- 1Département de Biologie and Québec-Océan, Université Laval, Quebec, QC, Canada
- 2Takuvik, Joint International Laboratory, UMI 3376, Centre National de la Recherche Scientifique (CNRS, France) and Université Laval, Québec, QC, Canada
- 3Institut de Biologie Intégrative et des Systèmes, Université Laval, Quebec, QC, Canada
Recent studies have focused on how climate change could drive changes in phytoplankton communities in the Arctic. In contrast, ciliates and dinoflagellates that can contribute substantially to the mortality of phytoplankton have received less attention. Some dinoflagellate and ciliate species can also contribute to net photosynthesis, which suggests that species composition could reflect food web complexity. To identify potential seasonal and annual species occurrence patterns and to link species with environmental conditions, we first examined the seasonal pattern of microzooplankton and then performed an in-depth analysis of interannual species variability. We used high-throughput amplicon sequencing to identify ciliates and dinoflagellates to the lowest taxonomic level using a curated Arctic 18S rRNA gene database. DNA- and RNA-derived reads were generated from samples collected from the Canadian Arctic from November 2007 to July 2008. The proportion of ciliate reads increased in the surface toward summer, when salinity was lower and smaller phytoplankton prey were abundant, while chloroplastidic dinoflagellate species increased at the subsurface chlorophyll maxima (SCM), where inorganic nutrient concentrations were higher. Comparing communities collected in summer and fall from 2003 to 2010, we found that microzooplankton community composition change was associated with the record ice minimum in the summer of 2007. Specifically, reads from smaller predatory species like Laboea, Monodinium, and Strombidium and several unclassified ciliates increased in the summer after 2007, while the other usually summer-dominant dinoflagellate taxa decreased. The ability to exploit smaller prey, which are predicted to dominate the future Arctic, could be an advantage for these smaller ciliates in the wake of the changing climate.
Introduction
The climate driven changes now occurring across the Arctic are expected to affect marine phytoplankton communities in many ways, for example, by limiting nutrient supply, lowering surface salinities, and potentially by ocean acidification (Coupel et al., 2012, 2014; Riebesell et al., 2013; Thoisen et al., 2015). The loss of multi-year ice has implications for the sea-ice communities (Comeau et al., 2013), and consequently the Arctic food chain (Søreide et al., 2010). But less intuitively, the loss of multi-year ice can have an impact on the pelagic communities, for example by lengthening the open water season and influencing the depth of the upper mixed layer in this salinity stratified ocean. In the Canada Basin and elsewhere, changes in the timing and character of phytoplankton production have already been reported (Li et al., 2009; Ardyna et al., 2014). Less attention has been given to the microzooplankton (ciliates and dinoflagellates) that consume from 22 to 75% of the daily phytoplankton production in the Arctic and other cold water oceans (Paranjape, 1990; Landry and Calbet, 2004; Sherr and Sherr, 2009; Sherr et al., 2013). Ciliates and dinoflagellates are also influenced by top-down processes, where changes in macrozooplankton species are linked to the successional progression of the microzooplankton communities (Riisgaard et al., 2014).
Microzooplankton species, especially dinoflagellates, use a variety of techniques to capture prey (e.g., Nielsen and Kiørboe, 2015), with some ciliates being fastidious in terms of nutritional requirements (e.g., Johnson, 2011), and others having a narrow size specificity range (e.g., Park and Kim, 2010). Because of such selectivity, microzooplankton could have a top-down effect on phytoplankton and protist community composition (Irigoien, 2005), with implications for microbial food webs, nutrient, and carbon cycles, as well as zooplankton nutrition and higher food webs. In addition to their major role as phagotrophs, many microzooplankton are mixotrophic and able to carry out photosynthesis. In particular, about half of the described species of dinoflagellates are photosynthetic with integrated chloroplasts (Saldarriaga et al., 2001; Hansen, 2011). Other dinoflagellates, along with some ciliates, host photosynthetic symbionts, or are kleptoplasidic (Esteban et al., 2010). The range of trophic roles among microzooplankton suggest that the microzooplankton species composition could be used as indicators of differences in microbial food webs. Such an approach would require testing for associations among particular taxa and the conditions where they occur. However, there have been few studies focusing on occurrence patterns of ciliates and dinoflagellates in the Arctic (Nelson et al., 2014).
The use of 18S rRNA gene surveys has highlighted the prevalence of dinoflagellates and ciliates in the Arctic, but most studies have been restricted to summer and early fall (Comeau et al., 2011). Dinoflagellates and ciliates persist over winter darkness in the Arctic (Terrado et al., 2011; Marquardt et al., 2016), but their seasonal progression is poorly understood. To resolve temporal patterns at finer taxonomic resolution, and to test for trends consistent with environmental selection among Arctic microzooplankton, we investigated samples collected from November 2007 to July 2008 during the International Polar Year Circumpolar Flaw Lead Study (IPY-CFL; Barber et al., 2010, 2012). The IPY samples from both surface waters and the top of the Pacific halocline, where a subsurface chlorophyll maximum (SCM) usually develops (McLaughlin and Carmack, 2010; Monier et al., 2015). We hypothesized that the strong seasonality and stratification in Amundsen Gulf (Beaufort Sea) would be sufficient to lead to niche partitioning and select different species assemblages at the two depths and over time. Both DNA and RNA (converted to cDNA) were used as templates for targeted amplicon sequencing to compare the community with potential for active protein synthesis (from RNA) and communities from DNA, which could include free DNA and cells in state of dormancy or encystment (Jones and Lennon, 2010; Hunt et al., 2013). Specifically, we applied high throughput sequencing (HTS) targeting the V4 region of 18S rRNA (referred to as rRNA) and the 18S rRNA gene (referred to as rDNA) to determine species level composition in the communities. To test for interannual variability vs. trends over time, we re-analyzed the 8 years of ciliate and dinoflagellate amplicon data from Amundsen Gulf reported in Comeau et al. (2011). For both the seasonal and interannual studies, we classified the microzooplankton reads using an improved reference database (Lovejoy et al., 2016) and constructed robust phylogenies. We applied multivariate statistics to link changes in community structure and composition with prevalent environmental drivers, to provide insights on how microzooplankton communities respond to seasonal changes and inter-annual variability.
Materials and Methods
Sample Collection, Extraction, and Sequencing
Seasonal samples were collected onboard the Canadian Coast Guard Research Icebreaker CCGS Amundsen every 2–4 weeks from November 2007 to July 2008 from Amundsen Gulf and adjacent bays (Figure 1A). We targeted the polar mixed layer (PML) near the surface (5–20 m), and the top of a halocline (22–80 m), which separates the PML from Pacific Summer Water (PSW). In late spring the Beaufort Sea SCM typically forms at this halocline (Bergeron and Tremblay, 2014). During winter and early spring, the halocline sample depth was identified by salinity and temperature profiles with salinities of 31–32, and from May to July confirmed by the deeper chlorophyll fluorescence peak.
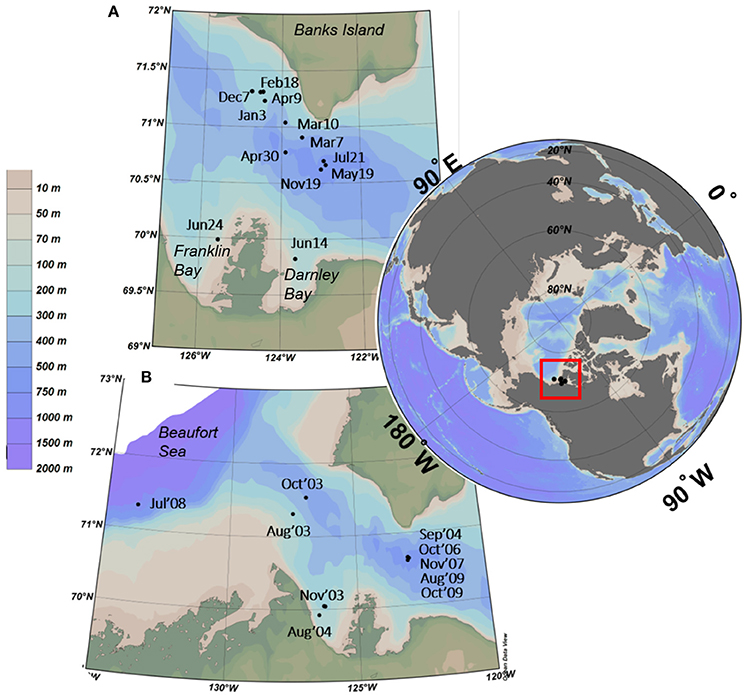
Figure 1. Sites and dates of collection of water samples used for (A) seasonal (November 2007-July 2008) and (B) interannual (2003-2010) studies from the Amundsen Gulf region and adjacent Darnley and Franklin Bays.
Samples used to investigate interannual variability of ciliate and dinoflagellate communities in the SCM were collected during multiple missions to the Amundsen Gulf and Beaufort Sea between 2003 and 2010, aboard the CCGS Amundsen (2003-2006, 2009-2010), Louis S. St. Laurent (2007), and Sir Wilfred Laurier (2008). Details of these missions have been reported elsewhere (Barber et al., 2010, 2012; Comeau et al., 2011; Terrado et al., 2011).
Except for 2010, all sample were preserved using the same methods, and following extraction, all were amplified and sequenced using the same protocols. Briefly, water samples for DNA (ca. 6 L) and RNA (ca. 4 L) were collected into cleaned acid rinsed carboys from 12-L (CCGS Amundsen) or 10-L (CCGS Louis St Laurent, Sir Wilfred Laurier) Niskin-type bottles mounted on a Rosette system equipped with a conductivity, temperature and depth (CTD; Sea-Bird Electronics Inc., California), chlorophyll fluorescence (Seapoint Sensors Inc.), and relative nitrate from ultraviolet spectrophotometer (ISUS, Satlantic) profilers. Target depths were identified by fluorescence, salinity, temperature, and relative nitrate from the downcast and water collected on the upcast. All samples were filtered and preserved within 2 h of collection. Following pre-filtration through a 50-μm mesh to remove macrozooplankton, samples were sequentially filtered using a peristaltic pump (Cole-Parmer, USA) onto a 3-μm pore-size 47-mm polycarbonate filter (PC, AMD Manufacturing), and 0.2 μm pore-size Sterivex™ unit (Millipore) for the DNA and a 47-mm 0.2 μm pore-size PC filter for the RNA. All PC filters were placed in 2-mL cryovials. Prior to 2010, DNA was preserved by adding 1.8 mL of lysis buffer to the Sterivex units (0.2–3 μm fraction) and cryovials (3–50 μm fraction), and RNA was preserved in RLT buffer (Qiagen) as in Terrado et al. (2011) and Thaler and Lovejoy (2015). Samples were either frozen immediately at −80°C or placed in liquid nitrogen after adding buffer. Samples collected in 2010 were preserved using RNALater (Ambio). After 30–60 min in buffer, the filters were flash frozen in liquid nitrogen and then kept at −80°C until processing in the laboratory. We note that preliminary tests on paired samples from both marine and freshwater environments showed no significant differences in DNA and RNA recovery or community compositions between the protocols (Lovejoy pers. comm.). For samples collected before 2010, DNA was extracted from the filters as in Terrado et al. (2011) using a salt based method (Aljanabi and Martinez, 1997) while RNA was extracted using the RNAEasy micro Kit (Qiagen). The sample collected in 2010 was extracted using the AllPrep DNA/RNA Mini Kit (Qiagen) with DNA and RNA from the same filters. Conversion of RNA to cDNA was carried out using the High Capacity Reverse Transcription Kit (Applied Biosystems, Foster City, CA, USA). The V4 region of 18S rRNA in both DNA and cDNA samples was amplified using eukaryotic specific forward primers E572F with the Roche A adapter and reverse primer E1009R as described by Comeau et al. (2011). For the seasonal samples, large and small fractions were pooled after normalization based on relative chlorophyll concentrations (Supplementary Table 1). Only the 0.2–3 μm fraction was used in the interannual study. Separate sequencing runs were carried out for the seasonal and interannual studies. For each run, equimolar concentrations of amplicons from each sample were pooled for multiplex sequencing using the GS FLX Titanium Roche 454 platform (Roche/454 Life Sciences, Branford, CT, USA) at IBIS/Université Laval Plateforme d'Analyses Génomiques (Quebec, QC, Canada). All reads have been deposited in the Short Read Archive of NCBI under accession codes: PRJNA283142 (IPY-CFL) and SRA029114 (Amundsen Gulf Time Series, Comeau et al., 2011).
Post-sequence Data Processing and Taxonomic Classification
The seasonal data reads were quality filtered using the Quantitative Insights into Microbial Ecology program (QIIME; Caporaso et al., 2010b). Short sequences and primers were identified in mothur and removed (Schloss et al., 2009). Data were then de-noised following Reeder and Knight (2010). Chimeric sequences were identified in UCHIME (Edgar et al., 2011) and removed. Interannual data was processed using mothur (Schloss et al., 2009) as described in Comeau et al. (2011). The two data sets were then processed for OTU picking at 98% similarity utilizing USEARCH (Edgar, 2010) against the Silva Reference Database v.102 (Pruesse et al., 2007). All OTU representative reads were aligned in PyNast (Caporaso et al., 2010a), manually curated in BioEdit v.7.2.5 (Hall, 1999) and used to construct a phylogenetic tree (FastTree; Price et al., 2010). Assignment of taxonomic identity was performed in mothur with a 0.8 confidence threshold against the Northern Reference Database v.1.0 (Lovejoy et al., 2016), which follows the Silva taxonomy but includes high-quality longer environmental sequences from the Arctic and North Atlantic (Terrado et al., 2009, 2011; Charvet et al., 2012; Monier et al., 2013; Dasilva et al., 2014). However, using this database, around 30–45% of the reads were still not classified beyond “Other Dinoflagellates” or “Other Ciliates.”
To further increase taxonomic resolution, dinoflagellate sequences from Saldarriaga et al. (2001, 2004), Logares et al. (2007), and Potvin et al. (2013), and ciliate sequences from Dunthorn et al. (2014), along with Evolutionary Placement Algorithm, Randomized-Axelerated Maximum Likelihood- (EPA RAxML, Stamatakis, 2014) abundant Arctic-derived HTS reads were added to the v.1.0 of Northern Reference Database (v.1.1, Lovejoy et al., 2016). Taxonomic classifications of the dinoflagellate sequences used as references were verified in the AlgaeBase.org (Guiry and Guiry, 2015) and by literature searches, while ciliate taxonomic classification followed Lynn (2012). Using the v.1.1 of the database, taxonomy assignment was re-performed to generate the final OTU matrix (OTU reads per sample) with improved results (i.e., “Other Dinoflagellates” and “Other Ciliates” went down to 5–10%). OTUs matching metazoa, fungi, higher plants and reads that could not be classified to any taxonomic level below Eukaryota were removed and not analyzed further. This cleaned data set was considered as the total microbial eukaryotic community. To facilitate diversity comparisons, the OTU matrix was then rarefied, based on the sample with the fewest reads in the separate data sets, resulting in 4953 reads per sample for the seasonal study, and 7041 reads per sample for the interannual comparison.
To investigate the OTUs that contributed to the changes in July 2008 for the interannual data (see Section Results), the remaining abundant “Other Ciliate” OTUs were searched for in NCBI and their most similar nearly full length 18S rRNA gene sequences were used to construct reference trees as in Thaler and Lovejoy (2015). Then, the abundant but unclassified ciliate OTUs were then mapped back onto the reference trees using EPA RAxML v.8 (Stamatakis, 2014). We then inferred their probable taxonomic identity based on the nearest or most similar annotated reference sequences.
Statistical and Diversity Analyses
Statistical tests were carried out in the R environment v 3.0 (R Development Core Team, 2008). Spearman's Rank Correlation (rho) in the Vegan package (Dixon and Dixon, 2003) was used to test correlations between taxa and environmental parameters, and results were plotted in igraph (Csárdi and Nepusz, 2006). Only correlations with Spearman's rho ≥ 0.3 and significant at p < 0.001 were retained (Barberán et al., 2012). Species-variable relationships were then visualized by network analysis as target-source plots in Cytoscape 3.0 (Shannon et al., 2003; see Supplementary Methods 1). Analysis of variance (ANOVA) was applied to determine significant differences between samples and linear regression tests were used to infer relationships, both analysis were carried out using PAST v3.0 (Hammer et al., 2001).
Alpha diversity (Chao1 index) of the ciliate and dinoflagellate communities in each seasonal sample was estimated as implemented in QIIME (Chao and Shen, 2003; Caporaso et al., 2010b). Since the samples used for the interannual part of the study were collected in different months (Jul to Nov) over the 8 years, we tested for variability associated with the month of collection and found no significant differences in species richness of OTUs in ciliate or dinoflagellate abundance between summer and autumn (Supplementary Figure 1). Phylogenetic unweighted UniFrac dissimilarities (beta diversity) among the taxa were computed separately for seasonal and interannual datasets by the jackknife method and generalized UniFrac procedure (Lozupone and Knight, 2005; Chen et al., 2012). All dinoflagellate and ciliate OTUs in the seasonal study were included in the beta diversity measure. To avoid potential rarefication artifacts (Ramette, 2007) in the interannual data, rare OTUs that were defined as < 1% of the total ciliate or dinoflagellate reads, were not included in the beta diversity and remaining analysis. The pairwise dissimilarity matrices were used to generate dendrograms using UPGMA (Price et al., 2010). The weighted contributions of seasons, depths (surface or SCM-halocline) and templates (RNA or DNA) to community structuring were computed using ADONIS (Fierer et al., 2012). The checkerboard score (C-score) was used to test for non-random co-occurrence patterns under the null hypothesis parameter using the oecosimu function in the R library bipartite with 1000 simulations (Dormann et al., 2008).
Results
Physico-Chemical Regimes of the Amundsen Gulf
For the seasonal study (Figure 1A), surface PML waters remained cold (−1.7 to 2°C) from mid-January to mid-May and increased toward summer reaching 8°C in July, when the region was ice-free. Phosphate concentration changed little over time with concentrations from 0.6 to 1.6 mg m−3. Over the 9 months, nitrate concentrations were significantly greater in the halocline compared to the surface (t-test, p < 0.001), with greatest concentrations from February to April (Table 1). Nitrate concentrations started to decrease beginning in early April in the surface and in May at the halocline. The chlorophyll a (Chl a) concentrations, as estimated from in situ fluorescence, were negligible until 9 April and were greater in the surface waters compared to halocline through 19 May. Concentrations were below 1 mg m−3 in both the surface and halocline until July when a strong SCM was apparent at the halocline (Table 1).
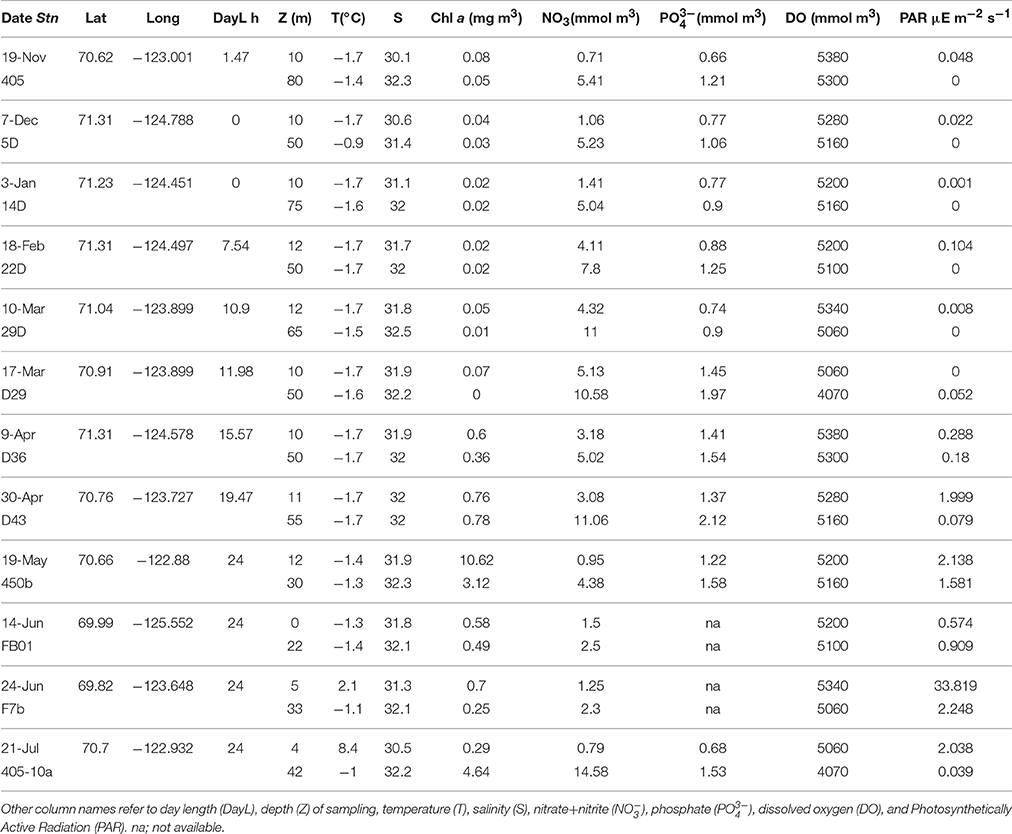
Table 1. Dates of collection 2007-2008 (Date), Stations (Stn), collection Latitude, and Longitude (Lat-Long), physico-chemical parameters, and chlorophyll a (Chl a) concentrations of the samples used for amplicon tag pyrosequencing.
The physical characteristics of the time series stations (Figure 1B) have been reported elsewhere (Comeau et al., 2011). Briefly, over the 8 years, nutrients in the SCM ranged from 0.09 to 8.22 mmol m−3 for , 0.46–1.29 mmol m−3 for PO4 and the highest Chl a (total) was detected on September 2005 reaching 2.14 mg m−3 (Supplementary Table 2). After binning the data from before and after 2007, total summer-fall Chl a values went from 0.43 mg m−3 to 0.31 mg m−3 in the SCM.
Community Clustering Based on 18S rRNA and 18S rRNA Genes
The seasonal rDNA and rRNA OTU communities separated into distinct surface and halocline clusters, with clear separation between the rDNA and rRNA communities within the depth defined clusters (Figure 2). Within the template clusters samples separated by season as Autumn-Winter (Nov-April) and Spring-Summer (May-July) categories, except for one rRNA surface sample collected on June 14, which clustered with the winter rRNA samples (Supplementary Figure 2). The similarity was mainly driven by the presence of several OTUs that occurred in the rRNA surface community on Jan 3 and June 14 but were absent in the other summer samples (Supplementary Table 3). We note that, because of ship time constraints, the June 14 samples were collected from Darnley Bay, which is an extension of Amundsen Gulf. For all samples, the ADONIS test also showed individual contributions to clustering of around 14% (adj. R2 = 0.148 and 0.146, p < 0.001) grouping by depth category and sample type, while season contributed the least to the variance observed (adj. R2 = 0.073, p < 0.001). The C-score test further supported the non-random distribution of the microzooplankton communities (p < 0.001).
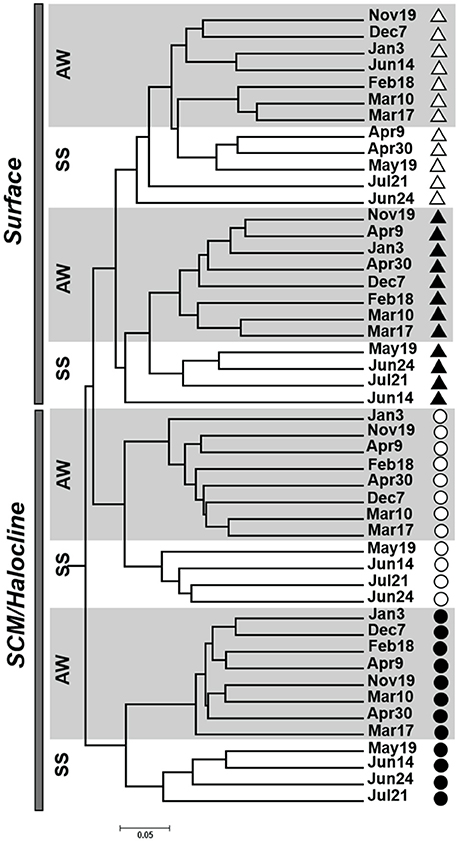
Figure 2. A dendrogram of the unweighted UniFrac dissimilarity matrix for DNA- (filled shapes) and RNA–based (open shapes) communities collected from surface (triangles) and subsurface chlorophyll maxima/halocline (SCM; circles) layers representing different seasons including Autumn-Winter (AW) and Spring -Summer (SS), collected over the course of the IPY-CFL study.
Seasonal Succession and Distribution
Since DNA can be retrieved from both living and senescent cells, and free DNA can persist in the environment (Torti et al., 2015), the RNA-sourced samples were used to investigate potential influence by local conditions. Based on rRNA reads, ciliates, and dinoflagellates were found in all samples (Figure 3, Supplementary Tables 4 and 5), with greater proportions during summer (ca. 40%) and lowest in spring (ca. 5%) when larger photosynthetic taxa (mostly diatoms, Joli et al., accepted) dominated the planktonic community. The combined mean seasonal alpha diversity (Chao 1 index) of both ciliates and dinoflagellates based on rDNA, decreased from 125 in winter to 83 after spring (Supplementary Figure 3). In terms of total relative abundance, ciliate proportions were similar in both depths during winter (Figures 3A,C) while dinoflagellates were better represented in the surface (Figures 3B,D). Ciliate read relative abundance increased in the surface starting early June (Figure 3A), while dinoflagellates increased in the halocline (Figure 3D).
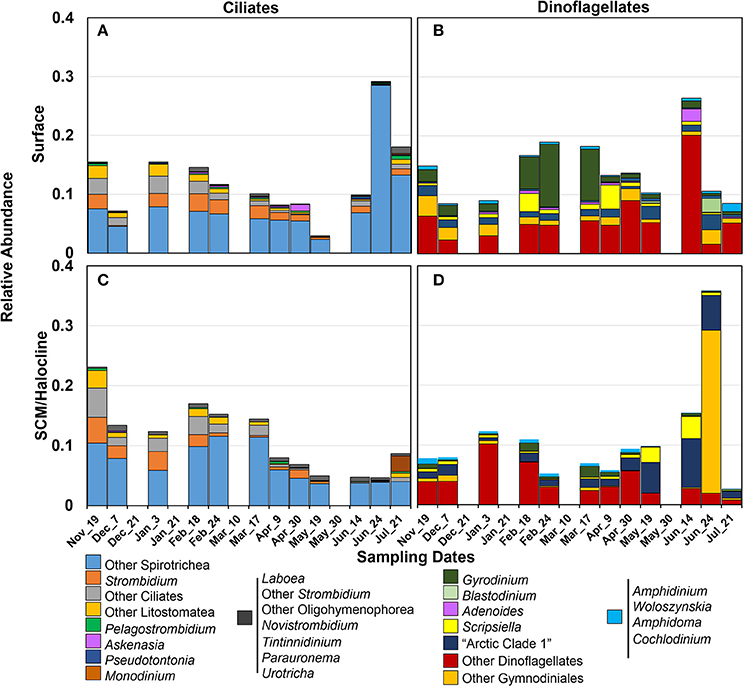
Figure 3. Distribution of potentially active (based on rRNA reads) major taxa of the lowest possible ranks in (A,C) ciliates and (B,D) dinoflagellates (98% similarity) with the changing seasons (sampling date) in surface and SCM halocline layers (see text). Samples were collected from Amundsen Gulf, Darnley Bay and Franklin Bay.
Significant associations (Spearman's rho > 0.3, p < 0.001) among taxa and environmental variables were detected in the network analysis (Figure 4). The associations were consistent with the seasonal succession and depth (water mass) seen in the UniFrac clustering (Figure 2). For example, surface-associated ciliates: Strombidium, Pseudotontonia, Askenasia, and dinoflagellates Gymnodinium-like, Adenoides-like, Gyrodinium c.f. gutrula, and Scripsiella (Figure 4, taxa 1–7) were associated with higher DO, which was a characteristic of the surface PML. In addition, abundances of Amphidoma, “Arctic Clade 1,” Blastodinium, Monodinium, and Novostrombidium (Figure 4, taxa 8–13) were higher when Chl a concentrations were greater, which was associated with longer day lengths and warmer water, suggesting characteristic surface summer taxa. Tintinnidium, Parauronema, Oligohymenophorea, Gyrodinium helveticum, and Amphidinium-like taxa (Figure 4, taxa 15–18) were more associated with the deeper, more saline and nutrient-richer SCM or halocline layer. Although Laboea (Figure 4, taxon 14) was also strongly correlated with the SCM/halocline conditions, it was most abundant when the days were longer. In contrast, Woloszynskia, Gymnodinium sp., and “Other Gyrodinium” (Figure 4, taxa 19, 21, 22) were more abundant in the halocline during winter when ice cover was more extensive. These species, correlated with specific environmental variables, provide evidence for succession and seasonality within the two water masses. Binned OTUs of poorly defined groups that were not classified beyond their respective ranks, including “Other” Dinoflagellates, Ciliophora, Spirotrichea, Litostomatea, and Strombidium (Figure 4, taxa 23–30), did not correlate with any of the environmental variables tested.
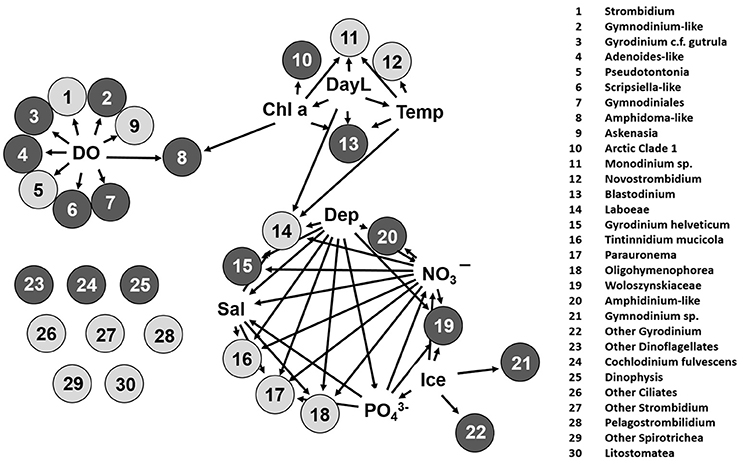
Figure 4. A network source-target plot showing the significant correlations based on relative abundances of OTUs binned at the lowest possible ranks and square root transformed environmental parameters. Only vertices (circles) and edges (arrows) that have Spearman's rho ≥ 0.3 significant at p < 0.001 were retained. Black circles are dinoflagellates and gray are ciliates. Environmental variables tested include dissolved oxygen (DO), total chlorophyll a (chl a), daylength (DayL), temperature (Temp), depth (Dep), salinity (Sal), nitrate+nitrite (NO3−), phosphate (), and ice. Numbers correspond to the identities on the right.
Interannual Variability
Ciliate communities were consistently dominated by “Other Spirotrichea” with annual mean abundance of 9.4 ± 2% relative to the total microbial eukaryotic reads. “Other ciliates” (1.7 ± 1%), Strombidium sp., Pelagostrombidium sp., “Other Oligohymenophorea,” “Other Litostomatea,” Askenasia sp., and Monodinium sp. abundances ranged from 0.01 to 1.5% of total microbial eukaryote relative abundance (Supplementary Figure 4A). The most abundant dinoflagellate OTUs were from Gymnodiniales, Blastodinium-like, “Arctic clade 1,” Gyrodinium, Adenoides-like, and unclassified Dinoflagellates, each accounting for 1–6% of the total microbial eukaryotic reads (Supplementary Figure 4B).
Here, using our refined taxonomic database we found that microzooplankton community composition also changed, especially in summer 2008. Specifically, based on abundant OTUs (>1%), the July 2008 sample showed the greatest dissimilarity from other communities (Figure 5A). This dissimilarity was driven by the July 2008 increase in both abundance and OTU richness of Strombidium, Laboea, Monodinium, and “Other Ciliates” (Figure 5B), with a significant correlation with decreasing salinity (r2 = 0.82, p < 0.01). After 2008, the relative abundances of these groups returned to previous levels by 2010. Their relative abundances were also inversely correlated (multiple regression) with SiO3 (r2 = 0.29, p < 0.001), salinity (r2 = 0.22, p = 0.1), and nitrate (r2 = 0.18, p = 0.2). Phylogenetic placement of the “Other Ciliate” reads further revealed that these were in the Class Spirotrichea, and included sub-classes Oligotrichia and Choreotrichia. Most of these Spirotrichea clustered with environmental sequences, which we refer to as Environmental Clades 1, 2, 3, 4, 5, and 6 (Supplementary Figure 5). Aggregated read counts of Oligotrichia, and Environmental Clades 1, 4, 5, and 6 showed significant increase after 2007 (Krustal Wallis, p < 0.01), while Choreotrichia, and Environmental Clades 2 and 3 decreased.
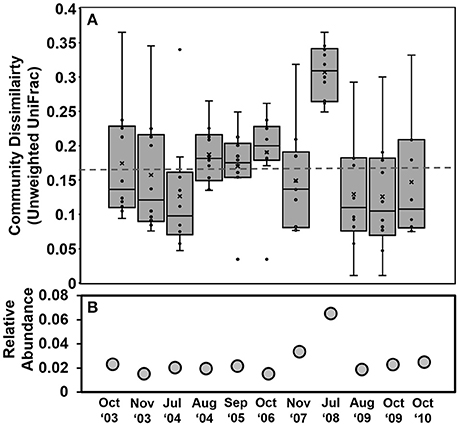
Figure 5. (A) The pairwise unweighted UniFrac community dissimilarity of combined ciliate and dinoflagellate communities. In the boxplots, one point (dot) represents the pairwise Unweighted UniFrac dissimilarity (Y-axis) of a particular sample against another sample. Thus, for each date there are 10 dots (overlapping dots are obscured) representing that date compared against 10 dates. The black line within the box, is the mean UniFrac distance of a particular sample against all the other samples. The broken line indicates the mean dissimilarity value among all samples. (B) Combined relative abundances of the major taxa contributing to the high July 8 dissimilarity, particularly of Strombidinium, Laboea, Monodinium sp. and other unclassified ciliates.
Discussion
Temporal and distributional studies of ciliates and dinoflagellates are usually restricted to morphologically recognizable species (Montagnes, 1996; Levinsen and Nielsen, 2002). The use of HTS coupled with the improved reference database provided higher resolution of potential species that suggests high diversity compared to previous reports from the Arctic. Overall, we discriminated around 30 taxa per 100 reads, with the total number of reads always higher for dinoflagellates than for ciliates. This translated into 251 dinoflagellates and 141 ciliate species based on OTUs defined as 98% similar as estimated using Choa1. These species estimates are considerably higher than the 55 ciliate species reported in the Western Canada Basin (Jiang et al., 2013) and the <20 dinoflagellate species reported in the Beaufort Sea (Okolodkov and Dodgeb, 1996) based on microscopy. The high proportion of environmental clades here suggests that there are likely ciliate and dinoflagellate species confined to the Arctic, as is the case for diatoms (Luddington et al., 2016) and prasinophytes (Lovejoy et al., 2007).
Interpretation of DNA and RNA-Derived Abundances and Diversity
Eukaryotic microbial communities from rDNA have been used to infer water mass history (Hamilton et al., 2008; Monier et al., 2013; Zhang et al., 2014), whereas taxa from rRNA are thought to more closely indicate active representatives of the community (Campbell and Kirchman, 2013; Hunt et al., 2013). Here, we found that for both DNA and RNA sourced samples, the surface communities always clustered together separate from the SCM communities. Earlier microscopy (Lovejoy et al., 1993; Okolodkov and Dodgeb, 1996; Jiang et al., 2013) and clone library studies using longer 18S rRNA sequences (Bachy et al., 2011; Lovejoy and Potvin, 2011) have also highlighted the differences between SCM and surface communities over the Arctic. Within the two water masses, communities separated by template suggests a pool of historic DNA, which could include dormant or less active stages, e.g., cysts (Verni and Rosati, 2011; Bravo and Figueroa, 2014), advected non-active species (Lovejoy and Potvin, 2011), preserved free DNA, or non-living material in marine snow (Nielsen et al., 2007; Boere et al., 2011). All of these sources can be transported by currents and persist over long distances (Heiskanen, 1993; Brocks and Banfield, 2009).
Although RNA-derived reads cannot be directly used to estimate biomass (Medinger et al., 2010; Blazewicz et al., 2013), the patterns of relative read abundance were consistent with specific environmental drivers operating on particular species or clades, with communities separating by season. One exception was the June 14 rRNA surface sample from Darnley Bay, which based on OTU composition clustered with the January 3 rRNA sample. The placement of the June sample in an otherwise winter cluster was consistent with deeper unmodified Pacific Winter Water appearing in Darnley Bay, which was evident in the physico-chemical sample clustering (Supplementary Figure 2). Upwelling and on-shelf transport of deeper Pacific Water from Amundsen Gulf has been reported previously (Garneau et al., 2006; Paulic et al., 2012). Unfortunately, CTD transects were not taken along the shelf break in June and the source of this water remains speculative. The biological clustering was driven by 15 OTUs belonging to “Arctic Clade 1,” Gyrodinium, Gymnodinium, “Other Dinoflagellates,” Askenasia, Tintinndinium, “Other Spirotrichea,” and “Other Ciliates.”
Environmental Influences on Microzooplankton
While the dependence on phytoplankton prey can generate annual bimodal or unimodal microzooplankton bloom patterns in some regions, with temperature having an influence (Levinsen and Nielsen, 2002; Godhantaraman and Uye, 2003), there is little understanding of species composition over annual cycles. Here we found that ciliate and dinoflagellate taxa showed distinct seasonal patterns that could be partially explained by salinity, temperature, and nutrient changes from winter to summer (Figure 4). Specifically, the proportion of ciliate reads were generally higher under ice free conditions, when the surface waters were less saline and at the end of the spring bloom when nitrate concentrations were drawn down by photosynthetic activity (Forest et al., 2010; Tremblay et al., 2011). Although many ciliates have a wide range of salinity tolerance (Montagnes, 1996), this has not been investigated for Arctic species. In contrast dinoflagellate proportions were higher in the surface in winter, but more abundant in the SCM toward summer. Dinoflagellates can produce the osmolyte dimethylsulfoniopropionate (DMSP), which allow cells to regulate internal homeostasis (see review by Stefels, 2000), which may provide an advantage relative to ciliates under conditions of slightly higher salinity.
During late spring and toward summer with increasing light availability, the putative plastid-containing ciliates and dinoflagellates increased proportionally in the rRNA OTUs, suggesting that photosynthesis may have provided additional energy for photosynthetic or mixotrophic taxa. In Amundsen Gulf, in summer, the higher sun angle and longer day-length mean that more light reaches the nutrient-rich halocline leading to the establishment of the SCM. We found that ciliate and dinoflagellate reads made up on average ca. 60% of the total microbial eukaryotic reads in early summer in the SCM. In contrast, a microscopy study in 2005-2006 reported that photosynthetic and non-photosynthetic dinoflagellates constitute only around 3–6% of the total mean phytoplankton cell counts in the SCM (Martin et al., 2010). The discrepancy is likely related to cell breakage during collection, misidentification with smaller dinoflagellates grouped with other flagellates, and loss during storage. Ciliates, can also be fragile and tend to be either ignored or under sampled using standard Lugol's techniques (Lovejoy et al., 1993). Ciliate and dinoflagellate dominance in 18S rRNA gene libraries has also been attributed to their comparatively larger genomes and multiple copies of the rRNA gene (Godhe et al., 2008). Irrespective of the actual cell abundance, our analysis clearly revealed otherwise undetected changes in species over seasons (Supplementary Tables 4 and 5). Our seasonal data ended in July, but the interannual data in the SCM showed that both alveolate groups remain through summer to late autumn.
Ecological Functions
Season and depth were also the major factors that grouped ciliates and dinoflagellates using the network analyses. The seasonal changes in microbial composition also suggested shifts in dominant function within the two groups (Figure 4). For example, winter communities were mainly dominated by heterotrophic taxa. The surface winter communities would have access to small planktonic species such as Micromonas (Lovejoy et al., 2007), haptophytes, and smaller heterotrophic flagellates (Terrado et al., 2011). The relative scarcity of food resources during winter likely selected for particular groups as reported for Kongsfjorden, Svalbard (Seuthe et al., 2011), and Disko Bay (Levinsen et al., 2000; Levinsen and Nielsen, 2002). Dinoflagellates can also graze on smaller ciliates using pseudopodia or feeding veils (Jacobson and Anderson, 1986, 1996). Such trophic interactions, with dinoflagellates most likely preying on ciliates, could contribute to differences in relative abundances in different depths and times (Hansen et al., 1999; Møller et al., 2006; Seuthe et al., 2011). Additional trophic interactions were indicated in the summer when primary production was higher and zooplankton were more abundant in surface waters (Seuthe et al., 2007; Forest et al., 2011), with the appearance of the putative copepod parasite Blastodinium.
After spring and toward summer, the correlation of some ciliates and dinoflagellates with Chl a might also be associated with predation on phytoplankton. Ciliates and dinoflagellates consume from 30% (15.6 g C m−2) of gross primary production in Amundsen Gulf to as much as 56% in some Arctic fjords (Seuthe et al., 2007; Forest et al., 2011). The availability of light in spring and summer could favor mixotrophy as well. For example, the obligate mixotrophic Laboea and most litostomatids, and the potentially plastidic Scripsiella-like, “Other Gyrodinium,” “Other Gymnodiniales,” and Adenoides further increased in abundance in the SCM over this period when both light and prey would be available.
Interannual Microzooplankton and the Changing Arctic
The summer-autumn ciliate and dinoflagellate assemblages tended to be similar in the different years, with a consistent predictable re-establishment of communities most years, despite samples being collected from across a large geographical distance. The SCM in the Beaufort Sea forms near the upper surface of the Pacific Winter Water, which is transported as a distinct entity over long distances (Carmack and Macdonald, 2002). Previous studies consistently show that protist communities in the SCM of Beaufort Sea and Amundsen Gulf change little over geographical space and for a given year are strongly associated with their water mass of origin (e.g., Comeau et al., 2011; Lovejoy and Potvin, 2011; Monier et al., 2015). The strong association with water mass was evident in the two samples collected in July 2008 that were analyzed separately because of differences in sample preparation protocols between the seasonal study and the interannual study. The internannual study was only from the small fraction, while the seasonal study included amplicons from large and small fractions. Despite this, the dinoflagellate and ciliate OTUs from Amundsen Gulf collected 8 July, were similar to those collected further offshore in the Beaufort Sea on 21 July, consistent with both 2008 samples from the same water mass and similar conditions.
Lovejoy et al. (2002) showed in microcosm experiments in the Arctic that ciliates and dinoflagellates dominated when nutrients were depleted following blooms of large phytoplankton. Ciliates are able to exploit oligotrophic conditions, by grazing on smaller phytoplankton and bacteria either as strict heterotrophs or by way of mixotrophy. By the end of July 2008, the Beaufort Sea SCM was nitrate limited and had the lowest mean phosphate concentrations measured from 2003 to 2010. Overall primary productivity was low (Martin et al., 2013) with small cells (<3 μm) dominating the Chl a biomass (Comeau et al., 2011). The mean salinity at the SCM was lower in 2008 compared to the preceding years (2003-2007), which was due to the input of additional freshwater from multi-year ice melt in 2007. This event was part of the ongoing trend in decreasing summer ice extent that has been recorded since the onset of the satellite era. The continuing low ice volume over winter was associated with the 2008 ice break-up 2 weeks before the historic average. This precocious ice breakup stimulated an earlier pelagic spring bloom and earlier depletion of nutrients in the euphotic zone (Forest et al., 2011). These July 2008 conditions were reflected in the microzooplankton assemblage, characterized by the greater prevalence of Strombidinium, Laboea, Monodinium sp., other unclassified ciliates and relative decrease in most dinoflagellates, which was also noted in the seasonal data set. The relative abundance of ciliate taxa in the above community gradually returned to earlier levels along with the partial recovery of summer ice extent. At the OTU level, Spirotrichea showed different trends, before and after 2007. Spirotrichea ciliates are obligate mixotrophs, feeding on smaller prey and presumably able to use retained chlorophyte or prymnesiophyte-derived plastids for photosynthesis (Stoecker et al., 1988, 2009; McManus and Katz, 2009). Similar observations were reported by Jiang et al. (2013) in Western Arctic Ocean where a few ciliate groups became dominant during the 2012 record ice melt, supporting the notion that microzooplankton are highly sensitive to changing physico-chemical regimes, including those associated with low-ice or freshening events.
The mean vertical positions of the SCM and the nitracline in the Beaufort Sea and Amundsen Gulf deepened from 2003 to 2011 (Bergeron and Tremblay, 2014). The increasing distance between the depth at which light is still sufficient for photosynthesis and the nitracline will continue to accelerate under a changing climate regime (Yamamoto-Kawai et al., 2008; McLaughlin and Carmack, 2010; Krishfield et al., 2014), which could drive both the surface and SCM layers to become more nutrient-limited. Such an effect has already been reported for the Canada Basin with an increase in smaller phytoplankton and lower nitrate levels in the upper 200 m (Li et al., 2009). Under these new conditions ciliates and dinoflagellates that are better able exploit smaller prey would be selected for. Our results support recent mesocosm and modeling experiments that suggest that under future conditions, the microzooplankton and microbial loop will become more prominent as efficient intermediates between bacteria-picoplankton and the classical food webs (Skjoldborg et al., 2003; O'Connor et al., 2009; Montagnes et al., 2010; Lewandowska et al., 2014; D'Alelio et al., 2016).
In summary, this study shows that in Amundsen Gulf, ciliates and dinoflagellates exhibit complex temporal dynamics and are influenced by biological and physico-chemical regulators. Ciliate reads were more abundant in the surface when salinity was lower toward summer while dinoflagellates dominated in the SCM where nutrients and light were available for potential mixotrophic or photosynthetic activity. Despite this strong seasonality, the microzooplankton assemblages were also highly similar every summer from 2003 to 2010 except in 2008, following the 2007 summer ice minimum. Mixotrophic taxa increased drastically when nutrient concentrations were low and small prey were presumably available. Low nutrient-small cell conditions are predicted to occur more frequently in the Arctic, and this may lead to a change in dominant microzooplankton species that link to the classical food webs.
Author Contributions
DO and CL conceived the project. CL and EM collected samples. EM and AC carried out the laboratory work, and pre-processed the sequence data. DO, AC, and CL analyzed the data, DO and CL wrote the manuscript and prepared the figures. MB was responsible oceanographic analysis supervision. All authors commented on the text and agreed to this submission.
Funding
The seasonal data was collected during Circumpolar Flaw Lead—International Polar Year (CFL-IPY) study supported by the Natural Sciences and Engineering Research Council of Canada (NSERC), and the Network of Centers of Excellence ArcticNet. NSERC Discovery grants and ArcticNet funding to CL facilitated completion of the study. DFLO received scholarships from Université Laval and the Canadian Excellence Research Chair—Remote Sensing of Canada's New Arctic Frontier (CERC) grant, and additional support from the Fonds de recherche du Québec Nature et Technologies (FRQNT) to Quebéc-Océan aided in this research. We also acknowledge support from Compute Canada.
Conflict of Interest Statement
The authors declare that the research was conducted in the absence of any commercial or financial relationships that could be construed as a potential conflict of interest.
Acknowledgments
The authors acknowledge the help and assistance of staff at the Institute of Ocean Sciences, Department of Fisheries and Oceans Canada, the Captains and Crews of CCGS Amundsen, Louis S St. Laurent, and Wilfred Laurier. We are also grateful to Jonathan Gagnon and Jean-Éric Tremblay for the nutrient data, and to Ramon Terrado colleagues from ICM, CSIC Barcelona and Marianne Potvin in carrying out some of the sampling and laboratory work.
Supplementary Material
The Supplementary Material for this article can be found online at: http://journal.frontiersin.org/article/10.3389/fmars.2017.00016/full#supplementary-material
References
Aljanabi, S. M., and Martinez, I. (1997). Universal and rapid salt-extraction of high quality genomic DNA for PCR-based techniques. Nucleic Acid Res. 25, 4692–4693. doi: 10.1093/nar/25.22.4692
Ardyna, M., Babin, M., Gosselin, M., Devred, E., Rainville, L., and Tremblay, J. È. (2014). Recent Arctic Ocean sea ice loss triggers novel fall phytoplankton blooms. Geophys. Res. Lett. 41, 1–6. doi: 10.1002/2014gl061047
Bachy, C., López-García, P., Vereshchaka, A., and Moreira, D. (2011). Diversity and vertical distribution of microbial eukaryotes in the snow, sea ice and seawater near the north pole at the end of the polar night. Front. Microbiol. 2:106. doi: 10.3389/fmicb.2011.00106
Barber, D. G., Asplin, M. G., Raddatz, R. L., Candlish, L. M., Nickels, S., and Prinsenberg, S. J. (2012). Change and variability in sea ice during the 2007 – 2008 Canadian International Polar Year program. 115, 115–133. doi: 10.1007/s10584-012-0477-6
Barber, D., Leitch, D., Asplin, M. G., Gratton, Y., Lukovich, J. V., and Galley, R. J. (2010). The International Polar Year (IPY) Circumpolar Flaw Lead (CFL) system study: overview and the physical system. Atmos. Ocean 48, 225–243. doi: 10.3137/OC317.2010
Barberán, A., Bates, S. T., Casamayor, E. O., and Fierer, N. (2012). Using network analysis to explore co-occurrence patterns in soil microbial communities. ISME J. 6, 343–351. doi: 10.1038/ismej.2011.119
Bergeron, M., and Tremblay, J.-É. (2014). Shifts in biological productivity inferred from nutrient drawdown in the southern Beaufort Sea (2003–2011) and northern Baffin Bay (1997–2011), Canadian Arctic. Geophys. Res. Lett. 41, 3979–3987. doi: 10.1002/2014GL059649
Blazewicz, S. J., Barnard, R. L., Daly, R. A., and Firestone, M. K. (2013). Evaluating rRNA as an indicator of microbial activity in environmental communities: limitations and uses. ISME J. 7, 2061–2068. doi: 10.1038/ismej.2013.102
Boere, A. C., Rijpstra, W. I., De Lange, G. J., Damste, J. S., Coolen, M. J., Burg, D., et al. (2011). Preservation potential of ancient plankton DNA in pleistocene marine sediments. Geobiology 9, 377–393. doi: 10.1111/j.1472-4669.2011.00290.x
Bravo, I., and Figueroa, R. (2014). Towards an ecological understanding of dinoflagellate cyst functions. Microorganisms 2, 11–32. doi: 10.3390/microorganisms2010011
Brocks, J. J., and Banfield, J. (2009). Unravelling ancient microbial history with community proteogenomics and lipid geochemistry. Nat. Rev. Microbiol. 7, 601–609. doi: 10.1038/nrmicro2167
Campbell, B. J., and Kirchman, D. L. (2013). Bacterial diversity, community structure and potential growth rates along an estuarine salinity gradient. ISME J. 7, 210–220. doi: 10.1038/ismej.2012.93
Caporaso, J. G., Bittinger, K., Bushman, F. D., Desantis, T. Z., Andersen, G. L., and Knight, R. (2010a). PyNAST: a flexible tool for aligning sequences to a template alignment. Bioinformatics 26, 266–267. doi: 10.1093/bioinformatics/btp636
Caporaso, J. G., Kuczynski, J., Stombaugh, J., Bittinger, K., Bushman, F. D., Costello, E. K., et al. (2010b). Correspondence QIIME allows analysis of high- throughput community sequencing data intensity normalization improves color calling in SOLiD sequencing. Nat. Methods 7, 335–336. doi: 10.1038/nmeth.f.303
Carmack, E. C., and Macdonald, R. W. (2002). Oceanography of the Canadian shelf of the Beaufort Sea : a setting for marine life. Arctic 55, 29–45. doi: 10.14430/arctic733
Chao, A., and Shen, T. (2003). Non-parametric estimation of Shannon's index of diversity when there are unseen species in sample. Environ. Ecol. Stat. 10, 429–443. doi: 10.1023/A:1026096204727
Charvet, S., Vincent, W. F., Comeau, A., and Lovejoy, C. (2012). Pyrosequencing analysis of the protist communities in a High Arctic meromictic lake: DNA preservation and change. Front. Microbiol. 3:422. doi: 10.3389/fmicb.2012.00422
Chen, J., Bittinger, K., Charlson, E. S., Hoffmann, C., Lewis, J., Wu, G. D., et al. (2012). Associating microbiome composition with environmental covariates using generalized UniFrac distances. Bioinformatics 28, 2106–2113. doi: 10.1093/bioinformatics/bts342
Comeau, A. M., Li, W. K., Tremblay, J. É., Carmack, E. C., and Lovejoy, C. (2011). Arctic Ocean microbial community structure before and after the 2007 record sea ice minimum. PLoS ONE 6:e27492. doi: 10.1371/journal.pone.0027492
Comeau, A. M., Philippe, B., Thaler, M., Gosselin, M., Poulin, M., and Lovejoy, C. (2013). Protists in arctic drift and land-fast sea ice. J. Phycol. 49, 229–240. doi: 10.111/jpy.12026
Coupel, P., Jin, H. Y., Joo, M., Horner, R., Bouvet, H. A., Garçon, V., et al. (2012). Phytoplankton distribution in unusually low sea ice cover over the Pacific Arctic. Biogeosci. Discuss. 9, 2055–2093. doi: 10.5194/bgd-9-2055-2012
Coupel, P., Ruiz-Pino, D., Sicre, M. A., Chen, J. F., Lee, S. H., Schiffrine, N., et al. (2014). The impact of freshening on phytoplankton production in the Pacific Arctic Ocean. Prog. Oceanog. 131, 113–125. doi: 10.1016/j.pocean.2014.12.003
Csárdi, G., and Nepusz, T. (2006). The igraph software package for complex network research. Inter J Complex Syst. 1695.
D'Alelio, D., Libralato, S., Wyatt, T., and Ribera d'Alcalá, M. (2016). Ecological-network models link diversity, structure and function in the plankton food-web. Sci. Rep. 6:21806. doi: 10.1038/srep21806
Dasilva, C. R., Li, W. K. W., and Lovejoy, C. (2014). Phylogenetic diversity of eukaryotic marine microbial plankton on the Scotian Shelf, Northwestern Atlantic Ocean. J. Plankton Res. 36, 344–363. doi: 10.1093/plankt/fbt123
Dixon, P., and Dixon, P. (2003). VEGAN, a package of R functions for community ecology. J. Veg. Sci. 14, 927–930. doi: 10.1111/j.1654-1103.2003.tb02228.x
Dormann, C. F., Gruber, B., and Fründ, J. (2008). Introducing the bipartite package: analysing ecological networks. R News 8, 8–11.
Dunthorn, M., Otto, J., Berger, S. A., Stamatakis, A., Consortium, B., Stock, A., et al. (2014). Placing environmental next-generation sequencing amplicons from microbial eukaryotes into a phylogenetic context. Mol. Biol. Evol. 31, 993–1009. doi: 10.1093/molbev/msu055
Edgar, R. C. (2010). Search and clustering orders of magnitude faster than BLAST. Bioinformatics 26, 2460–2461. doi: 10.1093/bioinformatics/btq461
Edgar, R. C., Haas, B. J., Clemente, J. C., Quince, C., and Knight, R. (2011). UCHIME improves sensitivity and speed of chimera detection. Bioinformatics 27, 2194–2200. doi: 10.1093/bioinformatics/btr381
Esteban, G. F., Fenchel, T., and Finlay, B. J. (2010). Mixotrophy in ciliates. Protist 161, 621–641. doi: 10.1016/j.protis.2010.08.002
Fierer, N., Leff, J. W., Adams, B. J., Nielsen, U. N., Bates, S. T., Lauber, C. L., et al. (2012). Cross-biome metagenomic analyses of soil microbial communities and their functional attributes. Proc. Natl. Acad. Sci. U.S.A. 109, 21390–21395. doi: 10.1073/pnas.1215210110
Forest, A., Bélanger, S., Sampei, M., Sasaki, H., Lalande, C., and Fortier, L. (2010). Three-year assessment of particulate organic carbon fluxes in Amundsen Gulf (Beaufort Sea): satellite observations and sediment trap measurements. Deep. Res. Part I Oceanogr. Res. Pap. 57, 125–142. doi: 10.1016/j.dsr.2009.10.002
Forest, A., Tremblay, J.-É., Gratton, Y., Martin, J., Gagnon, J., Darnis, G., et al. (2011). Biogenic carbon flows through the planktonic food web of the Amundsen Gulf (Arctic Ocean): a synthesis of field measurements and inverse modeling analyses. Prog. Oceanogr. 91, 410–436. doi: 10.1016/j.pocean.2011.05.002
Garneau, M.-È., Vincent, W. F., Alonso-sáez, L., Gratton, Y., and Lovejoy, C. (2006). Prokaryotic community structure and heterotrophic production in a river-influenced coastal Arctic ecosystem Aquat. Microb. Ecol. 42, 27–40. doi: 10.3354/ame042027
Godhantaraman, N., and Uye, S. (2003). Geographical and seasonal variations in taxonomic composition, abundance and biomass of microzooplankton across a brackish-water lagoonal system of Japan. J. Plankton Res. 25, 465–482. doi: 10.1093/plankt/25.5.465
Godhe, A., Asplund, M. E., Härnström, K., Saravanan, V., Tyagi, A., and Karunasagar, I. (2008). Quantification of diatom and dinoflagellate biomasses in coastal marine seawater samples by real-time PCR. Appl. Environ. Microbiol. 74, 7174–7182. doi: 10.1128/AEM.01298-08
Guiry, M. D., and Guiry, G. M. (2015). AlgaeBase. World-wide electronic publication, National University of Ireland, Galway. Available online at: http://www.algaebase.org (Accessed 11 July, 2015).
Hall, T. (1999). BioEdit: a user-friendly biological sequence alignment program for Windows 95/98/NT. Nucleic Acids Symp. Ser. 41, 95–98.
Hamilton, A. K., Lovejoy, C., Galand, P. E., Gk, C., and Ingram, R. G. (2008). Water masses and biogeography of picoeukaryote assemblages in a cold hydrographically complex system. Limnol. Oceanogr. 53, 922–935. doi: 10.4319/lo.2008.53.3.0922
Hammer, Ø., Harper, D. A. T., and Ryan, P. D. (2001). PAST : Paleontological statistics software package for education and data analysis. Paleontol. Electron. 4, 1–9. Available online at: http://palaeo-electronica.org/2001_1/past/issue1_01.htm
Hansen, B. W., Nielsen, T. G., and Levinsen, H. (1999). Plankton community structure and carbon cycling on the western coast of Greenland during the stratified summer situation. III. Mesozooplankton. Aquat. Microb. Ecol. 16, 233–249. doi: 10.3354/ame016233
Hansen, P. J. (2011). The role of photosynthesis and food uptake for the growth of marine mixotrophic dinoflagellates. J. Eukaryot. Microbiol. 58, 203–214. doi: 10.1111/j.1550-7408.2011.00537.x
Heiskanen, A. (1993). Mass encystment and sinking of dinoflagellates during a spring bloom. Mar. Biol. 116, 161–167. doi: 10.1007/BF00350743
Hunt, D. E., Lin, Y., Church, M. J., Karl, D. M., Tringe, S. G., Izzo, L. K., et al. (2013). Relationship between abundance and specific activity of bacterioplankton in open ocean surface waters. Appl. Environ. Microbiol. 79, 177–184. doi: 10.1128/AEM.02155-12
Irigoien, X. (2005). Phytoplankton blooms: a “loophole” in microzooplankton grazing impact? J. Plankton Res. 27, 313–321. doi: 10.1093/plankt/fbi011
Jacobson, D. M., and Anderson, D. M. (1986). Thecate heterotrophic dinoflagellates: feeding behavior and mechanisms. J. Phycol. 22, 249–258. doi: 10.1111/j.1529-8817.1986.tb00021.x
Jacobson, D. M., and Anderson, D. M. (1996). Widespread phagocytosis of ciliates and other protists by marine mixotrophic and heterotrophic thecate dinoflagellates. J. Phycol. 32, 279–285. doi: 10.1111/j.0022-3646.1996.00279.x
Jiang, Y., Yang, E. J., Min, J. O., Kang, S. H., and Lee, S. H. (2013). Using pelagic ciliated microzooplankton communities as an indicator for monitoring environmental condition under impact of summer sea-ice reduction in Western Arctic Ocean. Ecol. Indic. 34, 380–390. doi: 10.1016/j.ecolind.2013.05.026
Johnson, M. D. (2011). Acquired phototrophy in ciliates: a review of cellular interactions and structural adaptations. J. Eukaryot. Microbiol. 58, 185–195. doi: 10.1111/j.1550-7408.2011.00545.x
Joli, N., Logares, R., Monier, A., and Lovejoy, C. (accepted). Seasonal patterns in Arctic prasinophytes inferred ecology of Bathycoccus unveiled in an Arctic winter metagenome. ISME J.
Jones, S. E., and Lennon, J. T. (2010). Dormancy contributes to the maintenance of microbial diversity. Proc. Natl. Acad. Sci. U.S.A. 107, 5881–5886. doi: 10.1073/pnas.0912765107
Krishfield, R. A., Proshutinsky, A., Tateyama, K., Williiams, W., Carmack, E. C., McLaughlin, F., et al. (2014). Deterioration of perennial sea ice in the Beaufort Gyre from 2003 to 2012 and its impact on the oceanic freshwater cycle R. J. Geophys. Res. Ocean 119, 1271–1305. doi: 10.1002/2013JC008999
Landry, M. R., and Calbet, A. (2004). Microzooplankton production in the oceans. ICES J. Mar. Sci. 61, 501–507. doi: 10.1016/j.icesjms.2004.03.011
Levinsen, H., and Nielsen, T. G. (2002). The trophic role of marine pelagic ciliates and heterotrophic dinoflagellates in arctic and temperate coastal ecosystems: a cross-latitude comparison. Limnol. Oceanogr. 47, 427–439. doi: 10.4319/lo.2002.47.2.0427
Levinsen, H., Nielsen, T. G., and Hansen, B. W. (2000). Annual succession of marine pelagic protozoans in Disko Bay, West Greenland, with emphasis on winter dynamics. Mar. Ecol. Prog. Ser. 206, 119–134. doi: 10.3354/meps206119
Lewandowska, A. M., Boyce, D. G., Hofmann, M., Matthiessen, B., Sommer, U., and Worm, B. (2014). Effects of sea surface warming on marine plankton. Ecol. Lett. 17, 614–623. doi: 10.1111/ele.12265
Li, W. K., McLaughlin, F. A., Lovejoy, C., and Carmack, E. C. (2009). Smallest algae thrive as the Arctic Ocean freshens. Science 326, 539. doi: 10.1126/science.1179798
Logares, R., Shalchian-Tabrizi, K., Boltovskoy, A., and Rengefors, K. (2007). Extensive dinoflagellate phylogenies indicate infrequent marine-freshwater transitions. Mol. Phylogenet. Evol. 45, 887–903. doi: 10.1016/j.ympev.2007.08.005
Lovejoy, C., and Potvin, M. (2011). Microbial eukaryotic distribution in a dynamic Beaufort Sea and the Arctic Ocean. J. Plankton Res. 33, 431–444. doi: 10.1093/plankt/fbq124
Lovejoy, C., Comeau, A., and Thaler, M. (2016). Curated reference database of SSU rRNA for northern marine and freshwater communities of archaea, bacteria and microbial eukaryotes, v. 1.1. Nordicana D23. doi: 10.5885/45409XD-79A199B76BCC4110
Lovejoy, C., Legendre, L., and Price, N. (2002). Prolonged diatom blooms and microbial food web dynamics: experimental results from an Arctic polynya. Aquat. Microb. Ecol. 29, 267–278. doi: 10.3354/ame029267
Lovejoy, C., Massana, R., and Pedro, C. (2007). Diversity and distribution of marine microbial eukaryotes in the Arctic Ocean and adjacent seas. Appl. Environ. Microbiol. 72, 3085–3095. doi: 10.1128/AEM.72.5.3085-3095.2006
Lovejoy, C., Vincent, W. F., Frenette, J., and Dodson, J. (1993). Microbial gradients in a turbid estuary: application of a new method for protozoan community analysis. Limnol. Oceanogr. 38, 1295–1303. doi: 10.4319/lo.1993.38.6.1295
Lozupone, C., and Knight, R. (2005). UniFrac: a new phylogenetic method for comparing microbial communities. Appl. Environ. Microbiol. 71, 8228–8235. doi: 10.1128/AEM.71.12.8228-8235.2005
Luddington, I. A., Lovejoy, C., and Kaczmarska, I. (2016). Species-rich meta-communities of the diatom order Thalassiosirales in the Arctic and northern Atlantic Ocean. J. Plankt. Res. 38, 781–797. doi: 10.1093/plankt/fbw030
Lynn, D. H. (2012). Ciliophora. eLS 1–12. Wiley on Line Library. doi: 10.1002/9780470015902.a0001966.pub3
Marquardt, M., Vader, A., Stubner, E., Reigstad, M., and Gabrielsen, T. M. (2016). Strong seasonality of marine microbial eukaryotes in a high-Arctic fjord (Isfjorden, West Spitsbergen). Appl. Environ. Microbiol. 82, 1866–1880. doi: 10.1128/aem.03208-15
Martin, J., Dumont, D., and Tremblay, J.-É. (2013). Contribution of subsurface chlorophyll maxima to primary production in the coastal Beaufort Sea (Canadian Arctic): a model assessment. J. Geophys. Res. Ocean. 118, 5873–5886. doi: 10.1002/2013JC008843
Martin, J., Tremblay, J., Gagnon, J., Tremblay, G., Lapoussière, A., Jose, C., et al. (2010). Prevalence, structure and properties of subsurface chlorophyll maxima in Canadian Arctic waters. Mar. Ecol. Prog. Ser. 412, 69–84. doi: 10.3354/meps08666
McLaughlin, F. A., and Carmack, E. C. (2010). Deepening of the nutricline and chlorophyll maximum in the Canada Basin interior, 2003-2009. Geophys. Res. Lett. 37:L24602. doi: 10.1029/2010GL045459
McManus, G. B., and Katz, L. A. (2009). Molecular and morphological methods for identifying plankton: what makes a successful marriage? J. Plankton Res. 31, 1119–1129. doi: 10.1093/plankt/fbp061
Medinger, R., Nolte, V., Pandey, R. V., and Jost, S. (2010). Diversity in a hidden world : potential and limitation of next- generation sequencing for surveys of molecular diversity of eukaryotic microorganisms. Mol. Ecol. 19, 32–40. doi: 10.1111/j.1365-294X.2009.04478.x
Møller, E. F., Nielsen, T. G., and Richardson, K. (2006). The zooplankton community in the Greenland Sea: composition and role in carbon turnover. Deep. Res. I 53, 76–93. doi: 10.1016/j.dsr.2005.09.007
Monier, A., Comte, J., Babin, M., Forest, A., Matsuoka, A., and Lovejoy, C. (2015). Oceanographic structure drives the assembly processes of microbial eukaryotic communities. ISME J. 9, 990–1002. doi: 10.1038/ismej.2014.197
Monier, A., Terrado, R., Thaler, M., Comeau, A., Medrinal, E., and Lovejoy, C. (2013). Upper Arctic Ocean water masses harbor distinct communities of heterotrophic flagellates. Biogeosciences 10, 4273–4286. doi: 10.5194/bg-10-4273-2013
Montagnes, D. J. S. (1996). Growth responses of planktonic ciliates in the genera Strobilidium and Strombidium. Mar. Ecol. Prog. Ser. 130, 241–254. doi: 10.3354/meps130241
Montagnes, D. J. S., Allen, J., Brown, L., Bulit, C., Davidson, R., Fielding, S., et al. (2010). Role of ciliates and other microzooplankton in the Irminger Sea (NW Atlantic Ocean). Mar. Ecol. Prog. Ser. 411, 101–115. doi: 10.3354/meps08646
Nelson, R. J., Ashjian, C. J., Bluhm, B. A., Conlan, K. E., Gradinger, R. R., Grebmeier, J. M., et al. (2014). “Biodiversity and biogeography of the lower trophic taxa of the Pacific Arctic Region : sensitivities to climate change,” in The Pacific Arctic Region: Ecosystem Status and Trends in a Rapidly Changing Environment, eds J. M. Grebmeier and W. Maslowski (Dordrecht: Springer), 269–336.
Nielsen, K. M., Johnsen, P. J., Bensasson, D., and Daffonchio, D. (2007). Thematic issue on horizontal gene transfer release and persistence of extracellular DNA. Environ. Biosafety Res. 6, 37–53. doi: 10.1051/ebr:2007031
Nielsen, L. T., and Kiørboe, T. (2015). Feeding currents facilitate a mixotrophic way of life. ISME J. 9, 2117–2127. doi: 10.1038/ismej.2015.27
O'Connor, M. I., Piehler, M. F., Leech, D. M., Anton, A., and Bruno, J. F. (2009). Warming and resource availability shift food web structure and metabolism. PLoS Biol. 7:e1000178. doi: 10.1371/journal.pbio.1000178
Okolodkov, Y. B., and Dodgeb, J. D. (1996). Biodiversity and biogeography of planktonic dinoflagellates in the Arctic Ocean. J. Exp. Mar. Bio. Ecol. 202, 19–27. doi: 10.1016/0022-0981(96)00028-7
Paranjape, M. A. (1990). Microzooplankton herbivory on the Grand Bank (Newfoundland, Canada): a seasonal study. Mar. Biol. 328, 321–328. doi: 10.1007/BF01319832
Park, M. G., and Kim, M. (2010). Prey specificity and feeding of the thecate mixotrophic dinoflagellate Fragilidium duplocampaforme. J. Phycol. 46, 424–432. doi: 10.1111/j.1529-8817.2010.00824.x
Paulic, J., Bartzen, B., Bennett, R., Conlan, K., Hardwood, L., Howland, K., et al. (2012). Ecosystem Overview Report for the Darnley Bay Area of Interest (AOI). DFO Canadian Science Advisory Secretariat Research Document 2011/062, 1–63.
Potvin, É., Rochon, A., and Lovejoy, C. (2013). Cyst-theca relationship of the arctic dinoflagellate cyst Islandinium minutum (Dinophyceae) and phylogenetic position based on SSU rDNA and LSU rDNA. J. Phycol. 49, 848–866. doi: 10.1111/jpy.12089
Price, M. N., Dehal, P. S., and Arkin, A. P. (2010). FastTree 2 – approximately maximum-likelihood trees for large alignments. PLoS ONE 5:e9490. doi: 10.1371/journal.pone.0009490
Pruesse, E., Quast, C., Knittel, K., Fuchs, B. M., Ludwig, W., Peplies, J., et al. (2007). SILVA: a comprehensive online resource for quality checked and aligned ribosomal RNA sequence data compatible with ARB. Nucleic Acids Res. 35, 7188–7196. doi: 10.1093/nar/gkm864
R Development Core Team (2008). R: A Language and Environment for Statistical Computing. Vienna: R Foundation for Statistical Computing.
Ramette, A. (2007). Multivariate analyses in microbial ecology. FEMS Microbiol. Ecol. 62, 142–160. doi: 10.1111/j.1574-6941.2007.00375.x
Reeder, J., and Knight, R. (2010). Rapidly denoising pyrosequencing amplicon reads by exploiting rank-abundance distributions. Nat. Methods 7, 668–669. doi: 10.1038/nmeth0910-668b
Riebesell, U., Gattuso, J. P., Thingstad, T. F., and Middelburg, J. J. (2013). Arctic ocean acidification: pelagic ecosystem and biogeochemical responses during a mesocosm study. Biogeosciences 10, 5619–5626. doi: 10.5194/bg-10-5619-2013
Riisgaard, K., Swalethorp, R., Kjellerup, S., Juul-Pedersen, T., and Nielsen, T. G. (2014). Trophic role and top-down control of a subarctic protozooplankton community. Mar. Ecol. Prog. Ser. 500, 67–82. doi: 10.3354/meps10706
Saldarriaga, J. F., Taylor, F. J. R., Cavalier-Smith, T., Menden-Deuer, S., and Keeling, P. J. (2004). Molecular data and the evolutionary history of dinoflagellates. Eur. J. Protistol. 40, 85–111. doi: 10.1016/j.ejop.2003.11.003
Saldarriaga, J. F., Taylor, F. J., Keeling, P. J., and Cavalier-Smith, T. (2001). Dinoflagellate nuclear SSU rRNA phylogeny suggests multiple plastid losses and replacements. J. Mol. Evol. 53, 204–213. doi: 10.1007/s002390010210
Schloss, P. D., Westcott, S. L., Ryabin, T., Hall, J. R., Hartmann, M., Hollister, E. B., et al. (2009). Introducing mothur: open-source, platform-independent, community-supported software for describing and comparing microbial communities. Appl. Environ. Microbiol. 75, 7537–7541. doi: 10.1128/AEM.01541-09
Seuthe, L., Darnis, G., Wexels, C., Wassmann, P., and Fortier, L. (2007). Winter – spring feeding and metabolism of Arctic copepods: insights from faecal pellet production and respiration measurements in the southeastern Beaufort Sea. Polar Biol. 30, 427–436. doi: 10.1007/s00300-006-0199-1
Seuthe, L., Iversen, K. R., and Narcy, F. (2011). Microbial processes in a high-latitude fjord (Kongsfjorden, Svalbard): II. Ciliates and dinoflagellates. Polar Biol. 34, 751–766. doi: 10.1007/s00300-010-0930-9
Shannon, P., Markiel, A., Ozier, O., Baliga, N. S., Wang, J. T., Ramage, D., et al. (2003). Cytoscape : A software environment for integrated models of biomolecular interaction networks. Genome Res. 13, 2498–2504. doi: 10.1101/gr.1239303
Sherr, E. B., and Sherr, B. F. (2009). Capacity of herbivorous protists to control initiation and development of mass phytoplankton blooms. Aquat. Microb. Ecol. 57, 253–262. doi: 10.3354/ame01358
Sherr, E. B., Sherr, B. F., and Ross, C. (2013). Microzooplankton grazing impact in the Bering Sea during spring sea ice conditions. Deep. Res. II 94, 57–67. doi: 10.1016/j.dsr2.2013.03.019
Skjoldborg, A., Gissel, T., Levinsen, H., Madsen, S. D., Thingstad, T. F., and Winding, B. (2003). Impact of changing ice cover on pelagic productivity and food web structure in Disko Bay, West Greenland: a dynamic model approach. Deep. Res. Part I 50, 171–187. doi: 10.1016/S0967-0637(02)00133-4
Søreide, J. E., Leu, E., Berge, J., Graeve, M., and Falk-Petersen, S. (2010). Timing of blooms, algal food quality and Calanus glacialis reproduction and growth in a changing Arctic. Glob. Chang. Biol. 16, 3154–3163. doi: 10.1111/j.1365-2486.2010.02175.x
Stamatakis, A. (2014). RAxML version 8 : a tool for phylogenetic analysis and post-analysis of large phylogenies. Bioinformatics 30, 1312–1313. doi: 10.1093/bioinformatics/btu033
Stefels, J. (2000). Physiological aspects of the production and conversion of DMSP in marine algae and higher plants. J. Sea Res. 43, 183–197. doi: 10.1016/S1385-1101(00)00030-7
Stoecker, D. K., Johnson, M. D., De Vargas, C., and Not, F. (2009). Acquired phototrophy in aquatic protists. Aquat. Microb. Ecol. 57, 279–310. doi: 10.3354/ame01340
Stoecker, D. K., Taniguchi, A., and Michaels, A. E. (1988). Abundance of autotrophic, mixotrophic and heterotrophic planktonic ciliates in shelf and slope waters. Mar. Ecol. Prog. Ser. 50, 241–254.
Terrado, R., Medrinal, E., Dasilva, C., Thaler, M., Vincent, W. F., and Lovejoy, C. (2011). Protist community composition during spring in an Arctic flaw lead polynya. Polar Biol. 34, 1901–1914. doi: 10.1007/s00300-011-1039-5
Terrado, R., Vincent, W., and Lovejoy, C. (2009). Mesopelagic protists: diversity and succession in a coastal Arctic ecosystem. Aquat. Microb. Ecol. 56, 25–39. doi: 10.3354/ame01327
Thaler, M., and Lovejoy, C. (2015). Biogeography of heterotrophic flagellate populations indicates the presence of generalist and specialist taxa in the Arctic Ocean. Appl. Envron. Microbiol. 81, 2137–2148. doi: 10.1128/aem.02737-14
Thoisen, C., Riisgaard, K., Lundholm, N., and Nielsen, T. G. (2015). Effect of acidification on an Arctic phytoplankton community from Disko Bay, West Greenland. Mar. Ecol. Prog. Ser. 520, 21–34. doi: 10.3354/meps11123
Torti, A., Lever, M. A., and Jørgensen, B. B. (2015). Origin, dynamics, and implications of extracellular DNA pools in marine sediments. Mar. Genomics 24, 185–196. doi: 10.1016/j.margen.2015.08.007
Tremblay, J.-É., Bélanger, S., Barber, D. G., Asplin, M., Martin, J., Darnis, G., et al. (2011). Climate forcing multiplies biological productivity in the coastal Arctic Ocean. Geophys. Res. Lett. 38:L18604. doi: 10.1029/2011gl048825
Verni, F., and Rosati, G. (2011). Resting cysts: a survival strategy in protozoa Ciliophora. Ital. J. Zool. 78, 134–145. doi: 10.1080/11250003.2011.560579
Yamamoto-Kawai, M., McLaughlin, F. A., Carmack, E. C., Nishino, S., and Shimada, K. (2008). Freshwater budget of the Canada Basin, Arctic Ocean, from salinity, δ18O, and nutrients. J. Geophys. Res. 113:C01007. doi: 10.1029/2006JC003858
Keywords: microzooplankon, 18S rRNA pyrosequencing, interannual variability, indicator species, Arctic ice loss
Citation: Onda DFL, Medrinal E, Comeau AM, Thaler M, Babin M and Lovejoy C (2017) Seasonal and Interannual Changes in Ciliate and Dinoflagellate Species Assemblages in the Arctic Ocean (Amundsen Gulf, Beaufort Sea, Canada). Front. Mar. Sci. 4:16. doi: 10.3389/fmars.2017.00016
Received: 12 July 2016; Accepted: 11 January 2017;
Published: 07 February 2017.
Edited by:
George S. Bullerjahn, Bowling Green State University, USAReviewed by:
Rebecca Gast, Woods Hole Oceanographic Institution, USAAlison Clare Cleary, Independent Researcher, San Francisco, United States
Copyright © 2017 Onda, Medrinal, Comeau, Thaler, Babin and Lovejoy. This is an open-access article distributed under the terms of the Creative Commons Attribution License (CC BY). The use, distribution or reproduction in other forums is permitted, provided the original author(s) or licensor are credited and that the original publication in this journal is cited, in accordance with accepted academic practice. No use, distribution or reproduction is permitted which does not comply with these terms.
*Correspondence: Connie Lovejoy, Connie.Lovejoy@bio.ulaval.ca
†Present Address: André M. Comeau, Centre for Comparative Genomics and Evolutionary Bioinformatics-Integrated Microbiome Resource, Department of Pharmacology, Dalhousie University, Canada