- 1Israel Oceanographic and Limnological Research, The Kinneret Limnological Laboratory, Migdal, Israel
- 2Israel Oceanographic and Limnological Research, National Institute of Oceanography, Haifa, Israel
- 3Institute of Marine Science, University of California, Santa Cruz, CA, USA
The oligotrophic southeastern Mediterranean Sea (SEMS) is frequently exposed to desert-dust deposition which supplies nutrients, trace metals and a wide array of viable airborne microorganisms. In this study, we experimentally examined the impact of aerosol addition, collected during an intense dust storm event in early September 2015, on the biomass and activity of pico-phytoplankton and heterotrophic bacterial populations at the sea-surface micro layer (SML) relative to the sub surface layer (SSL). Aerosol (1.5 mg L−1) was added to SML and SSL water samples in microcosms (4.5 L) and the water was frequently sampled over a period of 48 h. While the aerosol amendment triggered a moderate 1.5–2-fold increase in primary production in both the SML and the SSL, bacterial production increased by ~3 and ~7-folds in the SSL and SML, respectively. Concurrently, the abundance and flow-cytometric characteristics (green fluorescence and side scatter signals) of high nucleic acid (HNA) and low nucleic acid (LNA) bacterial cells showed a significant increase in the %HNA, in both SML and SSL samples following aerosol amendment. This shift in nucleic acid content took place at a much faster rate in the SML, suggesting a more active heterotrophic community. These changes were likely a result of higher rates of carbon utilizations in the SML following the dust addition, as assessed by a selected hydrocarbons and saccharides analysis. Additionally, a high absorption rate of hydrocarbons by the aerosol particles was measured following the additions, leaving less than 10% of these molecules available for potential heterotrophic microbial utilization. Our results suggest that the heterotrophic microbial community inhabiting the SML is more efficient in utilizing aerosol associated constituents than the community in the SSL.
Introduction
The sea-surface microlayer (SML) is the uppermost layer of the oceans (20–400 μm thick), located between the subsurface layer (SSL) waters and the atmosphere (Liss and Duce, 1997). The SML is a unique physiochemical and biological habitat that covers ~70% of Earth's surface. This boundary layer may play a significant role in many biogeochemical processes including air-sea gas and heat exchange (Liss and Duce, 1997) and the cycling of various elements (Wurl and Holmes, 2008). Its chemical content is different from the one usually found in the SSL. For example, higher concentrations of saccharides, hydrocarbons, amino acids, and polysaccharides were detected at the SML compared to the SSL (Engel et al., 2004). Hydrocarbons in the form of alkyl chains (i.e., n-alkanes) are highly enriched in the microlayer due to their hydrophobic character and low density (Marty and Saliot, 1976). It is assumed that high concentrations of organic matter in the SML may aggregate with sticky Transparent Exopolymer Particles (TEP) that are also enriched in the SML, resulting in gel-like particulate matter (Cunliffe et al., 2010). These aggregates may also include bacteria cells (Passow and Alldredge, 1994) and potentially various other molecules. In addition bacteria cells embedded in these particles may use these hydrocarbon molecules in the aggregates as an energy source (Grossi et al., 2007; Yakimov et al., 2007; Sevilla et al., 2015). Unsaturated alkyl chain like molecules as in the case of α-olefins (1-alkenes), might show elevated photo-oxidation rates and as a result light induced degradation (Mouzdahir et al., 2001). These differences may affect microbial activity (Reinthaler et al., 2008; Sarmento et al., 2015), enhance/reduce extracellular enzymatic activity (Kuznetsova and Lee, 2001; Engel and Galgani, 2016) and affect microbial diversity and abundance (Reinthaler et al., 2008; Vila-costa et al., 2013).
The SML communities (frequently termed “Neuston”) are composed of diverse groups of phytoneuston (autotrophs) and bacterioneuston (heterotrophs) (Liss and Duce, 1997; Cunliffe et al., 2010), which thrive on the relatively enriched organic matrix (Guitart et al., 2013; Engel and Galgani, 2016). One of the main external sources of nutrients to the SML is wet or dry atmospheric depositions (Cunliffe et al., 2010; Guieu et al., 2014). Deposited aerosols first interact with the SML and then sink through the SSL to deeper waters, supplying nutrients such as N, P, and Fe (e.g., Herut et al., 1999, 2002; Chien et al., 2016). They may also introduce viable airborne microbes (Griffin, 2010; Peter et al., 2014; Rahav et al., 2016a,b). Therefore, any dry or wet deposition has the potential of changing the diversity and activity of the phytoneuston and bacterioneuston communities.
The southeastern Mediterranean Sea (SEMS) is a low nutrients low chlorophyll marine province (Berman et al., 1984; Krom et al., 1991; Yacobi et al., 1995; Rahav et al., 2013; Kress et al., 2014) dominated by small-size microbes with low productivity (Yacobi et al., 1995; Bar-zeev and Rahav, 2015; Raveh et al., 2015). Recent studies showed that these autotrophic microorganisms are primarily limited by N or co-limited by N&P, whereas heterotrophic bacteria are P or C limited during summertime (Kress et al., 2005; Zohary et al., 2005; Rahav et al., 2016c). Previous studies emphasized the significant role of atmospheric deposition in supplying limiting nutrients to the SEMS (Herut et al., 1999, 2002; Guieu et al., 2014). While these studies have assessed the impact of atmospheric dust deposition on surface phytoplankton and heterotrophic bacterial communities in the SEMS (e.g., Ridame et al., 2011; Rahav et al., 2016b), none of these studies distinguished between the SML and the SSL.
In this study, we present a microcosm experimental assay that examines the response of the neuston and bulk bacterioplankton communities, collected from the SML and SSL respectively to the addition of dry deposition of aerosol including desert dust (1.5 mg L−1). Temporal dynamics of the autotrophic and heterotrophic microbial abundances and activity were recorded for 48 h at high temporal resolution (every 4–8 h). High nucleic acid (HNA) concentration per bacterial cell, was reported as an efficient measure for bacterial activity (Lebaron et al., 2001; Talarmin et al., 2011; Van Wambeke et al., 2011) and thus was included in this study in addition to bacterial productivity. Chemical analyses of saccharides and selected hydrocarbons were also examined. We hypothesized that due to the physiochemical and biological differences between the SML and SSL, any external atmospheric addition that delivers micro and macro-nutrients may trigger distinct responses in these two layers.
Materials and Methods
SML and SSL Sampling
SML water samples were collected on December 15, 2015 by using a custom made rotating drum sampler as described in Harvey (1966) with minor changes: the rotating drum used was a glass tube (r = 18 cm; l = 60 cm). The drum was pre-cleaned with a concentrated HCl solution and washed with sample water for a few min prior collection. No silicon or plastic tubes were used. The collector includes an indurated non-contaminating silicon blade (0.5 m) fixed on an aluminum grip holder, collecting the water into a pre-combusted glass bottle. These changes are consistent with the glass plate collection procedure (Harvey and Burzell, 1972) by using similar materials. In addition, these improvements allow for a fast cleaning procedure, avoiding the contamination caused by reusing materials such as plastics and silicon tubing. The SML sample (23 L in total) was collected by connecting the sampler in parallel to a small boat (rotating speed ~5 RPM). SML thickness was approximately 75 μm thick, and collection time was 90 min. Sampling starting point selected was 1 km offshore (32°49′34N, 34°57′20E); collection ended 560 m northeast of the starting point. The study area is a coastal oligotrophic water zone (see discussion below); with low influence of urban runoff. The port of Haifa is located a few kilometers north of our study area; however, the general water circulation carries most of the bulk water away from our study zone. The average bottom depth along the sampling was 13 m. SSL water sample (23 L in total) was collected from 1 m depth along the same cruise-track. Samples were kept in acid prewashed sealed containers until processed at the Israel Oceanographic and Limnological Research (IOLR) institute. The wind speed was <3.5 knots, with waves of up to 0.3 m, and the seawater surface temperature was 18°C. Though waves in the same magnitude showed no impact on SML surfactants tension and spreading rates (Hale and Mitchell, 1997), it is possible that the SML collection may include minor amounts of SSL water and thus the results presented here may be an underestimation of the trends observed (i.e., dilution of the microlayer water with sub-surface water).
Aerosol Collection and Experimental Design
A dry deposition sample was collected on September 8–9, 2015, using a pre-clean 2 m2 glass plate (more details in Rahav et al., 2016b). The pre cleaned glass plate was exposed for 48 h during an extreme dust storm arriving from the north-east (Supplementary Figure S1). Over this time 1 gr m−2 of aerosol was deposited of plate area. Assuming a deposition rate of up to 50 g dust m−2 yr−1 in the Mediterranean Sea (Lawrence and Neff, 2009), the accumulated aerosol in this time period corresponds to ~2–3% of the annual dust deposition in this system. We added 1.5 mg L−1 of the collected aerosol (in triplicate) to acid-cleaned 4.5 L transparent High-density polyethylene Nalgene bottles containing either SML or SSL water (6.7–6.8 mg aerosol in total to each bottle). This addition is equivalent to the concentration reported for the upper mixed layer (top 5 m) during a heavy dust storm (Herut et al., 2002, 2005; Rahav et al., 2016b), and is similar to the amounts tested in other studies from the SEMS (Ridame et al., 2011; Herut et al., 2016). Samples were incubated in an outdoor pool with seawater flow-through to maintain ambient seawater temperature. The bottles used allowed the penetration of most of the light spectrum, excluding UV light. Blank treatments of SML or SSL water without an aerosol addition were also carried out in parallel. SSL bottles were submerged to a depth of 1 m in the pool by using weights. Subsamples of seawater from each incubation bottle were collected for Synechococcus abundance, pico-eukaryotes abundance, nano-eukaryotic abundance, heterotrophic bacterial abundance, primary production, and bacterial production measurements at 0, 1.5, 5, 9, 17, 21, 26, and 44 h after the aerosol addition.
Pico-Phytoplankton and Bacterial Abundance
Water samples (1.8 mL) were fixed with 50% glutaraldehyde (6 μl, Sigma-Aldrich G7651), frozen in liquid nitrogen and stored at −80°C until analyzed. Pico-phytoplankton abundance (namely Synechococcus and autotrophic eukaryotes algae) was determined using an Attune® Acoustic Focusing Flow Cytometer (Applied Biosystems) equipped with a syringe-based fluidic system and 488 and 405-nm lasers at a flow rate of 100 μL min−1 (Bar-zeev and Rahav, 2015). For heterotrophic bacterial abundance determination, subsamples (100 μl) were separately incubated at room temperature for 15 min with the nucleic acid stain SYTO9 (1:105 vol:vol) and then run at a low flow rate of 25 μL min−1 (Vaulot and Marie, 1999). Low nucleic acid (LNA) and HNA bacteria were differentiated by coupling their green fluorescence and side-scatter (Lebaron et al., 2001; Talarmin et al., 2011; Van Wambeke et al., 2011). For more details, see (Rahav et al., 2016b).
Primary Production
Photosynthetic carbon fixation rates were estimated using the 14C incorporation method (Nielsen, 1952). For more details, see (Rahav et al., 2016b).
Bacterial Production
Bacterial production was estimated using the [4,5-3H]-leucine incorporation method (Simon et al., 1990). A conversion factor of 3 kg C mol−1 leucine incorporated was used, assuming an isotopic dilution of 2.0 (Simon and Azam, 1989). For more details, see (Rahav et al., 2016b).
Dissolved Monosaccharides Concentration
SML and SSL samples (10 ml) were filtered using 0.22 μm polycarbonate membranes (Osmonics INC). The dissolved monosaccharides' (no hydrolysis) concentration was determined using the 2,4,6-Tripyridyl-s-Triazine (TPTZ) reagent (Sigma-Aldrich) method according to Myklestad et al. (1997). All samples were analyzed using an Uvikon 9100/9400 spectrophotometer (SECOMAM).
GC/MS Analysis
Samples (200 ml) were filtered using 0.22 μm non-absorbing membrane, followed by the extraction of dissolved n-alkanes and α-olefins from the water samples by liquid:liquid extraction, using 3 × 30 ml n-hexane extraction repeats, followed by 3 × 30 ml dichloromethane. The organic extracts were combined, concentrated to 1 ml via rotary evaporation and dried by passing through an MgSO4 column. Samples were analyzed using an Agilent 6890 gas chromatograph coupled with a 5973 Agilent mass spectrometer. Identification of the hydrocarbons was based on ions analysis (alkane ions = 43, 57, 71…α-olefins = 41, 55, 69…) in addition to library matching (NIST 2) and external standards R.T as described next. Quantification of the results was achieved by using C8-C30 external standards of n-alkanes (Sigma-Aldrich) and α-olefins (AccuStandard®, Inc). Alkanes and α-olefins adsorption to the membrane and to the experiment flasks was lower than 10%, method quantitation level = 10 ng L−1.
Statistical Analyses
The different variables presented in the figures and tables are averages and standard deviation (biological replicates, n = 3). Changes in primary production, bacterial production, the abundance of Synechococcus, pico/nano-eukaryotes, and heterotrophic bacteria throughout the experiments (0–44 h) were evaluated using a one-way analysis of variance (ANOVA), followed by a Fisher LSD multiple comparison post hoc-test with a confidence level of 95% (α = 0.05). Prior analyses, the ANOVA assumptions were examined. The statistical analyses were carried out using the XLSTAT software.
Results
Initial SML and SSL Characteristics Prior to Aerosol Addition
The SML at the study site was characterized by a higher concentration of n-alkanes (52.5 ng L−1) and olefins, also termed “1-alkenes” (1.12 mg L−1), relative to the SSL (46.4 ng n-alkanes L−1 and 0.70 mg α-olefins L−1). Similarly, the SML contained a higher concentration of monosaccharides relative to the SSL, 890 μg L−1 vs. 613 μg L−1, respectively (Table 1). The total α-olefins (1-alkenes) concentration in the SML was more than 3 orders of magnitude higher than the alkanes and monosaccharides, suggesting α-olefins were a dominant source of carbon (Table 1).

Table 1. Dissolved Σ [alkanes(C8−C30)], Σ [α-olefins(C11−C32)] and monosaccharides in the SML and SSL, prior (T0) and 44 h after (T44) aerosol addition.
Synechococcus dominated the picophytoplankton in the SML and SSL (~2.3 × 104 cells ml−1), whereas in both layers the pico-eukaryotes abundance was lower by an order of magnitude (1.0 × 103 to 1.5 × 103 cells ml−1) (Table 2). Heterotrophic bacterial abundance was similar in both water layers (~1.5 × 105 cells ml−1), although high nucleic acid bacteria (HNA) accounted for 7% at the SML and ~15% at the SSL. Primary production (PP) was overall low, with 60% higher rates measured at the SML (0.08 ± 0.00 μg C L−1 h−1) compared to the SSL (0.05 ± 0.00 μg C L−1 h−1) (Table 2). Similarly, bacterial production (BP) was ~50% higher at the SML (0.06 ± 0.00 μg C L−1 h−1) than at the SSL (0.04 ± 0.01 μg C L−1 h−1) (Table 2). Nevertheless, these activity rates resulted in a similar BP:PP ratio (~0.75:1), suggesting a weak dominance of autotrophic metabolism (over heterotrophic metabolism) in both water layers.
Aerosol Characteristics
The aerosol sample used in our experiment was collected during an exceptional dust storm event that arrived from the northeast rather than the more common southwest (Saharan desert) sources (Supplementary Figure S1). Based on the aluminum (Al) concentration in the collected aerosol, the Al settling velocity, and the weight of the particles collected, we calculated a dry deposition concentration of 1.05 mg L−1 at the upper 5 m mixed layer as reported in Rahav et al. (2016b). While such an episodic and strong deposition event is considered high in this system (reviewed in Guieu et al., 2014), it is well in the range previously reported for the Mediterranean Sea (e.g., Herut et al., 2002; Ridame et al., 2011, 2013) and similar to additions tested in previous microcosm bioassays (Herut et al., 2005; Rahav et al., 2016c). The micro and macro solubilized nutrients concentrations that were leached off the added aerosol sample are detailed in Rahav et al. (2016b) and discussed below.
Bacterioplankton Response to Aerosol Addition
Following aerosol addition, the abundances of Synechococcus (ranging from ~2 × 104 cells ml−1 to ~7 × 104 cells ml−1) and nano-eukaryote (ranging from 250 cells ml−1 to 1200 cells ml−1) in both layer samples were similar to the unamended treatments (Figure 1) and thus they were not significantly affected by the addition of the aerosols. Pico-eukaryote abundance following the aerosol addition was similar to the unamended control during the first 21 h in both layers (~1000–2000 cells ml−1, P > 0.05), thereafter increased ~2-fold relative to the unamended controls in the SML microcosms only (5000 cells ml−1, P < 0.01) (Figures 1B,E, Table 3). Heterotrophic bacterial abundance increased ~2-fold in the SML and SSL following the addition of aerosol (from ~1.5 × 105 cells ml−1 to ~4 × 105 cells ml−1, P < 0.05), with more profound differences between the treated versus the non-treated sample for the SML (Figures 2A,C). These differences were also apparent in the increased relative abundance of HNA over LNA bacteria, with 2-fold higher HNA bacteria (P = 0.02) recorded following the aerosol addition at both the SML and the SSL (Figure 2, Table 3). While the changes in microorganism abundance were relatively modest (Figures 1, 2), more profound differences were recorded for both PP and BP rates following the aerosol addition (Figure 3, Table 3). PP rates increased by ~2-fold relative to the untreated control (P < 0.05) 17 h post aerosol addition in both the SML and SSL. This enhancement lasted for the experiment's entire duration; 44 h post addition (Figures 3A,D, Table 3). Simultaneously, BP exhibited a stronger response to the aerosol addition, ~3-fold higher rates recorded at the SSL (increasing from 0.04 μg C L−1 h−1 to 0.11 μg C L−1 h−1, P < 0.05) and up to a ~7-fold increase at the SML (from 0.06 μg C L−1 h−1 to 0.44 μg C L−1 h−1, P < 0.05) relative to the unamended control that showed no change (Figures 3B,E, Table 3). These differences resulted in a higher BP:PP ratio at both water layers following the aerosol addition relative to the unamended controls (Figure 3, P < 0.05). Enrichment factors (EF) were calculated as the ratio between the abundance or the activity in the SML divided by those measured in the SSL (0–44 h). The EF-values for Synechococcus and nano-eukaryotes were similar in both the unamended and the aerosol addition bioassays (an insignificant change from a 1:1 ratio, P > 0.05). In contrast, pico-eukaryotes abundance, heterotrophic bacterial abundance, PP, and BP all exhibited significant higher EF-values (>1) following aerosol addition (Figure 4, P < 0.05).
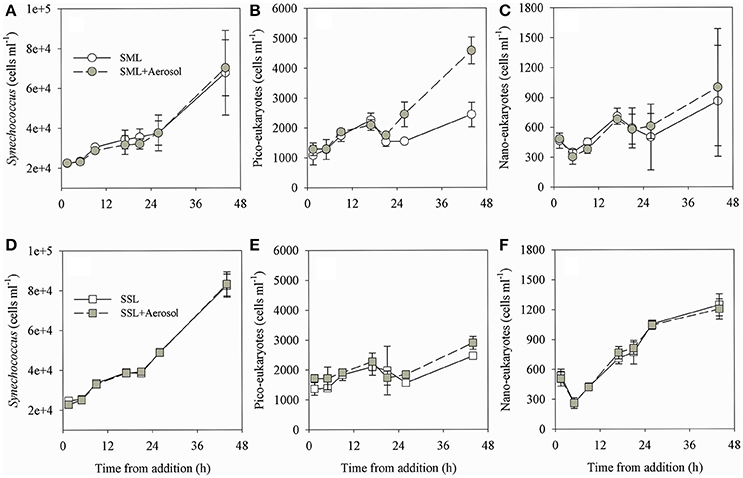
Figure 1. The temporal dynamics of Synechococcus (A,D), pico-eukaryotes (B,E) and nano-eukaryotes (C,F) in the SML (top panels) and SSL (bottom panels) microcosms. Experiments including aerosol additions (1.5 mg L−1) are presented in gray, control experiments (containing no additions) are colored white. Values are the averages and standard deviation from 3 biological replicates (n = 3).
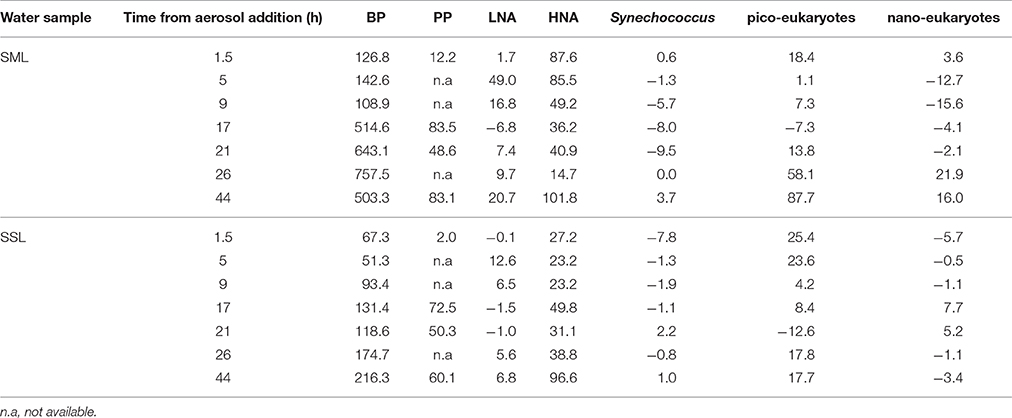
Table 3. Summary of the net change (%) observed following aerosol addition (1.5 mg L−1) in the SML and the SSL microcosms relative to unamended controls.
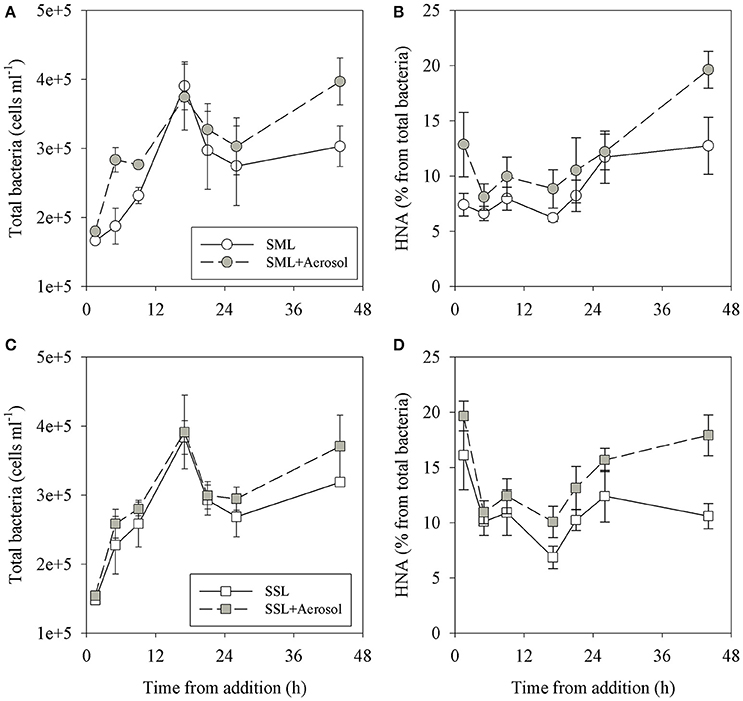
Figure 2. The temporal dynamics of total heterotrophic bacteria (A,C) and the percentage of HNA from the total bacteria (B,D) in the SML (A,B) and SSL (C,D) layers. Experiments including aerosol additions (1.5 mg L−1) are presented in gray, control experiments (containing no additions) are colored white. Values are the averages and standard deviation from 3 biological replicates (n = 3).
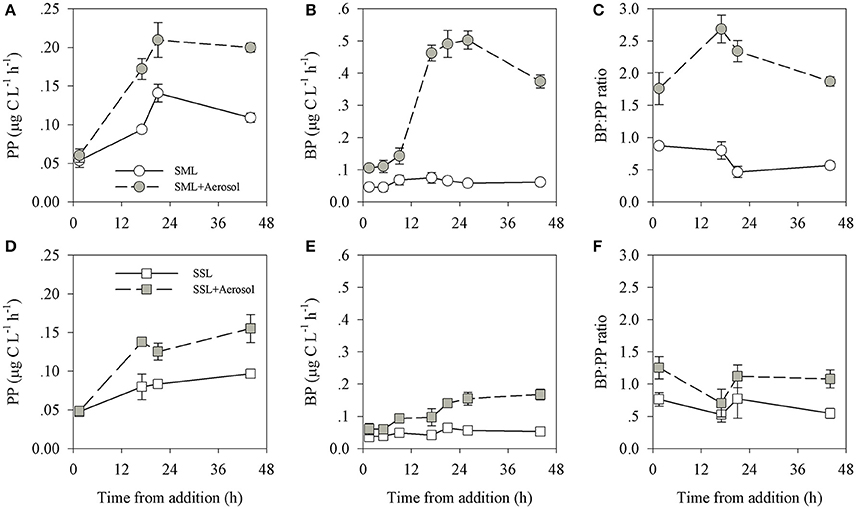
Figure 3. Temporal dynamics of primary production (A,D), bacterial production (B,E) and the BP: PP ratio (C,F) in the SML (A–C) and SSL (D–F) layers. Experiments including aerosol additions (1.5 mg L−1) are presented in gray, control experiments (containing no additions) are colored white. Values are the averages and standard deviation from 3 biological replicates (n = 3).
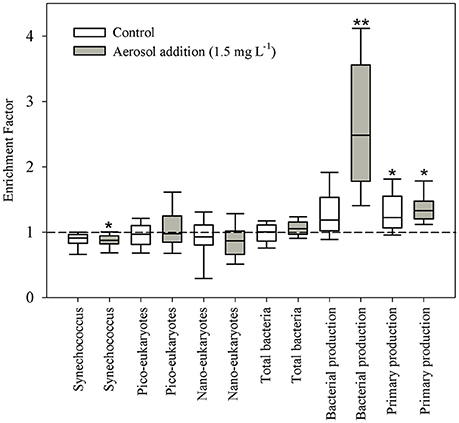
Figure 4. Box-plot distribution of the abundance or activity enrichment factors (SML: SSL ratio). Central line indicates median value. Interquartile box plot of abundance and activity enrichment factors: bottom and top horizontal lines indicate the 10 and 90th percentiles respectively. *P < 0.05; **P < 0.01.
Dissolved Hydrocarbons Post Aerosol Addition
Overall, the dissolved n-alkane concentration decreased substantially with time (T0 vs. T44 h) in the SML and to a lower level in the SSL following aerosol additions (Table 1). n-alkanes at the SML were reduced by ~50% at the conclusion of the experiment (reaching 23.7 ng L−1), whereas a decrease to below detection limit was observed in the SSL (Table 1). α-olefins, which were the major carbohydrate found, were reduced by two orders of magnitude in the SML and to below detection limit in the SSL at T44 h (Table 1). Finally, monosaccharides decreased by ~50% at the SML (472 μg L−1) and by ~66% at the SSL (190 μg L−1) (Table 1). Based on the BP rates measured in the control treatments and the C-rich monosaccharides concentration at the end of the experiment (T44), bacterial activity can account for 2.7 and 2.2 μg C L−1 in the SML and SSL unamended microcosms, respectively. Simultaneously, the total consumption of [4,5-3H]-leucine (T44) in the SSL+aerosol can account for 5.4 μg C L−1 and up to 15.7 μg C L−1 in the SML+aerosol treatments. The concentration (μg/Kg) of hydrocarbons delivered by the aerosol particles only was negligible relative to the ambient concentration in the water.
Discussion
Several studies have examined the effect of dust and aerosols on the marine bacterioplankton biomass and/or activity using model simulations (e.g., Mahowald, 2007; Guieu et al., 2014; Chien et al., 2016) and microcosm/mesocosm bioassays (e.g., Herut et al., 2005; Paytan et al., 2009; Marañón et al., 2010; Guieu et al., 2010; Romero et al., 2011; Rahav et al., 2016a). To the best of our knowledge, none of these studies distinguished between the responses triggered by aerosol additions to the SML and those triggered by such additions to the SSL. In fact, these studies mostly considered the SSL (referred as “surface water” or “surface mixed layer”). Since the SML is the uppermost water layer that interacts with the atmosphere and to which atmospheric particles are directly deposited, its bacteria and plankton (neuston) inhabitants may respond differently than the SSL populations (Cunliffe et al., 2010; Vila-costa et al., 2013). Here we experimentally tested the response of these two water layers to natural dry deposition aerosol addition and compared the responses of the bacterioplankton communities in these two layers at the SEMS.
SML Organic Enrichment
Our measurements demonstrate moderate, yet significant (P > 0.05), differences in saccharides and hydrocarbons concentration between the SML and the SSL prior to the aerosol addition, resulting in EF >1 (Table 1). Thus, the SML was enriched in dissolved organic carbon, potentially bioavailable for bacterial consumption, as observed in other marine environments (Cincinelli et al., 2001; Guigue et al., 2011; Santos et al., 2011). The major carbon source found in both layers was α-olefins. These hydrocarbons may originate from oil contamination (Riley et al., 1982), phytoplankton (Youngblood and Blumer, 1973) or even aerosol particles downwind to algae bloom (Ovadnevaite et al., 2011). It is possible that in the proximity to Haifa Bay industrial and rural area, aerosol particles, or water runoff could enrich the water with these hydrocarbons. Yet no other sign of such events was observed.
SML Bacterioplankton Enrichment
Heterotrophic bacterial abundances prior to the aerosol addition were similar in the SML and in the underlying SSL water layer (EF ~1, Table 2). These results agree with several studies (Cunliffe et al., 2009, 2010), yet oppose others, which show significantly higher bacterial abundances in the SML (e.g., MacIntyre, 1974; Hardy, 1982). Picophytoplankton abundance at the SML was lower than at the SSL, especially the abundance of pico-eukaryotes (EF < 1). Although studies reported an elevated phyto-neuston abundance relative to the SSL (e.g., Södergren, 1993; Guitart et al., 2013), it is apparent that several eukaryotic phytoplankton species, such as Chaetoceros, are less abundant in the SML (Hardy et al., 1984) or are differently effected by high light irradiation levels (Ruiz-González et al., 2012). A low autotrophic abundance in the SML might be explained by the intense radiation levels at this layer (particularly UV), which may cause photo-damage to the cells, especially in environments such as the SEMS in summer where radiation levels may reach >1000 μmol quanta m−2 s−1 (Dishon et al., 2012).
Activity in the SML and SSL
PP rates were higher at the SML relative to the SSL prior to and after the aerosol addition (Table 3, Figure 3). In contrast, BP rates were similar in both SML and SSL unamended microcosms and were elevated in both layers following aerosol additions, with a stronger response in the SML+aerosol microcosm. These results reflect higher cell-specific activity (production per cell) in the neuston communities that inhabit the SML relative to the bacterioplankton in the SSL. Relative to the very low concentration of inorganic nutrients characterizing this study area (Azov, 1986; Krom et al., 1991; Yacobi et al., 1995; Raveh et al., 2015), the addition of aerosol leached nutrients (as reported for this aerosol in Rahav et al. (2016b) would result in nearly doubling the levels of nitrate+nitrite (N, ~550 nM) and in a small increase in phosphorus concentration (P, ~12 nM). Assuming bacteria in the SEMS as P limited (Krom et al., 2010) and a 10–20 fg C per bacterial cell (Simon and Azam, 1989), the addition of P from the aerosol tested here (using a 106:1 Redfield C:P ratio), could explain an increase in bacterial abundance of up to ~1 × 106 cells−1 mL−1, which is one order of magnitude higher than that measured for both the SML and SSL (net change of ~1 × 105 cells−1 mL−1). It is possible that a great fraction of the added phosphorous was consumed by bacteria cells to fulfill their cellular metabolic needs rather than for growth/cell devision. This is also examplified in the increase in BP rates and the increase in %HNA bacteria following aerosol addition but not in the overall abundance of bacteria (Figures 2, 3). It may also suggest that the added P was taken by other compartments within the food-web (Thingstad et al., 2005; Pitta et al., 2016), or that P is not the limiting nutirent for bacterial activity as recently suggested (Tanaka et al., 2011; Rahav et al., 2016c). Alternativly, phosphorous might have been utilized faster by other microorganisms and transferred through the microbial food web directly to higher trophic levels (e.g., Thingstad et al., 2005), or that heterotrophic bacteria are not primarily limited by this element (e.g., Tanaka et al., 2011; Rahav et al., 2016c). Autotrophic microbes did not exhibit any significant increase in biomass, yet were more active as derived from the elevated PP rate (Figures 2, 3). Further, although relatively higher concentrations of hydrocarbons and saccharides were measured at the SML prior to the aerosol addition (as compared to the SSL), only slightly elevated BP or PP rates were recorded at the SML. Thus, the different carbon concentrations alone cannot explain the elevated BP and PP rates following the aerosol addition. These results are in agreement with a recent study performed in the same area showing a moderate increase in BP rates following the addition of glucose as a single amendment, while a significant enhancement in BP was reached after the addition of glucose, nitrogen and phosphate simultaneously (Rahav et al., 2016c). In addition to the aerosol derived N and P input, other micronutrients from the aerosols may also be limiting factors for microbial activity (e.g., Paytan et al., 2009). Tovar-sánchez et al. (2014) showed that Fe originating from aerosol particles was found to be unevenly distributed between the SML and SSL following a dust storm event. Thus, when considering the results described here and the work presented by Tovar-sánchez and collegues, we assume that aerosol depositions may trigger different impacts in these distinct layers. It is also likely that a high concentration of carbon, along with other aerosol-derived nutrients such as N and P or Fe that are added to a nutrient-poor aquatic system such as the SEMS, may not only alter the total microbial production, but also elevate the activity of specific groups in the SML. The difference in microbial production rates between the SML and SSL measured here are in agreement with a few studies that showed an equal or higher microbial activity in the SML relative to the SSL (e.g., Kuznetsova and Lee, 2001; Obernosterer et al., 2005), yet opposes other studies (Stolle et al., 2009; Santos et al., 2011; Sarmento et al., 2015). It is well-known that diverse bacterial groups have different sensitivities to UV radiation (UVR). SAR11 activity, for example, is known to be inhibited by high UVR, whereas Gammaproteobacteria and Bacteroidetes show UVR resistance (Alonso-Sáez et al., 2007; Santos et al., 2011). Hence, it is likely that the unique community in the SML in the SEMS is well adapted to the high-light conditions in this environment and can take advantage of the higher carbon and nutrients concentration in the SML, thus grow faster than the SSL microbial communities despite the high radiation. We assume that the bulk microbial activity rate depends on the bacterial species relative abundance, their adaption to the microenvironment and the availability of nutrients in the SML and SSL. The major reduction in dissolved n-alkanes and α-olefins, measured 44 h after the aerosol addition in the SSL and SML, may be attributed to either heterotrophic consumption or adsorption by aerosol particles. So far only a few bacterial strains isolated from polluted aquatic habitats show high uptake rates of α-olefins or alkanes on timescales similar to those examined here (Whyte et al., 1998). Thus, we estimate that in addition to possible heterotrophic utilization of these carbons, the major reduction in soluble alkanes and α-olefins concentration was a result of adsorption to aerosol particles.
Carbons Adsorption to Aerosol Particles
Contrary to alkanes and α-olefins for which concentrations were almost completely reduced following aerosol amendment (T0 vs. T44 h), monosaccharides decreased by only ~50–60% throughout the experiment's duration (Table 1). The monosaccharides' consumption (after 44 h) was almost the same in the SML and the SSL following aerosol additions (~420 μg L−1), although different initial and end concentrations were recorded in both water layers (Table 1). Monosaccharides are considered as the energetically-favored carbon sources for heterotrophic bacterial metabolism (Kirchman, 2012), as opposed to alkanes and α-olefins, which can be utilized only by a few specialized groups (Yakimov et al., 2007; Sevilla et al., 2015). Yet, based on the total leucine C incorporation during the experiment (derived from the BP measurements), less than 10% of the monosaccharides' reduction could be attributed to bacterial consumption in both water layers. We therefore assume that the physical adsorption of saccharides by the aerosol particles was more significant, and accounted for the remaining monosaccharides reduction. Relative to alkanes and α-olefins, monosaccharides are more hydrophilic, we assume that as a result, these saccharides show a reduced adsorption rate to the surface of the aerosol particles (“preferring” the water phase). Thus, unlike alkanes and α-olefins that are more hydrophobic and were cleared from the soluble fraction following aerosol addition (primarily via adsorption), monosaccharides showed only a low reduction in concentration in the soluble fraction. Considering the abiotic removal via adsorption onto dust particles, a dust storm event such as the one examined here (September 2015), could substantially change the carbons availability to heterotrophic bacteria in the upper water layer and thus shift the PP to BP ratio from being “autotrophic-dominated” to “heterotrophic dominated.” Moreover, since considerable amounts of dust and aerosol are usually deposited in the SEMS (Herut et al., 1999, 2002), a chronic adsorption of various carbon substances to aerosol particles and their transfer down the water column may be a mechanism to transfer carbon to the deep water. The different types of carbon containing compounds (polar and hydrophobic) that were possibly adsorbed by the aerosol particles could later be leached off the particles at greater depths such as the deep chlorophyll maxima (usually 100–150 m in the SEMS, Kress et al., 2014) or even at the aphotic layer, thereby contributing to deep-water heterotrophic microbial activity. This adsorption may depend on aerosol concentration, composition, and timing of the deposition, and may thus have significant implications on the microbial loop. This mechanism may contribute to the relatively high metabolic activity of bacteria in the deep waters of the Mediterranean Sea attributed to the relatively warm conditions of this system (Luna et al., 2012). Further work should examine this mechanism of carbon transfer to the aphotic layers, as well as its availability to the subsurface microbial communities in the SEMS.
Conclusions
We suggest that one of the major differences between the SML and SSL is the higher SML bacterioneuston activity rates that most probably resulted in the higher carbon utilization rate observed. It is possible that in this experiment, the consumption rate of monosaccharides differs between the SML and the SSL, although the same amount was consumed at the experiment end. Vila-costa et al. (2013), who studied freshwater SML's bacteria and archaea communities following two Saharan dust storms, reported that the abundance of these groups did not change significantly following the dust storms. This finding is also supported by other studies in the Mediterranean (e.g., Tovar-sánchez et al., 2014). Yet, Vila Costa's group reported that the composition of these groups was altered following aerosol introduction, resulting in a community shift (Vila-costa et al., 2013). In this study however, we did not characterize bacterial diversity (using molecular approaches such as 16S rRNA or 18S rRNA) and thus we cannot determine whether community shifts occurred following aerosol additions. Nevertheless, the increase in bacterial activity in the SML following aerosol addition and the relative increase in HNA bacterial abundance (Table 3), may be an indication of the presence of bacterioneuston that are specifically adapted to take advantage of the constituents supplied by aerosols.
This study demonstrates the opportunistic character of the bacterioneuston community once nutrient-carrying airborne particles are introduced to the SML. Further, studies of the seasonal changes in the biodiversity and physiology of these communities in relation to atmospheric deposition from different sources are needed. Finally, the nature and dynamics of nutrients, metals (and microorganisms) exchange between the SML and SSL is currently unknown and warrants more study. In addition to carbon utilization, physical adsorption of these molecules by the aerosol particles may deliver carbon from the surface into deeper water, from the enriched SML to more oligotrophic layers of the sea.
Author Contributions
Conceived and designed the experiment: PA and ER. Performed the experiment: PA and ER. Analyzed the data: PA, AP, BH and ER. Contributed reagents/materials/analysis tools: PA and ER. Wrote the paper: PA, AP, BH and ER.
Conflict of Interest Statement
The authors declare that the research was conducted in the absence of any commercial or financial relationships that could be construed as a potential conflict of interest.
Acknowledgments
We would like to thank Lilach Baumer for English editing. This study was supported by a grants awarded by the Ministry of National infrastructures, Energy and Water Resources grants 21317028 to PA, and 3-11519 to ER, by the Ministry of environmental Protection (145–1–2) to ER, by the PERSEUS project's (EC contract 287600) contribution to BH, partially by the ENVIMED MERMEX TRACOMED project's contribution to BH and by the NSF-OCE (grant 0850467) to AP.
Supplementary Material
The Supplementary Material for this article can be found online at: http://journal.frontiersin.org/article/10.3389/fmars.2016.00222/full#supplementary-material
Supplementary Figure S1. A three-day back trajectory analysis arriving at 100, 500, and 1000m altitude levels commencing at 10.00 UTC using the HYSPLIT (Hybrid Single-Particle Lagrangian Integrated Trajectory) model from the Air Resources Laboratory. The star represents the study site off the SEMS.
References
Alonso-Sáez, L., Arístegui, J., Pinhassi, J., Gómez-Consarnau, L., González, J. M., Vaqué, D., et al. (2007). Bacterial assemblage structure and carbon metabolism along a productivity gradient in the NE Atlantic Ocean. Aquat. Microbia. Ecol. 46, 43–53. doi: 10.3354/ame046043
Azov, Y. (1986). Seasonal patterns of phytoplankton productivity and abundance in nearshore oligotrophic waters of the Levant Basin (Mediterranean). J. Plankton Res. 8, 41–53. doi: 10.1093/plankt/8.1.41
Bar-zeev, E., and Rahav, E. (2015). Microbial metabolism of transparent exopolymer particles during the summer months along a eutrophic estuary system. Front. Microbiol. 6:403. doi: 10.3389/fmicb.2015.00403
Berman, T., Townsend, D. W., ElSayed, S. Z., Trees, C. C., and Azov, Y. (1984). Optical transparency, chlorophyll and primary productivity in the Eastern Mediterranean near the Israeli coast. Oceanol. Acta 7, 367–372.
Chien, C.-T., Mackey, K. R. M., Dutkiewicz, S., Mahowald, N. M., Prospero, J. M., and Paytan, A. (2016). Effects of African dust deposition on phytoplankton in the western tropical Atlantic Ocean off Barbados. Glob. Biogeochem. Cycles 30, 716–734. doi: 10.1002/2015GB005334
Cincinelli, A., Stortini, A. M., Pergini, M., Checchini, L., and Lepri, L. (2001). Organic pollutants in sea-surface microlayer and aerosol in the coastal environment of Leghorn - (Tyrrhenian Sea). Mar. Chem. 76, 77–98. doi: 10.1016/S0304-4203(01)00049-4
Cunliffe, M., Salter, M., Mann, P. J., Whiteley, A. S., Upstill-Goddard, R. C., and Murrell, J. C. (2009). Dissolved organic carbon and bacterial populations in the gelatinous surface microlayer of a Norwegian fjord mesocosm. FEMS Microbiol. Lett. 299, 248–254. doi: 10.1111/j.1574-6968.2009.01751.x
Cunliffe, M., Upstill-goddard, R. C., and Murrell, J. C. (2010). Microbiology of aquatic surface microlayers. FEMS Microbiol. Rev. 35, 233–246. doi: 10.1111/j.1574-6976.2010.00246.x
Dishon, G., Dubinsky, Z., Caras, T., Rahav, E., Bar-Zeev, E., Tzubery, Y., et al. (2012). Optical habitats of ultraphytoplankton groups in the Gulf of Eilat (Aqaba), Northern Red Sea. Int. J. Remote Sens. 33, 2683–2705. doi: 10.1080/01431161.2011.619209
Engel, A., and Galgani, L. (2016). The organic sea-surface microlayer in the upwelling region off the coast of Peru and potential implications for air–sea exchange processes. Biogeosciences 13, 989–1007. doi: 10.5194/bg-13-989-2016
Engel, A., Thoms, S., Riebesell, U., Rochelle-Newall, E., and Zondervan, I. (2004). Polysaccharide aggregation as a potential sink of marine dissolved organic carbon. Nature 428, 929–932. doi: 10.1038/nature02453
Griffin, D. W. (2010). Atmospheric movement of microorganisms in clouds of desert dust and implications for human health. Clin. Microbiol. Rev. 20, 459–477. doi: 10.1128/CMR.00039-06
Grossi, V., Cravo-Laureau, C., Méou, A., Raphel, D., Garzino, F., and Hirschler-Réa, A. (2007). Anaerobic 1-alkene metabolism by the alkane- and alkene-degrading sulfate reducer Desulfatibacillum aliphaticivorans strain CV2803T. Appl. Environ. Microbiol. 73, 7882–7890. doi: 10.1128/AEM.01097-07
Guieu, C., Aumont, O., Paytan, A., Bopp, L., Law, C. S., Mahowald, N., et al. (2014). The significance of the episodic nature of atmospheric deposition to Low Nutrient Low Chlorophyll regions. Glob. Biochem. Cycles 28, 1179–1198. doi: 10.1002/2014GB004852
Guieu, C., Dulac, F., Desboeufs, K., Wagener, T., Pulido-Villena, E., Grisoni, J. M., et al. (2010). Large clean mesocosms and simulated dust deposition: a new methodology to investigate responses of marine oligotrophic ecosystems to atmospheric inputs. Biogeosciences 7, 2765–2784. doi: 10.5194/bg-7-2765-2010
Guigue, C., Tedetti, M., Giorgi, S., and Goutx, M. (2011). Occurrence and distribution of hydrocarbons in the surface microlayer and subsurface water from the urban coastal marine area off Marseilles, Northwestern Mediterranean Sea. Mar. Pollut. Bull. 62, 2741–2752. doi: 10.1016/j.marpolbul.2011.09.013
Guitart, C., Murrell, J. C., Cunliffe, M., Engel, A., Frka, S., Salter, M., et al. (2013). Sea surface microlayers : a unified physicochemical and biological perspective of the air – ocean interface. Progr. Oceanogr. 109, 104–116. doi: 10.1016/j.pocean.2012.08.004
Hale, M. S., and Mitchell, J. G. (1997). Sea surface microlayer and bacterioneuston spreading dynamics. Mar. Ecol. Progr. Ser. 147, 269–276. doi: 10.3354/meps147269
Hardy, J. T. (1982). The sea surface microlayer: biology, chemistry and anthropogenic enrichment. Progr. Oceanogr. 11, 307–328. doi: 10.1016/0079-6611(82)90001-5
Hardy, J. T., Apts, C. W., and Marine, B. (1984). The sea-surface microlayer: phytoneuston productivity and effects of atmospheric particulate matter. Mar. Biol. 82, 293–300. doi: 10.1007/BF00392409
Harvey, G. (1966). Collection from the sea surface : a new method and initial results. Limnol. Oceanogr. 11, 608–613. doi: 10.4319/lo.1966.11.4.0608
Harvey, G. W., and Burzell, L. A. (1972). A simple microlayer method for small samples. Limnol. Oceanogr. 17, 156–157. doi: 10.4319/lo.1972.17.1.0156
Herut, B., Collier, R., and Krom, M. D. (2002). The role of dust in supplying nitrogen and phosphorus to the Southeast Mediterranean. Limnol. Oceanogr. 47, 870–878. doi: 10.4319/lo.2002.47.3.0870
Herut, B., Krom, M. D., Pan, G., and Mortimer, R. (1999). Atmospheric input of nitrogen and phosphorus to the Southeast Mediterranean: sources, fluxes, and possible impact. Limnol. Oceanogr. 44, 1683–1692. doi: 10.4319/lo.1999.44.7.1683
Herut, B., Rahav, E., Tsagaraki, T. M., Giannakourou, A., Tsiola, A., Psarra, S., et al. (2016). The potential impact of Saharan dust and polluted aerosols on microbial populations in the East Mediterranean Sea, an overview of a mesocosm experimental approach. Front. Mar. Sci. 3:226. doi: 10.3389/fmars.2016.00226
Herut, B., Zohary, T., Krom, M. D., and Mantoura, R. F. C. (2005). Response of East Mediterranean surface water to Saharan dust : on-board microcosm experiment and field observations. Deep Sea Res. II 52, 3024–3040. doi: 10.1016/j.dsr2.2005.09.003
Kress, N., Thingstad, T. F., Pitta, P., Psarra, S., Tanaka, T., Zohary, T., et al. (2005). Effect of P and N addition to oligotrophic Eastern Mediterranean waters influenced by near-shore waters: a microcosm experiment. Deep Sea Res. Part II 52, 3054–3073.
Kress, N., Gertman, I., and Herut, B. (2014). Temporal evolution of physical and chemical characteristics of the water column in the Easternmost Levantine basin (Eastern Mediterranean Sea) from 2002 to 2010. J. Mar. Syst. 135, 6–13. doi: 10.1016/j.jmarsys.2013.11.016
Krom, M. D., Emeis, K.-C., and Van Cappellen, P. (2010). Why is the Eastern Mediterranean phosphorus limited? Progr. Oceanogr. 85, 236–244. doi: 10.1016/j.pocean.2010.03.003
Krom, M. D., Kress, N., Brenner, S., and Gordon, L. I. (1991). Phosphorus limitation of primary productivity in the eastern Mediterranean Sea. Limnol. Oceanogr. 36, 424–432. doi: 10.4319/lo.1991.36.3.0424
Kuznetsova, M., and Lee, C. (2001). Enhanced extracellular enzymatic peptide hydrolysis in the sea-surface microlayer. Mar. Chem. 73, 319–332. doi: 10.1016/S0304-4203(00)00116-X
Lawrence, C. R., and Neff, J. C. (2009). The contemporary physical and chemical flux of aeolian dust: a synthesis of direct measurements of dust deposition. Chem. Geol. 267, 46–63. doi: 10.1016/j.chemgeo.2009.02.005
Lebaron, P., Servais, P., Agogué, H., Courties, C., and Joux, F. (2001). Does the high nucleic acid content of individual bacterial cells allow us to discriminate between active cells and inactive cells in aquatic systems? Appl. Environ. Microbiol. 67, 1775–1782. doi: 10.1128/AEM.67.4.1775-1782.2001
Liss, P. S., and Duce, R. A. (1997). The Sea Surface and Global Change. New York, NY: Cambridge University Press.
Luna, G. M., Bianchelli, S., Decembrini, F., De Domenico, E., Danovaro, R., and Dell'Anno, A. (2012). The dark portion of the Mediterranean Sea is a bioreactor of organic matter cycling. Glob. Biogeochem. Cycles 26, 1–14. doi: 10.1029/2011GB004168
MacIntyre, F. (1974). The top millimeter of the ocean. Sci. Am. 230, 62–77. doi: 10.1038/scientificamerican0574-62
Mahowald, N. M. (2007). Anthropocene changes in desert area: sensitivity to climate model predictions. Geophys. Res. Lett. 34, L18817. doi: 10.1029/2007GL030472
Marañón, E., Fernández, A., Mouriño-Carballido, B., MartÍnez-GarcÍa, S., Teira, E., Cermeño, P., et al. (2010). Degree of oligotrophy controls the response of microbial plankton to Saharan dust. Limnol. Oceanogr. 55, 2339–2352. doi: 10.4319/lo.2010.55.6.2339
Marty, J. C., and Saliot, A. (1976). Hydrocarbons (normal alkanes) in the surface microlayer of seawater. Deep Sea Res. 23, 863–873. doi: 10.1016/0011-7471(76)90853-6
Mouzdahir, A., Grossi, V., Bakkas, S., and Rontani, J.-F. (2001). Visible light-dependent degradation of long-chain alkenes in killed cells of Emiliania huxleyi and Nannochloropsis salina. Phytochemistry 56, 677–684. doi: 10.1016/S0031-9422(00)00468-4
Myklestad, S. M., Skånøy, E., and Hestmann, S. (1997). A sensitive and rapid method for analysis of dissolved mono- and polysaccharides in seawater. Mar. Chem. 56, 279–286. doi: 10.1016/S0304-4203(96)00074-6
Nielsen, S. E. (1952). The use of radio-active carbon (C14) for measuring organic production in the sea. J. Cons. Perm. Ins. Explor. Mer. 18, 117–140. doi: 10.1093/icesjms/18.2.117
Obernosterer, I., Catala, P., Reinthaler, T., Herndl, G. J., and Lebaron, P. (2005). Enhanced heterotrophic activity in the surface microlayer of the Mediterranean Sea. Aquatic Microbial Ecol. 39, 293–302. doi: 10.3354/ame039293
Ovadnevaite, J., O'Dowd, C., Dall'Osto, M., Ceburnis, D., Worsnop, D. R., and Berresheim, H. (2011). Detecting high contributions of primary organic matter to marine aerosol: a case study. Geophys. Res. Lett. 38, L02807. doi: 10.1029/2010GL046083
Passow, U., and Alldredge, A. L. (1994). Distribution, size and bacterial colonization of transparent exopolymer particles (TEP) in the ocean. Mar. Ecol. Progress Ser. 113, 185–198. doi: 10.3354/meps113185
Paytan, A., Mackey, K. R. M., Chen, Y., Lima, I. D., Doney, S. C., Mahowald, N., et al. (2009). Toxicity of atmospheric aerosols on marine phytoplankton. Proc. Natl. Acad. Sci. U.S.A. 106, 4601–4605. doi: 10.1073/pnas.0811486106
Peter, H., Hörtnagl, P., Reche, I., and Sommaruga, R. (2014). Bacterial diversity and composition during rain events with and without Saharan dust influence reaching a high mountain lake in the Alps. Environ. Microbiol. Rep. 6, 618–624. doi: 10.1111/1758-2229.12175
Pitta, P., Nejstgaard, J. C., Tsagaraki, T. M., Zervoudaki, S., Egge, J. K., Frangoulis, C., et al. (2016). Confirming the “Rapid phosphorus transfer from microorganisms to mesozooplankton in the Eastern Mediterranean Sea” scenario through a mesocosm experiment. J. Plankton Res. 38, 502–521. doi: 10.1093/plankt/fbw010
Rahav, E., Giannetto, M. J., and Bar-Zeev, E. (2016c). Contribution of mono and polysaccharides to heterotrophic N2 fixation at the eastern Mediterranean coastline. Sci. Rep. 6:27858. doi: 10.1038/srep27858
Rahav, E., Herut, B., Stambler, N., Bar-Zeev, E., Mulholland, M. R., and Berman-frank, I. (2013). Uncoupling between dinitrogen fixation and primary productivity in the eastern Mediterranean Sea. J. Geophys. Res. 118, 195–202. doi: 10.1002/jgrg.20023
Rahav, E., Ovadia, G., Paytan, A., and Herut, B. (2016a). Contribution of airborne microbes to bacterial production and N2 fixation in seawater upon aerosol deposition. Geophys. Res. Lett. 43, 719–727. doi: 10.1002/2015GL066898
Rahav, E., Paytan, A., Chien, C.-T., Ovadia, G., Katz, T., and Herut, B. (2016b). The impact of atmospheric dry deposition associated microbes on the Southeastern Mediterranean Sea surface water following an intense dust storm. Front. Mar. Sci. 3:127. doi: 10.3389/fmars.2016.00127
Raveh, O., David, N., Rilov, G., and Rahav, E. (2015). The temporal dynamics of coastal phytoplankton and bacterioplankton in the Eastern Mediterranean Sea. PLoS ONE 10:e0140690. doi: 10.1371/journal.pone.0140690
Reinthaler, T., Sintes, E., and Herndl, G. J. (2008). Dissolved organic matter and bacterial production and respiration in the sea – surface microlayer of the open Atlantic and the western Mediterranean Sea. Limnol. Oceanogr. 53, 122–136. doi: 10.4319/lo.2008.53.1.0122
Ridame, C., Guieu, C., and L'Helguen, S. (2013). Strong stimulation of N2 fixation in oligotrophic Mediterranean Sea: results from dust addition in large in situ mesocosms. Biogeosciences 10, 7333–7346. doi: 10.5194/bg-10-7333-2013
Ridame, C., Le Moal, M., Guieu, C., Ternon, E., Biegala, I. C., and Helguen, S. L. (2011). Nutrient control of N2 fixation in the oligotrophic Mediterranean Sea and the impact of Saharan dust events. Biogeosciences 8, 2773–2783. doi: 10.5194/bg-8-2773-2011
Riley, R. G., Garland, T. R., O'Malley, M. L., Mann, D. C., and Wildung, R. E. (1982). 1-Alkenes as potential indicators of sediment shale oil contamination. Environ. Sci. Technol. 16, 709–713.
Romero, E., Peters, F., Marrasé, C., Guadayol, Ò., Gasol, J. M., and Weinbauer, M. G. (2011). Coastal Mediterranean plankton stimulation dynamics through a dust storm event: an experimental simulation. Estuar. Coast. Shelf Sci. 93, 27–39. doi: 10.1016/j.ecss.2011.03.019
Ruiz-González, C., Lefort, T., Galí, M., Montserrat Sala, M., Sommaruga, R., Simó, R., et al. (2012). Seasonal patterns in the sunlight sensitivity of bacterioplankton from Mediterranean surface coastal waters. FEMS Microbiol. Ecol. 79, 661–674. doi: 10.1111/j.1574-6941.2011.01247.x
Santos, L., Santos, A. L., Coelho, F. J. R. C., Gomes, N. C. M., Dias, J. M., Cunha, A., et al. (2011). Relation between bacterial activity in the surface microlayer and estuarine hydrodynamics. FEMS Microbiol. Ecol. 77, 636–646. doi: 10.1111/j.1574-6941.2011.01147.x
Sarmento, H., Casamayor, E. O., Auguet, J., Vila-Costa, M., Felip, M., Camarero, L., et al. (2015). Microbial food web components, bulk metabolism, and single-cell physiology of piconeuston in surface microlayers of high-altitude lakes. Front. Microbiol. 6:361. doi: 10.3389/fmicb.2015.00361
Sevilla, E., Yuste, L., and Rojo, F. (2015). Marine hydrocarbonoclastic bacteria as whole-cell biosensors for n-alkanes. Microb. Biotechnol. 8, 693–706. doi: 10.1111/1751-7915.12286
Simon, M., Alldredge, A. L., and Azam, F. (1990). Bacterial carbon dynamics on marine snow. Mar. Ecol. Prog. Ser. 65, 205–211.
Simon, M., and Azam, F. (1989). Protein content and protein synthesis rates of planktonic marine bacteria. Mar. Ecol. Prog. Ser. 51, 201–213. doi: 10.3354/meps051201
Södergren, A. (1993). Role of aquatic surface microlayer in the dynamics of nutrients and organic compounds in lakes, with implications for their ecotones. Hydrobiologia 251, 217–225. doi: 10.1007/BF00007181
Stolle, C., Nagel, K., Labrenz, M., and Jürgens, K. (2009). Bacterial activity in the sea-surface microlayer : in situ investigations in the Baltic Sea and the influence of sampling devices. Aquatic Microbial Ecol. 58, 67–78. doi: 10.3354/ame01351
Talarmin, A., Van Wambeke, F., Catala, P., Courties, C., and Lebaron, P. (2011). Flow cytometric assessment of specific leucine incorporation in the open Mediterranean. Biogeosci. 8, 253–265. doi: 10.5194/bg-8-253-2011
Tanaka, T., Thingstad, T. F., Christaki, U., Colombet, J., Cornet-Barthaux, V., Courties, C., et al. (2011). Lack of P-limitation of phytoplankton and heterotrophic prokaryotes in surface waters of three anticyclonic eddies in the stratified Mediterranean Sea. Biogeosci. 8, 525–538. doi: 10.5194/bg-8-525-2011
Thingstad, T. F., Krom, M. D., Mantoura, R. F. C., Flaten, G. A. F., Groom, S., Herut, B., et al. (2005). Nature of phosphorus limitation in the ultraoligotrophic Eastern Mediterranean. Science 309, 1068–1071. doi: 10.1126/science.1112632
Tovar-sánchez, A., Arrieta, J. M., Duarte, C. M., and Sañudo-wilhelmy, S. A. (2014). Spatial gradients in trace metal concentrations in the surface microlayer of the Mediterranean Sea. Front. Mar. Sci. 1:79. doi: 10.3389/fmars.2014.00079
Van Wambeke, F., Catala, P., Pujo-Pay, M., and Lebaron, P. (2011). Vertical and longitudinal gradients in HNA-LNA cell abundances and cytometric characteristics in the Mediterranean Sea. Biogeosciences 8, 1853–1863. doi: 10.5194/bg-8-1853-2011
Vaulot, D., and Marie, D. (1999). Diel variability of photosynthetic picoplankton in the equatorial Pacific. J. Geophys. Res. Oceans 104, 3297–3310. doi: 10.1029/98JC01333
Vila-costa, M., Barberan, A., Auguet, J. C., Sharma, S., Moran, M. A., and Casamayor, E. O. (2013). Bacterial and archaeal community structure in the surface microlayer of high mountain lakes examined under two atmospheric aerosol loading scenarios. FEMS Microbiol. Ecol. 84, 387–397. doi: 10.1111/1574-6941.12068
Whyte, L. G., Hawari, J., Zhou, E., Bourbonnie, L. U. C., Inniss, W. E., and Greer, C. W. (1998). Biodegradation of variable-chain-length alkanes at low temperatures by a psychrotrophic Rhodococcus sp. Appl. Environ. Microbiol. 64, 2578–2584.
Wurl, O., and Holmes, M. (2008). The gelatinous nature of the sea-surface microlayer. Mar. Chem. 110, 89–97. doi: 10.1016/j.marchem.2008.02.009
Yacobi, Y. Z., Zohary, T., Kress, N., Hecht, A., Robarts, R. D., Waiser, M., et al. (1995). Chlorophyll distribution throughout the southeastern Mediterranean in relation to the physical structure of the water mass. J. Mar. Syst. 6, 179–190. doi: 10.1016/0924-7963(94)00028-A
Yakimov, M. M., Timmis, K. N., and Golyshin, P. N. (2007). Obligate oil-degrading marine bacteria. Curr. Opin. Biotechnol. 18, 257–266. doi: 10.1016/j.copbio.2007.04.006
Youngblood, W. W., and Blumer, M. (1973). Alkanes and Alkenes in marine benthic algae. Mar. Biol. 21, 163–172. doi: 10.1007/BF00355246
Keywords: microlayer, aerosols, microcosms, mediterranean region, bacterial productivity
Citation: Astrahan P, Herut B, Paytan A and Rahav E (2016) The Impact of Dry Atmospheric Deposition on the Sea-Surface Microlayer in the SE Mediterranean Sea: An Experimental Approach. Front. Mar. Sci. 3:222. doi: 10.3389/fmars.2016.00222
Received: 24 August 2016; Accepted: 26 October 2016;
Published: 16 November 2016.
Edited by:
Stelios Katsanevakis, University of the Aegean, GreeceReviewed by:
Ashley Ballantyne, University of Montana, USAAntoni Tovar-Sanchez, Spanish National Research Council, Spain
Maria Montserrat Sala, Spanish National Research Council, Spain
Copyright © 2016 Astrahan, Herut, Paytan and Rahav. This is an open-access article distributed under the terms of the Creative Commons Attribution License (CC BY). The use, distribution or reproduction in other forums is permitted, provided the original author(s) or licensor are credited and that the original publication in this journal is cited, in accordance with accepted academic practice. No use, distribution or reproduction is permitted which does not comply with these terms.
*Correspondence: Peleg Astrahan, cGVsZWcuYXN0cmFoYW5Ab2NlYW4ub3JnLmls
Eyal Rahav, ZXlhbC5yYWhhdkBvY2Vhbi5vcmcuaWw=