- 1UR MaNE, Faculté des Sciences de Bizerte, Université de Carthage, Zarzouna, Tunisia
- 2Marine and Environmental Sciences Centre (MARE), Faculty of Sciences, University of Lisbon, Lisbon, Portugal
- 3Department of Chemistry, UCIBIO-REQUIMTE, Faculty of Sciences and Technology, Universidade Nova de Lisboa, Caparica, Portugal
- 4Department of Plant Biology, Faculty of Sciences, University of Lisbon, Lisbon, Portugal
Information on localization of Al and As in Tamarix gallica is required in order to better understand the detoxification mechanisms that confer tolerance in this halophyte plant species. Plants were subjected to different Al and As concentrations with and without salt supplementation. High concentrations of As and Al have been found in Tamarix gallica leaves and roots without symptoms of toxicity to the plant, which may be related to the particular compartmentation. A sequential extraction was carried out on leaves and roots to determine and to compare the metal compartmentation in the plant. In this study, subcellular localization of As and Al was determined for the first time in roots and leaves of T. gallica, and provided evidence of the detoxification mechanisms of high As and Al concentrations. These results suggest that the subcellular distribution of As and Al play important roles in avoidance of metal toxicity. The most part of Al (that has high toxicity to the plant when available forms are present) was immobilized in cell wall, potentially suppressing its transportation to other subcellular compartments more susceptible to Al toxicity. On the other hand, the greater sequestration of As in the vacuole reduces its toxicity to the remaining cell organelles in the roots, but cell wall confinement remains an important tolerant mechanism in the leaves.
Introduction
Although metals are natural constituents of soils and occur naturally in the environment (Rascio and Navari-Izzo, 2011). Metal pollution is one of the most serious environmental problems aggravated by human activities as mining and smelting, electroplating, gas exhaust, energy, and fuel production, fertilizer, and pesticide application (Alkorta et al., 2004). In high concentrations, essential and non-essential elements like Cd, Zn, Hg, Pb, Ag, As, Al, among others, are strongly toxic (Upadhyaya et al., 2010). Trace metal elements exert different actions on plants, like blocking essential functional groups, displacing other metal ions, or altering the active conformation of biological molecules (Collins and Stotzky, 1989). In fact, Aluminum (Al) represents about 7% of the earth's solid surface weight. Al is not a free element (Bhalerao and Prabhu, 2013) and Al toxicity increases with soil acidity (Jeffrey et al., 1996). The trivalent cation Al3+ has been reported as inhibiting root growth and development. This could be due through Al interactions within symplast, plasma membrane, and cell wall (Kochian, 1995).
Arsenic (As) is one of the most toxic element and can be found in every environmental compartment. In soil, As-inorganic form, as oxidized arsenate (As5+) and reduced arsenite (As3+), are generally more toxic than As-organic form (Mateos-Naranjo et al., 2012). It has been known to cause growth inhibition and membrane disintegration (Moreno-Jiménez et al., 2012). Arsenic toxicity is induced by oxidative stress through generation of reactive oxygen species (ROS) and inhibition of antioxidant defenses (Mateos-Naranjo et al., 2012).
However, plants can regulate their metabolism in response to the trace metal element and protect themselves against their toxicity to a certain extent. Understanding plant-metal interactions may help to reduce the risks associated with the introduction of trace metals into the food chain and to address safety issues in the environment.
Studies have been conducted to improve the knowledge on the tolerance mechanism of plants facing elevated trace metals accumulation without major metabolic alterations (Revathi and Subhashree, 2013). Plants have developed highly complex systems to control the uptake, accumulation and detoxification of trace metals (Leitenmaier and Küpper, 2013). In general, these mechanisms range from exclusion, inclusion and accumulation (Raskin and Ensley, 2000). Possible mechanisms that govern metal tolerance in plant cells (Barceló and Poschenrieder, 1992; Sanità di Toppi and Gabbrielli, 1999) are selective exclusion of metal during uptake, metal excretion, metal retention in the roots, specific tolerance of enzymatic systems, immobilization by means of cell wall, and extracellular carbohydrates, complexation by binding low-molecular weight peptides (phytochelatins) or by ligands such as organic acids and amino acids, and finally by compartmentation (Hall, 2002; Carrier et al., 2003). Thus, trace metals may be stored/accumulated either in cell walls (Lozano-Rodriguez et al., 1997; Carrier et al., 2003), cytoplasm (Rauser and Ackerley, 1987; Carrier et al., 2003), or in cell vacuoles (Carrier et al., 2003). Tolerance may also be achieved in some metal-hyperaccumulating plants by root to shoot metal transport, maintaining low metal concentration in roots (Kramer et al., 1997).
Tamarix gallica L. is a halophyte from coastal and desert regions, living also in some saline depressions, which are usually used as sites of accumulation of industrial and urban effluents contaminated by metals (Ghnaya et al., 2007). In order to survive in these sites and according to their tolerance capacity, plants may have mechanisms to regulate internal, and cell wall metal concentrations by which determine their survival (Sousa et al., 2008). The genus Tamarix is a relatively long-living plant that can tolerate a wide range of environmental conditions, resisting abiotic stresses such as salt, high temperature, and drought stresses (Saïdana et al., 2008). Additionally, Tamarix spp. has also been studied as a model for morphological traits for adaptation to saline and contaminated environments (Moreno-Jiménez et al., 2009; Haiyan et al., 2011; Sghaier et al., 2015).
The mechanisms for internal detoxification of As and Al in T. gallica have not been well documented and little known about the localization and speciation of As and Al in this halophyte. To determine the localization and distribution of elements inside plants, different methods can be used, such as histochemical (Vázquez et al., 1992b; Seregin and Ivanov, 1997), cell fractionation (Lozano-Rodriguez et al., 1997), X-ray microanalysis (Khan et al., 1984; Vázquez et al., 1992a,b), particle-induced X-ray emission (micro-PIXE) (Ager et al., 2002), or nuclear micro-probe technique (NMP) (Ager et al., 2003). The aim of the present work is to determine the tissue and cellular localization of As and Al in roots and leaves of T. gallica by using cell fractionation. Knowledge of As and Al distribution in plants can help to clarify the process involved in the uptake, transport, and deposition or detoxification of these elements in T. gallica.
Materials and Methods
Plant Sampling and Experimental Setup
Young plants were obtained by cutting propagation, 5 cm length leafy stem were taken from mother plants, rinsed abundantly with distilled water, and placed for rooting in plastic pots (3 dm3) containing a mixture of perlite/gravel (in a 2:1 ratio) as a substrate. In these conditions, rooting occurred 1 month after planting. During a 6-week period of rooting, the cuttings were irrigated with non-saline tap water. After this period, plants were regularly irrigated with a nutrient solution (Hewitt, 1966) enriched with iron and micronutrients and supplemented or not with NaCl (200 mM). After this acclimation period, plants were divided into eight groups of three plants that were supplied for 3 months with aluminum chloride (AlCl3) and sodium arsenate (Na2HAsO4, 7H2O) which is a pentavalent form, As(V). Control plants were regularly irrigated with the same nutritive solution and the remaining groups were subjected to Hewitt solution added with, (a) Al or As 200 μM; (b) Al or As (500 μM; c) Al or As 800 μM; (d) Al or As 200 μM + NaCl 200 mM; (e) Al or As 500 μM + NaCl 200 mM; (f) Al or As 800 μM + NaCl 200 mM. After 4 months of the start of the experiments, plants were harvested and divided into shoots and roots and rinsed three times in cold distilled water and blotted with filter paper. In order to eliminate trace elements adsorbed at the root surface, these organs were dipped by hand in a cold solution of CaCl2 during 5 min (Stolt et al., 2003). Finally, the harvested shoots and roots were dried in the oven at 70°C for 7 days.
Metals Extraction Procedure
A sequential extraction was carried out in order to evaluate the metal content in the cellular components of T. gallica (Farago and Pitt, 1977). Different parts of oven dried (70°C for 7 days) plant materials (leaves and roots; 1 g DW; n = 3) were processed individually. The first extracting agent used was ethanol 80% (p.a., Merck, 10 ml) for 24 h; then, the residue was placed in 10 ml of bi-distillated water and subjected to shake for 24 h. In the following step, the residue was put in a solution of 100 ml ultra-pure water (pH 7.5; temperature 37°C) with 0.2 g pronase E (from Streptomyces griseus, Merck) added to 0.03 g chloramphenicol (P98%, TLC) and subjected to continuous shaking for 24 h. Later, the same residue was added to 10 ml of a pectinase solution (1% P5146, Sigma; pH 4, temperature 25°C) and shaken for 24 h. The fourth extraction step consisted of adding 10 ml NaOH solution (0.5 M) (p.a. P98%, Sigma) to the residue and shaking for 24 h, and after that, final continuous shaking with 100 ml HCl 5% (prepared from HCl 37% p.a., Merck) was performed for 12 h at 25°C. The final step consists of an acid digestion to the plant residue (the digestion was processed in Teflon bombs) with HNO3/HClO4 (7,1, v,v) (HNO3 65% p.a., Merck; HClO4 70% p.a. ACS-ISO, Panreac) and then dried into the oven at 110°C for 3 h. After cooling, all extracts/fractions (ethanolic, aqueous, proteic, pectic, polysaccharidic, ligninic, and cellulosic) were filtered and diluted with 10 ml of a 0.01 M HNO3 solution. By this method, the different types of proteins cannot be determined, which implies that its exact location in the cell will not be defined. Metals bound to the cell wall were thus designated by their constituents, which are pectic, polysaccharidic, ligninic, and cellulosic fractions (Figure 1). The metals bound to some amino acids, chlorophyll, low weight compounds (all extracted by ethanol) and those extracted in the aqueous fraction are considered as soluble metal fractions (Farago and Pitt, 1977).
Total elements present in each extracted fraction were determined by inductively coupled plasma atomic emission spectroscopy [ICP-AES; Horiba Jobin-Yvon, Horiba Jobin-Yvon, France, model Ultima].
Translocation Factor
The translocation factor (TF) was calculated by the ratio of [metal] leaves/[metal] roots, expressing the metal's translocation within the plant, from the roots to the leaves (Deng et al., 2004).
Statistical Analysis
The contents of As and Al in different subcellular fractions (cell wall, proteic, and soluble fractions) of T. gallica roots and leaves were expressed as micrograms of metal per gram of plant material on a dry weight basis. Experiments were repeated in triplicate for statistical analysis. A one-way analysis of variance (ANOVA) or when parametric test assumptions were not met, Kruskal-Wallis test were used to compare mean accumulation of As and Al in the different fractions. Depending on the type of test (parametric or non-parametric), Bonferroni test or pairwise multiple comparisons were performed when significant differences were found (α = 0.05 significance level). The data in the tables are presented as mean ± standard error of the mean (dry weight). Analysis was performed with SPSS v.22.0 for Windows.
Results
Roots presented significantly higher metal concentrations than the leaves, for all studied treatments (Table 1). Metal translocation from roots to leaves can be expressed by the translocation factor (TF), and varied from 0.192 ± 0.034 to 0.087 ± 0.010 in the case of Al, showing that the treatment with the lowest Al concentration had the highest TF. The opposite pattern was observed for As, with TF varying from 0.071 ± 0.012 to 0.188 ± 0.028, with the highest TF being found in the highest metal concentration supplied with salt (Table 2).
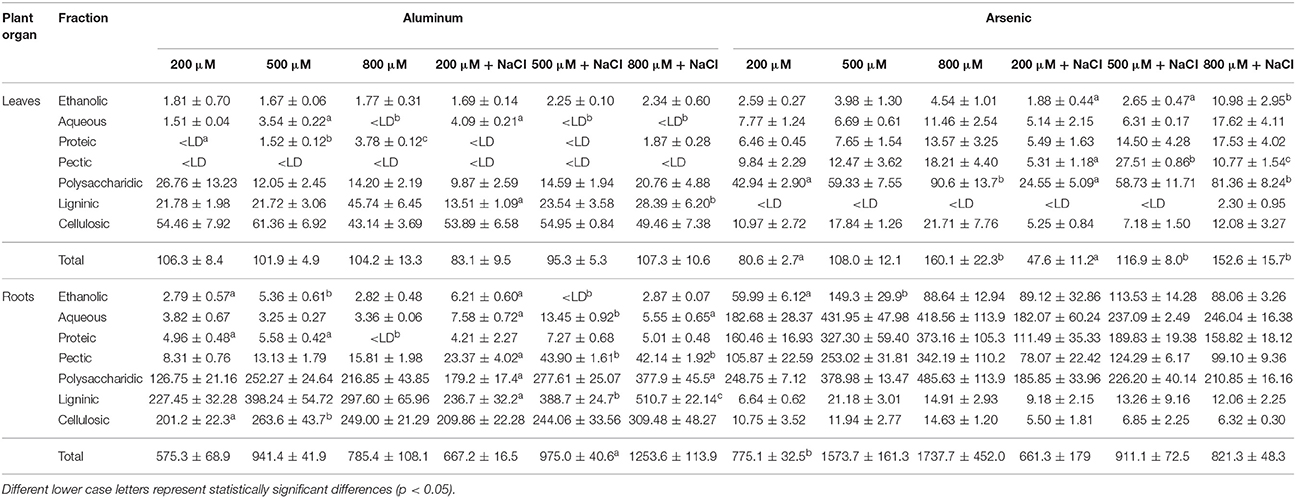
Table 1. Metal concentrations (μg g−1 DW) (average ± SE; n = 3) on different fractions of leaves and roots of Tamarix gallica.
Aluminum was predominantly bound to cellulose in the leaves (Figure 2A), ranging from 43.14 ± 3.69 μg.g−1 to 61.36 ± 6.92 μg.g−1 (Table 1). In the roots, Al was mainly bound to lignin (Figure 2B), with concentrations ranging from 227.45 ± 32.28 μg.g−1 to 510.66 ± 22.14 μg.g−1 (Table 1). Concerning As, higher concentration was found in the polysaccharidic fraction in the leaves (Figure 2C), ranging between 24.55 ± 5.09 μg. g−1 and 90.60 ± 13.75 μg.g−1 (Table 1); in the roots, the difference between fractions was less evident (Figure 2D), and similar concentrations were present in the polysaccharidic, the aqueous, and the proteic fractions (Table 1).
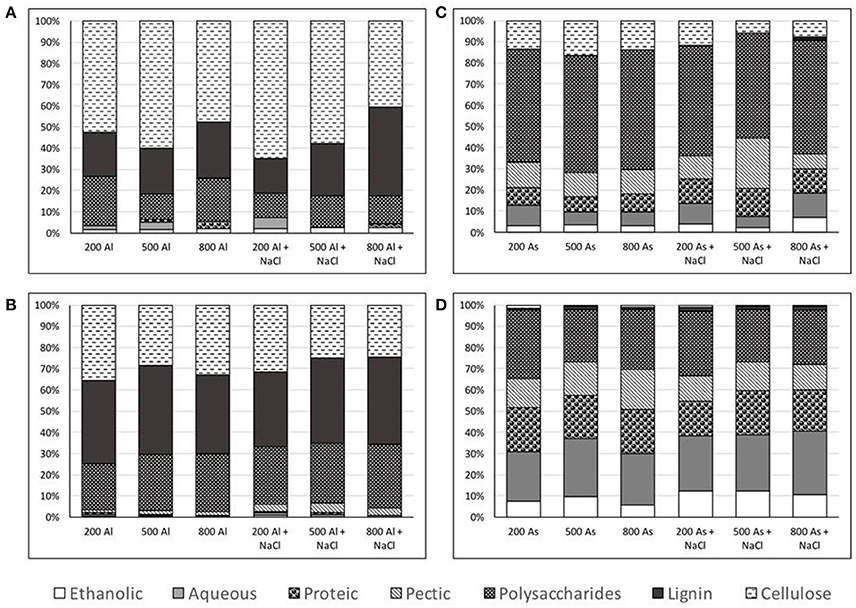
Figure 2. Metal concentrations (average %; n = 3) in different fractions of Tamarix gallica corresponding to Aluminum, leaves (A) and roots (B), and Arsenic, leaves (C) and roots (D). Regarding the fractions, from bottom to top (ethanolic, aqueous, proteic, pectic, polysaccharidic, ligninic, and cellulosic).
Considering the three fractions in which metal compartmentation can be grouped in this work (cell wall, proteic and intracellular/soluble fractions, Figure 3), 95.17 ± 1.1% of Al in the leaves was accumulated in the cell wall (with total absence in the pectic fraction) and with very low values in the soluble (3.66 ± 1.2%) and in the proteic fraction (1.17 ± 0.9%) (Figures 2A, 3A). The same predominance of Al in the cell wall components (98.3 ± 0.4%) was observed in the roots, with a residual presence of Al bound to proteins (0.5 ± 0.2%), and 1.2 ± 0.3% bound to soluble components (Figures 2B, 3B). Shoots accumulated on average 78.0 ± 2.8% of As into cell wall components (pectin, polysaccharides, lignin and cellulose), 9.9 ± 1.4% was retained in the proteic fraction and 12.1 ± 2.2% in the soluble fraction (Figure 3C). In the roots, 36.0 ± 2.6% of metal, on average, was retained inside the cell, 44.3 ± 2.3% in the cell wall, and the proteic fraction retained 19.7 ± 1.0% (Figure 3D).
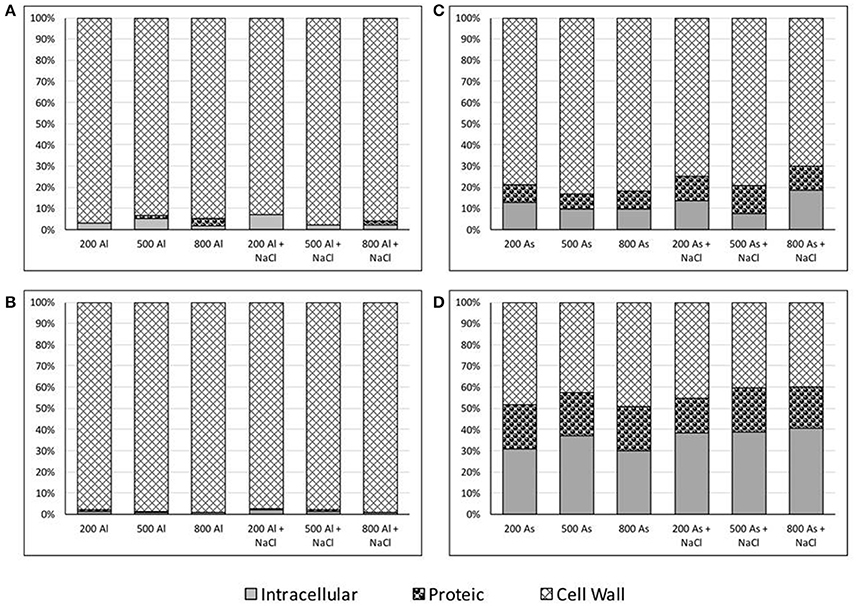
Figure 3. Metal concentration (average %; n = 3) located intracellularly (ethanolic + aqueous fraction), on the proteic fraction, and the cell wall (pectic + polysaccharidic + ligninic + cellulosic fractions) of Tamarix gallica. Metal concentrations corresponding to Aluminum in leaves (A) and roots (B), and Arsenic in leaves (C) and roots (D).
In the absence of salt, total concentration of Al in the leaves showed non-significant differences (p > 0.05) with increasing treatment concentrations (Table 1). There were, however, significant differences in Al compartmentation in the leaves, the intracellular / soluble fraction presented significant differences (F = 39.462, p < 0.001), showing a peak accumulation under the 500 μM Al treatment, and with the highest concentration (800 μM Al) resulting in the lowest accumulation in this fraction. Within the soluble fraction, the difference was particularly marked in the aqueous fraction (H = 7.448, p = 0.024), since Al was not detected there when the highest treatment concentration was applied. On the other hand, Al bound to proteins was not detected for the lowest concentration (200 μM Al), but increased with increasing treatment concentrations (F = 366.373, p < 0.001). The pectic fraction did not show any detectable concentration of Al in the leaves for any of the treatments. Unlike what was observed in the leaves, total Al concentration in the roots presented significant differences across treatments (F = 5.656, p = 0.046), with the highest accumulation being verified when 500 μM Al were supplied in the nutritive solution; a corresponding increase was observed in the Al bound to cell wall components (H = 6.489, p = 0.039). In the more detailed partitioning scheme (Figure 2B), it was possible to observe that when the highest concentration of Al was present in the nutritive solution (800 μM), no Al was detected bound to the proteic fraction (H = 6.161, p = 0.046); Al in the ethanolic fraction increased with 500 μM Al in solution, but decreased when the highest concentration was used (Table 1).
With the addition of 200 μM of NaCl, the response of total Al accumulation in the leaves was similar to that without salt, with no significant differences across treatments (p > 0.05). On a closer look, however, some differences were found compared to the “no salt” treatments, Al in the aqueous fraction was only detected in the lowest treatment concentration (200 μM), and the proteic fraction was only present when 800 μM Al was used in the experiment. Parallel to the experiment without NaCl, no Al was detected in the pectic fraction. There was also a displacement of Al toward lignin as more metal was present in the nutritive solution, with significant differences between the two extreme concentrations, 200 μM and 800 μM (F = 14.676, p = 0.005). In the roots, there was an increase in total Al accumulation with increasing treatment concentrations, but differences were only significant between extreme values (H = 6.489, p = 0.039). In the presence of salt there was generally a higher accumulation by T. gallica's roots (Table 1). Cell wall components accumulated more Al with the 800 μM treatment (H = 6.489, p = 0.039), particularly polysaccharides (F = 9.871, p = 0.013) and lignin (F = 26.429, p = 0.001); the pectic fraction showed a peak accumulation from the 200 μM to the 500 μM treatment, but remained stable when more metal was added to the solution (F = 28.1812, p = 0.001). Intracellular/soluble Al was more readily accumulated in the roots at lower concentrations in the presence of salt, but decreased with 800 μM Al in solution (F = 13.915, p = 0.006).
Total concentration of As in the leaves increased significantly with increasing treatment concentrations, both with and without NaCl added (Table 1). In the absence of salt, the difference was only significant between extreme concentrations (F = 7.520, p = 0.023), while with salt added to the nutritive solution the greatest increase was observed in the 500 μM treatment (F = 19.602, p = 0.002). In the absence of salt, the increase in total As corresponded to an increase in the cell wall components (F = 8.215, p = 0.019), specifically in the polysaccharidic fraction (F = 6.906, p = 0.028). With salt added to the treatments, there was also a significant increase in the accumulation of As in cell wall components (F = 14.377, p = 0.005), not only involving the polysaccharides as before (F = 10.623, p = 0.011), but also the pectic fraction (F = 89.366, p = 0.00003). Arsenic was only bound to lignin at the highest concentration of As. Increasing As concentration with NaCl has also promoted significant changes in the soluble fractions (F = 13.915, p = 0.006), with the 800 μM treatment resulting in greater As accumulation in the ethanol fraction (F = 8.370, p = 0.018). As for the roots of T. gallica, no significant differences were observed across treatments with the addition of salt in neither total As accumulation nor in its compartmentation.
Discussion
Translocation—the process by which metal ions taken up by roots are channeled to the shoots and to the intracellular organelles (Revathi and Subhashree, 2013) was rather low in the present study regarding all treatments under consideration. As and Al concentrations decreased markedly from roots to leaves, suggesting a retention of both elements in their long-distance translocation, which can be considered as the primary tolerance/defense mechanism of T. gallica. Although, As and Al concentrations in the aerial part increased with increasing metal supply, a lower translocation factor was recorded when higher concentrations of both elements were present, implying that As and Al translocations to the aerial part are restricted by internal barriers to defend the above ground part. Metal-tolerant plants accumulate higher concentration of toxic metals in roots and lower in shoots, contrary to hyperaccumulators (Lu et al., 2013). The root system is the dominant metal uptake route, with only small amounts being translocated to aboveground parts (Caçador et al., 2009). Arsenic is known to have low mobility regarding translocation from roots to shoots, except in hyperaccumulators. In wild-type Arabidopsis thaliana, 2.6% of As taken up by the roots was translocate to the shoots (Quaghebeur and Rengel, 2004), and several other studies reported an inefficient As translocation ratio (e.g., Marin et al., 1992; Carbonell-Barrachina et al., 1998; Burló et al., 1999; Pickering et al., 2000). Arsenic limited translocation may result from rapid reduction of arsenate, As(V), to arsenite, As(III), in the roots, followed by complexation with thiols, and possibly sequestration in the root vacuoles (Zhao et al., 2009). When the arsenate reductase AtACR2 in A. thaliana was silenced using RNAi, As accumulation in the shoots increased markedly (Dhankher et al., 2006). It was suggested that blocking AtACR2 would lead to more arsenate in the roots available for xylem transport to the shoots, presumably via the phosphate transport pathway. Metal uptake can also be inhibited by the formation of organic acids-metal complexes (Revathi and Subhashree, 2013), for example, citrate is known to inhibit Al uptake, working as an Al-tolerance mechanism in several plant species (De la Fuente et al., 1997; Papernik et al., 2001; Pineros and Kochian, 2001), and malic acid released from the radicle apex of wheat increases when Al is supplied (Delhaize and Ryan, 1995).
In general, metals are accumulated in the plant in different cell compartments, linked to different cell compounds (Reboreda and Caçador, 2007; Sousa et al., 2008). To reduce metal toxicity to the plant, they are preferentially driven away from metabolic active compartments (such as chloroplast and mitochondria) and are stored/sequestered in vacuoles and in the cell wall (Küpper et al., 2001; Psaras and Manetas, 2001; Sousa et al., 2008). To our knowledge, no other studies of As and Al localization in T. gallica have been undertaken. The present study was carried out to investigate the uptake and the distribution of As and Al in this species, after previous works suggested that T. gallica possesses great ability in tolerance and accumulation for both As and Al (Sghaier et al., 2015).
In T. gallica, As and Al were found to bind to cell wall compounds in different percentage, in roots and leaves. Arsenic presents chemical similarity with P, although its soil chemistry is more diverse, and binds readily with S and C (Wenzel, 2013), while Al has high affinity with oxygen donors, like carboxylate and phosphate (Chang et al., 1999) and organic acids like malate and citrate (Gupta et al., 2013).
Globally, Al compartmentation presented greater consistency between roots and leaves, with most metal bound to the cell wall (>95%) with either absence or presence of NaCl. It has been reported that the diminution of the metal concentration in the cytoplasm could be related to the metal accumulation in cell walls playing a role as a barrier against harmful effects by metals (Ramos et al., 2002; Zornoza et al., 2002). Aluminum forms such strong bounds to the cell wall that its amount will generally remain unaltered with the addition of other metallic cations (Krzesłowska, 2011). Arsenic partitioning resulted differently from what was observed with Al, most As in the leaves was found bound to the cell wall, but the importance of this location showed a slight decrease in the presence of salt. Arsenic compartmentation in the roots was more evenly distributed, with the proteic and soluble fractions containing more than 50% of total As together.
Aluminum is the third most abundant element and the most abundant metal in the earth's crust (Ma, 2005), but the most common forms in which occurs render Al generally unavailable to the plants (Delhaize and Ryan, 1995). However, when available, Al presents high toxicity to plants, namely though its soluble ionic form, Al3+ (Ma, 2007), promoting growth impairment, inhibition of root elongation, plasma membrane, and cell wall damages (Ma, 2005 and references therein), as a result of binding to intra- and/or extra-cellular compounds (Ma, 2000). Compartmentation of Al in cell walls is a very important mechanism responsible for Al detoxification, as it was observed in the major Al hyperaccumulator, Camellia sinensis (Gao et al., 2014), in Chara corallina (Tolra et al., 2011), and in cultured tobacco cells, where Al uptake and distribution showed that most Al (>90%) accumulated in the cell wall (Chang et al., 1999). The fact that the main percentage of metals was bound to the cell wall rather than located intracellular may have crucial significance as a detoxifying mechanism in T. gallica leaves and roots. Early works (Matsumoto et al., 1976) explained that Al localization in epidermal cell walls was linked to the movement of the water mass flow from the xylem to the epidermis, where the water evaporates into the air, and therefore Al is concentrated in the cell walls at the sites of evaporation. The limitation of translocation into shoots and metals binding to the cell wall are well known mechanisms for non hyperaccumulator plants (Wagner, 1993; Grant et al., 1998). However, the pattern of compartmentation seems to be species and metal-specific. For instance, heavy metals as Ni, Cd and Zn are generally stored in the vacuoles of epidermis cells (Küpper et al., 1999; Psaras et al., 2000). Aluminum forms strong bonds with oxygen donors, such as phosphates and carboxylates, and has been described as strongly binding to the carboxylate group of pectin in the cell wall (Chang et al., 1999). In the present work Al did not accumulate in the pectic fraction in the leaves, regardless of the treatment applied, and its accumulation in the roots was of minor expression (<3% of total Al, on average). Instead, cellulose was the preferential binding site in the leaves, and lignin, cellulose, and polysaccharide's fractions were the preferred binding locations in the roots. The binding of Al to cell wall pectin may not always be an essential tolerance mechanism (Krzesłowska, 2011). At high external concentrations, the proteic fraction acquired importance in the leaves, particularly in the absence of salt. With NaCl present in the nutritive solution, Al bound to the proteic fraction was only detected at the highest treatment tested. Tolerance mechanisms in plant cells include chelators such as phytochelatins to avoid metal stress into the cytoplasm; these polypeptides can bind metal cations and sequester them in the vacuole, allowing the cells to cope with higher external metal concentrations (Krzesłowska, 2011). At higher concentrations, the roots showed a displacement of Al toward lignin. The increase in cell wall lignification, and subsequent metal deposits therein, is another mechanism that has been described to protect plant cells from toxic effects of high concentrations (Probst et al., 2009).
A large proportion of As was bound to cell wall components in the leaves, which seems to function as the first barrier protecting plant from As toxicity. In lettuce leaves, 64% of total Cd was bound to cell walls (Ramos et al., 2002) and a similar proportion of Cd associated with the cell wall fraction was observed in Lupinus albus (Zornoza et al., 2002). Large portions of metals are accumulated in the cell wall-plasma membrane interfaces and thus it could be considered as the potential tolerance-related site (Hossain et al., 2012). The cell wall played an important role in retaining As in the leaves of T. gallica outside metabolic sites, with over 70% of the As in the root was retained there. It is possible that As forms strong binding to cell wall components like cellulose, pectin and lignin (Koch et al., 1999), which could immobilize excess As in T. gallica leaves. Besides the cell wall, the vacuole seems to be another site of preferential As binding in leaves. In our study, As analysis at the subcellular level of plant tissue demonstrated the importance of the soluble fraction. The vacuole in root cells is most likely a sink for As. Vacuolar compartmentation has been described as playing a very significant role in detoxification and tolerance, which prevents the circulation of free ions in the cytosol and forces them into a limited area (Sanità di Toppi and Gabbrielli, 1999). The proteic fraction has also showed great importance in the compartmentation of As in T. gallica roots. Transport to and storage in the vacuole requires increasingly sulfur-rich peptides, including phytochelatins and organic acids (Sanità di Toppi and Gabbrielli, 1999). Phytochelatins (PC) are synthesized from glutathione by PC-synthases, and play a role in the distribution and accumulation of As and some other highly toxic metals like Ag, Hg, and Cd (Rauser, 1999; Cobbett, 2000) thus removing these toxic elements from the cytosol (Verbruggen et al., 2009). The inactivation of toxic metal ions through the synthesis of phytochelatins and then the formation of metal–phytochelatin complexes is a general mechanism for trace metal homeostasis in plants (Zenk, 1996). It has also been demonstrated that efflux transporter (PvACR3) is oriented by tonoplast, which mediates As(III) sequestration into the vacuoles in hyperaccumulator fern, Pteris vittata (Indriolo et al., 2010). Additionally, Pickering et al. (2000) reported that As(III) in Brassica juncea is coordinated with sulfur groups. Depending to the acidic conditions which confer the stability of such complexes, As-PC complexes could be stored in the vacuoles (Meharg and Hartley-Whitaker, 2002). Further, complexation of metals with organo-ligands within the storage sites results in decreased free ion activity and thus reduced toxicity (Sanità di Toppi and Gabbrielli, 1999; Bhatia et al., 2005). Previous study by X-ray microanalyses, revealed that As was localized in the central intracellular portion of cells. Since the majority of this area is occupied by the vacuole, this suggests that As was mainly contained in the vacuoles (Lombi et al., 2002), which is in accordance with our findings of decreased importance of the cell wall as a defense mechanism to As accumulation. Further, an observation using EDXA suggested that the compartmentation in the vacuoles could be considered as an efficient strategy to maintain a low As concentration in the cytoplasm (Lombi et al., 2002).
Compartmentation is a key aspect in removing trace metal elements outside of key metabolic sites, contributing to the plants survival in salt depressions contaminated by these elements. This study gives an insight into the different distribution and localization of As and Al inside T. gallica. Further analyses to examine the distribution of As and Al and their bonding state at the ultra-structural level may improve our understanding of the effects of both elements on cell ultrastructure within the plant. A better understanding of metal sequestration in plants may eventually contribute toward the development of biorecovery techniques for the remediation of soils contaminated with these two traces metals.
Conclusion
From our study, we deduce that both the vacuole and the cell wall are involved in the As and Al tolerance mechanisms to protect metabolically active cellular compartments from toxic concentrations, and different bonding strategies are clearly involved depending on the element. This should not only be related to metal ligand specificities, but also to the distinct abundance of Al and As in the environment, and their degree of toxicity to the plant. Salt appears to exert some influence on metal compartmentation at a finer level, but overall there are fewer differences regarding the distribution among soluble, proteic, and cell wall bound metal. Thus, it can be stated that compartmentation in T. gallica is metal(loid) and organ specific, and a crucial tolerance mechanism.
Author Contributions
DBS and SP carried out all the experiments, assured data analysis and prepared the manuscript. BD and MSD assured samples' analysis. IC and NS conceived the idea, supervised the work and corrected the manuscript. All authors read and approved the final manuscript.
Conflict of Interest Statement
The authors declare that the research was conducted in the absence of any commercial or financial relationships that could be construed as a potential conflict of interest.
Acknowledgments
The authors would like to thank to the FCT for funding the research in the Marine and Environmental Sciences Centre (MARE) through the project UID/MAR/04292/2013.
References
Ager, F. J., Ynsa, M. D., Dominguez-Solis, J. R., Gotor, C., Respaldiza, M. A., and Romero, L. C. (2002). Cadmium localization and quantification in the plant Arabidopsis thaliana using micro-PIXE. Nucl. Instr. Meth. B 189, 494–498. doi: 10.1016/S0168-583X(01)01130-2
Ager, F. J., Ynsa, M. D., Dominguez-Solis, J. R., López-Martin, M. C., Gotor, C., and Romero, L. C. (2003). Nuclear micro-probe analysis of Arabidopsis thaliana leaves. Nucl. Instr. Meth. B 210, 401–406. doi: 10.1016/S0168-583X(03)01046-2
Alkorta, I., Hernandez-Allica, J., Becerril, J. M., Amezaga, I., Albizu, I., and Garbisu, C. (2004). Recent findings on the phytoremediation of soils contaminated with environmentally toxic heavy metals and metalloids such as zinc, cadmium, lead, and arsenic. Environ. Sci. Bio. Tech. 3, 71–90. doi: 10.1023/b:resb.0000040059.70899.3d
Barceló, J., and Poschenrieder, C. (1992). Respuestas de las plantas a la contaminación por metales pesados. Suelo. Planta 2, 345–361.
Bhalerao, S. A., and Prabhu, D. V. (2013). Aluminium toxicity in plants: a review. J. Appl. Chem. 2, 447–474.
Bhatia, N. P., Walsh, K. B., and Baker, A. J. M. (2005). Detection and quantification of ligands involved in nickel detoxification in a herbaceous Ni hyperaccumulator Stackhousia tryonii Bailey. J. Exp. Bot. 56, 1343–1349. doi: 10.1093/jxb/eri135
Burló, F., Guijarro, I., Carbonell-Barrachina, A. A., Valero, D., and Martinez-Sánchez, F. (1999). Arsenic species: effects on and accumulation by tomato plants. J. Agric. Food. Chem. 47, 1247–1253. doi: 10.1021/jf9806560
Caçador, I., Caetano, M., Duarte, B., and Vale, C. (2009). Stock and losses of trace metals from salt marsh plants. Mar. Environ. Res. 67, 75–82. doi: 10.1016/j.marenvres.2008.11.004
Carbonell-Barrachina, A. A., Aarabi, M. A., DeLaune, R. D., Gambrell, R. P., and Patrick, W. H. (1998). The influence of arsenic chemical form and concentration on Spartina patens and Spartina alterniflora growth and tissue arsenic concentration. Plant.Soil. 198, 33–43. doi: 10.1023/A:1004285625998
Carrier, P., Baryla, A., and Havaux, M. (2003). Cadmium distribution and microlocalization in oilseed rape (Brassica napus) after long-term growth on cadmium-contaminated soil. Planta 216, 939–950. doi: 10.1007/s00425-002-0947-6
Chang, Y. C., Yamamoto, Y., and Matsumoto, H. (1999). Accumulation of aluminium in the cell wall pectin in cultured tobacco (Nicotiana tabacum L.) cells treated with a combination of aluminium and iron. Plant.Cell. Environ. 22, 1009–1017. doi: 10.1046/j.1365-3040.1999.00467.x
Cobbett, C. S. (2000). Phytochelatins and their roles in heavy metal detoxification. Plant. Physiol. 123, 825–832. doi: 10.1104/pp.123.3.825
Collins, Y. E., and Stotzky, G. (1989). “Factors affecting the toxicity of heavy metals to microbes,” in Metal Ions and Bacteria, eds T. J. Beveridge and R. J. Doyle (Toronto, ON: Wiley), 31–90.
De la Fuente, J. M., Ramirez-Rodriguez, V., Cabrera-Ponce, J. L., and Herrera-Estrella, L. (1997). Aluminium tolerance in transgenic plants by alteration of citrate synthesis. Science 276, 1566–1568. doi: 10.1126/science.276.5318.1566
Delhaize, E., and Ryan, R. (1995). Aluminium toxicity and tolerance in plants. Plant Physiol. 107, 315–321. doi: 10.1104/pp.107.2.315
Deng, H., Yea, Z. H., and Wong, M. H. (2004). Accumulation of lead, zinc, copper and cadmium by 12 wetland plant species thriving in metal contaminated sites in China. Environ. Pollut. 132, 29–40. doi: 10.1016/j.envpol.2004.03.030
Dhankher, O. P., Rosen, B. P., McKinney, E. C., and Meagher, R. B. (2006). Hyperaccumulation of arsenic in the shoots of Arabidopsis silenced for arsenate reductase (ACR2). Proc. Natl. Acad. Sci. U.S.A. 103, 5413–5418. doi: 10.1073/pnas.0509770102
Farago, M. E., and Pitt, M. J. (1977). Plants which accumulate metals. Part III. A further investigation of two australian species which take up zinc. Inorg. Chim. Acta 24, 211–214. doi: 10.1016/S0020-1693(00)93876-3
Gao, H. J., Zhao, Q., Zhang, X. C., Wan, X. C., and Mao, J. D. (2014). Localization of fluoride and aluminium in subcellular fractions of tea tea leaves and roots. J. Agric. Food. Chem. 62, 2313–2319. doi: 10.1021/jf4038437
Ghnaya, T., Slama, I., Messedi, D., Grignon, C., Ghorbel, M. H., and Abdelly, C. (2007). Effects of Cd2+ on K+, Ca2+ and N uptake in two halophytes Sesuvium portulacastrum and Mesembryanthemum crystallinum: consequences on growth. Chemosphere 67, 72–79. doi: 10.1016/j.chemosphere.2006.09.064
Grant, C. A., Buckley, W. T., Bailey, L. D., and Selles, F. (1998). Cadmium accumulation in crops.Can. J. Plant. Sci. 78, 1–17. doi: 10.4141/P96-100
Gupta, D. K., Vandenhove, H., and Inouhe, M. (2013). “Role of phytochelatins in heavy metal stress and detoxification mechanisms in plants,” in Heavy Metal Stress in Plants, eds D. K. Gupta, F. J. Corpas, and J. M. Palma (Berlin: Springer-Verlag), 242.
Haiyan, M. A., Chang-Yan, T., Gu, F., and Jun-Feng, Y. (2011). Ability of multicellular salt glands in Tamarix species to secrete Na+ and K+ selectively. Sci. China. Life Sci. 3, 282–289. doi: 10.1007/s11427-011-4145-2
Hall, J. L. (2002). Cellular mechanisms for heavy metal detoxification and tolerance. J. Exp. Bot. 53, 1–11. doi: 10.1093/jexbot/53.366.1
Hewitt, E. J. (1966). Sand and Water Culture Methods Used in the Study of Plant Nutrition. Farnham Royal: Common wealth Agricultural Bureaux. Technical Communication No. 22 (Revised 2nd Edition) of the Commonwealth Bureau of Horticulture and Plantation Crops, East Malling, Maidstone, Kent.
Hossain, M. A., Piyatida, P., Teixeira da Silva, J. A., and Fujita, M. (2012). Molecular mechanism of heavy metal toxicity and tolerance in plants: central role of Glutathione in detoxification of reactive oxygen species and methylglyoxal and in heavy metal chelation. J. Bot. 2012:872875. doi: 10.1155/2012/872875
Indriolo, E., Na, G., Ellis, D., Salt, D. E., and Banks, J. A. (2010). A vacuolar arsenite transporter necessary for arsenic tolerance in the arsenic hyperaccumulating fern Pteris vittata is missing in flowering plants. Plant. Cell 22, 2045–2057. doi: 10.1105/tpc.109.069773
Jeffrey, E. H., Abreo, K., Burgess, E., Cannata, J., and Greger, J. L. (1996). Systemic aluminium toxicity: effects on bone, hematopoietic tissue, and kidney. J. Toxicol. Environ. Health 48, 649–665. doi: 10.1080/009841096161122
Khan, D. H., Duckett, J. G., Frankland, B., and Brian Kirkham, J. (1984). An X-ray microanalytical study of the distribution of cadmium in roots of Zea mays L. J. Plant. Physiol. 115, 19–28. doi: 10.1016/S0176-1617(84)80047-4
Koch, I., Feldmann, J., Wang, L., Andrewes, P., Reimer, K. J., and Cullen, W. R. (1999). Arsenic in the meager creek hot springs environment, British Columbia, Canada. Sci. Total. Environ. 236, 101–117. doi: 10.1016/S0048-9697(99)00273-9
Kochian, L. V. (1995). Cellular mechanisms of aluminium toxicity and resistance in plants. Annu. Rev. Plant. Physiol. Plant. Mol. Biol. 46, 237–260. doi: 10.1146/annurev.pp.46.060195.001321
Kramer, U., Smith, R. D., Wenzel, W. W., Raskin, I., and Salt, D. E. (1997). The role of metal transport and tolerance in nickel hyperaccumulation by Thlaspi goesingense. Plant Physiol. 115, 1641–1650. doi: 10.1104/pp.115.4.1641
Krzesłowska, M. (2011). The cell wall in plant cell response to trace metals: polysaccharide remodeling and its role in defense strategy. Acta Physiol. Plant. 33, 35–51. doi: 10.1007/s11738-010-0581-z
Küpper, H., Lombi, E., Zhao, F. J., Wieshammer, G., and McGrath, S. P. (2001). Cellular compartmentation of nickel in the hyperaccumulators Alyssum lesbiacum, Alyssum bertolonii and Thlaspi goesingense. J. Exp. Bot. 52, 2291–2300. doi: 10.1093/jexbot/52.365.2291
Küpper, H., Zhao, F. J., and McGrath, S. P. (1999). Cellular compartmentation of zinc in leaves of the hyperaccumulator Thlaspi caerulescens. Plant. Physiol. 119, 305–311. doi: 10.1104/pp.119.1.305
Leitenmaier, B., and Küpper, H. (2013). Compartmentation and complexation of metals in hyperaccumulator plants. Front. Plant. Sci. 4:374. doi: 10.3389/fpls.2013.00374
Lombi, E., Zhao, F. J., Fuhrmann, M., Ma, L. Q., and McGrath, S. P. (2002). Arsenic distribution and speciation in the fronds of the hyperaccumulator Pteris vittata. New. Phytol. 156, 195–203. doi: 10.1046/j.1469-8137.2002.00512.x
Lozano-Rodriguez, E., Hernandez, L. E., Bonay, P., and Carpena-Ruiz, R. O. (1997). Distribution of cadmium in shoot and root tissues of maize and pea plants: physiological disturbances. J. Exp. Bot. 48, 123–128. doi: 10.1093/jxb/48.1.123
Lu, L., Tian, S., Zhang, J., Yang, X., Labavitch, J. M., and Webb, S. M. (2013). Efficient xylem transport and phloem remobilization of Zn in the hyperaccumulator plant species Sedum alfredii. New. Phytol. 198, 721–731. doi: 10.1111/nph.12168
Ma, J. F. (2000). Role of organic acids in detoxification of aluminium in higher plants. Plant.Cell. Physiol. 41, 383–390. doi: 10.1093/pcp/41.4.383
Ma, J. F. (2005). Plant root responses to three abundant soil minerals: silicon, aluminium and iron. Crit. Rev. Plant. Sci. 24, 267–281. doi: 10.1080/07352680500196017
Ma, J. F. (2007). Syndrome of aluminium toxicity and diversity of aluminium resistance in higher plants. Int. Rev. Cytol. 264, 225–252. doi: 10.1016/S0074-7696(07)64005-4
Marin, A. R., Masscheleyn, P. H., and Patrick, W. H. (1992). The influence of chemical form and concentration of arsenic on rice growth and tissue arsenic concentration. Plant. Soil. 139, 175–183. doi: 10.1007/BF00009308
Mateos-Naranjo, E., Andrades-Moreno, L., and Redondo-Gómez, S. (2012). Tolerance and accumulation of arsenic in the cordgrass Spartina densiflora, Brongn. Bioresour. Technol. 104, 187–194. doi: 10.1016/j.biortech.2011.11.006
Matsumoto, H., Hirasawa, E., Morimura, S., and Takahashi, E. (1976). Localization of aluminium in tea leaves. Plant. Cell. Physiol. 17, 627–631.
Meharg, A. A., and Hartley-Whitaker, J. (2002). Arsenic uptake and metabolism in arsenic resistant and non resistant plant species. New.Phytol. 154, 29–44. doi: 10.1046/j.1469-8137.2002.00363.x
Moreno-Jiménez, E., Esteban, E., Carpena-Ruiz, R. O., and Pe-alosa, J. M. (2009). Induced phytotoxicity in Mediterranean shrubs P. lentiscus and T. gallica after arsenic or mercury accumulation. Ecotoxicol. Environ. Saf. 72, 1781–1789. doi: 10.1016/j.ecoenv.2009.04.022
Moreno-Jiménez, E., Esteban, E., and Pe-alosa, J. M. (2012). The fate of arsenic in the soil–plant system. Rev. Environ. Contam. Toxico. 215, 1–37. doi: 10.1007/978-1-4614-1463-6_1
Papernik, L. A., Bethea, A. S., Singleton, T. E., Magalhaes, J. V., Garvin, D. F., and Kochian, L. V. (2001). Physiological basis of reduced Al tolerance in ditelosomic lines of Chinese Spring wheat. Planta 212, 829–834. doi: 10.1007/s004250000444
Pickering, I. J., Prince, R. C., George, M. J., Smith, R. D., George, G. N., and Salt, D. E. (2000). Reduction and coordination of arsenic in Indian mustard. Plant Physiol. 122, 1171–1177. doi: 10.1104/pp.122.4.1171
Pineros, M. A., and Kochian, L. V. (2001). A patch-clamp study on the physiology of aluminium toxicity and aluminium tolerance in maize. Identification and characterization of Al3+ induced anion channels. Plant Physiol. 125, 292–305. doi: 10.1104/pp.125.1.292
Probst, A., Liu, H., Fanjul, M., Liao, B., and Hollande, E. (2009). Response of Vicia faba L. to metal toxicity on mine tailing substrate: geochemical and morphological changes in leaf and root. Environ. Exp. Bot. 66, 297–308. doi: 10.1016/j.envexpbot.2009.02.003
Psaras, G. K., Constantinidis, Th., Cotsopoulos, B., and Manetas, Y. (2000). Relative abundance of nickel in the leaf epidermis of eight hyperaccumulators: evidence that the metal is excluded from both guard cells and trichomes. Ann. Bot. 86, 73–78. doi: 10.1006/anbo.2000.1161
Psaras, G. K., and Manetas, Y. (2001). Nickel localization in seeds of the metal hyperaccumulator Thlaspi pindicum Hausskn. Ann. Bot. 88, 513–516. doi: 10.1006/anbo.2001.1470
Quaghebeur, M., and Rengel, Z. (2004). Arsenic uptake, translocation and speciation in pho1 and pho2 mutants of Arabidopsis thaliana. Physiol. Planta 120, 280–286. doi: 10.1111/j.0031-9317.2004.0240.x
Ramos, I., Esteban, E., Lucena, J. J., and Gàrate, A. (2002). Cadmium uptake and subcellular distribution in plants of Lactuca sp. Cd-Mn interaction. Plant. Sci. 162, 761–767. doi: 10.1016/S0168-9452(02)00017-1
Rascio, N., and Navari-Izzo, F. (2011). Heavy metal hyperaccumulating plants: how and why do they do it? And what makes them so interesting? Plant. Sci. 180, 169–181. doi: 10.1016/j.plantsci.2010.08.016
Raskin, I., and Ensley, B. (2000). Phytoremediation of Toxic Metals: Using Plants to Clean Up the Environment. New York, NY: John Wiley & Sons Inc.
Rauser, W. E. (1999). Structure and function of metal chelators produced by plants: the case for organic acids, amino acids, phytin and metallothioneins. Cell Biochem. Biophys. 31, 19–48. doi: 10.1007/BF02738153
Rauser, W. E., and Ackerley, C. A. (1987). Localization of cadmium in granules within differentiating and mature root cells. Can. J. Bot. 65, 643–646. doi: 10.1139/b87-084
Reboreda, R., and Caçador, I. (2007). Halophyte vegetation influences in salt marsh retention capacity for heavy metals. Environ. Pollut. 146, 147–154. doi: 10.1016/j.envpol.2006.05.035
Revathi, S., and Subhashree, V. (2013). Physiological and biochemical mechanisms of heavy metal tolerance. Int. J. Environ. Sci. 3, 1339–1354. doi: 10.1038/srep11433
Saïdana, D., Mahjoub, M. A., Boussaada, O., Chria, J., Chéraif, I., Daami, M., et al. (2008). Chemical composition and antimicrobial activity of volatile compounds of Tamarix boveana (Tamaricaceae). Microbiol. Res. 163, 445–455. doi: 10.1016/j.micres.2006.07.009
Sanità di Toppi, L., and Gabbrielli, R. (1999). Response to cadmium in higher plants. Environ. Exp. Bot. 41, 105–130. doi: 10.1016/S0098-8472(98)00058-6
Seregin, I. V., and Ivanov, V. B. (1997). Histochemical investigation of cadmium and lead distribution in plants. Russ. J. Plant. Physiol. 44, 791–796.
Sghaier, D. B., Duarte, B., Bankaji, I., Caçador, I., and Sleimi, N. (2015). Growth, chlorophyll fluorescence and mineral nutrition in the halophyte Tamarix gallica cultivated in combined stress conditions: Arsenic and NaCl. J. Photochem. Photobiol. B Biol. 149, 204–214. doi: 10.1016/j.jphotobiol.2015.06.003
Sousa, A. I., Caçador, I., Lillebø, A. I., and Pardal, M. A. (2008). Heavy metal accumulation in Halimione portulacoides: intra- and extra-cellular metal binding. Chemosphere 70, 850–857. doi: 10.1016/j.chemosphere.2007.07.012
Stolt, J. P., Sneller, F. E. C., Brynelsson, T., Lundborg, T., and Schat, H. (2003). Phytochelatin and cadmium accumulation in wheat. Environ. Exp. Bot. 49, 21–28. doi: 10.1016/S0098-8472(02)00045-X
Tolra, R., Vogel-Mikus, K., Hajiboland, R., Kump, P., Pongrac, P., Kaulich, B., et al. (2011). Localization of aluminium in tea (Camellia sinensis L.) leaves using low energy X-ray fluorescence spectro-microscopy. J. Plant. Res. 124, 165–172. doi: 10.1007/s10265-010-0344-3
Upadhyaya, H., Panda, S. K., Bhattacharjee, M. K., and Dutta, S. (2010). Role of arbuscular mycorrhiza in heavy metal tolerance in plant: prospect for phytoremediation. J. Phytol. 2, 16–27.
Vázquez, M. D., Barceló, J., Poschenrieder, Ch., Mádico, J., Hatton, P., Baker, A. J. M., et al. (1992a). Localization of zinc and cadmium in Thlaspi caerulescens (Brassicaceae), a metallophyte that can accumulate both metals. J. Plant. Physiol. 140, 350–355. doi: 10.1016/S0176-1617(11)81091-6
Vázquez, M. D., Poschenrieder, Ch., and Barceló, J. (1992b). Ultrastructural effects and localization of low cadmium concentrations in bean roots. New. Phytol. 120, 215–226.
Verbruggen, N., Hermans, C., and Schat, H. (2009). Molecular mechanisms of metal hyperaccumulation in plants. New. Phytol. 181, 759–796. doi: 10.1111/j.1469-8137.2008.02748.x
Wagner, G. J. (1993). Accumulation of cadmium in crop plants and its consequences to human health. Adv. Agro. 51, 173–212. doi: 10.1016/S0065-2113(08)60593-3
Wenzel, W. W. (2013). “Arsenic” in Heavy Metals in Soils. Trace Metals and Metalloids in Soils and Their Bioavailability, 3rd Edn., ed B. J. Alloway (Wenzel: Springer), 613.
Zenk, M. H. (1996). Heavy metal detoxification in higher plants. Gene 179, 21–30. doi: 10.1016/S0378-1119(96)00422-2
Zhao, F. J., Ma, J. F., Meharg, A. A., and McGrath, S. P. (2009). Arsenic uptake and metabolismin plants. New. Phytol. 181, 777–794. doi: 10.1111/j.1469-8137.2008.02716.x
Keywords: arsenic, aluminum, cellular compartmentation, detoxification, Tamarix gallica
Citation: Sghaier DB, Pedro S, Diniz MS, Duarte B, Caçador I and Sleimi N (2016) Tissue Localization and Distribution of As and Al in the Halophyte Tamarix gallica under Controlled Conditions. Front. Mar. Sci. 3:274. doi: 10.3389/fmars.2016.00274
Received: 27 September 2016; Accepted: 12 December 2016;
Published: 27 December 2016.
Edited by:
Julian Blasco, Spanish National Research Council, SpainReviewed by:
Natalia Ospina-Alvarez, Universität Potsdam, GermanyPeriyadan K. Krishnakumar, King Fahd University of Petroleum and Minerals, Saudi Arabia
Copyright © 2016 Sghaier, Pedro, Diniz, Duarte, Caçador and Sleimi. This is an open-access article distributed under the terms of the Creative Commons Attribution License (CC BY). The use, distribution or reproduction in other forums is permitted, provided the original author(s) or licensor are credited and that the original publication in this journal is cited, in accordance with accepted academic practice. No use, distribution or reproduction is permitted which does not comply with these terms.
*Correspondence: Noomene Sleimi, bm9vbWVuZS5zbGVpbWlAZ21haWwuY29t