- 1Israel Oceanographic and Limnological Research, National Institute of Oceanography, Haifa, Israel
- 2Mina and Everard Goodman Faculty of Life Sciences, Bar-Ilan University, Ramat-Gan, Israel
- 3Institute of Oceanography, Hellenic Centre for Marine Research, Heraklion, Crete, Greece
- 4Department of Biology, University of Bergen, Bergen, Norway
- 5Institute of Oceanography, Hellenic Centre for Marine Research, Anavyssos, Attiki, Greece
- 6Department of Chemistry, University of Crete, Heraklion, Crete, Greece
- 7Laboratory of Environmental Chemistry, Department of Chemistry, University of Athens, Athens, Greece
- 8School of Earth and Environment, University of Leeds, Leeds, UK
- 9Department of Marine Biology, Charney School of Marine Sciences, Haifa University, Haifa, Israel
- 10Department of Geography, Earth and Environmental Sciences, Birmingham University, Birmingham, UK
- 11MARUM Center for Marine Environmental Sciences, Bremen, Germany
- 12Laboratoire d'Océanographie Physique et Biogéochimique, Université de la Méditerranée, Marseille, France
Recent estimates of nutrient budgets for the Eastern Mediterranean Sea (EMS) indicate that atmospheric aerosols play a significant role as suppliers of macro- and micro- nutrients to its Low Nutrient Low Chlorophyll water. Here we present the first mesocosm experimental study that examines the overall response of the oligotrophic EMS surface mixed layer (Cretan Sea, May 2012) to two different types of natural aerosol additions, “pure” Saharan dust (SD, 1.6 mg l−1) and mixed aerosols (A—polluted and desert origin, 1 mg l−1). We describe the rationale, the experimental set-up, the chemical characteristics of the ambient water and aerosols and the relative maximal biological impacts that resulted from the added aerosols. The two treatments, run in triplicates (3 m3 each), were compared to control-unamended runs. Leaching of ~2.1–2.8 and 2.2–3.7 nmol PO4 and 20–26 and 53–55 nmol NOx was measured per each milligram of SD and A, respectively, representing an addition of ~30% of the ambient phosphate concentrations. The nitrate/phosphate ratios added in the A treatment were twice than those added in the SD treatment. Both types of dry aerosols triggered a positive change (25–600% normalized per 1 mg l−1 addition) in most of the rate and state variables that were measured: bacterial abundance (BA), bacterial production (BP), Synechococcus (Syn) abundance, chlorophyll-a (chl-a), primary production (PP), and dinitrogen fixation (N2-fix), with relative changes among them following the sequence BP>PP≈N2-fix>chl-a≈BA≈Syn. Our results show that the “polluted” aerosols triggered a relatively larger biological change compared to the SD amendments (per a similar amount of mass addition), especially regarding BP and PP. We speculate that despite the co-limitation of P and N in the EMS, the additional N released by the A treatment may have triggered the relatively larger response in most of the rate and state variables as compared to SD. An implication of our study is that a warmer atmosphere in the future may increase dust emissions and influence the intensity and length of the already well stratified water column in the EMS and hence the impact of the aerosols as a significant external source of new nutrients.
Introduction
In low-nutrient low-chlorophyll (LNLC) marine environments, nutrient and trace metal inputs via atmospheric aerosols are considered important sources of macro and micro nutrients (Duce et al., 1991, 2008; Jickells et al., 2005; Kanakidou et al., 2012), fueling microbial production and influencing the bacterioplankton community structure (Moore et al., 2013; Guieu et al., 2014a; Chien et al., 2016; Rahav et al., 2016a). The Eastern Mediterranean Sea (EMS), located in the so-called dust-belt (Astitha et al., 2012), is considered extremely oligotrophic (reviewed in Siokou-Frangou et al., 2010) and is strongly influenced by natural desert sources that contain some of the highest atmospheric dust (mineral aerosol) concentrations near the Earth's surface (Klingmüller et al., 2016). The EMS region has been identified in the last decade as a hot-spot of climate change, showing a decrease in precipitation and an increase in the temperature and annual number of unusually hot days (Hoerling et al., 2012; Lelieveld et al., 2012; IPCC, 2014). It is suggested that the increasing temperatures together with the decreasing relative humidity of the last decade, have promoted soil drying, leading to increased dust emissions in the EMS, a process that is expected to continue in the future due to climate change (Klingmüller et al., 2016) and consequently supply more macro and micro nutrients into its surface oligotrophic water.
The EMS is also exposed to relatively high pollution levels of aerosols (Lelieveld et al., 2002), which interact with the already high background levels of natural mineral particles. The bioavailability of nutrients and trace metals from aerosols is related to both, the original aerosol chemical and mineralogical composition and the interactions and chemical transformations during atmospheric transport (Baker et al., 2006; Baker and Jickells, 2006; Mackey et al., 2015). Recent studies have shown that the exposure to acid processes in the atmosphere can also change the phosphorus (P) bioavailability of dust/aerosols (Nenes et al., 2011; Bougiatioti et al., 2016).
Atmospheric deposition of nutrients to LNLC provinces is particularly important in regions where there is little input of new nutrients from other external sources (e.g., Jickells et al., 2005; Duce et al., 2008), as is the case in the EMS (Herut et al., 1999, 2002; Krom et al., 2004). Such inputs are more significant during stratified periods, as they enable new production in a period of generally low autotrophic production (Guieu et al., 2010). In addition to new production, it has been shown that leachable nutrients from atmospheric inputs may enhance N2 fixation, changes in phytoplankton species composition and carbon sequestration (e.g., Mills et al., 2004; Guo et al., 2012; Moore et al., 2013; Bressac et al., 2014; Guieu et al., 2014b; Rahav et al., 2016a). While a low availability of macronutrients (N, P) and metal micronutrients (e.g., Fe, Co) can limit or co-limit phytoplankton growth in the ocean (e.g., Moore et al., 2013), high concentrations of some metals (e.g., Cu or Al) can be toxic to phytoplankton (e.g., Paytan et al., 2009; Jordi et al., 2012; Krom et al., 2016). Yet, the impact of these dust inputs on microbial populations has not been fully investigated in the EMS. The response of oligotrophic systems to dust inputs, whether as positive or as negative feedbacks to autotrophic and heterotrophic production (and thus to biogeochemical cycling), must therefore be further examined (Guieu et al., 2014a).
To date, these influences were assessed in the EMS mainly by on-board dust enrichment microcosm experiments (Herut et al., 2005; Ternon et al., 2011) or in situ observations (Rahav et al., 2016a), which showed an increase in primary production and in heterotrophic bacterial activity, while no previous mesocosm experiment has been performed. The use of large-scale mesocosms may be particularly important to reduce the effects of bottle enclosure that may change the autotrophic-heterotrophic biomass ratio, especially in oligotrophic regions such as the EMS (Calvo-Díaz et al., 2011). Similarly, a mesocosm experiments in the Western Mediterranean Sea examined the addition of a dust analog (treated Saharan soil) to surface seawater (Guieu et al., 2014b). In that experiment, the Saharan dust amendment strongly stimulated primary production and algal biomass and altered the autotrophic phytoplankton communities (Guieu et al., 2014b). Furthermore, these additions affected the structure, diversity and functioning of the microbial food web (Pulido-Villena et al., 2014) while also increasing the N2 fixation rates (Ridame et al., 2013).
Here we present an overview of a mesocosm experimental study that examines the microbial response of the oligotrophic EMS surface mixed layer (Cretan Sea, May 2012) to two different types of natural aerosol additions; “pure Saharan dust” and “mixed aerosols” (a natural mixture of desert dust and polluted particles). To the best of our knowledge, this is the first mesocosm study in which naturally collected aerosols were used in the Mediterranean Sea. The added particles were designed to mimic the impact of an intense dry atmospheric deposition to the upper mixed layer (1–1.5 mg of dust l−1) on the physiology and biomass of the ambient microbial populations of the EMS, using natural aerosols collected across the Levantine basin (Crete and Israel). The two aerosol types were compared to control runs in triplicates for a total duration of 8 days.
This article provides a brief overview of the design, rationale, characteristics of the aerosols and the principal responses of the microbial variables in the mesocosm experiment, as part of a Research Topic entitled “Impact of aerosols (Saharan dust and mixed) on the East Mediterranean oligotrophic ecosystem, results from experimental studies.” Other components of the mesocosm experiment, including microbial community structure and temporal dynamics, microbial biodiversity and function are discussed in other articles of this special issue (Guo et al., 2016; Rahav et al., 2016b; and others in this special issue).
Materials and Methods
Mesocosm Experimental Design and Sampling
An aerosol-enrichment mesocosm experiment was performed at the CRETACOSMOS facility of the Hellenic Centre for Marine Research (HCMR, www.cretacosmos.eu) in Crete, Greece, during the 10–18 of May 2012. The facility consists of a 350 m3 land-based concrete pond, 5 m deep, supplied with continuous seawater flow-through in order to maintain ambient surface water temperature. The experiment was carried out using surface (~10 m depth) seawater that was collected using a rotary submersible pump placed onboard the R/V Philia from a location 5 nautical miles north of Heraklion (Crete, Greece, 35° 24.957 N, 25° 14.441 E) at a bottom depth of 170 m during the 8–9 of May 2012. The collected seawater was equally distributed by gravity into nine food-grade polyethylene mesocosm bags to ensure the homogeneity of the collected seawater between bags. The mesocosms were mounted on aluminum frames (1.12 m diameter) attached to the pool's walls. Each mesocosm had a total volume of 3 m3 (Figure 1). The mesocosms were gently mixed throughout the experiment, using an airlift pump to avoid stratification. The mesocosms were covered with a two-layer lid in order to protect them from natural atmospheric aerosol depositions during the experiment and mimic the light conditions at a 10 m water depth. HOBO data loggers (ONSET Corporation) were installed to measure the temperature and light (irradiation) in each bag as well as in the main pond.
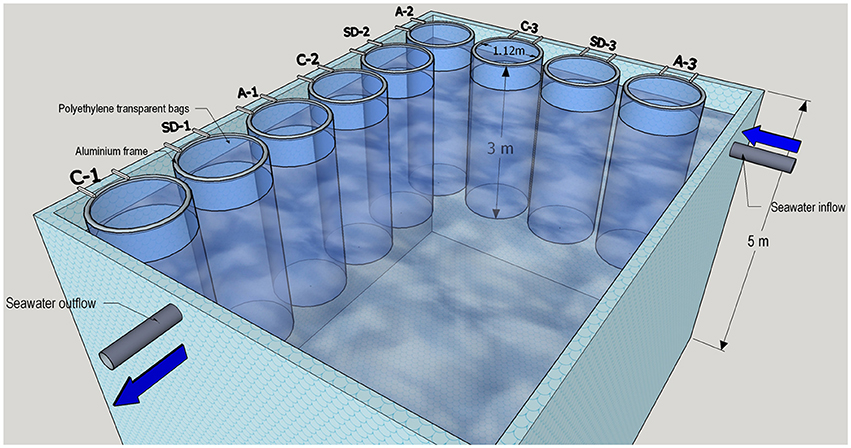
Figure 1. A schematic illustration of the experimental mesocosm setup facility held in May 10-18 2012 in Crete. Two treatments (SD and A) and an unamended control (C) were run in triplicate 3 m3 mesocosm bags.
On May 10th at 08:30, samples were collected from all the mesocosms prior to the dust/aerosol additions to serve as reference conditions. At 11:45, the dust/aerosols were added to the mesocosms and the first sampling was carried out 3 h later at 14:45. Each mesocosm was sampled daily during the morning (08:30) from May 11th to 15th, 2012, and then once every 2 days until May 18th, apart from parameters that required more frequent monitoring, which were sampled every day throughout the experiment. Acid-washed silicone tubes were used for transferring the samples collected at about 50 cm depth into similarly treated 20 L Nalgene containers.
Two different types of natural additions were performed in triplicates: “pure” Saharan dust, (labeled as SD) and mixed aerosol containing a natural mixture of desert dust and polluted European particles (labeled as A). The additions of SD and A resulted in a final concentration of 1.6 and 1 mg l−1, respectively, in the mesocosm bags (Table 1). Triplicate control (labeled C) mesocosm treatments were run in parallel. Each replicate addition was performed by pouring a concentrated mixture of the aerosol/dust with filtered (0.2 μm) seawater into the bags. This mixture was prepared just prior to the addition in pre-cleaned (10% hydrochloric acid washed) 100 ml polyethylene bottles. In order to mimic an intense dust storm effect, it was decided to add quantity equivalent to final concentration of 1.6 mg l−1 of aerosols in the mesocosm bags. Previous studies yielded a deposition of ~1 mg of dust l−1 in the upper 5 m mixed layer during dust storm events in the EMS (Herut et al., 2005; Rahav et al., 2016a). Yet, due to the limited mass availability of aerosols representing an European origin (Figure 2), we prepared a mixture of desert/mineral dust and polluted aerosols (treatment A), allowing for an addition equivalent to only 1 mg l−1 in the relevant mesocosms (Table 1, Figure 1). Aerosol chemical composition—Analyses of the chemical compositions of SD and A aerosols were carried out after total digestion with HF and aqua regia, following the procedure of ASTM (1983) and Herut et al. (2001). The digested samples were diluted in 25 ml with Milli-Q water and filtered through a Whatman 42 filter paper. Briefly, 1 ml of aqua regia solution and 4 ml of hydrofluoric acid were added to ca. 0.15 g of dry aerosol in 125 ml plastic bottles (that can withstand temperatures of up to 1300°C on a sand bath). Prior to dilution, 5 ml of saturated boric acid was added to the resultant solution. The concentrations of trace elements were measured using an Agilent 280FS atomic absorption spectrometer, a graphite furnace Agilent 240Z AA and ICP-MS. Major elements were measured on an ICP-AES. The bottle blanks were usually less than 2% of the measured concentrations. The accuracy of the method was evaluated based on analyses of International Certified Reference Materials: MESS-4, SRM 2702, and IAEA 158. All elements gave results within 94–110% of the certified values, except for Cd, which gave an 80% recovery.
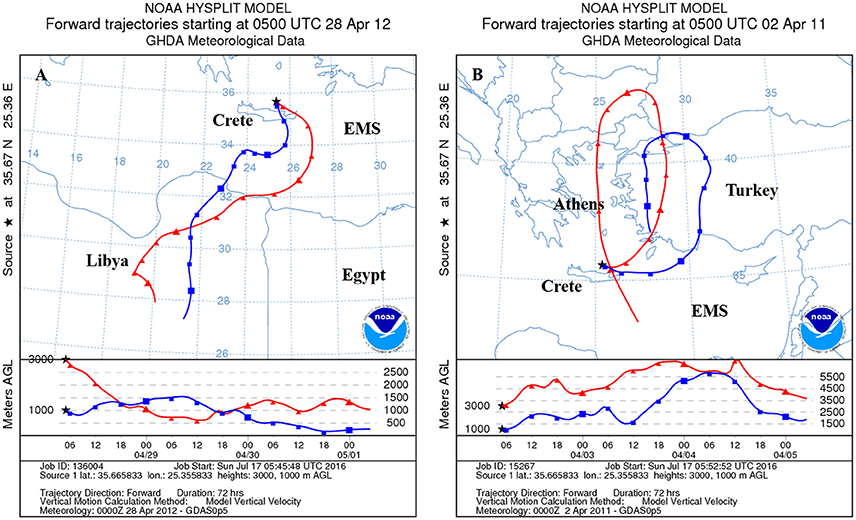
Figure 2. Representative air mass back trajectories derived from the backward trajectories model NOAA/ARL HYSPLIT-4 (www.noaa.gov), showing the origin and track of the Saharan-dominated population (A) and the anthropogenic-dominated population (B) over the 3 days that preceded their collection at Crete and Israel.
Aerosol Collection and Leaching Experiments
Dry deposited material was collected in Crete (Heraklion and Sambas) and Israel (Beit Yannay and Tel Shikmona, see Herut et al., 2002) during major Saharan dust storms and during periods that showed the transport of air masses was from Europe (Table 1, Figure 2). These two sites represent aerosols across the Levantine basin in the SE Mediterranean Sea. Aerosol origin and route was tracked by calculating 3-day back trajectories using the NOAA HYSPLIT model from the Air Resources Laboratory at 1000 and 3000 m altitude levels (Figure 2). We needed between 9 and 15 g of material for the additions to the different mesocosm replicates to obtain the final concentrations in the mesocosm bags (1 and 1.6 mg l−1), and therefore material collected on different sampling dates was pooled, representing the two types of treatments (Table 1). However, while for the SD treatment sufficient material was collected, only a limited amount could be collected to represent a clear dominated polluted European derived aerosols. Therefore, treatment A represents a mixture of European as well as desert aerosols (Table 1).
Leaching experiments were performed according to two different methodologies using sterile surface seawater from different origins, in the nutrient laboratories of the Israel Oceanographic and Limnological Research (Haifa) and at the University of Leeds (Figure S1). In Haifa, 250 ml plastic containers were pre-cleaned with 10% hydrochloric acid and washed afterwards with Milli-Q water. Each bottle was filled with filtered (0.2 μm), aged and poisoned (with 50 μL chloroform) SE Mediterranean surface seawater (collected with the R/V Shikmona, 40 km off the Israeli coast), to which 30 mg of SD or A powder was added. The bottles were covered with aluminum foil and shaken at room temperature for a total of 48 h. Subsamples (10 ml) collected at 0, 0.75, 2, 6, 24, and 48 h were pipetted using pre-cleaned (10% HCl) syringes through a 0.2 μm filter and immediately analyzed for nitrate+nitrite and phosphate concentrations. Nutrients were measured with a Seal Analytical AA-3 system (Kress et al., 2014; Ozer et al., in press). The precision level for nitrate+nitrite and phosphate was 0.02 and 0.003 μM, respectively. All analytical results were corrected against unamended blanks. The limit of detection (2 times the standard deviation of the blank) for the procedures was 0.075 μM for nitrate+nitrite and 0.008 μM for phosphate. For simplicity, we refer in the text to nitrate+nitrite as NOx and to ortho-phosphate as P. The quality assurance of the nutrient measurements was confirmed by the results of inter-comparison exercises (NOAA/NRC, JAPAN, QUASIMEME).
In Leeds, prior to the leaching of phosphate, 120 ml plastic containers were coated with iodine by adding a crystal or two of elemental iodine and placed in an oven at 40°C for 10 min. The containers were then cooled to room temperature (22°C) and swilled with Milli-Q water to remove all the excess I2. For the nitrate leaching, uncoated 250 ml plastic containers were used. For the phosphate leaching experiment, 50 ml of sterile seawater was added to each container in a biological safety cabinet. ~6 mg of accurately weighed dust was then added and the containers were placed on a shaking table for 30 min, 2, 6, 24, and 49 h situated in a light proof box. The duration of the leaching experiment was based on the study of Mackey et al. (2012), which found an increasing amount of leached P after 24–48 h, and assumed stabilization. At each sampling, ~7 ml were removed by syringe from the sample, filtered through 0.45 μm polycarbonate filters and stored at 4°C for subsequent analysis. For the nitrate leaching experiment, 100 ml of sterile seawater were used and sampled in the same way as the other determinants, after 30 min of leaching. The analysis of all the nitrate and phosphate samples was carried out after the 48 h sample was collected. A series of 6 blank samples were run through the sampling procedure and the average values were subtracted from each sample analyzed (mean blank values were −3 ± 4 nmol P l−1 and 48 ± 50 nmol NOx l−1). Nutrient content was determined by standard SEAL AA-3 automated methods for phosphate (using the molybdate blue method), nitrate (as nitrite after Cd column reduction) and ammonium (using a fluorescence method). The precision of the replicate analysis was 1.8 ± 0.01 μM for phosphate, 6.0 ± 0.05 μM for nitrate and 5.75 ± 0.05 μM for ammonium.
Measurement of 33PO4 Uptake
Samples (10 ml) for PO4 turnover times were collected every day and measured using 33P-orthophosphate (Thingstad et al., 1993). Carrier-free 33P-orthophosphate (PerkinElmer, specific activity: 370 MBq ml−1) was added to the samples at a final concentration of 20–79 pM. Samples used for the subtraction of the background and abiotic adsorption were fixed with 100% trichloroacetic acid (TCA) (final conc. 0.5%) before the isotope's addition. The samples were then incubated under subdued (laboratory) illumination. The incubation time varied between 1 and 20 min: short enough to assure a linear relationship between the fractions of the isotope adsorbed vs. the incubation time but long enough to reliably detect any isotope uptake above the background levels. Incubation was terminated by a cold chase of 100 mM KH2PO4 (final conc. 1 mM). Subsamples (3.3 ml) were filtered in parallel onto 25 mm polycarbonate filters with 2, 0.6, and 0.2 μm pore sizes. All filters were placed on a Millipore 12 place manifold with Whatman (GF/C) glass fiber filters saturated with 100 mM KH2PO4 as support. After filtration, the filters were placed in polyethylene vials with an Ultima Gold (Packard) scintillation cocktail and radio-assayed. After the radio-activities of the filter were corrected for those of the blank filter obtained from fixed samples, the phosphate turnover time (T[PO4]: h) was calculated as T[PO4] = −t/ln(1 − f), where f is the fraction (no dimension) of added isotope collected on the 0.2 μm filter after the incubation time (t: h).
Phosphate Concentrations in the Mesocosm Bags
Water samples were collected and analyzed immediately for their phosphate concentrations using the MAGIC method (Rimmelin and Moutin, 2005). The detection limit was 1.6 nM for phosphate.
Chlorophyll a
Seawater samples (500 ml) were passed through a Whatman GF/F filter (~0.7 μm pore size) and extracted overnight (16 h) in 10 ml of 90% acetone solution in the dark (Holm-Hansen et al., 1965). Chlorophyll a concentrations were determined by the non-acidification method (Welschmeyer, 1994) using a TD700 fluorometer equipped with 436 nm excitation and 680 nm emission filters.
Picophytoplankton and Heterotrophic Bacterial Abundance
Samples for determining the picophytoplankton and heterotrophic bacterial abundance were collected every day throughout the experiment's duration. The samples were fixed with 0.2 μm filtered glutaraldehyde (a final concentration of 0.5%), kept at 4°C for ~45 min, flash-frozen in liquid nitrogen and then transferred to a −80°C refrigerator until further processing. Frozen samples were thawed at room temperature and sub-samples were stained with SYBR Green I and incubated for 10 min in the dark, according to Vaulot and Marie (1999). Samples for picophytoplankton abundance were analyzed based on their auto-fluorescence signals, without pre-staining, using a FACSCalibur (Becton Dickinson) flow cytometer equipped with an air-cooled laser at 488 nm and a standard filter set-up. Flow cytometry data were acquired and processed with the Cell Quest Pro software (Becton Dickinson). An average estimated flow rate of 58 μL min−1 was used. The picophytoplankton carbon biomass was calculated from cell counts, assuming 175 fg C cell−1 for Synechococcus cells, 53 fg C cell−1 es (Campbell and Yentsch, 1989).
Primary Productivity (PP)
Photosynthetic carbon fixation rates were estimated using the 14C incorporation method (Steemann-Nielsen, 1952). For each mesocosm, three light and one dark 320-mL polycarbonate bottles were filled with sample water during morning time (09:00–10:00 a.m.), inoculated with 5 μCi of NaH14CO3 tracer (Perkin-Elmer) and incubated in a land-based tank for 3 h. At the end of the incubation, the spiked seawater samples were filtered through 0.2 μm polycarbonate filters (47 mm diameter) under low vacuum pressure (<150 mmHg) and the filters were collected for the determination of primary production rate. The filters were placed in 5-ml scintillation vials and were acidified with 1 ml of 0.1 N HCL in order to remove excess 14C-bicarbonate overnight. After the addition of 4 ml scintillation cocktail (ULTIMA-GOLD), the radioactivity of the samples (disintegrations per minute, dpm) was measured in a Liquid Scintillation Counter (Packard Tri-Card 4000). Primary production rates were calculated by subtracting the dpm of the dark bottles from the respective light ones. We used a value of 26,400 mg C m−3 for the concentration of dissolved inorganic carbon (Triantaphyllou et al., 2010) and a value of 1.05 for the isotopic discrimination factor (Lagaria et al., 2011).
Bacterial Productivity (BP)
BP was estimated by the 3H-leucine method (Kirchmann et al., 1986), as modified by Smith and Azam (1992). For each mesocosm, duplicate SD, A and control samples were incubated with a mixture of L-[45 3H]-leucine (Perkin Elmer, 115 Ci mmol−1) and non-radioactive leucine to a final concentration of 20 nM. Samples were incubated for 2 h in the dark at the in-situ temperature, after which they were fixed and treated following the micro-centrifugation protocol (Smith and Azam, 1992), as described in detail by Van Wambeke et al. (2008). In brief, incubations were terminated after 2 h by the addition of trichloroacetic acid (TCA). Samples were then centrifuged at 16,000 × g and the resulting cell pellet was washed twice with 5% TCA and with 80% ethanol. Incorporation of 3H- leucine into the TCA-insoluble fraction was measured by liquid scintillation counting (Packard Tri-Carb 4000TR) after resuspension of the cell pellet in scintillation cocktail (Ultima-Gold). Bacterial production was calculated according to Kirchmann (1993), from 3H-leucine incorporation rates. Duplicate incubations had an analytical error <10%. Concentration kinetics optimization was also performed to ensure that the bacterial growth was not limited by the concentration of leucine.
Dinitrogen (N2) Fixation Rates
15N2 uptake measurements were performed using the 15N-enriched seawater protocol described by Mohr et al. (2010), with minor modifications for the EMS (Rahav et al., 2013). The detailed methodology is presented in Rahav et al. (2016a) this Special Issue.
Dissolved Trace Metal Analysis
The samples used for the analysis of trace metals (each 1 L in volume) were collected in PTFE bottles (pre-treated overnight with 2N HNO3 and rinsed afterwards with ultrapure Milli-Q water), and within 12 h from their collection the samples were filtered through pre-weighted nitrocellulose membrane filters (Millipore 0.45 μm pore size) under a clean laminar hood (class 100) in order to separate the particulate form from the dissolved form of the metals. The dissolved metals in the filtered samples were determined immediately after filtration through a process of pre-concentration accomplished by passing the sample through Chelex-100 resin columns for retaining the metals and by eluting them using 10 ml nitric acid 2N s.p. under the clean laminar hood (the pre-concentration factor = 100). The pre-concentration procedure is a slight modification (Scoullos et al., 2007) of the procedure proposed by Riley and Taylor (1968) and Kingston et al. (1978).
Trace metal concentrations (Cu, Pb, Zn, Mn, Al, Fe) in the eluates were determined by employing a Varian SpectrAA 200 Flame Atomic Absorption Spectrophotometer (FAAS) for Zn, Al and Fe and a Varian SpectrAA-640Z Graphite Furnace Atomic Absorption Spectrophotometer (GFAAS) with Zeeman background correction for Cu, Pb and Mn. The relative standard deviation (Sr = (S/χ) × 100) of the measurements that resulted from replicate (3–4) determinations and standard addition experiments was <5%.
Statistical Analyses
The tests of statistical significance was carried out using a one-way analysis of variance (ANOVA) followed by a Fisher LSD means comparison test. Prior to analyses, the ANOVA assumptions, namely the normality and the heterogeneity of variances of the data, were examined. These tests were used to compare between the controls and the different treatments (P < 0.05) at the conclusion of the mesocosm experiment or between the results measured here and those compiled from the literature (Figures 5, 6). All tests were performed using the XLSTAT.
Results
The Ambient Water and Initial Conditions
The characteristics of the ambient surface (10 m) water collected during the 8–9 of May north of the Island of Crete and the initial conditions of the experiment (1 day prior to the additions) were typical oligotrophic, representing the EMS offshore waters (Kress et al., 2014; Pitta et al., 2016) as well as other LNLC systems. All the measured state and rate parameters showed values typical of oligotrophic systems (Figure 3), which are consistent with previous observations of the spring conditions in the EMS (references in Figure 3). The chlorophyll a (chl-a) concentrations, primary production (PP) and bacterial production (BP) were at the lower range of the oligotrophic systems; 0.06 ± 0.00 μg l−1, 0.42 ± 0.02 μg C l−1 h−1, and 15.59 ± 7.57 ng C l−1 h−1, respectively. Bacterial abundance (BA, 4.2 × 105 cells ml−1), Synechococcus abundance (Syn., 2.3 × 104 cells ml−1) and dinitrogen fixation (N2-fix, 0.21 ± 0.01 nmol N l−1 d−1) were within the oligotrophic range. The phosphate and phosphate turnover time were also characteristic to oligotrophic conditions, 0.012 μM and ~1 h, respectively. The seawater temperature during the experiment was in the range of 19–20°C, with no marked stratification of the water column inside the mesocosms.
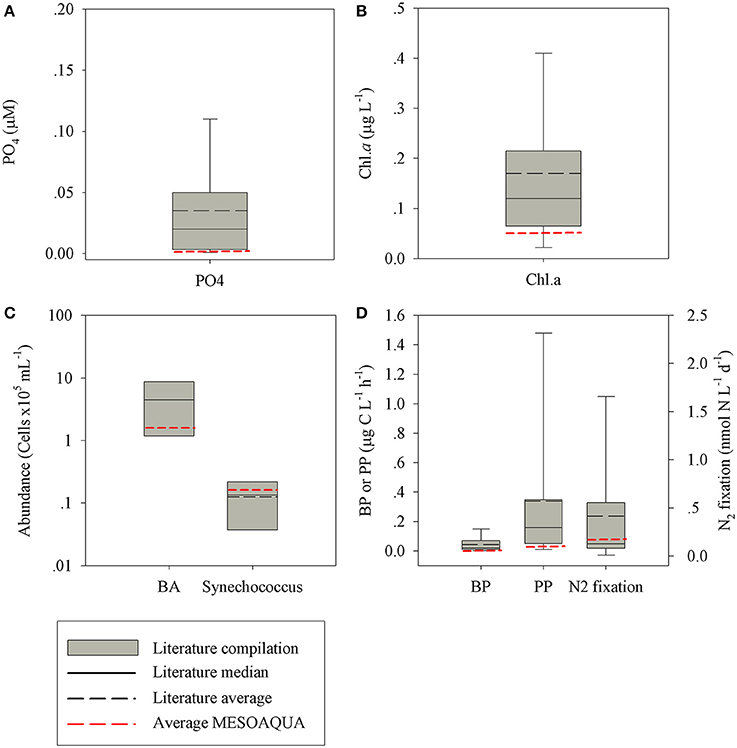
Figure 3. A box plot distribution of phosphorus (A), chl-a (B), microbial cell abundances (C), and microbial production rates (D), as reported for oligotrophic marine environments worldwide (Azov, 1986; Zohary and Robarts, 1998; Herut et al., 2000; Pinhassi and Hagström, 2000; Ignatiades et al., 2002; Wu et al., 2003; Krom et al., 2005; Pulido-Villena et al., 2008; Foster et al., 2009; Fernández et al., 2010; Christaki et al., 2011; Van Wambeke et al., 2011; Watkins-Brandt et al., 2011; Moisander et al., 2012; Rahav et al., 2013, 2015, 2016b; Tanhua et al., 2013; Kress et al., 2014; Keuter et al., 2015; Raveh et al., 2015; Tsiola et al., 2016). The black line represents the median (solid) and average (dashed) value. In red: the average values measured during this study (MESOAQUA).
The Chemical Composition and Nutrient Leachability of the Aerosols
The heavy metal concentrations were enriched in the SD compared to A, while the major element concentrations show similar values (Table 2). The Si/Al ratios were at the upper range found in northern African mineral dusts, while the Fe/Ca ratios were relatively low (Formenti et al., 2011, 2014). The latter indicate a certain depletion in Al and Ca compared to typical African mineral dust. The Mn/Fe, Pb/Fe, Ni/Fe, and Cd/Fe ratios were similar to the equivalent ratios in the African aerosols sampled by Chien et al. (2016), while the Cu/Fe ratios in this study are somewhat higher. The Mn/Fe and P/Fe ratios (wt./wt.) in SD were similar to the Saharan soil used by Guieu et al. (2010) (0.015 vs. 0.015 for Mn/Fe and 0.014 vs. 0.017 for P/Fe) and to those measured by Chien et al. (2016) in African aerosols. The P/Fe, Pb/Fe, Cd/Fe, and Cu/Fe ratios in A were higher than in SD by 2.2, 1.7, 2.2, and 1.75, respectively, indicating enrichment in these elements probably due to a larger anthropogenic fraction.
The amount of P leached was 2.1–2.7 and 2.2–3.7 nmol PO4 per mg of SD and A, respectively (Table 3; Figure S1), representing an addition of ~25–50% of the ambient concentrations. Similar P leached values were observed in other studies: 1.9 nmol phosphate per mg Saharan dust (Ridame and Guieu, 2002); 2.6–2.7 nmol phosphate per mg of Saharan dust (Chien et al., 2016); 4.2 nmol phosphate per mg of total suspended particles (TSP) sampled in the Red Sea (Mackey et al., 2012). The amount of nitrate leached was 20–26 and ~54 nmol NOx per mg of SD and A, respectively (Table 3; Figure S1). The average leached N and P resulted in a distinctly lower N:P ratio in treatment SD (~9:1) than in A (~18:1) or 7:1 vs. 15:1, considering the larger leachability of P by Leeds laboratory (Table 3). The higher leachable values of P obtained in Leeds compared to those retrieved in Haifa (~30 and ~75% for SD and A, respectively, Table 3; Figure S1) were probably attributed to the coating with iodine done in Leeds, which prevented adsorption into the walls of the containers. These leaching experiments were performed using sterile seawater, at different particle concentrations than those used in the mesocosm experiments. They also represent higher particle concentrations than those naturally found at the surface mixed layer after dust storms/aerosol depositions. Such differences, both in the seawater's biological reactivity and in the particle concentrations, may impact the amount of nutrient release (e.g., Ridame and Guieu, 2002; Mackey et al., 2012) and hence the leaching dynamics of N, P and other micronutrients. We therefore consider the experimental leaching amounts of N and P as an approximation of the total amount released in the mesocosms using the average values.
The turnover time of phosphorus (~1 h), regardless of the treatment, represents extreme oligotrophic P starved conditions. The P released from the dust/aerosols was probably immediately consumed, as no increase in Tt was recorded even 3 h after addition.
Dissolved Trace Metal Concentrations
The presented dissolved trace metal concentrations were measured in the initial conditions (prior to additions) and also 3 and 24 h after addition. The amount of trace metals added to the experimental mesocosm after the SD and A additions is presented in Table 4. Fast enrichment (3 h after addition) was observed for Mn in both the SD and A treatments compared to the initial conditions and the control. A relatively fast enrichment of Ni, Fe, and Pb was observed in the SD treatment. Such a fast release of trace metals coincides with the observations in other studies (Baker et al., 2006; Séguret et al., 2011). Generally, the dissolved concentrations of Mn, Ni, and Cu were similar to the values reported in Chien et al. (2016), while the Fe and Pb concentrations were enriched. The dissolved trace metal concentrations were similar to the range of values measured at the EMS offshore Israel (Figure 4).
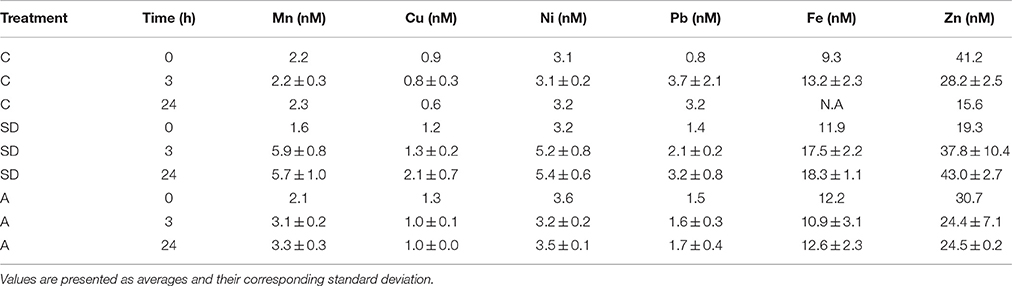
Table 4. Summary of the trace metal concentrations in the different mesocosms 24 h after the SD or A additions.
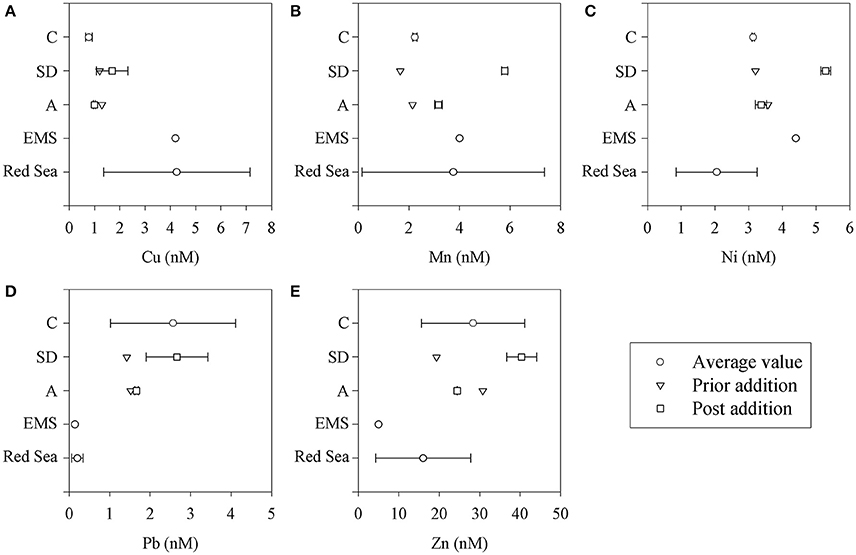
Figure 4. Dissolved trace metal concentrations (A-E) in the mesocosm tanks (C, SD, and A) and in representative oligotrophic stations in the eastern Mediterranean (EMS) and Red (northern Gulf of Aqaba) Seas (Herut et al., unpublished). Values are presented in nM.
Mn increased by ~4 nM following the SD and ~1 nM in the A treatments. This represents an increase by a factor of ~2 and 1.3 in the ambient concentrations, respectively. Ni increased by ~ 2 nM following the SD treatment. Pb and Fe increased by ~1 and ~6 nM in SD, respectively.
Biological Parameters
Both types of treatments triggered a positive change (relative to the unamended control mesocosms) in most of the performed rate and state measured parameters such as BA and BP, Syn abundance, chl-a, PP, and N2-fix. These changes are in agreement with other dust microcosm/mesocosm additions from the Mediterranean Sea and are presented as the maximal difference (treatment minus control, Figures 5A–E) or normalized to 1 mg l−1 of SD or A addition (Figures 6A,B). The added aerosol/dust concentrations (1 and 1.6 mg l−1) in our experiment fall within the linear dose-response range previously studied in the EMS (e.g., Herut et al., 2005; 0.2–4.9 mg l−1) and therefore supports a linear normalization. Overall, the maximal observed changes in the two treatments for the different parameters ranged from 25 to 660% per 1 mg l−1 of dust/aerosol addition (Figure 6A). For SD treatment, the maximal change (increase) was observed after 48 h for BP (98%, 11.8 ± 1.2 ng C l−1 h−1), chl-a (27%, 33.6 ± 3.3 ng l−1) and N2-fix (223%, 0.21 ± 0.03 nmol N l−1 d−1) and 72 h for PP (61%, 0.28 ± 0.05 μg C l−1 h−1) and Syn. (23%, 2.75 ± 0.64 μg C l−1). BA showed a progressive increase (from 25%; 2.78 ± 0.43 μg C l−1 after 24 h to 48% at day 8) along the experiment. For A treatment, an immediate maximal change (increase) was observed after 3 h for BP (660%, 24.2 ± 8.5 ng C l−1 h−1), after 1 day for chl-a (47%, 33.2 ± 3.8 ng l−1), after 2 days for PP (102%, 0.29 ± 0.08 μg C l−1 h−1) and N2-fix (141%, 0.09 ± 0.03 nmol N l−1 d−1) and after 3 days for Syn.(41%, 2.89 ± 0.89 μg C l−1). BA showed a progressive increase (from 39%; 2.53 ± 0.46 ng C l−1 after 24 h to 63% at day 8) along the experiment.
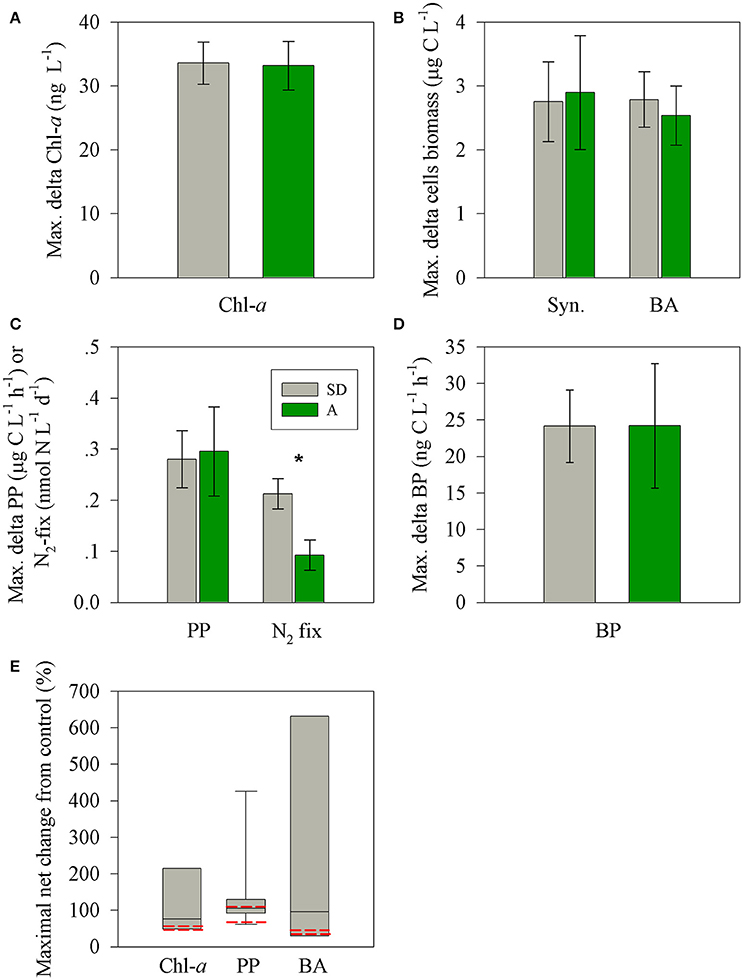
Figure 5. The observed maximal net changes of for the autotrophic and heterotrophic microbial variables (A–D) following SD (gray) or A (green) additions in the surface EMS during May 2012 and a box plot distribution of the net change reported for chl-a, PP, and BP in Mediterranean Sea studies. Data were taken from Herut et al. (2005); Ternon et al. (2011); Ridame et al. (2013); Rahav et al. (2016b)); Rahav et al., unpublished and this study (E). The red lines represents the observed values measured for SD and A during this study. The asterisks above the columns represent statistically significant differences (one-way ANOVA and a Fisher LSD means comparison test, P < 0.05) for mean values of P additions between stations.
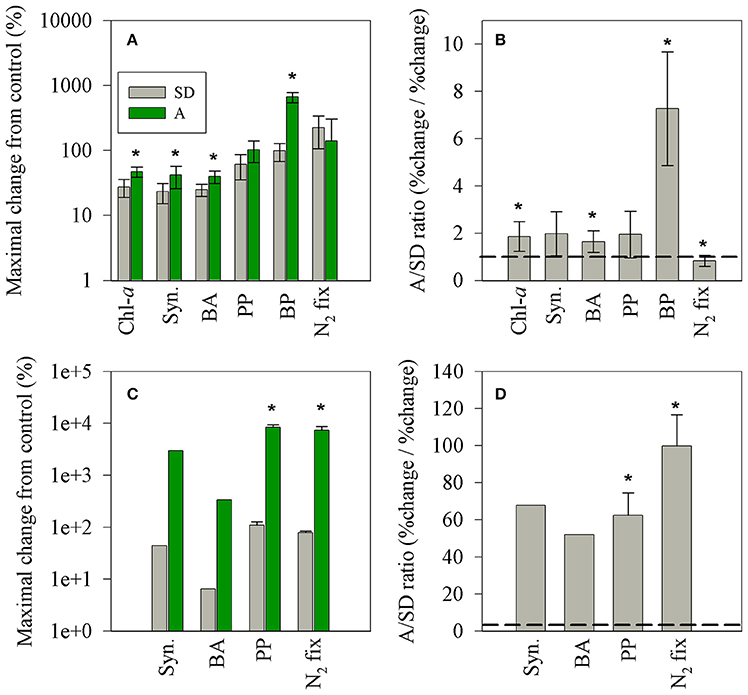
Figure 6. The maximal net changes (normalized to 1 mg l−1 dust) in autotrophic and heterotrophic microbial populations (A,C) and the A/SD ratio (B,D) measured in this study (A,B) and that reported in the BOUM campaign (Station B, Ternon et al., 2011, C,D). The black line represents a 1:1 ratio between A and SD values. The asterisks above the columns represent statistically significant differences (one-way ANOVA and a Fisher LSD means comparison test, P < 0.05).
Discussion
Here we assess the impact of pure Saharan dust vs. mixed aerosols on the surface seawater autotrophic and heterotrophic microbial populations, mimicking the potential effects of an intense Saharan dust storm and a relatively intense mixed aerosol deposition. While the EMS is exposed to a relative high frequency of Saharan and other desert dust storms (Koçak et al., 2004; Ganor et al., 2010), which will probably increase in the future due to climate change (Lelieveld et al., 2016), anthropogenic aerosols were found to be enriched in dust when it arrived at sampling sites after passing through populated and industrialized urban areas (Koçak et al., 2012). It has been observed that the anthropogenic component increased the trace metal content and changed their speciation in EMS aerosols (Kocak et al., 2010), and that such aerosols are enriched in nitrate compared to bioavailable phosphate (e.g., Chien et al., 2016). Therefore, any increase in anthropogenic aerosol deposition in the future and hence in the relative fraction of N deposition may influence the phytoplankton community structure in LNLC areas (Chien et al., 2016). Such a trend, of a relatively higher release of nitrate vs. phosphate, was observed here. While in the SD treatment more phosphate (by ~34%) was added to the mesocosms compared to the A treatment, the opposite trend was observed for nitrate. The A treatment added more nitrate (by ~40%) than the SD treatment. Consequently, the nitrate/phosphate ratios added in the A treatment were twice than those added in the SD treatment, 18 vs. 9 using the average or 15 vs. 7 considering a larger release of P (Table 3).
The phosphate turnover time prior to the additions of dust/aerosol was ~1 h, a typical value of P deficiency in the EMS (Zohary and Robarts, 1998; Flaten et al., 2005; Tanaka et al., 2011). The SD and A additions did not trigger an increase in the phosphate turnover time caused by the release of phosphate from the dust/aerosol. By definition, the Tt equals the amount of bioavailable phosphate divided by its consumption (or uptake) rate. This suggests that the amount of phosphate added to the system from the dust/aerosol through leaching was relatively small and that it was rapidly removed by the microbial community. Bioassays in which a significant amount of phosphate was released showed an increase in phosphate Tt (Herut et al., 2005; Tanaka et al., 2011).
The amount of leachable phosphate and nitrate in the SD treatment, 3.8 and ~23 nM, respectively, corresponds to a potential increase of ~58 ng chl-a l−1 by using P:C ratios or ~55 ng chl a l−1 by using N:C ratios of Redfield (1:16:106) and C:chl a ≈ 80 (wt., Behrenfeld et al., 2005). The observed chl-a increase is somewhat lower, ~30 ng chl-a l−1. However, the highest bacterial production rate was ~3.3 nmol C l−1 h−1, at 24 h after addition, which corresponds to ~1.6 nmol P l−1d−1, using a C/P ratio of 50 for bacteria (Fagerbakke et al., 1996), although the latter is not constant (Zimmerman et al., 2014; Godwin and Cotner, 2015). Assuming that out of the released phosphate ~ 1.6 nM was used by the bacteria and 2.2 nM was used by the autotrophs, the latter corresponds to the observed increase in chl-a (~30 ng l−1). The lower observed chl-a values may also infer some grazing activity. A similar calculation for the A treatment shows a similar result, which corresponds to the observed increase in chl-a.
Additional trace metals, micronutrients (Fe, Zn, Mn, Co-not measured) or potentially toxic metals that have possibly been delivered by the dust were not assessed via leaching experiments in this study. However, these were measured 3 h and 1 day after the additions (Table 4), showing values similar to an open remote EMS station (100 km offshore Israel), to the Red Sea (Herut et al., unpublished) or, for part of the elements, to the mean ocean concentration, although the Mn, Fe and Zn values were significantly higher (Statham and Hart, 2005; Semeniuk et al., 2009; Moore et al., 2013).
The SD treatments were relatively enriched (p < 0.05) in dissolved Mn, Ni, and Fe concentrations compared to the A mesocosms, probably due to the larger mass addition (1.6 mg l−1 vs. 1 mg l−1). All the measured dissolved metal concentrations were well below the ecological ambient water quality criteria for chronic levels (Buchman, 2008). Metal bioavailability may determine phytoplankton productivity, especially by iron, copper, cobalt, zinc and nickel, via scarcity or toxic effects (Huertas et al., 2014). Their specific impact, including manganese, on biological processes is mainly related to the metalloproteins state (Cvetkovic et al., 2010). The enrichment of Mn in SD and A treatments as compared to C (p < 0.05) may serve as a micronutrient supporting photosynthesis (Huertas et al., 2014) and/or may reverse toxicity effects of Cu and Zn (Sunda, 1987). Although the bioavailability of the measured dissolved Fe is not certain (Rue and Bruland, 1995), the amount is high enough to exclude possible Fe limitation (e.g., Statham and Hart, 2005). Assuming that particulate Fe dissolution in seawater is ~0.06% (0.03–0.17%, Blain et al., 2004; Chien et al., 2016), a maximum amount of ~50 nmol Fe l−1 (900 nmol particulate Fe l−1 × 0.06%) was released from the SD addition, larger than in the A treatment (~24 nmol Fe l−1), and probably supplying enough bioavailable Fe2+. The significantly higher (p = 0.02) N2-fix rates measured in the SD treatment (Rahav et al., 2016b) may be related to a relatively larger release of Fe in this treatment and its relatively lower nitrate/phosphate ratio (~10:1). In addition, the significantly (p < 0.05) higher Ni concentrations in SD as compared to A or C, is known to play an important role in the cellular physiology of diazotrophic cyanobacteria such as Trichodesmium (Rodriguez and Ho, 2014) and supports the latter observation and the appearance of this N2-fixer in the SD treatment (Rahav et al., 2016b).
Both types of dry aerosol additions (SD and A) triggered a positive change (25–600% per 1 mg/L addition) in all rate and state measurements (Figures 5, 6), showing a general sequence (p < 0.05) of higher relative change in the rate parameters: N2-fix > BP > PP > Chla ≈ BA ≈ Syn for SD and BP > PP ≈ N2-fix > Chla ≈ BA ≈ Syn for A. Nevertheless, a larger normalized increase was observed in treatment A for all parameters, except for N2-fix (Figure 6B). In addition, the BP dramatically increased within 3 h after addition (~600%, yet reached 154% after 24 h), while no such response was observed for SD. The primary differences between the two treatments is the leachable molar ratio of nitrate/phosphate; almost twice in treatment A (~15:1) than SD (~9:1), and the additional amount of added leachable N by treatment A (~30% more than SD) (Table 3). No significant changes were observed after additions and between treatments in the dissolved organic nutrients, not likely to dominate the observed change in the heterotrophic bacterial activity. These differences in the relative impact (normalized to mass), between pure mineral/desert dust and mixed aerosols, were also observed by Ternon et al. (2011) for the addition of a dust analog (manipulated Saharan soil) and aerosols (total suspended material in air) collected by low volume sampler at sea (Figures 6C,D). While in the latter on-board experiment lower quantities of P were release by the aerosols (0.3–1.6 nmol P l−1) as compared to the Saharan analog (6 nmol P l−1), in our experiment similar amounts of P were added by A and SD, but larger quantities of N were added in A (Table 3). Yet, only the inorganic phase of nutrients was considered here, whereas the dissolved organic pools likely to be present in aerosols (e.g., Markaki et al., 2010) were not measured and should be further considered. Apart from the increased solubility in aerosols, the smaller size particles as compared to crustal mineral aerosols, increases the particle's surface/volume ratio and solubility (Baker and Jickells, 2006), implying a larger impact per mass of a mixed character as A treatment. Our data suggest that despite the co-limitation of P and N (Kress et al., 2005; Zohary et al., 2005), the additional N released by the A treatment may have triggered the relative larger response in most of the rate and state parameters. A similar observation was reported for the DUNE experiment in the Western Mediterranean Sea (Ridame et al., 2014) and was calculated for the data presented during the BOUM campaign across the Mediterranean Sea in which aerosol addition triggered stronger responses of the microbial community than Saharan dust (Tanaka et al., 2011; Ternon et al., 2011). Yet, the magnitude of the maximal net effect imposed by SD or A on the surface microbial populations (% change normalized to 1 mg l−1) is significantly lower than that reported from a microcosm experiment in the Ionian Sea during the BOUM campaign in summer 2008 (by ~1 order of magnitude, Figure 6C, Ternon et al., 2011). When normalizing the responses from the BOUM study, Saharan analog or aerosols addition resulted in a general sequence of PP ≈ N2-fix ≈ Syn. > BA. Furthermore, the overall responses triggered by the aerosol addition in the BOUM campaign (microbial biomass and activity) were much higher than that reported for the Saharan analog amendments. This results in a high calculated aerosol/Saharan analog ratios in the BOUM study, ranging from ~50 to 100% (Figure 6D). These different responses by different aerosols should be further studied in detail considering all bioavailable macro and micro nutrients or other inhibiting elements.
The role atmospheric deposition plays in influencing bacterioplankton dynamics in LNLC regions is important for understanding (observationally and via ocean biogeochemical models) the current and future functioning of LNLC regions (Guieu et al., 2014a). Increasing temperatures leading to increased dust emissions in the EMS (Klingmüller et al., 2016) and anthropogenic activities contributing to the Mediterranean atmospheric chemical composition and aerosols solubility (exposure to lower pH levels during transport), are expected to continue in the future due to climate change, and thereby supply more macro and micro nutrients into its surface oligotrophic water. A warmer atmosphere may influence the intensity and length of the already well stratified water column in the EMS and hence the impact of the atmosphere as a significant external source of new macro and micro nutrients. We show here that the above trends may lead to the alteration of the heterotrophic/autotrophic relationships.
Author Contributions
Planning of the original experimental design and carrying out of experiment and sampling in the field (BH, PP, TMT, TBM). Aerosols collection (NM, BH, CNT). Aerosols chemical composition (BH). Heavy metals (ES, MS). Leaching rate of nutrients from dust (BH, ER, MDK, AS, KV, ZS). Phosphorous turnover time (TT). Chlorophyll a concentration (NP). Primary production (AL, SP). Synechococcus abundance and biomass (AT). Bacterial abundance, biomass and rate (AT, AG). Nitrogen fixation (ER, IBF, BH). Statistics (ER). Organizing the operation of mesocosm and obtaining funding (PP, TMT). Writing up manuscript (BH, ER).
Conflict of Interest Statement
The authors declare that the research was conducted in the absence of any commercial or financial relationships that could be construed as a potential conflict of interest.
Acknowledgments
This work was financed by the European Union Seventh Framework Program (FP7/2007-2013) under grant agreement no. 228224, “MESOAQUA: Network of leading MESOcosm facilities to advance the studies of future AQUAtic ecosystems from the Arctic to the Mediterranean” through grants to BH, IBF, ER, ZS, and MDK. The authors wish to thank G. Piperakis for his technical assistance, A. Konstantinopoulou for assistance with bacterial production analyses, D. Podaras and S. Diliberto for assistance during the experiment and N. Sekeris for his help with constructions and ideas on technical solutions. The captain and the crew of the R/V Philia are also thanked for their assistance during the transportation of water from the sea to the CRETACOSMOS facility. Funding was also provided by the Israel Science Foundation grants (996/08) to IBF and BH. Funding was also provided by Leverhulme Trust (grant RPG 406) to MDK.
Supplementary Material
The Supplementary Material for this article can be found online at: http://journal.frontiersin.org/article/10.3389/fmars.2016.00226/full#supplementary-material
Figure S1. Leached nitrate (A) and phosphate (B) from the SD (circle) and A (triangle) particles as retrieved in the Leeds (white) and Haifa (red) laboratories.
References
Astitha, M., Lelieveld, J., Abdel Kader, M., Pozzer, A., and De Meij, A. (2012). Parameterization of dust emissions in the global atmospheric chemistry-climate model EMAC: impact of nudging and soil properties. Atmos. Chem. Phys. 12, 11057–11083. doi: 10.5194/acp-12-11057-2012
ASTM (1983). Designation - D3683-78. Standard Test Method for Trace Elements in Coal and Coke Ash by Atomic Absorption. Pennsylvania, PA: American Society for Testing and Materials Publisher.
Azov, Y. (1986). Seasonal patterns of phytoplankton productivity and abundance in nearshore oligotrophic waters of the Levant Basin (Mediterranean). J. Plankton Res. 8, 41–53. doi: 10.1093/plankt/8.1.41
Baker, A. R., French, M., and Linge, K. L. (2006). Trends in aerosol nutrient solubility along a west-east transect of the Saharan dust plume. Geophys. Res. Lett. 33, 10–13. doi: 10.1029/2005GL024764
Baker, A. R., and Jickells, T. D. (2006). Mineral particle size as a control on aerosol iron solubility. Geophys. Res. Lett. 33, 1–4. doi: 10.1029/2006GL026557
Behrenfeld, M. J., Boss, E., Siegel, D. A., and Shea, D. M. (2005). Carbon-based ocean productivity and phytoplankton physiology from space. Global Biogeochem. Cycles 19, 1–14. doi: 10.1029/2004GB002299
Blain, S., Guieu, C., Claustre, H., Leblanc, K., Moutin, T., Guiner, B. Q., et al. (2004). Availability of iron and major nutrients for phytoplankton in the north-east Atlantic Ocean. Limnol. Oceanogr. 49, 2095–2104. doi: 10.4319/lo.2004.49.6.2095
Bougiatioti, A., Nikolaou, P., Stavroulas, I., Kouvarakis, G., Weber, R., Nenes, A., et al. (2016). Particle water and pH in the eastern Mediterranean: source variability and implications for nutrient availability. Atmos. Chem. Phys. 16, 4579–4591. doi: 10.5194/acp-16-4579-2016
Bressac, M., Guieu, C., Doxaran, D., Bourrin, F., Desboeufs, K., Leblond, N., et al. (2014). Quantification of the lithogenic carbon pump following a simulated dust-deposition event in large mesocosms. Biogeosciences 11, 1007–1020. doi: 10.5194/bg-11-1007-2014
Buchman, M. F. (2008). NOAA Screening Quick Reference Tables, Office of Response and Restoration Division. Seattle, WA: National Oceanic and Atmospheric Administration.
Calvo-Díaz, A., Díaz-Pérez, L., Suárez, L. Á., Morán, X. A. G., Teira, E., and Marañón, E. (2011). Decrease in the autotrophic-to-heterotrophic biomass ratio of picoplankton in oligotrophic marine waters due to bottle enclosure. Appl. Environ. Microbiol. 77, 5739–5746. doi: 10.1128/AEM.00066-11
Campbell, J. W., and Yentsch, C. M. (1989). variance within homogeneous phytoplankton populations, III: analysis of natural populations. Cytometry 10, 605–611. doi: 10.1002/cyto.990100516
Chien, C., Mackey, K. R., Dutkiewicz, S., Mahowald, N. M., Prospero, J. M., and Paytan, A. (2016). Effects of African dust deposition on phytoplankton in the western tropical atlantic Ocean off Barbados. Global Biogeochem. Cycles 30, 716–734. doi: 10.1002/2015GB005334
Christaki, U., Van Wambeke, F., Lefevre, D., Lagaria, A., Prieur, L., Pujo-Pay, M., et al. (2011). Microbial food webs and metabolic state across oligotrophic waters of the Mediterranean Sea during summer. Biogeosciences 8, 1839–1852. doi: 10.5194/bg-8-1839-2011
Cvetkovic, A., Menon, A. L., Thorgersen, M. P., Scott, J. W., Poole, F. L., Jenney, F. E. Jr., et al. (2010). Microbial metalloproteomes are largely uncharacterized. Nature 466, 779–782. doi: 10.1038/nature09265
Duce, R. A., LaRoche, J., Altieri, K., Arrigo, K. R., Baker, A. R., Capone, D. G., et al. (2008). Impacts of atmospheric anthropogenic nitrogen on the open ocean. Science 320, 893–897. doi: 10.1126/science.1150369
Duce, R. A., Liss, P. S., Merrill, J. T., Atlas, E. L., Buat-Menard, P., Hicks, B. B., et al. (1991). The atmospheric input of trace species to the world ocean. Global Biogeochem. Cycles 5, 193–259. doi: 10.1029/91GB01778
Fagerbakke, K. M., Heldal, M., and Norland, S. (1996). Content of carbon, nitrogen, oxygen, sulfur and phosphorus in native aquatic and cultured bacteria. Aquat. Microb. Ecol. 10, 15–27. doi: 10.3354/ame010015
Fernández, A., Mouriño-Carballido, B., Bode, A., et al. (2010). Latitudinal distribution of Trichodesmium spp. and N2 fixation in the Atlantic Ocean. Biogeosciences 7, 2195–2225. doi: 10.5194/bgd-7-2195-2010
Flaten, G. A., Skjoldal, E. F., Krom, M. D., Law, C. S., Mantoura, R. F. C., Pitta, P., et al. (2005). Studies of the microbial P-cycle during a Lagrangian phosphate-addition experiment in the Eastern Mediterranean. Deep Sea Res. II Top. Stud. Oceanogr. 52, 2928–2943. doi: 10.1016/j.dsr2.2005.08.010
Formenti, P., Caquineau, S., Desboeufs, K., Klaver, A., Chevaillier, S., Journet, E., et al. (2014). Mapping the physico-chemical properties of mineral dust in western Africa: mineralogical composition. Atmos. Chem. Phys. 14, 10663–10686. doi: 10.5194/acp-14-10663-2014
Formenti, P., Schütz, L., Balkanski, Y., Desboeufs, K., Ebert, M., Kandler, K., et al. (2011). Recent progress in understanding physical and chemical properties of African and Asian mineral dust. Atmos. Chem. Phys. 11, 8231–8256. doi: 10.5194/acp-11-8231-2011
Foster, R., Paytan, A., and Zehr, J. P. (2009). Seasonality of N2 fixation and nifH gene diversity in the Gulf of Aqaba (Red Sea). Limnol. Oceanogr. 54, 219–233. doi: 10.4319/lo.2009.54.1.0219
Ganor, E., Osetinsky, I., Stupp, A., and Alpert, P. (2010). Increasing trend of African dust, over 49 years, in the eastern Mediterranean. J. Geophys. Res. Atmos. 115, 1–7. doi: 10.1029/2009jd012500
Godwin, C. M., and Cotner, J. B. (2015). Aquatic heterotrophic bacteria have highly flexible phosphorus content and biomass stoichiometry. ISME J. 9, 2324–2327. doi: 10.1038/ismej.2015.34
Guieu, C., Aumont, O., Paytan, A., Bopp, L., Law, C. S., Mahowald, N., et al. (2014a). The significance of the episodic nature of atmospheric deposition to Low Nutrient Low Chlorophyll regions. Global Biogeochem. Cycles 28, 1179–1198. doi: 10.1002/2014GB004852
Guieu, C., Dulac, F., Desboeufs, K., Wagener, T., Pulido-Villena, E., Grisoni, J. M., et al. (2010). Large clean mesocosms and simulated dust deposition: a new methodology to investigate responses of marine oligotrophic ecosystems to atmospheric inputs. Biogeosciences 7, 2765–2784. doi: 10.5194/bg-7-2765-2010
Guieu, C., Dulac, F., Ridame, C., and Pondaven, P. (2014b). Introduction to project DUNE, a DUst experiment in a low nutrient, low chlorophyll ecosystem. Biogeosciences 11, 425–442. doi: 10.5194/bg-11-425-2014
Guo, C., Xia, X., Pitta, P., Herut, B., Rahav, E., Berman-Frank, I., et al. (2016). Shifts in microbial community structure and activity in the ultra-oligotrophic Eastern Mediterranean Sea driven by the deposition of Saharan dust and European aerosols. Front. Mar. Sci. 3:170. doi: 10.3389/fmars.2016.00170
Guo, C., Yu, J., Ho, T. Y., Wang, L., Song, S., Kong, L., et al. (2012). Dynamics of phytoplankton community structure in the South China Sea in response to the East Asian aerosol input. Biogeosciences 9, 1519–1536. doi: 10.5194/bg-9-1519-2012
Herut, B., Almogi-Labin, A., and Jannink, N. (2000). The seasonal dynamics of nutrient and chlorophyll a concentrations on the SE Mediterranean shelf-slope. Oceanol Acta 23, 771–782. doi: 10.1016/S0399-1784(00)01118-X
Herut, B., Collier, R., and Krom, M. D. (2002). The role of dust in supplying nitrogen and phosphorus to the Southeast Mediterranean. Limnol. Oceanogr. 47, 870–878. doi: 10.4319/lo.2002.47.3.0870
Herut, B., Krom, M. D., Pan, G., and Mortimer, R. (1999). Atmospheric input of nitrogen and phosphorus to the Southeast Mediterranean: sources, fluxes, and possible impact. Limnol. Oceanogr. 44, 1683–1692. doi: 10.4319/lo.1999.44.7.1683
Herut, B., Nimmo, M., Medway, A., Chester, R., and Krom, M. D. (2001). Dry atmospheric inputs of trace metals at the Mediterranean coast of Israel (SE Mediterranean): sources and fluxes. Atmos. Environ. 35, 803–813. doi: 10.1016/S1352-2310(00)00216-8
Herut, B., Zohary, T., Krom, M. D., Mantoura, R. F. C., Pitta, P., Psarra, S., et al. (2005). Response of East Mediterranean surface water to Saharan dust: on-board microcosm experiment and field observations. Deep Sea Res. II Top. Stud. Oceanogr. 52, 3024–3040. doi: 10.1016/j.dsr2.2005.09.003
Hoerling, M., Eischeid, J., Perlwitz, J., Quan, X., Zhang, T., and Pegion, P. (2012). On the increased frequency of mediterranean drought. J. Clim. 25, 2146–2161. doi: 10.1175/JCLI-D-11-00296.1
Holm-Hansen, O., Lorenzen, C. J., Holmes, R. W., and Strickland, J. D. H. (1965). Fluorometric determination of chlorophyll. ICES J. Mar. Sci. 30, 3–15. doi: 10.1093/icesjms/30.1.3
Huertas, M. J., López-Maury, L., Giner-Lamia, J., et al. (2014). Metals in cyanobacteria: analysis of the copper, nickel, cobalt and arsenic homeostasis mechanisms. Life 4, 865–886. doi: 10.3390/life4040865
Ignatiades, L., Psarra, S., Zervakis, V., et al. (2002). Phytoplankton size-based dynamics in the Aegean Sea (Eastern Mediterranean). J. Mar. Syst. 36, 11–28. doi: 10.1016/S0924-7963(02)00132-X
IPCC (2014). Intergovernmental Panel on Climate Change, Climate Change 2013: The Physical Science Basis. Cambridge: Cambridge University Press.
Jickells, T. D., An, Z. S., Andersen, K. K., Baker, A. R., Bergametti, G., Brooks, N., et al. (2005). global iron connections between desert dust, ocean biogeochemistry, and climate. Science 308, 67–71. doi: 10.1126/science.1105959
Jordi, A., Basterretxea, G., Tovar-Sánchez, A., Alastuey, A., and Querol, X. (2012). Copper aerosols inhibit phytoplankton growth in the Mediterranean Sea. Proc. Natl. Acad. Sci. U.S.A. 109, 21246–21249. doi: 10.1073/pnas.1207567110
Kanakidou, M., Duce, R. A., Prospero, J. M., Baker, A. R., Benitez-Nelson, C., Dentener, F. J., et al. (2012). Atmospheric fluxes of organic N and P to the global ocean. Global Biogeochem. Cycles 26, 1–12. doi: 10.1029/2011GB004277
Keuter, S., Rahav, E., Herut, B., and Rinkevich, B. (2015). Distribution patterns of bacterioplankton in the oligotrophic south-eastern Mediterranean Sea. FEMS Microbiol. 91, 1–39. doi: 10.1093/femsec/fiv070
Kingston, H. M., Barnes, I. L., Brady, T. J., Rains, T. C., and Champ, M. A. (1978). Separation of eight transition elements from alkali and alkaline earth elements in estuarine and seawater with chelating resin and their determination by graphite furnace atomic absorption spectrometry. Anal. Chem. 50, 2064–2070. doi: 10.1021/ac50036a031
Kirchmann, D. L. (1993). “Leucine incorporation as a measure of biomass production by heterotrophic bacteria,” in Handbook of Methods in Aquatic Microbial Ecology, eds P. F. Kemp, B. F. Sherr, E. B. Sherr, and J. J. Cole (Boca Raton, FL: Lewis), 509–512.
Kirchmann, D. L., Newell, S. Y., and Hodson, R. E. (1986). Incorporation versus biosynthesis of leucine: implications for measuring rates of protein syntheis and biomass production by bacteria in marine systems. Mar. Ecol. Prog. Ser. 32, 47–59. doi: 10.3354/meps032047
Klingmüller, K., Pozzer, A., Metzger, S., Stenchikov, G. L., and Lelieveld, J. (2016). Aerosol optical depth trend over the Middle East. Atmos. Chem. Phys. 16, 5063–5073. doi: 10.5194/acp-16-5063-2016
Koçak, M., Kubilay, N., and Mihalopoulos, N. (2004). Ionic composition of lower tropospheric aerosols at a Northeastern Mediterranean site: implications regarding sources and long-range transport. Atmos. Environ. 38, 2067–2077. doi: 10.1016/j.atmosenv.2004.01.030
Kocak, M., Kubilay, N., Tuǧrul, S., and Mihalopoulos, N. (2010). Atmospheric nutrient inputs to the northern levantine basin from a long-term observation: sources and comparison with riverine inputs. Biogeosciences 7, 4037–4050. doi: 10.5194/bg-7-4037-2010
Koçak, M., Theodosi, C., Zarmpas, P., Séguret, M. J. M., Herut, B., Kallos, G., et al. (2012). Influence of mineral dust transport on the chemical composition and physical properties of the Eastern Mediterranean aerosol. Atmos. Environ. 57, 266–277. doi: 10.1016/j.atmosenv.2012.04.006
Kress, N., Frede Thingstad, T., Pitta, P., Psarra, S., Tanaka, T., Zohary, T., et al. (2005). Effect of P and N addition to oligotrophic Eastern Mediterranean waters influenced by near-shore waters: a microcosm experiment. Deep Sea Res. II Top. Stud. Oceanogr. 52, 3054–3073. doi: 10.1016/j.dsr2.2005.08.013
Kress, N., Gertman, I., and Herut, B. (2014). Temporal evolution of physical and chemical characteristics of the water column in the easternmost Levantine Basin (Eastern Mediterranean Sea) from 2002 to 2010. J. Mar. Syst. 135, 6–13. doi: 10.1016/j.jmarsys.2013.11.016
Krom, M. D., Herut, B., and Mantoura, R. F. C. (2004). Nutrient budget for the Eastern Mediterranean: implications for phosphorus limitation. Limnol. Oceanogr. 49, 1582–1592. doi: 10.4319/lo.2004.49.5.1582
Krom, M. D., Shi, Z., Stockdale, A., Berman-Frank, I., Giannakourou, A., Herut, B., et al. (2016). Response of the Eastern mediterranean microbial ecosystem to dust and dust affected by acid processing in the atmosphere. Front. Mar. Sci. 3:133. doi: 10.3389/fmars.2016.00133
Krom, M. D., Thingstad, T. F., Brenner, S., et al. (2005). Summary and overview of the CYCLOPS P addition Lagrangian experiment in the Eastern Mediterranean. Deep Sea Res Part II Top. Stud. Oceanogr. 52, 3090–3108. doi: 10.1016/j.dsr2.2005.08.018
Lagaria, A., Psarra, S., Lefèvre, D., et al. (2011). The effects of nutrient additions on particulate and dissolved primary production and metabolic state in surface waters of three Mediterranean eddies. Biogeosciences 8, 2595–2607. doi: 10.5194/bg-8-2595-2011
Lelieveld, J., Hadjinicolaou, P., Kostopoulou, E., Chenoweth, J., El Maayar, M., Giannakopoulos, C., et al. (2012). Climate change and impacts in the Eastern Mediterranean and the Middle East. Clim. Change 114, 667–687. doi: 10.1007/s10584-012-0418-4
Lelieveld, J., Lelieveld, J., Berresheim, H., Borrmann, S., Crutzen, P. J., Dentener, F. J., et al. (2002). Global air pollution crossroads over the mediterranean.pdf. Science 298, 794–799. doi: 10.1126/science.1075457
Lelieveld, J., Proestos, Y., Hadjinicolaou, P., Tanarhte, M., Tyrlis, E., and Zittis, G. (2016). Strongly increasing heat extremes in the Middle East and North Africa (MENA) in the 21st century. Clim. Change 137, 1–16. doi: 10.1007/s10584-016-1665-6
Mackey, K. R., Chien, C., Te Post, A. F., Saito, M. A., and Paytan, A. (2015). Rapid and gradual modes of aerosol trace metal dissolution in seawater. Front. Microbiol. 5:794. doi: 10.3389/fmicb.2014.00794
Mackey, K. R., Roberts, K., Lomas, M. W., Saito, M. A., Post, A. F., and Paytan, A. (2012). Enhanced solubility and ecological impact of atmospheric phosphorus deposition upon extended seawater exposure. Environ. Sci. Technol. 46, 10438–10446. doi: 10.1021/es3007996
Markaki, Z., Loýe-Pilot, M. D., Violaki, K., Benyahya, L., and Mihalopoulos, N. (2010). Variability of atmospheric deposition of dissolved nitrogen and phosphorus in the Mediterranean and possible link to the anomalous seawater N/P ratio. Mar. Chem. 120, 187–194. doi: 10.1016/j.marchem.2008.10.005
Mills, M. M., Ridame, C., Davey, M., La Roche, J., and Geider, R. J. (2004). Iron and phosphorus co-limit nitrogen fixation in the eastern tropical North Atlantic. Nature 429, 292–294. doi: 10.1038/nature02550
Mohr, W., Großkopf, T., Wallace, D. W. R., and Laroche, J. (2010). Methodological underestimation of oceanic nitrogen fixation rates. PLoS ONE 49:e12583. doi: 10.1371/journal.pone.0012583
Moisander, P. H., Zhang, R., Boyle, E. A., et al. (2012). Analogous nutrient limitations in unicellular diazotrophs and Prochlorococcus in the South Pacific Ocean. ISME J. 6, 733–744. doi: 10.1038/ismej.2011.152
Moore, C. M., Mills, M. M., Arrigo, K. R., Berman-Frank, I., Bopp, L., Boyd, P. W., et al. (2013). Processes and patterns of oceanic nutrient limitation. Nature Geosci 6, 701–710. doi: 10.1038/ngeo1765
Nenes, A., Krom, M. D., Mihalopoulos, N., Van Cappellen, P., Shi, Z., Bougiatioti, A., et al. (2011). Atmospheric acidification of mineral aerosols: a source of bioavailable phosphorus for the oceans. Atmos. Chemi. Phys. 11, 6265–6272. doi: 10.5194/acp-11-6265-2011
Ozer, T., Gertman, I., Kress, N., Silverman, J., and Herut, B. (in press). Interannual thermohaline (1979-2014) nutrient (2002-2014) dynamics in the Levantine surface intermediate water masses, SE Mediterranean Sea. Global Planet. Change 1–8. doi: 10.1016/j.gloplacha.2016.04.001
Paytan, A., Mackey, K. R., Chen, Y., Lima, I. D., Doney, S. C., Mahowald, N., et al. (2009). Toxicity of atmospheric aerosols on marine phytoplankton. Proc. Natl. Acad. Sci. U.S.A. 106, 4601–4605. doi: 10.1073/pnas.0811486106
Pinhassi, J., and Hagström, Å. (2000). Seasonal succession in marine bacterioplankton. Aquat. Microb. Ecol. 21, 245–256. doi: 10.3354/ame021245
Pitta, P., Nejstgaard, J. C., Tsagaraki, T. M., Zervoudaki, S., Egge, J. K., Frangoulis, C., et al. (2016). Confirming the “Rapid phosphorus transfer from microorganisms to mesozooplankton in the Eastern Mediterranean Sea” scenario through a mesocosm experiment. J. Plankton Res. 38, 502–521. doi: 10.1093/plankt/fbw010
Pulido-Villena, E., Baudoux, A.-C., Obernosterer, I., Landa, M., Caparros, J., Catala, P., et al. (2014). Microbial food web dynamics in response to a Saharan dust event: results from a mesocosm study in the oligotrophic Mediterranean Sea. Biogeosciences 11, 337–371. doi: 10.5194/bgd-11-337-2014
Pulido-Villena, E., Wagener, T., and Guieu, C. (2008). Bacterial response to dust pulses in the western Mediterranean: implications for carbon cycling in the oligotrophic ocean. Global Biogeochem. Cycles 22, 1–12. doi: 10.1029/2007GB003091
Rahav, E., Bar-Zeev, E., Ohayon, S., Elifantz, H., Belkin, N., Herut, B., et al. (2013). Dinitrogen fixation in aphotic oxygenated marine environments. Front. Microbiol. 4:227. doi: 10.3389/fmicb.2013.00227
Rahav, E., Herut, B., Mulholland, M., Belkin, N., Elifantz, H., and Berman-Frank, I. (2015). Heterotrophic and autotrophic contribution to dinitrogen fixation in the Gulf of Aqaba. Mar. Ecol. Prog. Ser. 522, 67–77. doi: 10.3354/meps11143
Rahav, E., Paytan, A., Chien, C., Ovadia, G., Katz, T., and Herut, B. (2016a). The impact of atmospheric dry deposition associated microbes on the southeastern Mediterranean Sea surface water following an intense dust storme. Front. Mar. Sci. 3:127. doi: 10.3389/fmars.2016.00127
Rahav, E., Shun-Yan, C., Cui, G., Liu, H., Tsagaraki, T. M., Giannakourou, A., et al. (2016b). Evaluating the impact of atmospheric depositions on springtime dinitrogen fixation in the Cretan Sea (Eastern Mediterranean)—a mesocosm approach. Front. Mar. Sci. 3:180. doi: 10.3389/fmars.2016.00180
Raveh, O., David, N., Rilov, G., and Rahav, E. (2015). The temporal dynamics of coastal phytoplankton and bacterioplankton in the Eastern Mediterranean Sea. PLoS ONE 10:e0140690. doi: 10.1371/journal.pone.0140690
Ridame, C., Dekaezemacker, J., Guieu, C., Bonnet, S., L'Helguen, S., and Malien, F. (2014). Contrasted Saharan dust events in LNLC environments: impact on nutrient dynamics and primary production. Biogeosciences 11, 4783–4800. doi: 10.5194/bg-11-4783-2014
Ridame, C., and Guieu, C. (2002). Saharan input of phosphate to the oligotrophic water of the open western Mediterranean Sea. Limnol. Oceanogr. 47, 856–869. doi: 10.4319/lo.2002.47.3.0856
Ridame, C., Guieu, C., and L'Helguen, S. (2013). Strong stimulation of N2 fixation in oligotrophic Mediterranean Sea: results from dust addition in large in situ mesocosms. Biogeosciences 10, 7333–7346. doi: 10.5194/bg-10-7333-2013
Riley, J. P., and Taylor, D. (1968). Chelating resins for the concentration of trace elements from sea water and their analytical use in conjunction with atomic absorption spectrophotometry. Anal. Chim. Acta 40, 479–485. doi: 10.1016/S0003-2670(00)86764-1
Rimmelin, P., and Moutin, T. (2005). Re-examination of the MAGIC method to determine low orthophosphate concentration in seawater. Anal. Chim. Acta 548, 174–182. doi: 10.1016/j.aca.2005.05.071
Rodriguez, I. B., and Ho, T.-Y. (2014). Diel nitrogen fixation pattern of Trichodesmium: the interactive control of light and Ni. Sci. Rep. 4:4445. doi: 10.1038/srep04445
Rue, E. L., and Bruland, K. W. (1995). Complexation of iron(lll) by natural organic ligands in the Central North Pacific as determined by a new competitive ligand equilibration/adsorptive cathodic stripping voltammetric method. Mar. Chem. 5, 117–138. doi: 10.1016/0304-4203(95)00031-L
Scoullos, M. J., Sakellari, A., Giannopoulou, K., Paraskevopoulou, V., and Dassenakis, M. (2007). Dissolved and particulate trace metal levels in the Saronikos Gulf, Greece, in 2004. The impact of the primary Wastewater Treatment Plant of Psittalia. Desalination 210, 98–109. doi: 10.1016/j.desal.2006.05.036
Séguret, M. J. M., Koçak, M., Theodosi, C., Ussher, S. J., Worsfold, P. J., Herut, B., et al. (2011). Iron solubility in crustal and anthropogenic aerosols: the Eastern Mediterranean as a case study. Mar. Chem. 126, 229–238. doi: 10.1016/j.marchem.2011.05.007
Semeniuk, D. M., Cullen, J. T., Johnson, W. K., Gagnon, K., Ruth, T. J., and Maldonado, M. T. (2009). Plankton copper requirements and uptake in the subarctic Northeast Pacific Ocean. Deep Sea Res. I Oceanogr. Res. Papers 56, 1130–1142. doi: 10.1016/j.dsr.2009.03.003
Siokou-Frangou, I., Christaki, U., Mazzocchi, M. G., Montresor, M., Ribera d'Alcalá, M., Vaqué, D., et al. (2010). Plankton in the open Mediterranean Sea: a review. Biogeosciences 7, 1543–1586. doi: 10.5194/bg-7-1543-2010
Smith, D. C., and Azam, F. (1992). A simple, economical method for measuring bacterial protein synthesis rates in sea water using 3H-leucine. Mar Microb Food Webs 6: 107–114.
Statham, P. J., and Hart, V. (2005). Dissolved iron in the Cretan Sea (eastern Mediterranean). Limnol. Oceanogr. 50, 1142–1148. doi: 10.4319/lo.2005.50.4.1142
Steemann-Nielsen, E. (1952). On the determination of the activity for measuring primary production. J. Cons. Int. Explor. Mer. 18, 117–140.
Sunda, W. G. (1987). “Neritic-oceanic trends in tracemetal toxicity to phytoplankton communities,” in Oceanic Processes in Marine Pollution, ed Z. J. M. Capuzzo (Malabar: Krieger), 19–29.
Tanaka, T., Thingstad, T. F., Christaki, U., Colombet, J., Cornet-Barthaux, V., Courties, C., et al. (2011). Lack of P-limitation of phytoplankton and heterotrophic prokaryotes in surface waters of three anticyclonic eddies in the stratified Mediterranean Sea. Biogeosciences 8, 525–538. doi: 10.5194/bg-8-525-2011
Tanhua, T., Hainbucher, D., Schroeder, K., et al. (2013). The Mediterranean Sea system: a review and an introduction to the special issue. Ocean Sci. 9, 789–803. doi: 10.5194/os-9-789-2013
Ternon, E., Guieu, C., Ridame, C., L'Helguen, S., and Catala, P. (2011). Longitudinal variability of the biogeochemical role of Mediterranean aerosols in the Mediterranean Sea. Biogeosciences 8, 1067–1080. doi: 10.5194/bg-8-1067-2011
Thingstad, T. F., Skjoldal, E. F., and Bohne, R. A. (1993). Phosphorus cycling and algal bacterial competition in Sandsfjord, western Norway. Mar. Ecol. Prog. Ser. 99, 239–259. doi: 10.3354/meps099239
Triantaphyllou, M., Dimiza, M., Krasakopoulou, E., et al. (2010). Seasonal variation in Emiliania huxleyi coccolith morphology and calcification in the Aegean Sea (Eastern Mediterranean). Geobios 43, 99–110. doi: 10.1016/j.geobios.2009.09.002
Tsiola, A., Pitta, P., Fodelianakis, S., et al. (2016). Nutrient limitation in surface waters of the oligotrophic Eastern Mediterranean Sea: an enrichment microcosm experiment. Microb. Ecol. 71, 575–588. doi: 10.1007/s00248-015-0713-5
Van Wambeke, F., Catala, P., Pujo-Pay, M., and Lebaron, P. (2011). Vertical and longitudinal gradients in HNA-LNA cell abundances and cytometric characteristics in the Mediterranean Sea. Biogeosciences 8, 1853–1863. doi: 10.5194/bg-8-1853-2011
Vaulot, D., and Marie, D. (1999). Diel variability of photosynthetic picoplankton in the equatorial Pacific. Appl. Environ. Microbiol. 104, 3297–3310. doi: 10.1029/98jc01333
Wambeke, F. V., Obernosterer, I., Moutin, T., Duhamel, S., Ulloa, O., and Claustre, H. (2008). Heterotrophic bacterial production in the eastern South Pacific: longitudinal trends and coupling with primary production. Biogeosciences 5, 157–169. doi: 10.5194/bg-5-157-2008
Watkins-Brandt, K., Letelier, R., Spitz, Y., et al. (2011). Addition of inorganic or organic phosphorus enhances nitrogen and carbon fixation in the -oligotrophic North Pacific. Mar. Ecol. Prog. Ser. 432, 17–29. doi: 10.3354/meps09147
Welschmeyer, N. A. (1994). Fluorometric analysis of chlorophyll a in the presence of chlorophyll b and pheopigments. Limnol. Oceanogr. 39, 1985–1992. doi: 10.4319/lo.1994.39.8.1985
Wu, J., Chung, S.-W., Wen, L.-S., et al. (2003). Dissolved inorganic phosphorus, dissolved iron, and Trichodesmium in the oligotrophic South China Sea. Global Biogeochem. Cycles 17, 8–10. doi: 10.1029/2002GB001924
Zimmerman, A. E., Allison, S. D., and Martiny, A. C. (2014). Phylogenetic constraints on elemental stoichiometry and resource allocation in heterotrophic marine bacteria. Environ. Microbiol. 16, 1398–1410. doi: 10.1111/1462-2920.12329
Zohary, T., Herut, B., Krom, M. D., Fauzi, C., Mantoura, R., Pitta, P., et al. (2005). P-limited bacteria but N and P co-limited phytoplankton in the Eastern Mediterranean - A microcosm experiment. Deep Sea Res. II Top. Stud. Oceanogr. 52, 3011–3023. doi: 10.1016/j.dsr2.2005.08.011
Keywords: mesocosm experiments, dust, aerosols, nutrients, trace metals, Eastern Mediterranean Sea
Citation: Herut B, Rahav E, Tsagaraki TM, Giannakourou A, Tsiola A, Psarra S, Lagaria A, Papageorgiou N, Mihalopoulos N, Theodosi CN, Violaki K, Stathopoulou E, Scoullos M, Krom MD, Stockdale A, Shi Z, Berman-Frank I, Meador TB, Tanaka T and Paraskevi P (2016) The Potential Impact of Saharan Dust and Polluted Aerosols on Microbial Populations in the East Mediterranean Sea, an Overview of a Mesocosm Experimental Approach. Front. Mar. Sci. 3:226. doi: 10.3389/fmars.2016.00226
Received: 29 July 2016; Accepted: 27 October 2016;
Published: 15 November 2016.
Edited by:
Cosimo Solidoro, National Institute of Oceanography and Experimental Geophysics, ItalyReviewed by:
Ursula Scharler, University of KwaZulu-Natal, South AfricaMaurizio Ribera D'Alcala', Stazione Zoologica Anton Dihrn, Italy
Copyright © 2016 Herut, Rahav, Tsagaraki, Giannakourou, Tsiola, Psarra, Lagaria, Papageorgiou, Mihalopoulos, Theodosi, Violaki, Stathopoulou, Scoullos, Krom, Stockdale, Shi, Berman-Frank, Meador, Tanaka and Paraskevi. This is an open-access article distributed under the terms of the Creative Commons Attribution License (CC BY). The use, distribution or reproduction in other forums is permitted, provided the original author(s) or licensor are credited and that the original publication in this journal is cited, in accordance with accepted academic practice. No use, distribution or reproduction is permitted which does not comply with these terms.
*Correspondence: Barak Herut, YmFyYWtAb2NlYW4ub3JnLmls
Eyal Rahav, ZXlhbC5yYWhhdkBvY2Vhbi5vcmcuaWw=