward along the eastern shore and the west Adriatic current (WAC) flows southeastward along the western (Italian) shore (Poulain, 2001). At the timescales considered here, the surface currents vary in response to river runoff, heat fluxes, and exchange through the Strait of Otranto (Artegiani et al., 1997). In general, westward surface transport dominates the surface circulation and the WAC acts as an effective transport pathway to export drifters, and presumably surface waters and AMD, out of the basin (Carlson et al., 2016). Relatively short drifter (Poulain, 2001) and particle (Liubartseva et al., 2016) half-lives of 40–45 days suggest little accumulation of floating AMD in the Adriatic basin at longer timescales. For a detailed review of surface Lagrangian transport in the Adriatic see Carlson et al. (2016) and references therein.
As elsewhere in the world, anthropogenic waste has been found in all compartments of the Adriatic marine environment. Plastic litter has been reported from northern Adriatic beaches (Laglbauer et al., 2014; Munari et al., 2016), coastal sediments (Vianello et al., 2013; Blašković et al., 2017), surface waters (Suaria and Aliani, 2014; Gajšt et al., 2016; Suaria et al., 2016) and in very large amounts on the seafloor (Galgani et al., 2000; Strafella et al., 2015; Pasquini et al., 2016), where litter densities are among the highest of the entire Mediterranean basin. Ingestion of plastic by Adriatic fauna has been reported for marine turtles (Lazar and Gračan, 2011; Poppi et al., 2012), sperm whales (Mazzariol et al., 2011) and dolphins (Pribanic et al., 1999) as well as from commercial fish (Avio et al., 2015) and crustacean species (Wieczorek et al., 1999).
Liubartseva et al. (2016) modeled the transport of AMD using a high-resolution ocean model and a Markov chain to simulate passive particle trajectories, based on transition probability computed from ensemble model trajectories. Liubartseva et al. (2016) assumed a constant annual input of AMD, which was split into terrestrial and marine sources and terrestrial inputs were further split according to population. The methodology presented here differs from Liubartseva et al. (2016) in that we use observed debris as input to the particle tracking model. Furthermore, Liubartseva et al. (2016) used a statistical approach while we consider specific events that takes variability into account. Finally Liubartseva et al. (2016) parameterized sub-grid-scale turbulence and windage, which are not considered in our methodology (see Section 2.4).
2.2. Debris Surveys
Time, date, position, abundance, and typology of floating macro debris were recorded during three visual ship surveys performed in the Adriatic Sea in May 2013 and in March and November-December 2015 (Figure 1A). The May 2013 observations are summarized in Suaria and Aliani (2014) and the March 2015 observations were made by the same observer following the same monitoring protocol.
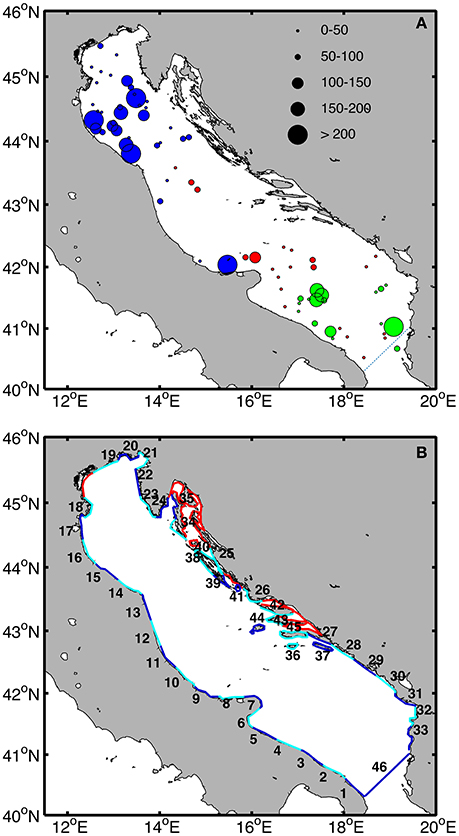
Figure 1. (A) Abundances of anthropogenic marine debris (items km−2) observed in the Adriatic Sea during ship-based visual surveys in May 2013 (red), March 2015 (green), and November–December 2015 (blue). (B) Coastline segments used to identify potential sources and sinks of debris are numbered for reference in Figures 4–10 and are highlighted in alternating dark and light blue. Red segments lack numbers as they were not identified as a source or a sink of debris.
The November-December 2015 observations were made during the SoleMon trawl-survey (Grati et al., 2013) following a 10 m fixed-width monitoring protocol as recommended by EU MSFD guidelines (Galgani et al., 2013). In all three surveys, all litter items larger than 2.5 cm were recorded along short transects of ~30 min (mean length 7.7 ± 2.6 km) during daytime navigation and under good visibility conditions (i.e., wind speed <20 kts). AMD abundances (expressed as items km−2) were then computed for all transects and plotted in Figure 1A. Overall results are summarized in Table 1.
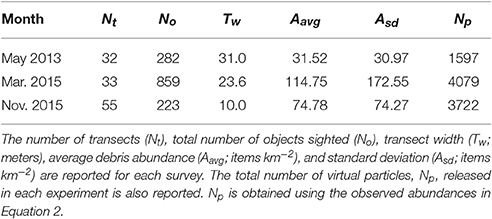
Table 1. Summary of visual transect surveys of floating macro anthropogenic debris in the Adriatic Sea.
2.3. Regional Ocean Model
Surface velocities from the AdriaROMS 4.0 ocean model (Russo et al., 2013a,b) are used to model the Lagrangian transport of the observed AMD. AdriaROMS 4.0 is the operational implementation of the Regional Ocean Modeling System (ROMS; Shchepetkin and McWilliams, 2005; Haidvogel et al., 2008) for the Adriatic Sea (see Russo et al., 2013b for details). The AdriaROMS model domain extends to the 5 m isobath, which varies in distance from the actual coastline due to differences in bottom slope across the Adriatic, and the open boundary in the southern Adriatic is shown in Figure 1. AdriaROMS has spatial and temporal resolutions of ° (approximately 2 km) and 1 h, respectively, with 22 terrain-following sigma levels. Fluxes are derived from the Consortium for Small-Scale Modeling (COSMO-I7) atmospheric model and river runoff includes daily Po River discharge and climatological discharges for other Adriatic rivers (Russo et al., 2009; Benetazzo et al., 2013).
Carlson et al. (2016) showed how synoptic wind events can alter surface Lagrangian pathways in the Adriatic and, as such, COSMO winds at 10 m height are use to qualitatively examine the role of winds in the transport of floating AMD, following Carlson et al. (2016). Basin-averaged wind is used as previous studies found high correlations in both magnitude and direction of surface winds over the Adriatic (Magaldi et al., 2010; Carlson et al., 2016).
2.4. Lagrangian Particle Tracking Model
Floating AMD are treated as buoyant, passive particles and are advected by modeled ocean currents using the Particle Tracking and Analysis TOolbox (PaTATO) for Matlab (Fredj et al., 2016). Particle positions are computed at hourly intervals by interpolating velocities to particle positions and integrating:
where v, x, and t correspond to the spatially and temporally varying velocity obtained from AdriaROMS, position, and time, respectively (Fredj et al., 2016). Velocities on the Arakawa C grid are interpolated to the center of each grid cell. The particle tracking scheme was validated by Carlson et al. (2016) by comparing virtual particle trajectories to observed surface drifter trajectories. Thus, the strengths and weaknesses of the model, in a Lagrangian sense, were known a priori in this case. The main weaknesses of the model are the drifter-particle separation rate and the difficulty in reproducing observed Lagrangian transport around the many small islands found in the eastern Adriatic Sea. Furthermore, considering that flows become non-linear close to shore, the dynamics of debris in these boundary areas are not included in this modeling. Particles are not re-floated or reflected at the boundaries and no attempts are made to model sub-grid-scale processes, windage, or degradation of AMD. Stagnation at a solid boundary signifies beaching (Lebreton et al., 2012; Mansui et al., 2015) and particles that encountered the open boundary are marked as having left the basin and are excluded from subsequent analyses. While these processes are undoubtedly important, the uncertainty associated with each parameter remains quite large (Critchell and Lambrechts, 2016). Furthermore, we did not use subgrid processes parametrization under 2 km because the parameters of subgrid processes are basically unknown at those scales and we would have added further uncertainty in the results (Griffa, 1996; Haza et al., 2012; Carlson et al., 2016).
Observed AMD positions, times and abundances are used to specify initial positions of virtual particles, which are tracked for 60 days forward and backward in time (120 days total). Transects within 4 km (2 grid points) of the AdriaROMS boundaries were discarded. Of the 120 transects conducted, 75 were located within the model domain and recorded non-zero AMD abundances. The number of particles, Np, to be released along each transect is defined as:
where D, L, and W correspond to the observed AMD abundance for a given transect (items km−2), transect length (km), and transect width, respectively. Here, we set W = 0.2 km, as the majority of debris sighted in Suaria and Aliani (2014) were within 100 m of the observer. In other words, while most of the AMD was observed along either a 10 m or 30 m strip width, the abundance estimates are assumed to be representative of a 100 m strip on either side of the vessel. Particles are distributed randomly in each polygon and released at the mid-point of the transect. A sensitivity test (not shown) using particles distributed uniformly along the transect line showed no differences. In total, 9,398 particles were released, with the number of particles per transect varying from 20 to 1693.
2.5. Analysis Methods
Potential near-shore sources and sinks are identified by dividing the Adriatic coastline into 50 km segments (Figure 1B). The model domain extends to the 5 m isobath, thereby preventing identification of actual terrestrial sources and sinks. Islands large enough to be resolved by AdriaROMS model are also included, resulting in 64 coastal segments. Small islands with short coastlines, like Tremiti and Palagruza, are not included in the analysis. The open boundary at the Otranto Strait is treated as a single segment, for a total of 65 segments. For clarity, only segments that served potential sources or sinks are shown. Percentages of particles beached, afloat, and that encounter the open boundary in backward and forward time are reported. The connection percentage, or percentage of particles reaching a coastline segment from a given transect, provides indications of coastal areas that could serve as sources and sinks.
The residence time is defined as the total duration of a particle in the Adriatic marine environment and is the sum of the time afloat in backwards and forwards time. Particles that remained afloat at the end of the ± 60 day integration period were not included in estimates of the residence time. Bootstrap estimates of the averages (Efron and Tibshirani, 1986) are used as the distributions of residence times are non-normal.
3. Results
3.1. Floating Debris Survey
While this paper focuses on using observed AMD abundances to initialize a particle tracking model, we briefly summarize the visual survey observations. Overall, 120 visual transect surveys were performed during the three cruises, covering a total length of 922.2 km. A total of 1,364 macro AMD objects were observed floating on the Adriatic. On average, the highest AMD abundances were observed in March 2015 in the southern gyre (Figure 1A, Table 1). In May 2013 litter abundances were significantly lower than in both March (p = 0.0016; Mann-Whitney U test with Bonferroni correction) and November–December 2015 (p = 0.008). On the other hand, no significant difference was found between the two surveys carried out in 2015 (p = 0.926). The available data cannot easily explain the differences in abundances observed in the three surveys as different regions of the Adriatic Sea were sampled in different seasons over two separate years.
3.2. Debris Tracking
3.2.1. May 2013: Central and Southern Adriatic Sea
Sixty two, twenty five, and thirteen percent of backward particle beached, remained afloat, or encountered the open boundary, respectively (Table 2). The average time from source to sighting location was 22.8 days. The forward trajectories suggest significant export, with 46% of particles encountering the open boundary. 41% of particles beached and 13% were still afloat after 60 days. The average time from sighting location to a coastal sink was approximately 39 days.
Example backward trajectories reveal spatial and temporal variability in surface transport pathways. AMD observed 9 May 2013 at approximately 42° N, 16° E (Figure 2A) were transported by the EAC, the central cyclonic sub-gyre, and the WAC while debris observed 9 days later and about 100 km to the east (42° N, 17° E) originated almost exclusively from the southern cyclonic sub-gyre (Figure 2C). AMD observed near the east coast originated from the nearby coastline and were transported by the EAC, with a small subset from the southern sub-gyre (Figure 2B). Backwards trajectories suggest that AMD observed 19 May at approximately 15° N, 43.5° E originated from the east and west coasts and were transported in the cyclonic central sub-gyre.
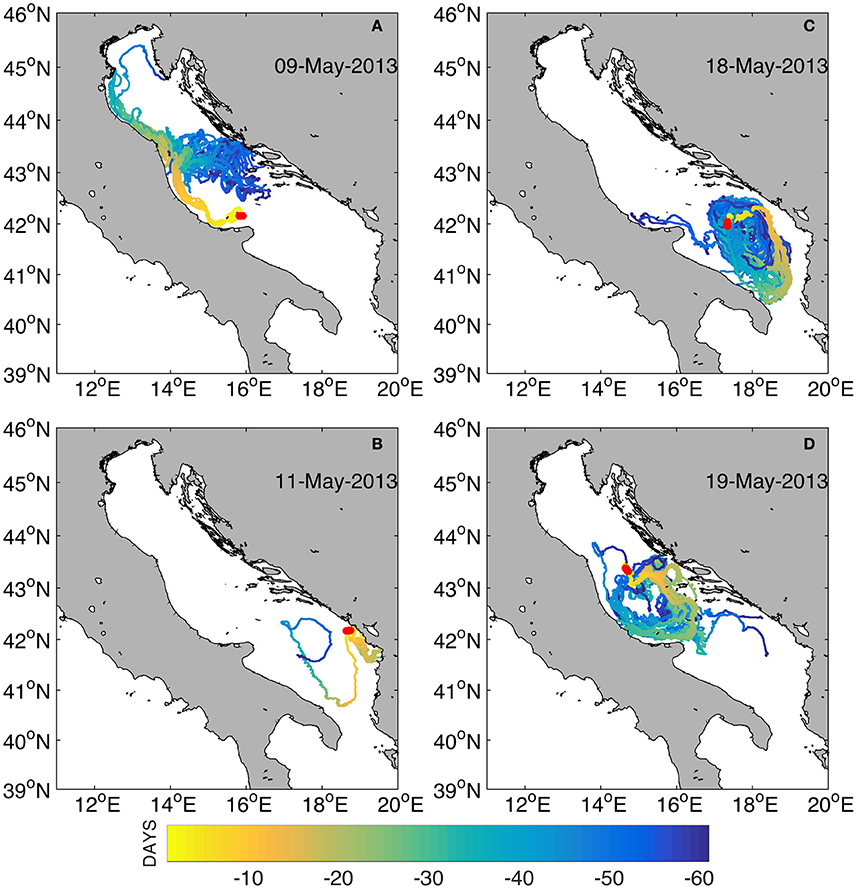
Figure 2. Selected backward particle trajectories based on observations of floating anthropogenic marine debris on 9 May 2013 (A), 11 May 2013 (B), 18 May 2013 (C), and 19 May 2013 (D). The release locations are indicated by red circles and the color scale corresponds to time in days since initial release.
Example forward trajectories show the influence of the same large-scale features, namely the EAC, WAC, and the southern gyre (Figures 3A–C). However, AMD observed 19 May at approximately 15° N, 43.5° E traveled north toward the Croatian coastline before looping west until they were entrained in the WAC and transported alongshore toward the Gargano Peninsula (Figure 3D).
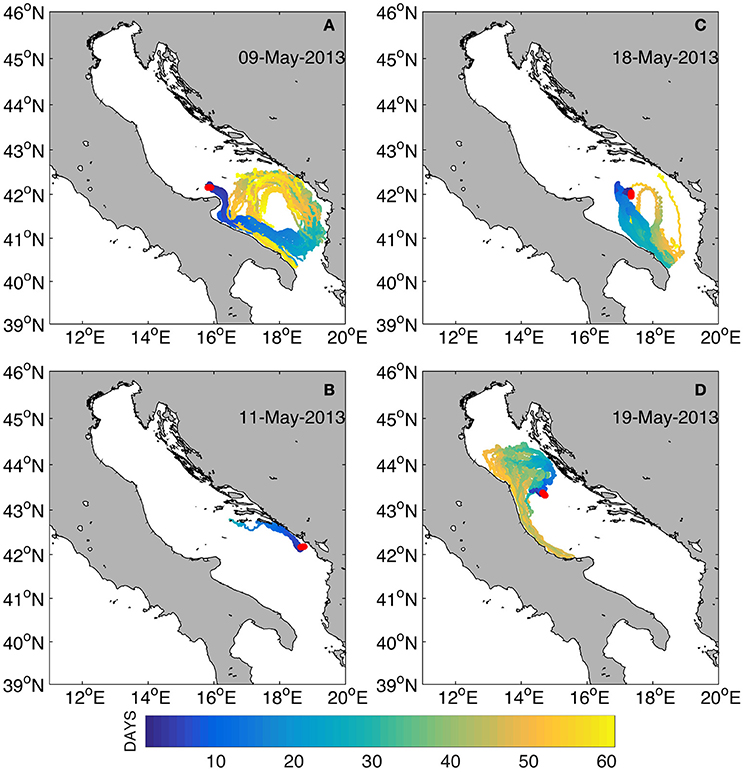
Figure 3. Selected forward particle trajectories based on observations of floating anthropogenic marine debris on 9 May 2013 (A), 11 May 2013 (B), 18 May 2013 (C), and 19 May 2013 (D). The release locations are indicated by red circles and the color scale corresponds to time in days since initial release.
The backwards and forwards connection percentages show that a continuous 400 km stretch of the central Italian coast (segments 7–14) acted as both sources and sinks (Figure 4). Segment 4, near Bari, provided more than 80% of the AMD sighted on transect 11. Much of the Croatian shoreline acted as both source and sink as well. Import and export of observed AMD across the open boundary is also likely, with large connection percentages observed along the open boundary segment for both backward and forward particle trajectories.
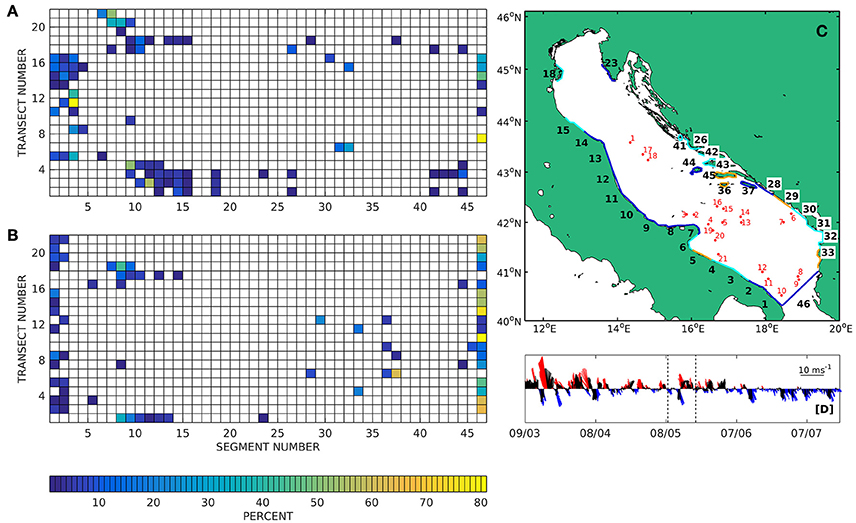
Figure 4. Percentages of particles from a given transect (rows) that reach a given coastal segment (columns) for backwards (A) and forwards (B) trajectories computed from the May 2013 debris observations. (C) Coastline segments that served as both sources and sinks are plotted in dark blue. Light blue segments acted as sources only and orange segments acted as sinks. Transect locations and numbers are shown in red. (D) Stick plot of winds during the particle tracking experiment. Red (blue) indicates Sirocco (Mistral) winds and the vertical dashed lines denote the time period of the AMD observations.
Winds during the backwards trajectories (9 March–9 May) were highly variable with several strong, sustained Sirocco events interspersed with weaker Mistral winds (Figure 4D). Winds remained variable, with a weaker Sirocco event, during visual debris sightings before transitioning to weaker, but more persistent Mistral winds from June to August 2013 (Figure 4D). Particles afloat after −60 days were distributed between the central and southern cyclonic sub-gyres while the 13% of particles afloat after +60 days were concentrated largely in the southern gyre (Figures 11A,B).
3.2.2. March 2015: Southern Adriatic Sea
Forty one, seventeen, and forty two percent of backwards particles beached, remained afloat, and originated from the open boundary, respectively, within −60 days of observation (Table 2). The average time from source to sighting location was 25 days. Thirty five, forty, and twenty five percent of forward particles beached, remained afloat, and reached the open boundary, respectively, within +60 days of observation. The average time from sighting location to coastal sink was 31.4 days. Example backwards trajectories reveal the influence of the southern cyclonic gyre and, to a lesser extent, the WAC and EAC (Figure 5). Forward trajectories suggest export via the WAC (Figures 6A,C) and recirculation in the southern gyre accompanied by limited exchange with the central Adriatic via the EAC (Figures 6B,D).
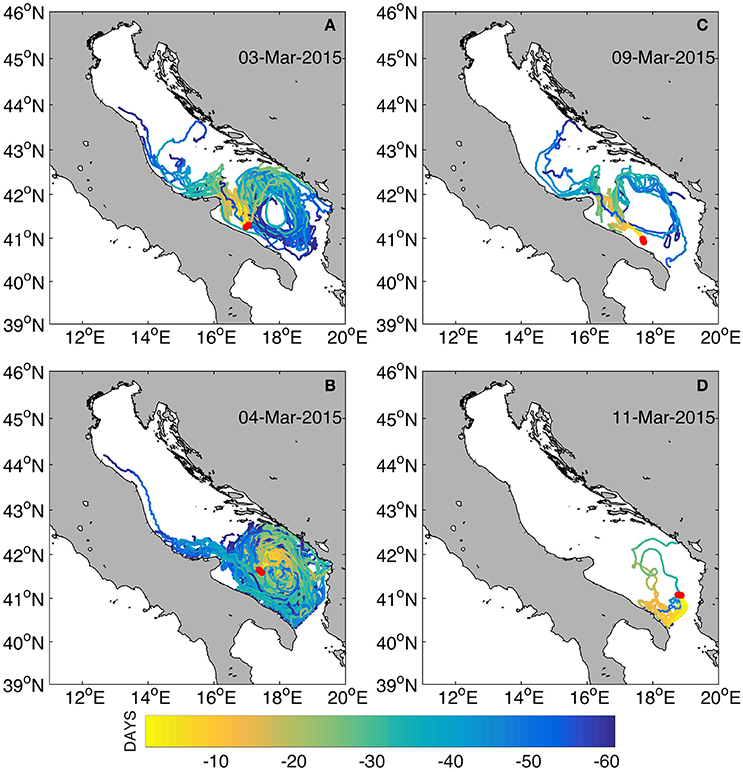
Figure 5. Selected backwards particle trajectories based on observations of floating anthropogenic marine debris on 3 March 2015 (A), 4 March 2015 (B), 9 March 2015 (C), and 11 March 2015 (D). The release locations are indicated by red circles and the color scale corresponds to time in days since initial release.
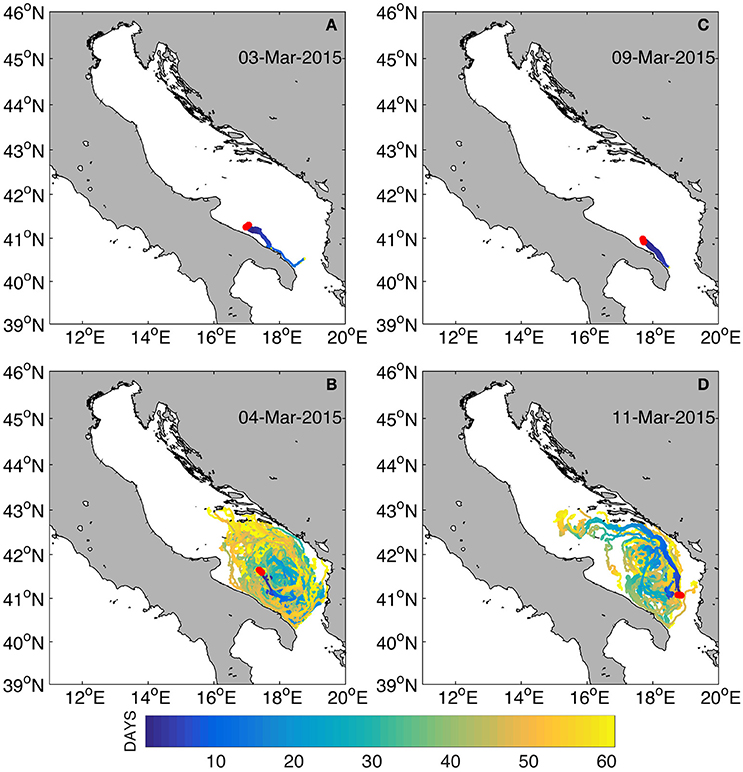
Figure 6. Selected forward particle trajectories based on observations of floating anthropogenic marine debris on 3 March 2015 (A), 4 March 2015 (B), 9 March 2015 (C), and 11 March 2015 (D). The release locations are indicated by red circles and the color scale corresponds to time in days since initial release.
Connection percentages (Figure 7) show that a 200 km stretch of the Italian coastline from the Otranto Strait to south of the Gargano Peninsula (segments 1–5) acted as both sources and sinks for the observed debris. The central Italian coastline, from the Gargano Peninsula to Conero (segments 7–13) acted as sources. Much of the central, eastern coast, from 42°N to 44°N acted as sinks. The southeastern coast acted as sources and sinks, with segment 33 on the Albanian coast providing over 80% of debris sighted on transects 13 and 14. Winds throughout the entire period were highly variable, with alternating Mistral and Sirocco events (Figure 7D).
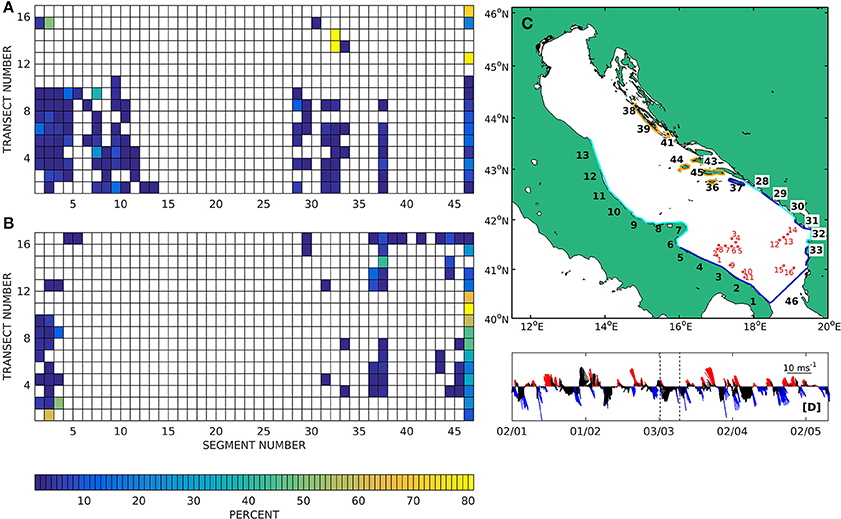
Figure 7. Percentages of particles from a given transect (rows) that reach a given coastal segment (columns) for backward (A) and forward (B) trajectories computed from the March 2015 debris. (C) Coastline segments that served as both sources and sinks are plotted in dark blue. Light blue segments acted as sources only and orange segments acted as sinks. Transect locations and numbers are shown in red. (D) Stick plot of winds during the particle tracking experiment. Red (blue) indicates Sirocco (Mistral) winds and the vertical dashed lines denote the time period of the AMD observations.
3.2.3. November 2015: Northern Adriatic Sea
Backwards particle trajectories suggest that sighted debris originated within the Adriatic, as none of the particles came from the open boundary (Table 2, Figures 8, 10A). Eighty eight and twelve percent of backwards particles were beached and still afloat, respectively, within −60 days of sighting (Table 2). The average time from source to sighting location was approximately 23 days. Example backward trajectories illustrate the complex, unpredictable nature of Lagrangian transport (Figure 8). Particles were transported by the EAC and the northern arm of the central cyclonic gyre, as well as the southern gyre (Figure 8). Backwards connection percentages confirm that most of the sighted debris originated from the central and northern Adriatic (Figure 10A). On the central Italian coastline, the most active source regions were segments 10 and 14 (Figure 10A). In the northern Adriatic, the Istrian Peninsula was also an active source (segments 21–24). Segment 38 was the most active source on the east coast, corresponding to the Croatian Island of Dugi otok.
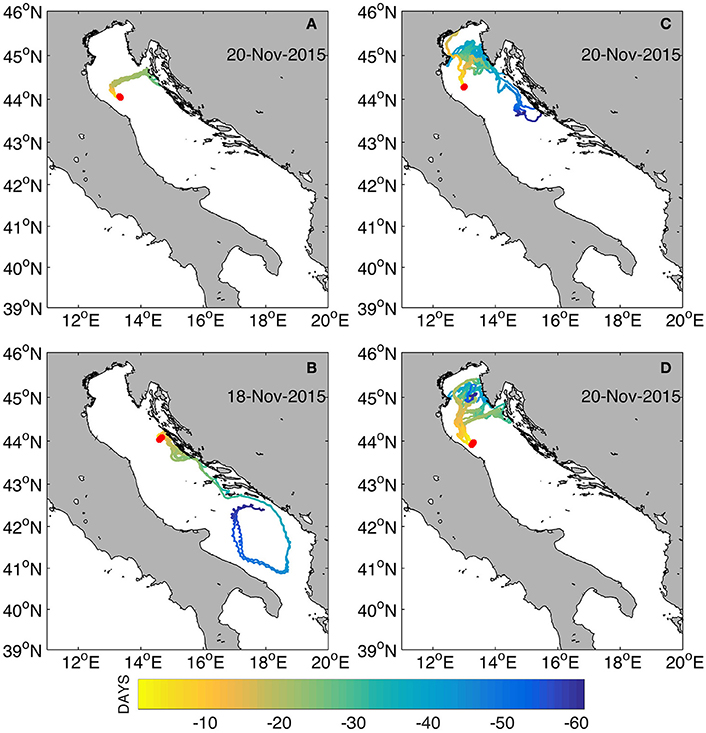
Figure 8. Selected backwards particle trajectories based on observations of floating anthropogenic marine debris on 20 November 2015 (A), 18 November 2015 (B), 20 November 2015 (C), and 20 November 2015 (D). The release locations are indicated by red circles and the color scale corresponds to time in days since initial release.
Forward trajectories also remained largely in the Adriatic region, with 82% beached and 17.9% afloat within +60 days of sighting (Table 2). A very small subset (0.1%) of forward particles reached the open boundary. The average time from coastal sink to sighting location was 21.5 days. Example forward trajectories show unexpected northward transport (Figure 9A) and recirculation (Figure 9B), as well as more typical alongshore transport in the WAC (Figures 9C,D). Connection percentages show that the central and southern Italian coastline acted as sinks, with the Po River delta (segment 18) and the northern Gargano Peninsula (segments 7–8) receiving much of the sighted debris (Figure 10B). Strong Sirocco wind events were likely responsible for deviations from mean circulation patterns. For example, the reversal of particles observed in forward particles (Figure 9A) was likely driven by upwelling-favorable Sirocco winds (Figure 10D).
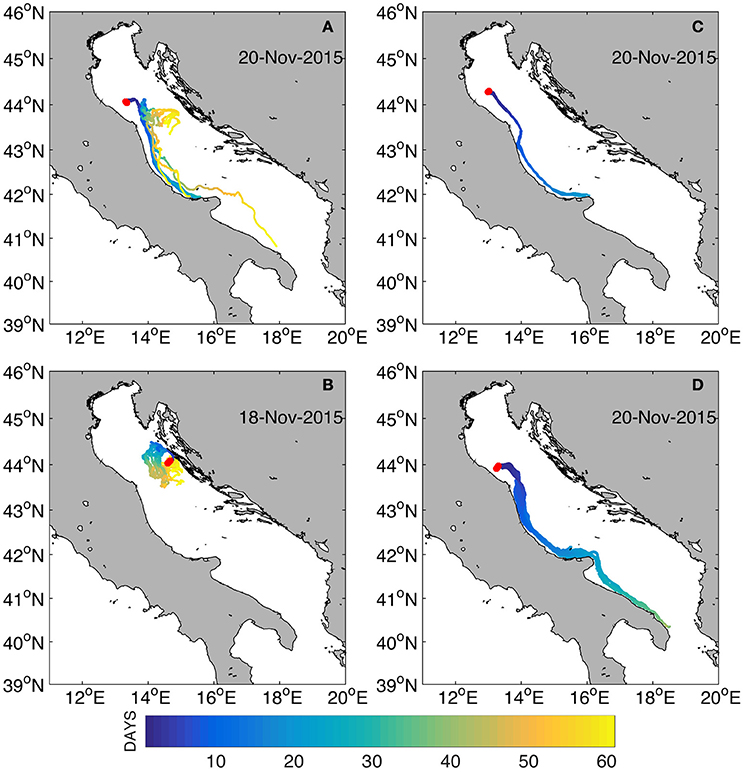
Figure 9. Selected forward particle trajectories based on observations of floating anthropogenic marine debris on 20 November 2015 (A), 18 November 2015 (B), 20 November 2015 (C), and 20 November 2015 (D). The release locations are indicated by red circles and the color scale corresponds to time in days since initial release.
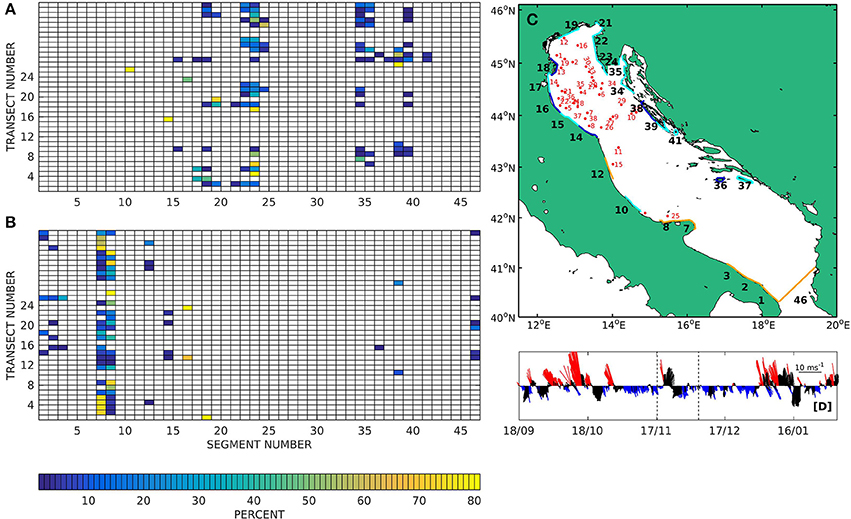
Figure 10. Percentages of particles from a given transect (rows) that reach a given coastal segment (columns) for backward (A) and forward (B) trajectories computed from the November 2015 debris. (C) Coastline segments that served as both sources and sinks are plotted in dark blue. Light blue segments acted as sources only and orange segments acted as sinks. Transect locations and numbers are shown in red. (D) Stick plot of winds during the particle tracking experiment. Red (blue) indicates Sirocco (Mistral) winds and the vertical dashed lines denote the time period of the AMD observations.
3.3. General Summary
Overall, the observed AMD originated largely from coastal segments near population centers and major rivers and was transported by the cyclonic surface circulation until either stranding, exiting the Adriatic, or recirculating in the southern gyre (Figures 2–9). Overall, 63, 16, and 21% of backwards particles beached, remained afloat, or originated from the open boundary, respectively (Table 2). In forward time, 55, 27, and 18% of particles beached, remained afloat, or were transported to the open boundary. The average residence time was 47.2 days and was slightly longer in forward time (24.3 days) when compared to backward time (22.9 days).
Of the 64 coastal segments used to identify possible sources and sinks of floating AMD in the Adriatic Sea, 41 segments acted as sources and 32 acted as sinks (Figure 1). The Venice lagoon segment did not serve as a source or sink for the observed floating AMD in any of the three experiments. The remaining unaffected coastal segments were concentrated in the northeastern Adriatic on the inshore, eastern sides of islands and peninsulas (Figure 1).
The results suggest that the central and southern gyres could have supplied AMD in May 2013 and March 2015 (Figure 11). Similarly, particles remained in the southern gyre in all three experiments after +60 days, suggesting that these regions can act as retention zones. However, the finite and relatively short integration period does not accurately reflect the lifetimes of AMD, especially plastics, and this restriction is addressed in Section 4.2.
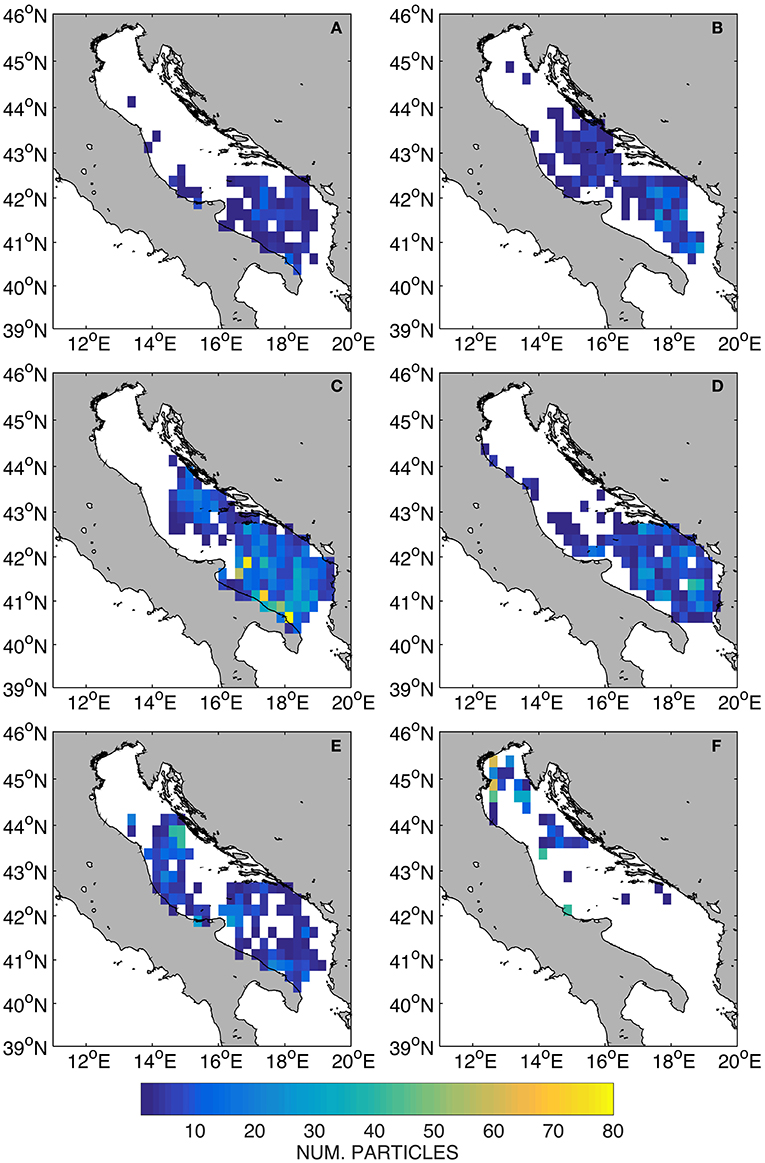
Figure 11. The number of particles per bin reveals the spatial distribution particles still afloat after 60 days in forward (left column) and backward (right column) time for debris observed in May 2013 (A,B), March 2015 (C,D), and November 2015 (E,F).
4. Discussion
The results suggest that the proposed methodology can advance the study and mitigation of marine debris by removing an often unrealistic and limiting assumption about abundances and source locations of macro debris. Here we discuss Adriatic-specific results and general aspects of the proposed methodology separately, and then propose improvements.
4.1. The Adriatic Sea
Potential coastal sources and sinks of floating AMD in the Adriatic Sea were identified using a two-way Lagrangian particle tracking model that was initialized by observed locations and times of debris abundances. Particle trajectories were computed using Equation 1 largely for simplicity but also because the Lagrangian validation presented in Carlson et al. (2016) suggests that an advective scheme is sufficient. Inclusion of a stochastic term complicates backwards particle tracking (see Section 4.2) and near-shore stochastic motion could cause a particle to erroneously ”jump” onto, or off of, land (Carlson et al., 2010). Windage and degradation are not considered as the effects of both variables on the transport of AMD are still poorly understood (Yoon et al., 2010; Critchell and Lambrechts, 2016). As such, the results presented here are most applicable to buoyant AMD with minimal above-water surface area, such as fragments made of polyethylene and polypropylene, which indeed represent the two most abundant polymers found in surface waters worldwide, as well as in the Mediterranean Sea (Suaria et al., 2016).
The 60 day integration time scale was chosen based on the average half-life of drifters and particles, drifter-particle separation rates (Carlson et al., 2016), and transit times reported in the literature. A ~45 day half-life of both drifters (Poulain, 2001) and virtual particles (Liubartseva et al., 2016) supports the 60 day integration time, allowing sufficient time for a particle to transit the Adriatic Sea while still maintaining a somewhat realistic trajectory.
The 5 m minimum depth of the AdriaROMS domain necessitates the use of a commonly-used, but physically unrealistic, assumption about beaching. Specifically, particles that stagnate on the boundary are treated as beached. Additionally, the model domain does not extend to the northern Ionian Sea and particles stagnate on the open boundary and cannot technically leave, nor re-enter the Adriatic Sea. As a result, the origin/fate of approximately 20% of particles from both backward and forward trajectories cannot be determined. Similarly, particles that remained afloat after ±60 days provide no definitive information about sources or sinks of the sighted debris. Additionally, the model domain does not extend to the shoreline and, therefore, result in additional uncertainty in the source and sink estimates.
The transport pathways, residence times, and probable sources and sinks identified agree well with previous studies of the surface circulation (Poulain, 1999, 2001; Ursella et al., 2006; Poulain and Hariri, 2013; Carlson et al., 2016) and marine debris (Liubartseva et al., 2016) in the Adriatic Sea. Carlson et al. (2016) also reported average transit times of 20–60 days from a coastal region in the northwest Adriatic to a coastal region in the southwest. Liubartseva et al. (2016) attempted to identify sources and sinks, as well as AMD concentrations in marine areas (see Section 2.1). The results presented here are derived from a simple advective Lagrangian particle tracking scheme and are consistent with the findings of Liubartseva et al. (2016), which were based on relatively complex Markov chain and ensemble techniques. Liubartseva et al. (2016) found high concentrations along the northwest coast as well as enhanced concentrations in the gyres during winter and higher concentration near the southeastern coast in fall. Their results also show qualitative agreement with the debris observation in Figure 1A, upon which our study is based. The main difference is the lack of debris originating from and traveling to the Venice coastal segment, which may simply be due to the timing and location of the debris surveys, pointing to the need for additional measurements to support the modeling component.
Finally, the application of the methodology to the Adriatic represents opportunistic synergy of marine litter surveys and Lagrangian particle tracking. The debris surveys were not designed with a modeling component in mind nor was the model designed to include beaching or stranding of debris, or export from the basin. Using observed abundances also limits the total number of particles available for analysis thereby impacting the statistical significance of the results. Improvements to the Adriatic implementation include higher resolution observations and velocity fields, validation of particle tracking results using observations of stranded debris, and resolution of near-shore dynamics. While the debris survey data used here represent the most comprehensive observations of floating macro debris in the Adriatic Sea, gaps exist in both space and time (Figure 1A). These biases are evident in complex, semi-enclosed basins like the Adriatic Sea where the mesoscale dominates the variability in the surface transport (Carlson et al., 2016).
4.2. General Assessment
While global and country-specific production, consumption, and disposal rates of plastic are available (Lebreton et al., 2012; Hardesty et al., 2015; Jambeck et al., 2015; Liubartseva et al., 2016), reliable estimates of the spatial and temporal distributions of plastic sources are lacking (Reisser et al., 2013). As a result, modeling studies often assume population-dependent, time-invariant AMD input (Lebreton et al., 2012; Liubartseva et al., 2016) or use spatially uniform initial positions (Yoon et al., 2010; Mansui et al., 2015). The consequences of such deployment schemes cannot be assessed until more reliable estimates of source locations and injection rates become available.
The proposed methodology merits continued development given the satisfactory agreement with previous studies in terms of identifying potential coastal source and sink regions as well as residence times (Poulain, 1999, 2001; Poulain and Hariri, 2013; Carlson et al., 2016; Liubartseva et al., 2016). The main deficiencies identified in Section 4.1 are not unique to the Adriatic Sea and should be addressed to maximize the potential of this method. Successful implementation of the methods demonstrated here will require observations of actual AMD abundances (Hardesty et al., 2015), high-resolution ocean models, observations of beaching and re-floating of debris over a wider range of coastline types (sandy beaches, rocky shores, marshes, etc.), and improved parameterizations of AMD lifetimes, degradation rates, windage, etc.
Boundary conditions in current Lagrangian particle tracking models poorly represent interactions of real AMD with actual shorelines. This, combined with the limited ability of most models to resolve nearshore dynamics, results in unrealistic numerical representations of beaching/stranding and re-floating of AMD. Beaching is a complex process that depends on a combination of coastline type, bathymetry, waves, winds, and tides, and other factors. Furthermore, cross-shore velocities outside the surf zone tend to be small (Largier, 2003) and “sticky waters” have been observed to retain tracers some distance offshore (Restrepo et al., 2014).
The temporal and spatial resolutions of the velocities employed in the particle tracking model must also adequately resolve the most energetic circulation features. However, even high-resolution models cannot resolve motion at the scales relevant to actual debris (mm to m). As a result, sub-grid-scale motions are often represented by adding a stochastic term to the particle velocities (Carlson et al., 2010; Fredj et al., 2016). Such an approach assumes that the flow can be decomposed into either a mean or “large-scale” component and a perturbation (Falco et al., 2000; Carlson et al., 2010). However, no clear separation has been observed in the wave number-frequency spectrum calling into question such an assumption (Carlson et al., 2010). Furthermore, the most common implementation of stochastic Lagrangian models assumes constant, isotropic eddy diffusivity, which may not be appropriate, especially near shore. The type of stochastic model, i.e., random walk or random flight, also depends on the statistics of the perturbation term (Carlson et al., 2010), a fact that is often glossed over in applied settings. Finally, a stochastic term complicates backwards particle tracking as the integration is no longer a reversible process. Thus, Lagrangian modeling of AMD, while a potentially powerful management tool, can be complicated by a number of factors, including assumptions about the amount of AMD and source regions, debris behavior parameters, and the proper sub-grid-scale turbulence parameterization.
Lagrangian evaluations of ocean model performance are also lacking (Zambianchi et al., 2017). As the transport of marine debris is inherently a Lagrangian problem, both ocean models and debris transport models should be evaluated more extensively using Lagrangian observations (Hardesty et al., 2017). Lagrangian assessments typically compare surface or drogued drifter trajectories to virtual particle trajectories (Liu and Weisberg, 2011; Carlson et al., 2016), yet quantitative comparisons between drifter and AMD trajectories over large distances in oceanic environments have not yet been reported. Parameterizations of AMD behavior (i.e., windage, degradation, and settling) also require validation (Critchell and Lambrechts, 2016). The proposed methodology can be implemented in any region where both AMD surveys are conducted and a high-resolution numerical model solution is in place.
Author Contributions
Visual surveys of floating debris were performed by GS, TF, and VM. EF developed the particle tracking scheme and analysis methods. AR ran the ocean and atmospheric models. DC performed the particle tracking and analysis. DC and GS prepared the bulk of the manuscript. DC, GS, SA, AG, EF, AR, and TF contributed to the experimental design and editing of the manuscript for publication.
Funding
Funding for ship-time was provided through EU FP-7 project COCONET (Grant Agreement No. 287844), the SOLEMON survey was supported by the FAOADRIAMED regional project. VM was supported by the DeFishGear (Derelict Fishing Gear Management System in the Adriatic Region) IPA Adriatic strategic project 1 str/00010 implemented with co-funding by the European Union, Instrument for Pre-Accession Assistance (IPA).
Conflict of Interest Statement
The authors declare that the research was conducted in the absence of any commercial or financial relationships that could be construed as a potential conflict of interest.
The reviewer CB declared a shared affiliation, though no recent collaboration, with two of the authors AG and SA to the handling Editor, who ensured that the process nevertheless met the standards of a fair and objective review.
Acknowledgments
The authors are thankful to crews and officers of RV Urania, RV Minerva Uno, and RV G. Dallaporta.
Abbreviations
AMD, anthropogenic marine debris.
References
Aliani, S., and Molcard, A. (2003). Hitch-hiking on floating marine debris: macrobenthic species in the western Mediterranean Sea. Hydrobiologia 503, 59–67. doi: 10.1023/B:HYDR.0000008480.95045.26
Artegiani, A., Bregant, D., Paschini, E., Pinardi, N., Raicich, F., and Russo, A. (1997). Applications of stochastic particle models to oceanographic problems. J. Phys. Oceanogr. 27, 1515–1532.
Avio, C. G., Gorbi, S., and Regoli, F. (2015). Experimental development of a new protocol for extraction and characterization of microplastics in fish tissues: first observations in commercial species from Adriatic Sea. Mar. Environ. Res. 111, 18–26. doi: 10.1016/j.marenvres.2015.06.014
Benetazzo, A., Carniel, S., Sclavo, M., and Bergamasco, A. (2013). Wave-current interaction: effect on the wave field in a semi-enclosed basin. Ocean Model. 70, 152–165. doi: 10.1016/j.ocemod.2012.12.009
Blašković, A., Fastelli, P., Čižmek, H., Guerranti, C., and Renzi, M. (2017). Plastic litter in sediments from the Croatian marine protected area of the natural park of Telaščica bay (Adriatic Sea). Mar. Pollut. Bull. 114, 583–586. doi: 10.1016/j.marpolbul.2016.09.018
Carlson, D., Griffa, A., Zambianchi, E., Suaria, G., Corgnati, L., Magaldi, M., et al. (2016). Observed and modeled surface Lagrangian transport between coastal regions in the Adriatic Sea with implications for marine protected areas. Cont. Shelf Res. 118, 23–48. doi: 10.1016/j.csr.2016.02.012
Carlson, D. F., Fredj, E., Gildor, H., and Rom-Kedar, V. (2010). Deducing an upper bound to the horizontal eddy diffusivity using a stochastic Lagrangian model. Environ. Fluid Mech. 10, 499–520. doi: 10.1007/s10652-010-9181-0
Critchell, K., Grech, A., Schlaefer, J., Andutta, F., Lambrechts, J., Wolanski, E., et al. (2015). Modelling the fate of marine debris along a complex shoreline: lessons from the Great Barrier Reef. Estuar. Coast. Shelf Sci. 167, 414–426. doi: 10.1016/j.ecss.2015.10.018
Critchell, K., and Lambrechts, J. (2016). Modelling accumulation of marine plastics in the coastal zone; what are the dominant physical processes? Estuar. Coast. Shelf Sci. 171, 111–122. doi: 10.1016/j.ecss.2016.01.036
Efron, B., and Tibshirani, R. (1986). Bootstrap methods for standard errors, confidence intervals, and other measures of statistical accuracy. Stat. Sci. 1, 54–77. doi: 10.1214/ss/1177013815
Falco, P., Griffa, A., Poulain, P.-M., and Zambianchi, E. (2000). Transport properties in the Adriatic Sea as deduced from drifter data. J. Phys. Oceanogr. 30, 2055–2071. doi: 10.1175/1520-0485(2000)030<2055:TPITAS>2.0.CO;2
Fredj, E., Carlson, D. F., Amitai, Y., Gozolchiani, A., and Gildor, H. (2016). The particle tracking and analysis toolbox (PaTATO) for Matlab. Limnol. Oceanogr. Methods 14, 586–599. doi: 10.1002/lom3.10114
Gajšt, Tamarat, T., Bizjak, T., Palatinus, A., Liubartseva, S., and Kržan, A. (2016). Sea surface microplastics in slovenian part of the northern adriatic. Mar. Pollut. Bullet. 113, 392–399. doi: 10.1016/j.marpolbul.2016.10.031
Galgani, F. (2015). Marine litter, future prospects for research. Front. Mar. Sci. 2:87. doi: 10.3389/fmars.2015.00087
Galgani, F., Hanke, G., Werner, S., Oosterbaan, L., Nilsson, P., Fleet, D., et al. (2013). Monitoring Guidance for Marine Litter in European Seas. JRC Scientific and Policy Reports, EUR 26113 EN, 120.
Galgani, F., Leaute, J., Moguedet, P., Souplet, A., Verin, Y., Carpentier, A., et al. (2000). Litter on the sea floor along European coasts. Mar. Pollut. Bullet. 40, 516–527. doi: 10.1016/S0025-326X(99)00234-9
Grati, F., Scarcella, G., Polidori, P., Domenichetti, F., Bolognini, L., Gramolini, R., et al. (2013). Multi-annual investigation of the spatial distributions of juvenile and adult sole (Solea solea L.) in the Adriatic Sea (northern Mediterranean). J. Sea Res. 84, 122–132. doi: 10.1016/j.seares.2013.05.001
Griffa, A. (1996). “Stochastic modeling in physical oceanography,” in Applications of Stochastic Particle Models to Oceanographic Problems, Vol. 39, eds R. Adler, P. Müller, and B. L. V. Rozovskii (Boston, MA: Springer Science & Business Media), 114–140.
Haidvogel, D., Arango, H., Budgell, W., Cornuelle, B., Curchitser, E., Lorenzo, E. D., et al. (2008). Ocean forecasting in terrain-following coordinates: formulation and skill assessment of the regional ocean modeling system. J. Comput. Phys. 227, 3595–3624. doi: 10.1016/j.jcp.2007.06.016
Hardesty, B., Good, T., and Wilcox, C. (2015). Novel methods, new results and science-based solutions to tackle marine debris impacts on wildlife. Ocean Coast. Manage. 115, 4–9. doi: 10.1016/j.ocecoaman.2015.04.004
Hardesty, B., Harari, J., Isobe, A., Lebreton, L., Maximenko, N., Potemra, J., et al. (2017). Using numerical model simulations to improve the understanding of micro-plastic distribution and pathways in the marine environment. Front. Mar. Sci. 4:30. doi: 10.3389/fmars.2017.00030
Haza, A. C., Özgökmen, T. M., Griffa, A., Garraffo, Z. D., and Piterbarg, L. (2012). Parameterization of particle transport at submesoscales in the Gulf Stream region using Lagrangian subgridscale models. Ocean Modell. 42, 31–49. doi: 10.1016/j.ocemod.2011.11.005
Isobe, A., Kubo, K., Tamura, Y., Kako, S., Nakashima, E., and Fujii, N. (2014). Selective transport of microplastics and mesoplastics by drifting in coastal waters. Mar. Pollut. Bullet. 89, 324–330. doi: 10.1016/j.marpolbul.2014.09.041
Jambeck, J. R., Geyer, R., Wilcox, C., Siegler, T. R., Perryman, M., Andrady, A., et al. (2015). Plastic waste inputs from land into the ocean. Science 347, 768–771. doi: 10.1126/science.1260352
Kako, S., Isobe, A., Kataoka, T., and Hinata, H. (2014). A decadal prediction of the quantity of plastic marine debris littered on beaches of the East Asian marginal seas. Mar. Pollut. Bullet. 81, 174–184. doi: 10.1016/j.marpolbul.2014.01.057
Kako, S., Isobe, A., and Magome, S. (2010). Sequential monitoring of beach litter using webcams. Mar. Pollut. Bullet. 60, 775–779. doi: 10.1016/j.marpolbul.2010.03.009
Kako, S., Isobe, A., Magnome, S., Hinata, H., Seino, S., and Kojima, A. (2011). Establishment of numerical beach-litter hindcast/forecast models: an application to Goto Islands, Japan. Mar. Pollut. Bullet. 62, 293–302. doi: 10.1016/j.marpolbul.2010.10.011
Laglbauer, B. J., Franco-Santos, R. M., Andreu-Cazenave, M., Brunelli, L., Papadatou, M., Palatinus, A., et al. (2014). Macrodebris and microplastics from beaches in Slovenia. Mar. Pollut. Bullet. 89, 356–366. doi: 10.1016/j.marpolbul.2014.09.036
Largier, J. (2003). Considerations in estimating larval dispersal distances from oceanographic data. Ecol. Appl. 13, S71–S89. doi: 10.1890/1051-0761(2003)013[0071:CIELDD]2.0.CO;2
Lazar, B., and Gračan, R. (2011). Ingestion of marine debris by loggerhead sea turtles, Caretta caretta, in the Adriatic Sea. Mar. Pollut. Bullet. 62, 43–47. doi: 10.1016/j.marpolbul.2010.09.013
Lebreton, L., Greer, S., and Borrero, J. (2012). Numerical modelling of floating debris in the world's oceans. Mar. Pollut. Bullet. 64, 653–661. doi: 10.1016/j.marpolbul.2011.10.027
Liu, Y., and Weisberg, R. H. (2011). Evaluation of trajectory modeling in different dynamic regions using normalized cumulative Lagrangian separation. J. Geophys. Res. Oceans 116, C09013. doi: 10.1029/2010jc006837
Liubartseva, S., Coppini, G., Lecci, R., and Creti, S. (2016). Regional approach to modeling the transport of floating plastic debris in the Adriatic Sea. Mar. Pollut. Bullet. 103, 115–127. doi: 10.1016/j.marpolbul.2015.12.031
Magaldi, M., Özgökmen, T., Griffa, A., and Rixen, M. (2010). On the response of a turbulent coastal buoyant current to wind events: the case of the western Adriatic current. Ocean Dyn. 60, 93–122. doi: 10.1007/s10236-009-0247-9
Mansui, J., Molcard, A., and Ourmières, Y. (2015). Modelling the transport and accumulation of floating marine debris in the Mediterranean basin. Mar. Pollut. Bullet. 91, 249–257. doi: 10.1016/j.marpolbul.2014.11.037
Maximenko, N., Hafner, J., and Niiler, P. (2012). Pathways of marine debris derived from trajectories of Lagrangian drifters. Mar. Pollut. Bullet. 65, 51–62. doi: 10.1016/j.marpolbul.2011.04.016
Mazzariol, S., Di Guardo, G., Petrella, A., Marsili, L., Fossi, C. M., Leonzio, C., et al. (2011). Sometimes sperm whales (Physeter macrocephalus) cannot find their way back to the high seas: a multidisciplinary study on a mass stranding. PLoS ONE 6:e19417. doi: 10.1371/journal.pone.0019417
Munari, C., Corbau, C., Simeoni, U., and Mistri, M. (2016). Marine litter on Mediterranean shores: analysis of composition, spatial distribution and sources in north-western Adriatic beaches. Waste Manage. 49, 483–490. doi: 10.1016/j.wasman.2015.12.010
Neumann, D., Callies, U., and Matthies, M. (2014). Marine litter ensemble transport simulations in the North Sea. Mar. Pollut. Bullet. 86, 219–228. doi: 10.1016/j.marpolbul.2014.07.016
Pasquini, G., Ronchi, F., Strafella, P., Scarcella, G., and Fortibuoni, T. (2016). Seabed litter composition, distribution and sources in the Northern and Central Adriatic Sea (Mediterranean). Waste Manage. 58, 41–51. doi: 10.1016/j.wasman.2016.08.038
Poppi, L., Zaccaroni, A., Pasotto, D., Dotto, G., Marcer, F., Scaravelli, D., et al. (2012). Post-mortem investigations on a leatherback turtle Dermochelys coriacea stranded along the northern Adriatic coastline. Dis. Aquat. Org. 100, 71–76. doi: 10.3354/dao02479
Poulain, P.-M. (1999). Drifter observations of surface circulation in the Adriatic Sea between December 1994 and March 1996. J. Mar. Syst. 20, 231–253. doi: 10.1016/S0924-7963(98)00084-0
Poulain, P.-M. (2001). Adriatic Sea surface circulation as derived from drifter data between 1990 and 1999. J. Mar. Syst. 29, 3–32. doi: 10.1016/S0924-7963(01)00007-0
Poulain, P.-M., and Hariri, S. (2013). Transit and residence times in the Adriatic Sea surface as derived from drifter data and Lagrangian numerical simulations. Ocean Sci. 9, 713–720. doi: 10.5194/os-9-713-2013
Pribanic, S., Holcer, D., and Miokovic, D. (1999). First report of plastic ingestion by striped dolphin (Stenella coeruleoalba) in the Croatian part of the Adriatic Sea. Eur. Res. Cetaceans 13, 443–446.
Putman, N. F., and He, R. (2013). Tracking the long-distance dispersal of marine organisms: sensitivity to ocean model resolution. J. R. Soc. Interface 10:20120979. doi: 10.1098/rsif.2012.0979
Reisser, J., Shaw, J., Wilcox, C., Hardesty, B., Proietti, M., Thums, M., et al. (2013). Marine plastic pollution in waters around Australia: Characteristics, concentrations, and pathways. PLoS ONE 8:e80466. doi: 10.1371/journal.pone.0080466
Restrepo, J., Venkataramani, S., and Dawson, C. (2014). Nearshore sticky waters. Ocean Model. 80, 49–58. doi: 10.1016/j.ocemod.2014.06.003
Russo, A., Carniel, S., and Benetazzo, A. (2013a). Support for ICZM and MSP in the Adriatic Sea region. Sea Technol. 54, 27–35.
Russo, A., Coluccelli, A., Carniel, S., Benetazzo, A., Valentini, A., Paccagnella, T., et al. (2013b). “Operational models hierarchy for short term marine predictions: the Adriatic Sea example,” in OCEANS, Bergen, 2013 MTS/IEEE, 1–6. doi: 10.1109/OCEANS-Bergen.2013.6608139
Russo, A., Coluccelli, A., Iermano, I., Falcieri, F., Ravaioli, M., Bortoluzzi, G., et al., M. R. (2009). An operational system for forecasting hypoxic events in the northern Adriatic Sea. Geofizika 26, 191–213.
Shchepetkin, A., and McWilliams, J. (2005). The regional ocean modeling system (ROMS): a split-explicit, free-surface, topography-following-coordinate oceanic model. Ocean Model. 9, 347–404. doi: 10.1016/j.ocemod.2004.08.002
Slavin, C., Grage, A., and Campbell, M. (2012). Linking social drivers of marine debris with actual marine debris on beaches. Mar. Pollut. Bullet. 64, 1580–1588. doi: 10.1016/j.marpolbul.2012.05.018
Strafella, P., Fabi, G., Spagnolo, A., Grati, F., Polidori, P., Punzo, E., et al. (2015). Spatial pattern and weight of seabed marine litter in the northern and central Adriatic Sea. Mar. Pollut. Bullet. 91, 120–127. doi: 10.1016/j.marpolbul.2014.12.018
Suaria, G., and Aliani, S. (2014). Floating debris in the Mediterranean Sea. Mar. Pollut. Bullet. 86, 494–504. doi: 10.1016/j.marpolbul.2014.06.025
Suaria, G., Avio, C. G., Mineo, A., Lattin, G. L., Magaldi, M. G., Belmonte, G., et al. (2016). The Mediterranean Plastic Soup: synthetic polymers in Mediterranean surface waters. Sci. Rep. 6:37551. doi: 10.1038/srep37551
Ursella, L., Poulain, P.-M., and Signell, R. P. (2006). Surface drifter derived circulation in the northern and middle Adriatic Sea: response to wind regime and season. J. Geophys. Res. Oceans 111, C03S04. doi: 10.1029/2005JC003177
van Sebille, E., England, M. H., and Froyland, G. (2012). Origin, dynamics, and evolution of ocean garbage patches from observed surface drifters. Environ. Res. Lett. 7:044040. doi: 10.1088/1748-9326/7/4/044040
Vianello, A., Boldrin, A., Guerriero, P., Moschino, V., Rella, R., Sturaro, A., et al. (2013). Microplastic particles in sediments of Lagoon of Venice, Italy: first observations on occurrence, spatial patterns and identification. Estuar. Coast. Shelf Sci. 130, 54–61. doi: 10.1016/j.ecss.2013.03.022
Wieczorek, S., Campagnuolo, S., Moore, P., Froglia, C., Atkinson, R., Gramitto, E., et al. (1999). The composition and fate of discards from Nephrops trawling in Scottish and Italian waters. EC Study Project Rep. 96:092.
Yoon, J.-H., Kawano, S., and Igawa, S. (2010). Modeling of marine litter drift and beaching in the Japan Sea. Mar. Pollut. Bullet. 60, 448–463. doi: 10.1016/j.marpolbul.2009.09.033
Keywords: marine litter, floating debris, Adriatic Sea, lagrangian particle tracking, ROMS
Citation: Carlson DF, Suaria G, Aliani S, Fredj E, Fortibuoni T, Griffa A, Russo A and Melli V (2017) Combining Litter Observations with a Regional Ocean Model to Identify Sources and Sinks of Floating Debris in a Semi-enclosed Basin: The Adriatic Sea. Front. Mar. Sci. 4:78. doi: 10.3389/fmars.2017.00078
Received: 01 November 2016; Accepted: 07 March 2017;
Published: 04 April 2017.
Edited by:
Francois Galgani, French Research Institute for Exploitation of the Sea, FranceReviewed by:
Gotzon Basterretxea, Consejo Superior de Investigaciones Científicas, SpainSvitlana Liubartseva, Fondazione CMCC - Centro Euro-Mediterraneo sui Cambiamenti Climatici, Italy
Carlo Brandini, Consiglio Nazionale delle Ricerche (CNR), Italy
Copyright © 2017 Carlson, Suaria, Aliani, Fredj, Fortibuoni, Griffa, Russo and Melli. This is an open-access article distributed under the terms of the Creative Commons Attribution License (CC BY). The use, distribution or reproduction in other forums is permitted, provided the original author(s) or licensor are credited and that the original publication in this journal is cited, in accordance with accepted academic practice. No use, distribution or reproduction is permitted which does not comply with these terms.
*Correspondence: Daniel F. Carlson, ZGFuZmNhcmxzb25AZ21haWwuY29t
Stefano Aliani, c3RlZmFuby5hbGlhbmlAaXNtYXIuY25yLml0
†Present Address: Aniello Russo, Science and Technology Organisation - Centre for Maritime Research and Experimentation (STO CMRE), NATO, La Spezia, Italy