- 1Hartley Anderson Ltd., Aberdeen, UK
- 2School of Ocean Science, Bangor University, Anglesey, UK
- 3Akvaplan-niva, FRAM–High North Centre for Climate and the Environment, Tromsø, Norway
Assessments of the impact of construction, operation, and removal of large infrastructures and other human activities on the marine environment are limited because they do not fully quantify the background baseline conditions and relevant scales of natural variability. Baselines as defined in Environmental Impact Assessments typically reflect the status of the environment and its variability drawn from published literature and augmented with some short term site specific characterization. Consequently, it can be difficult to determine whether a change in the environment subsequent to industrial activity is within or outside the range of natural background variability representative of an area over decades or centuries. An innovative approach that shows some promise in overcoming the limitations of traditional baseline monitoring methodology involves the analysis of shell material (sclerochronology) from molluscs living upon or within the seabed in potentially affected areas. Bivalves especially can be effective biomonitors of their environment over a wide range of spatial and temporal scales. A rapidly expanding body of research has established that numerous characteristics of the environment can be reflected in morphological and geochemical properties of the carbonate material in bivalve shells, as well as in functional responses such as growth rates. In addition, the annual banding pattern in shells can provide an absolute chronometer of environmental variability and/or industrial effects. Further, some species of very long-lived bivalves can be crossdated back in time, like trees, by comparing these annual banding patterns in their shells. It is therefore feasible to develop extended timeseries of certain marine environmental variables that can provide important insights into long temporal scales of baseline variability. We review recent innovative work on the shell structure, morphology, and geochemistry of bivalves and conclude that they have substantial potential for use as monitors of environmental variability and the effects of pollutants and disturbance.
Introduction
Effective monitoring plays a key role in protecting the environment and limiting anthropogenic impacts by providing evidence of the efficacy or otherwise of mitigation measures. Monitoring programs are carried out to minimise uncertainties and ensure that in situ effects do not go beyond the modeled predictions and remain within defined limits.
While standard environmental monitoring strategies assess the impacts of marine infrastructure in great detail, up to and including decommissioning, a full description of the baseline against which the results of such monitoring are assessed is precluded because the period between planning and the start of construction is too short to allow the range of natural background variability to be defined. Pre-existing long term instrumental records are sparse in the marine environment, often situated very close to the coast, and limited in the range of variables measured. In addition, new industrial developments and associated infrastructure can be situated in remote areas where long term records of environmental conditions are unavailable. Nevertheless, in the context of long period cycles and regime shifts in the marine environment that may be of the order of several decades (Drinkwater, 2006), and of even longer trends resulting from natural cycles and anthropogenic climate change (Kaufman et al., 2009), assessment of the full environmental impact attributable to an operation requires a realistic understanding of impacts that may be attributable to other sources (Figure 1).
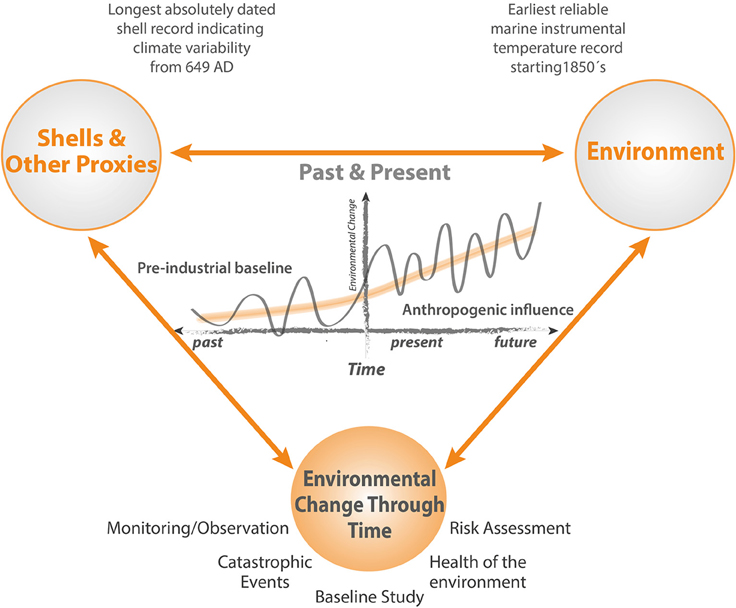
Figure 1. Schematic illustrating how cycles, trends, regime shifts and extrema are expressed in the context of an extended baseline. Short periods of monitoring reveal only a small fraction of the full picture. High resolution multidecadal and multicentennial timeseries based on bivalve sclerochronology can provide insights into the full range of natural and anthropogenically forced environmental variability, enabling a much more realistic and reliable approach to monitoring and risk assessment programs.
One approach to this issue involves measurement of the responses of organisms in an area of interest to environmental change. In particular, the use of the hard parts of bivalve molluscs—the field known as sclerochronology—is now established in marine palaeoclimate studies and presents an exciting option for extended baseline marine monitoring.
Sclerochronology is the study of physical and chemical variations in the microgrowth of hard tissues (Davenport, 1938; Buddemeier et al., 1974; Jones, 1980; Oschmann, 2008), which can be placed into a temporal context by periodic banding. Advances in bivalve-based sclerochronology over recent years have generated a range of new possibilities for environmental monitoring, including in particular the ability to obtain long (multi-decadal) baseline environmental information. However, this potential has not yet attained a wide recognition in the commercial and regulatory sectors, and as a result sclerochronological techniques have not become a standard aspect of monitoring programs. Here we review the state of the art of bivalve sclerochronology and assess its potential to address needs and gaps in current marine environmental monitoring practices.
Monitoring the Environment
Environmental monitoring is defined as “the regular collection, generally under regulatory mandate, of biological, chemical, or physical data from predetermined locations such that the present status and any ecological changes attributable to industry operations can be quantified” (GESAMP, 1999). Only with sufficiently good knowledge of the current status, extent, changes, and trends in the condition of the marine environment can stakeholders and policy makers make informed and reasonable decisions, set rational priorities, calculate costs and benefits, and evaluate risks of proceeding with or refraining from the proposed projects. A tight feedback response within the environmental management framework reinforces successful environmental management and its ability to protect the environment through quick responses and continuous improvements in prediction, observation, regulation, and operation. Furthermore, there is a particular need for monitoring procedures that can identify and quantify variability and trends through time and geographical space. Monitoring programs aim to provide an overview of general environmental conditions, variability and trends with the goal of robustly estimating and attributing any changes associated with marine developments.
Objectively interpreting results and evaluating impacts upon the environment is dependent upon the standardization of monitoring approaches (e.g., standardized taxonomic analysis and geochemical measurements), which in turn allows the intercalibration of data sets and comparison of relative impacts. While the contaminants of concern and the biological species available for monitoring will vary between marine regions, monitoring methods and assessment criteria should be harmonized as appropriate. In recent years, substantial effort has been put into developing biological effect tools (Beliaeff and Burgeot, 2002; Broeg et al., 2005; Dagnino et al., 2007; ICES, 2007; Thain et al., 2008; Edge et al., 2014). Moreover, new developments and improved technologies allow for measurements that were previously challenging and expensive to be made rapidly, in large numbers, and by technical staff with only basic skill requirements (Bowen and Depledge, 2006).
Baseline characterizations aim to establish pre-operational background environmental conditions, including variability and long-term trends, allowing site-specific operational planning to minimize environmental impact. Baseline survey data is used to define natural background levels and variability of physical, chemical, and biological parameters, which can subsequently be used as a point of comparison for later monitoring surveys. On a broader scale, baseline studies can also contribute to determining anthropogenic impacts and potentially distinguishing between natural and anthropogenic climate variations.
A further target of environmental monitoring is determination of “good environmental status” (GES) as defined by the European Union's Marine Strategy Framework Directive (MSFD). A number of descriptors have been put forward for the MSFD, intended to specify what is meant by GES (2010/477/EU, document C(2010) 5956, EC and MSFD, 2010).
The implementation of MSFD aims to protect and conserve the marine environment, prevent its decline, and, where necessary, restore marine ecosystems in areas where they have been negatively affected.
Biological Techniques in Environmental Monitoring
A biological effect is defined as the response of an organism, a population, or a community to changes in its environment. The usefulness and applicability of any biological-effect method will depend on how well it is able to separate anthropogenic stressors from the influence of other environmental factors or internal biological processes. Biological effects techniques include tools to indicate whether concentrations of contaminants are at safe levels, specifically in relation to the pollution effects of naturally occurring and synthetic chemicals in the marine environment. Applying these techniques enables the identification of pathways between contaminant and ecological receptor, as well as the detection of the impact of substances (or any combination of substances) that may not be analyzed as part of routine chemical monitoring programmes in the sense of “what you don't measure you don't find” (van der Oost et al., 2003; Thain et al., 2008). In general, biological indicators carry information about the status and health of the environment, and may reflect biological, chemical and/or physical conditions.
There have been clear examples in the aquatic environment where biological effect techniques have been used to identify problems and subsequently to monitor the efficacy of management interventions. For example, the Mussel Watch programs have been applied worldwide to assess pollution levels within coastal zones (Goldberg et al., 1978; Goldberg and Bertine, 2000). Primarily, indicators are used to characterize the current status and to track or predict significant changes; they often track effects with multiple causes in a more simplified and useful manner than direct contaminant measurements. In addition, quantitative measurements can be taken in the form of biomarkers; these are measurable responses in the biological system to exposure to doses of substances and are potential tools to detect exposure to or effects of contaminants. Biomarkers can measure responses at different biological levels: biochemical, physiological, organism, and population (Lam and Gray, 2003). Most of these biological effect techniques make use of markers in the tissue of the living animal.
Bivalves in Monitoring Studies—Current Approaches
In general, the term “biomarker” is applied to any change that can be detected in an individual living organism as a consequence of exposure to a harmful chemical (or chemicals). Depledge (1994) defines biomarkers more specifically as: “biochemical, cellular, physiological, or behavioral variations that can be measured in the tissue, body fluid samples, geochemistry, and morphology of the shell/exoskeleton or at the level of the whole organism, which provide evidence of exposure to and/or effects of, one or more chemical pollutants (radiation).” The selection of a biomarker depends on the nature of the environmental study and the information already available about the physical and chemical nature of the study area.
Biomarkers are being used in different countries as part of various marine monitoring programs e.g., the joint biological monitoring program for the North Sea (JAMP, 1998a,b), the UK National Marine Monitoring Programme (NMMP UK, CEFAS, 2004) and in Spain (Franco et al., 2002; Borja et al., 2004). One of the major concerns regarding the application of biomarkers as a regional monitoring tool has been the lack of standardization of methodologies. In response, a number of programs have developed standard operating procedures (SOPs) and undertaken inter-laboratory comparisons, including the standardization programs QUASIMEME, BEQUALM (Moore et al., 2004), and the SOPs of ICES. The use of biomarkers based on organic tissue or behavior only allows sampling of a “snapshot” in time, and longer time-series requires periodic sampling efforts. In this paper we argue that sclerochronological techniques present a viable and effective approach to long baseline environmental monitoring.
Shell material carries an imprint, in its chemistry, crystal structure and morphology, of the state of the ambient environment at the time of deposition (Figure 2). The periodically banded shells of long-lived bivalves can therefore be thought of as an archive of data reflecting pre- and post-impact conditions, with specimens from an unimpacted control site being used to define the pre-impact baseline. Data from extended multi-shell chronologies, where the precise timing of geochemical data points is determined by the periodic banding in the shells, can be used as a basis to study the background effects of seasonality and decadal (or even centennial) scale climate variability within the area of interest.
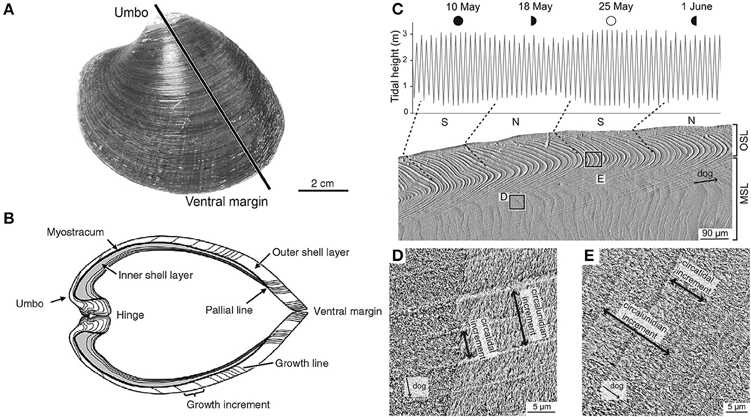
Figure 2. Morphological and microstructural features of the shell of Arctica islandica (left). (A) Outer shell surface of the left valve. The black line indicates the typical transect for cross-section. (B) Umbo-ventral margin cross-section as indicated in (A); this exposed surface is the typical site for high resolution geochemical sampling. Microstructure of Cerastodernum edule (right) showing (C) periodic banding with tidal resolution and (D,E) characteristic microstructural and crystallographic features, which show a high correlation with seawater temperatures. (A,B adapted from Schöne, 2003 C–E adapted from Milano et al., 2016).
Monitoring Targets
Coastal Monitoring
The increase in “competing users” of coastal and marine areas means that marine planning is becoming increasingly important for resolving conflicts between users and for managing environmental impacts on the sea (Douvere and Ehler, 2009). Marine spatial planning is a bottom-up process intended to improve collaboration and coordination among all coastal and ocean stakeholders, and to better inform and guide decision-making where it is perceived to affect their economic, environmental, security, and social and cultural interests (e.g., CMSP NOAA, 2015). In particular, marine coastal monitoring focuses on three general categories: major spill events (e.g., oil spills), chronic point-source pollution (e.g., outfalls, oil/gas platforms, coastal industry waste discharge), and multiple inputs (e.g., rivers), and all of these strategies have both a temporal and spatial aspect. During major spill events both the temporal and spatial components are complicated by rapidly changing conditions. In such cases pre-spill data is highly desirable for subsequent evaluation of the impacted site, but is most often not available.
Renewable Energy and Near-Shore Activities
Many technologies are currently being developed to harvest low carbon marine energy; these include offshore wind, wave and tidal power, salinity gradients, and thermal gradients. In 2007, European leaders agreed to source 20% of their energy needs from renewable sources by 2020 (Energy Policy for Europe 7224/07, the so-called Renewable Energy Roadmap; European Parliament, 2007). However, the large scale of planned offshore renewable energy developments (OREDs), will add to the existing pressures on coastal ecosystems, increasing the need for environmental and ecological monitoring. Based on Nedwell and Howell (2004) the lifecycle of a wind farm (~30 years) can be divided into four general phases: (1) the pre-construction phase (lasting 1–5 years), (2) the construction phase (1 year), (3) the operational phase (20–25 years), and (4) the decommissioning phase (1 year). Any significant environmental effects (Figure 3) are likely to depend on the natural disturbance regime and the stability and resilience of the communities (Gill, 2005). In terms of long-term ecological effects (Figure 3), the lifecycle time span and its individual phases need to be considered in ecological monitoring studies. A number of potential impacts of offshore wind farms on ecosystems have been identified, including underwater noise effects, bird collisions, the barrier effect, electromagnetic fields, visual/physical intrusion, and seabed disturbance during construction and operation (Figure 3; ETNWE, 2003; CEFAS, 2004; Carter et al., 2008).
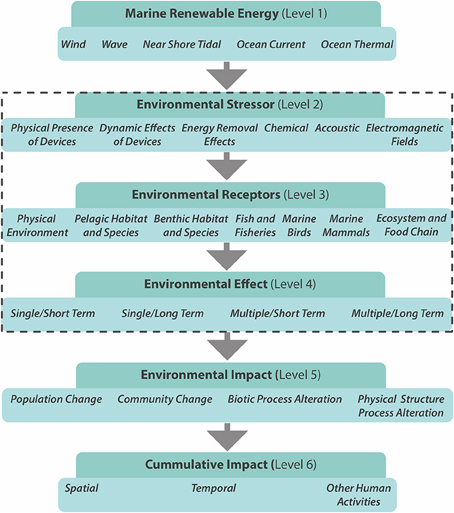
Figure 3. Framework for the consideration of environmental effects of marine renewable energy encompassing different scales (Figure: adapted from Boehlert and Gill, 2010). The dashed rectangle highlights the levels at which biomonitoring is most applicable. Level 2 summerizes some of the potential environmental stressors, which can effect receptors (physical, chemical, and biological) of level 3. Level four differentiates the duration and nature of the environmental effects.
Offshore renewable energy installations are likely to impact coastal ecosystems, since single developments have ecological footprints extending over several square kilometers of near-shore waters (e.g., Boehlert and Gill, 2010). Nevertheless, data on the environmental impacts of OREDs are limited (EC, 2015) and there is an urgent need to develop specific long-term observation methods to monitor their effects on the environment (Shumchenia et al., 2012; Lindeboom et al., 2015). Furthermore, various terrestrial and near-shore activities (Mason, 2002; Matthiessen and Law, 2002) have led to disturbance of habitat or local habitat loss. Other environmental aspects that need to be considered include changes in nutrient availability and cycling, sediment erosion or reduced sediment supply, changes in sea level and any change in vulnerability to natural and anthropogenic disturbances (McLusky et al., 1992; Schekkerman et al., 1994; Rogers and McCarty, 2000). Sclerochronology-based indicators, such as indicators of changes in food availability and/or foodweb structure [these indicators include shell growth, δ13Cshell, Ba/Cashell, etc.; see Section Ecosystem Variables (Trophic Levels, Food Supply, Metagenome)], can potentially be used for the establishment of baselines related to the pre-impact environment and for the in situ monitoring of any environmental change during the construction and operation of the installation.
Oil and Gas
While the total amount of oil and gas produced within the OSPAR area has decreased since 2001, the number of offshore installations has increased (OSPAR, 2010 Quality Status report). OSPAR is the legislative instrument by which 15 governments and the EU cooperate to protect the marine environment of the North-East Atlantic. In addition, rising global temperatures, particularly in the high northern latitudes, have coincided with a rapid decline in sea ice cover, making hydrocarbon resources in the Arctic increasingly accessible. The use of standard monitoring procedures to assess the impact of existing operations, decommissioning, and the opening up of new areas is enhanced and relevant where long term baseline conditions can be established for comparison.
Drilling discharge—a potential contamination source
Routine operations of production platforms can lead to the release of oil, chemicals, and naturally occurring radioactive materials; these occur especially through discharges of produced water, drainage water (water from platform decks etc.), drilling fluids, and from drill cuttings. Furthermore, accidental oil spills can occur during exploration and production operation. A main source of input are drill cuttings (drilling mud and fragments of overlying and reservoir rock) deposited onto the seafloor during the exploration phase. Drilling fluids, used to lubricate the drilling string, are divided into three types: water-based (WBM), oil-based (OBM), and synthetic-based (SBM). Water-based drilling fluids, consisting of inorganic components, are regarded as less harmful than the other two, and these are allowed to be discharged without treatment. The main constituents of WBM (bentonite clay and barite) are non-toxic, but they can smother sessile benthic animals.
In the North Sea alone the volume of drill cuttings is estimated at 12 million m3 (OLF, 2000). A study by Breuer et al. (2008) of a drill cuttings pile resulting from drilling oil based mud (such discharges were effectively banned in the OSPAR area in 1996) showed that microbially mediated diagenetic reactions in organic-rich cuttings can result in rapid removal of O2 within the top few millimeters resulting in anoxia within the cuttings pile. As a result, the rate of degradation of hydrocarbons is slowed and elevated concentrations of trace and heavy metals occur. Trace metals released into the porewater of the cutting pile can potentially diffuse into the overlying water by adsorbing onto Mn and Fe oxyhydroxides at the sediment water interface. Other metals (Cr, Cu, and Pb) can diffuse downward, becoming incorporated into Fe monosulfides. If the Fe sulfides are exposed to O2, e.g., by bioturbation, advection and/or pile resuspension during the decommission process, this may lead to the release of the associated metals into the water column (Huerta-Diaz et al., 1998; Saulnier and Mucci, 2000). Sclerochronological approaches that can be used to track some of these pollutants are described in Section Metal Pollution.
Another major source of oil discharge from routine production is produced water (PW), which is extracted from the reservoir along with the oil. PW can represent up to 80% of the waste and residual discharge to sea from natural oil production operations (Tibbetts et al., 1992; Carroll et al., 2001; McCormack et al., 2001) and contains hazardous substances including residues of chemicals used in the production process such as corrosion inhibitors and demulsifiers (chemicals that enhance the separation of oil from water) and inorganic compounds including heavy metals and radionuclides. The composition and amount of PW varies between fields. The ratio of PW to other fluids depends on the type of reservoir and its geochemical characteristics, as well as the production/processing techniques. The maximum permitted concentration of oil in discharged PW in the OSPAR area is 30 mg/l (e.g., Lee et al., 2011).
Other pressures from oil and gas activities include chemicals that can leak, e.g., from hydraulic valves, and leach from coatings and anodes of pipelines and other subsea structures. The seabed is physically disturbed not only during installation of pipelines, cables, subsea structures, and platforms, but also when they are decommissioned (an increasingly important issue as installations come to the end of their lifecycles, see below). Additionally, the risk of accidents such as leaks and spills may increase as the infrastructure ages. In recent years the oil industry has begun to decommission redundant installations and pipelines. The removal of the installations and associated infrastructure can cause sediment disturbance and subsequent localized impacts, such as turbidity. If there is a cutting pile at the base of the platform this may be disturbed and contaminated cuttings re-suspended. However, evidence indicates that these re-suspended particles do not disperse and settle back in the same area.
Decommissioning
Decommissioning is the process of removing or otherwise making safe oil or gas exploitation structures at the end of their life cycle. Decommissioning may be carried out by any one of four methods: complete removal, tow-and-place, partial removal (i.e., “topping”), or toppling (laying the structure on its side; Schroeder and Love, 2004; Macreadie et al., 2011; Fowler et al., 2014). Globally, more than 7000 oil and gas platforms distributed over 53 countries (Parente et al., 2006) will need to be decommissioned in the coming decades.
Of national and international bodies that regulate decommissioning in the NE Atlantic, OSPAR is particularly significant in terms of influencing the adopted approach. OSPAR Decision 98/3 prohibits leaving offshore installations wholly or partly in place but provides certain derogations to the legislation including the exception of concrete structures and the footing of large steel jackets with a weight of more than 10,000 tonnes.
Decommissioning assessment reports should take into account the effects of all decommissioning options, including energy budgets, biological and technological impact of discharges, secondary emissions, physical and habitat issues, fisheries, waste management, littering and drill cutting deposits (Parente et al., 2006).
Decommissioning alternatives to complete removal may include the creation of artificial reefs (so called “rig to reef” approach), which provide substrates for marine organisms (as for example the Gulf of Mexico). The objective of the “rig to reef” approach is to use the decommissioned structures for fisheries yield and production, for recreational activities, to prevent trawling, to repair degraded marine habitats, and for overall economic and social benefit. Ultimately a reef-based food chain may develop providing food sources for larger organisms such as fish (e.g., Claisse et al., 2014). Such a rig-reef community may vary considerably from the naturally occurring species composition and also affect local nutrient recycling within the water column and settling of nutrients to the seafloor, thereby affecting benthic organisms surrounding the reef area. One criterion for consideration of partial removal of decommissioned offshore oil platforms is its potential for conversion to a man-made reef that would provide a “net benefit” to the environment compared with complete removal of the structure. However, it has to be kept in mind that the removal of the structure may affect the environment negatively as well.
The decommissioning programme incorporates monitoring of the seabed after the asset has been decommissioned. The alternative disposal options will usually have different environmental effects and economic consequences, and with marine ecosystems expected to change rapidly in response to increasing anthropogenic influences and climate change, there is a strong need to assess and understand the long-term spatio-temporal variation in the environment and the marine ecosystem in the context of the physical presence of offshore structures. This would include the assessment of variability before, during and after the operational and decommissioning phases. Standard monitoring procedures reflect only the changes associated with the presence of the structure and compare them with a relatively short period before construction commences. Long baseline measurements of the kind described later in this paper could allow the combined effects of the construction, operation and decommissioning cycle to be evaluated in the context of long-term natural environmental variability.
Climate Change
In response to rapidly increasing greenhouse gas concentrations in the atmosphere, the oceans act as a buffer, taking up around 66% of the excess CO2 and around 93% of the excess heat content (IPCC et al., 2014). Consequences of increasing emissions from fossil fuels include rising seawater temperatures, deoxygenation, and ocean acidification, all of which can affect the benthos in various ways (Figure 4; ICES, 2008; Birchenough et al., 2015). In the context of the techniques discussed in this paper, it is important to understand and predict how these changes affect the benthic species used as environmental monitors, and how climate effects can be separated from natural background variability and effects attributable to an installation.
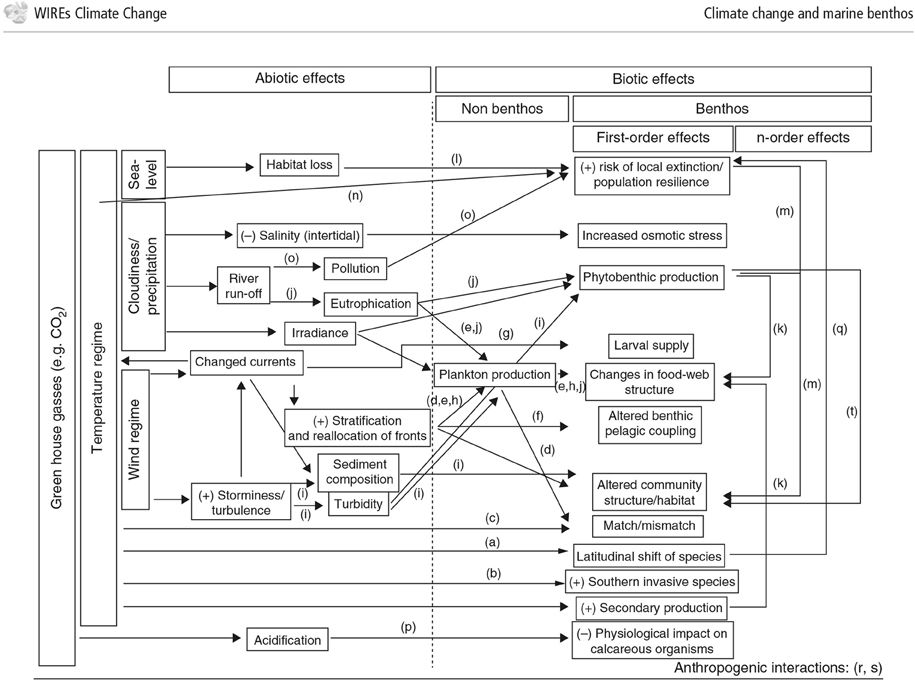
Figure 4. Conceptual diagram of the effects of climate change on benthic interactions. Illustration of the influence of increased CO2 and temperature and the effects of biotic and abiotic components (Figure from: Birchenough et al. (2015)).
Temperature and Hydrodynamics
Responses to changing temperature include distributional shifts, phenological changes, life history effects (reproduction and recruitment) and physiological responses (stress). The hydrodynamic regime can affect transport, dispersal, and settlement of larvae, consequently affecting species population dynamics (Levin, 2006).
Hypoxia
Changes in hydrodynamics may result in oxygen depletion as a result of stratification or eutrophication. Because pelagic and benthic processes can be tightly coupled, especially in coastal and selected shelf locations, the benthic ecosystem may be affected by changes in primary production and the transport pathways of benthic food sources. The quality and quantity of organic matter settling vertically through the water column is a vital factor affecting benthic abundance, biomass, growth and health (e.g., Dauwe et al., 1998).
Hypoxic zones (<2 mg l−1 dissolved oxygen) are projected to expand because of (a) increased water column stratification, (b) temperature-related increase in respiration and (c) changes in precipitation that cause amplified terrestrial fresh water discharges and an elevated volume of nutrients (incl. agricultural fertilizers). Mass mortality and decreased diversity in benthic species have been observed (e.g., Diaz and Rosenberg, 2008; Levin et al., 2009; Seitz et al., 2009). Furthermore, bottom water oxygen depletion is likely to alter biogeochemical processes and affect nutrient supply at the sediment-water interface (e.g., phosphorus release, denitrification). In contrast, climate change is also projected to induce more storm activity in some regions; a stormier environment could increase vertical mixing of the water column and decrease stratification, reducing the potential for oxygen depletion (Rabalais et al., 2007).
State of the Art of Bivalve Sclerochronology for Environmental Monitoring
Long-Lived Bivalves: A Brief Introduction
While the shells of a number of species have been used for environmental monitoring, the implementation of long baseline monitoring ideally depends on the availability of long-lived animals whose shells contain periodic growth increments which grow synchronously within populations and can therefore be used to generate multidecadal and multicentennial chronologies. These chronologies define a timeline of precisely dated shell material that can be used for geochemical analysis and to link patterns in functional responses (i.e., growth) to variability in environmental drivers. The two species that currently lend themselves best to these techniques are Arctica islandica (Linnaeus, 1767) and Glycymeris glycymeris (Linnaeus, 1758). Both occur over a relatively large geographic range in the temperate North Atlantic. A. islandica is the longest-lived (up to 507 years; Butler et al., 2013) and the most extensively researched species. In addition to its very significant presence in paleoclimatology research, this remarkable animal has contributed to studies in ecology, biology, pollution monitoring, gerontology and genetics. As well as being the longest living non-colonial animal whose actual age can be ascertained, A. islandica is known to grow synchronously within populations, allowing long chronologies to be constructed by crossdating living specimens with fossil shells (Butler et al., 2010, 2013). In addition to its potential for environmental monitoring, it can also be used for the effective reconstruction of marine climate on multicentennial timescales and at annual- and sub-annual resolution (Witbaard, 1997; Schöne et al., 2002, 2003; Goodwin et al., 2003; Butler et al., 2013; Mette et al., 2016).
Arctica islandica lives buried in surficial sediments with relatively short siphons which open at the sediment-water boundary. It is not attached to the substrate, and shows some ability to move vertically through the sediments (Abele, 2002; Morton, 2011), with regular reports of A. islandica burrowing several centimeters into the sediment (e.g., Taylor, 1976; Strahl et al., 2011) and remaining there for periods of several weeks. In respect of food, A. islandica is thought to be very selective, feeding only on fresh organic matter, and discarding older organic material lying at the sediment surface (Erlenkeuser, 1976). During prolonged periods (>60 days) of unfavorable conditions (e.g., anoxia) it can switch to anaerobic respiration and a reduced metabolism (Oeschger, 1990; Strahl et al., 2011).
G. glycymeris is found in surface areas of coarse-grained subtidal sediments, thus complementing A. islandica in terms of habitat and usefully extending the area for which shells suitable for long baseline monitoring are available. A study using stable oxygen isotopes in the shell (Berthou et al., 1986) demonstrated that the growth lines of G. glycymeris are formed annually, corresponding to winter shell growth checks. While not quite as long-lived as A. islandica, some individuals can live up to two centuries (Reynolds et al., 2013). Like A. islandica, G. glycymeris grows synchronously within populations.
Because bivalves such as A. islandica and G. glycymeris live in the boundary layer between the sediment and the water column, they are directly exposed to heavy metal pollution (Szefer and Szefer, 1990), and several studies have shown that heavy metal levels in both shells and soft tissue of A. islandica are elevated at polluted sites (Supplementary Table I). Measurements of trace and heavy metals have been carried out on transects through the annual growth increments in shells of A. islandica, showing its suitability as a monitor of contamination through time (Liehr et al., 2005; Dunca et al., 2008). This potential has been enhanced more recently with advances in analytical methods and improved knowledge of biomineralization (e.g., Holland et al., 2014; Shirai et al., 2014; Poulain et al., 2015).
Biomineralization: Environmental vs. Biological Effects
Molluscs construct their shells of calcite, aragonite, or both (mostly aragonite in A. islandica and G. glycymeris). The bulk of the shell is composed of calcium carbonate (CaCO3), with some trace elements that can substitute for calcium (e.g., Mg, Sr) and some organic substances (e.g., proteins, lipids, polysaccharides) that tend to concentrate in the growth lines that separate the wider increments.
The shell formation process is initiated at the early stage of larval development (trochophore) and sequential carbonate deposition continues to contribute to the shell growth after metamorphosis and throughout the entire life of the animal (Marin et al., 2007). The oxygen of the bicarbonate ions () is in isotopic equilibrium with that of the ambient water, enabling seawater temperature reconstructions to be based on stable oxygen isotopes in the carbonate shell [see Section Physical Variables (Temperature, Salinity)]. Most essential elements can diffuse through the mantle epithelia, gills and digestive gland and can be absorbed from ingested food and water. Because of isotopic fractionation, vital effects, and detoxification processes in the various transport pathways, elements other than oxygen are not precipitated in equilibrium with the ambient environment (see Sections Shell Growth—Measurement and Interpretation and Deciphering the Environmental Information in the Shell).
Organic materials from surrounding waters are incorporated in the soft tissue and shell of bivalves. Since soft tissues are continuously added and turned-over by metabolic processes, the stable isotope signature of bivalve soft tissue integrates environmental and dietary signals over the entire water column on relatively short timescales (Ellis et al., 2014). In contrast, material isolated within the shell mineral matrix is deposited in discrete annual increments, is not affected by subsequent metabolic processes (Bayne and Newell, 1983; Serban et al., 1988; Rosenberg and Hughes, 1991; Risk et al., 1996; Quitmyer et al., 1997) and can provide permanent proxy records of the depositional environment for the entire life span of the bivalve (Ellis et al., 2014). The proportions of metabolic and environmental carbon in the shell change with ontogeny during early growth and are likely species-specific (Lorrain et al., 2004), and this should be taken into account when using the stable carbon isotope as an environmental monitor.
Elements are typically reported as a molar ratio to calcium. The partitioning between the water and shell is expressed as a partition coefficient (DElement):
Mg fractionation in inorganic CaCO3 has been attributed to organic molecules within the calcifying solutions regulating the mineralization process (Orme et al., 2001; Elhadj et al., 2006), although it has also been reported that Mg incorporation into the crystal lattice is enhanced during inorganic precipitation (Stephenson et al., 2008). Other divalent cations, e.g., Sr, might also be affected by this fractionation preceding the mineralization process. Negatively charged carboxyl and sulfate groups, which influence electronic charging at the calcification site, are thought to be involved in regulating the biomineralization process and hence also elemental composition within biogenic CaCO3(e.g., Addadi et al., 2006; Marin et al., 2012).
Shell Growth—Measurement and Interpretation
Several studies have shown that annual growth increments in long-lived bivalve shells can be used to establish absolute chronologies for the marine environment, similar to the annually resolved terrestrial records based on tree rings (Witbaard, 1997; Marchitto et al., 2000; Schöne et al., 2002; Schöne, 2003; Scourse et al., 2006; Butler et al., 2010, 2013). By measuring the distance between consecutive growth lines, time series of increment widths (growth increment series; GIS) can be defined for each individual shell. Because the patterns of growth within populations are synchronous, multi-shell chronologies can be constructed by crossdating individual shell GIS. Where the date of death of any individual in the chronology is known, precise calendar dates can be assigned to the whole chronology, which can therefore extend for many hundreds of years before the lifetime of any living specimen (e.g., Butler et al., 2010, 2013). As well as providing the basis for chronology construction, synchronous growth within a population also constitutes prima facie evidence that shell growth is responding to a common environmental driver, allowing environmental changes to be inferred from variation in the growth increment width (Witbaard, 1997; Ambrose et al., 2006; Carroll et al., 2011). Further, environmental indicators can be derived from the geochemical and structural properties of the shell, and these indicators are also precisely dated.
Measurement of growth increments is carried out on the polished surface of a shell that has been sectioned perpendicular to the growth lines along the axis of maximum shell growth (umbo to ventral margin; see Figure 2A). The increments may be measured either in the umbo region or along the outer shell margin (Figure 2B).
Growth in A. islandica does not occur throughout the year, but is restricted to a growth season, which is itself affected by water depth and the depth of the thermocline (Schöne et al., 2005b,c). The duration of the growing season remains more or less the same during ontogeny. The start of growth line formation is thought to occur shortly after the seasonal temperature maximum (Weidman et al., 1994). In addition to the prominent annual growth lines, the existence of daily growth lines in A. islandica has been supported by successfully linking the micro-growth pattern to stable isotope data (Schöne et al., 2005b). With knowledge of daily/seasonal growth rates (Schöne et al., 2005b) it is possible to position geochemical analyses very precisely in time and thus establish very precise proxy records for environmental monitoring.
While food availability is likely the main driver of growth in A. islandica, analysis of A. islandica chronologies (e.g., Butler et al., 2010; Mette et al., 2016), as well as laboratory experiments (Witbaard, 1997) have also shown a link between seawater temperature and shell growth, although this is rather weak in natural settings (Witbaard, 1997; Witbaard et al., 2001; Schöne et al., 2005b,c).
Brocas et al. (2013) constructed cross-dated chronologies from two populations of G. glycymeris, from the east and south coasts of the Isle of Man. They identified a common growth signal in the two populations, indicating that a common environmental driver controls growth across the two sites. The positive correlation between the chronology and SST (sea surface temperature) was found to be much stronger for G. glycymeris than for A. islandica from the same region (Butler et al., 2010). In addition, G. glycymeris chronologies have been shown to reflect synoptic scale signals originating in the North Atlantic (Reynolds et al., 2013). Since the habitat preference of G. glycymeris complements that of A. islandica, it is possible to crossdate G. glycymeris chronologies with those from adjacent populations of A. islandica, allowing longer multi-species chronologies to be constructed.
Deciphering the Environmental Information in the Shell
In this section the existing and potential applications of molluscan sclerochronology to environmental monitoring will be discussed. These applications may make use of information encoded in the shell in various forms, including the growth increment pattern, the shell geochemistry, the shell crystal structure and the metagenome. Advantages and constraints associated with each of these archives will be discussed in the context of the relevant monitoring target.
Metal Pollution
Shell geochemistry has long been investigated as a potential means to record ambient seawater chemistry. The elemental composition of the shell can potentially be used not only to track long-term climate variability (e.g., Lazareth et al., 2003, 2006; Schöne et al., 2005a; Welsh et al., 2011; Butler et al., 2013) and improve climate predications but also to record environmental pollution events (e.g., Raith et al., 1996; Liehr et al., 2005).
Geochemical properties (trace element and heavy metal incorporation) offer a number of possibilities for the reconstruction of environmental variables. The elemental composition of the shell is strongly controlled by element availability and partitioning. The relationship between the chemistry of ambient water and shell biogeochemistry is complicated by multiple confounding factors (Carroll and Romanek, 2008; see refs. in Zuykov et al., 2013). Typically Element/Calcium ratios deviate from thermodynamically predicted values because of changes in growth rate (i.e., ontogeny) and crystal fabric (Swan, 1957; Gillikin et al., 2005a; Schöne, 2013; Schöne et al., 2013; Shirai et al., 2014).
More recently, significant advances in micro-scale analytical techniques have improved understanding of incorporation mechanisms. Calcium carbonate samples (tens of μg) from the outer shell layer are usually obtained by microdrilling/micromilling (Figure 5) for stable isotope mass spectrometry or ICP-OES element analysis, which can achieve a resolution on the order of weeks. Higher resolutions (down to days) can be achieved using laser ablation-inductively coupled plasma-mass spectrometry (LA-ICP MS) or secondary ion mass spectrometry (SIMS). Electron microprobe analysis (EPMA) allows for even higher resolution and provides the means to study the element distribution in bivalve shells with respect to their microstructure (Shirai et al., 2014).
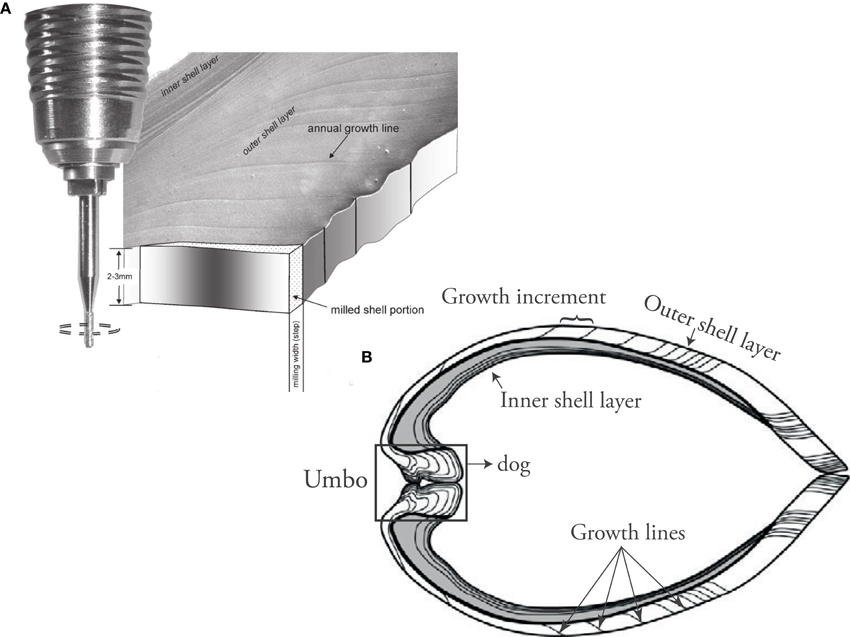
Figure 5. (A) Micromill sampling from shell carbonate for isotope analysis and Element/Ca ICP-MS wet chemistry (Figure from Schöne et al., 2005b). (B) Umbo-ventral margin shell cross section showing the parts of the shell typically used for geochemical analysis in sclerochronology.
Metals occur naturally in the environment, but their concentrations have increased since the beginning of mining activities in the Iron Age (800 BC–AD 100 in western Europe; Hooke, 2000; Breitenlechner et al., 2010; Wells, 2011). Since the industrial period, anthropogenic activities (mining, agriculture, combustion, and the use of metal containing products) have significantly increased pollution locally and globally (Larsen et al., 2011). Non-essential metal pollutants, such as mercury (Hg), cadmium (Cd), and lead (Pb), can affect the central nervous system and cause kidney damage in mammals. The European Union has regulated these metals by adopting the Water Framework Directive (2013/39/EU), in which environmental quality standards (EQSs) of 45 prioritized substances have been defined for water and biota samples. Other metals (e.g., Cu, Zn, Fe, Co, Mo, Se, Mg, Ca, and Mn), while they are an essential part of metabolic and biochemical processes (e.g., enzymes), can cause toxic effects at higher concentrations. Bivalves take up metals both through food and directly from the seawater and incorporate them in their shell and soft tissue. Assuming that metal accumulation is more concentrated in the soft tissue of bivalves (Brown and Depledge, 1998), most research efforts have focussed on soft tissue metal accumulation. However, significant advantages of using the shell to monitor metal contamination include less variability (Bourgoin, 1990; Lingard et al., 1992), serial incorporation of elements over the entire period of shell formation, higher preservation potential even after the organism's death, and relatively cheap and easy storage (Protasowicki et al., 2007).
The preference in monitoring programmes for the use of soft tissue in bivalves to record and measure pollutant concentrations in water (e.g., Goldberg et al., 1978; Larsen et al., 2011) likely reflects generally greater concentrations of metals in some organs; as a result there is little information in the literature about the quantitative relationship between metal concentrations in soft tissue and in the shell. For example, Pb is bioaccumulated by living organisms and more particularly by marine invertebrates (Neff, 2002). Chow et al. (1976) found that both the soft tissue and the shell of mussels reflected Pb concentrations in the environment, with higher concentrations in more urban areas indicating anthropogenic pollution. If such relationships can be reliably modeled, some monitoring studies could be simplified, since shells are easier to store than soft tissue (Koide et al., 1982).
Koide et al. (1982) found that Zn, Pb, and Cd levels were higher in the tissues of Mytilus edulis and M. californianus than in their shells, while conversely Cu concentrations were higher in the shells. Bourgoin (1990) also found higher Pb levels in the tissues of M. edulis than in the shell (specifically the nacre). In a trace metal study using M. edulis from the Baltic Sea, Protasowicki et al. (2007) emphasized the importance of the actual metal bioavailability at the sampling location. For the cockle Chione (Austrovenus) stutchburyi, Pb concentrations in the shell were similar to those in the soft tissues (based on dry weight; Purchase and Fergusson, 1986). Moreover, Gillikin et al. (2005b) found that there is large inter- and intra-annual Pb variability within shells of the clam Mercenaria mercenaria, suggesting that year to year, as well as intra-annual variations in Pb/Ca ratios, could potentially be interpreted (Figure 6). Even though it appears that concentrations of metals are generally lower in the shell compared to tissue, the feasibility of using bivalve shells for investigating metal contamination in the marine environment has nevertheless been demonstrated, and it may be that the practical advantages of using the chemically stable and easily storable shells outweigh the analytical advantages of the higher concentrations in the tissue, increasingly so as more sensitive techniques for measuring the elemental makeup of shells are developed (please see Supplementary Table I).
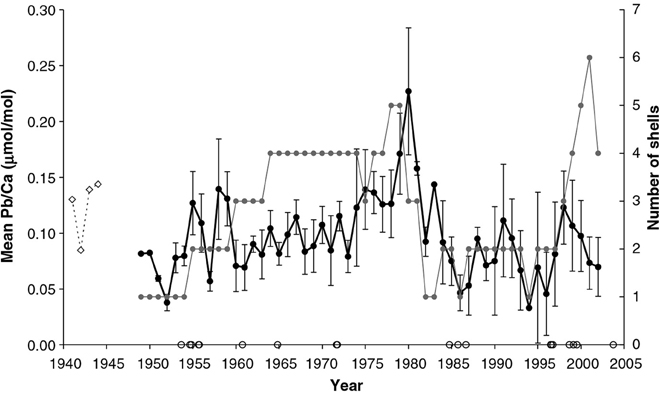
Figure 6. Mean of annually sampled Pb/Ca ratios from 11 M. mercenaria shells. The gray line and symbols indicate the number of M. mercenaria shells each mean is based on and error bars representing standard errors. The open symbols on the x-axis represent hurricane years (data from NCSCO, 2004). For comparison, a fossil Pliocene shell (P1) is shown (dashed line, arbitrarily positioned at 1941–1944 (Figure from Gillikin et al., 2005b).
Arctica islandica has been shown to be an effective bioindicator for contaminated sediments (Steimle et al., 1986), and its longevity (e.g., Ropes et al., 1984) makes it particularly suitable as a record of historical contamination events and of long term trends. By carrying out measurements in successive internal growth increments it is possible to obtain a chronological reconstruction of the metal content. Raith et al. (1996) showed using LA-ICP-MS that the Pb concentration in the shell of A. islandica reflects changes in seawater metal pollution. This study indicated that high-resolution methods such as LA-ICP-MS offer an accurate method of determining pollution levels in the environment, and can potentially be used in tracing the source of the pollution. This was confirmed by Richardson (2001) for Pb and Zn using shells of the horse mussel Modiolus modiolus. In a detailed comparative study, Liehr et al. (2005) investigated the potential use of A. islandica for pollutant biomonitoring, analysing the heavy metal concentrations in shells and soft body tissue of specimens from the western Baltic Sea (Figure 7). Samples were taken from an historic dump site in the inner Mecklenburg Bight and from an adjacent, less contaminated site (representing background contamination of the western Baltic Sea). The soft tissue from the dumping site had significantly higher Pb and Cu concentrations than those from the reference site. No difference in Zn concentrations was found. For the shell, Liehr et al. (2005) used LA-ICP-MS to analyze Cu, Pb and Zn concentrations and found that these were higher at the dumping site, indicative of contamination (Figure 7). The chronological reconstruction (profile) of the measured metals in the shell showed no significant trend, most likely because the analyzed specimens only reached an age of ~35 years and did not record the initial contamination (40–45 years). Liehr et al. (2005) concluded that processes such as bioturbation and other physio-chemical processes at the sediment water interface might affect metal incorporation into the shell. Nevertheless, this study showed the potential for pollutant biomonitoring using sclerochronological methods.
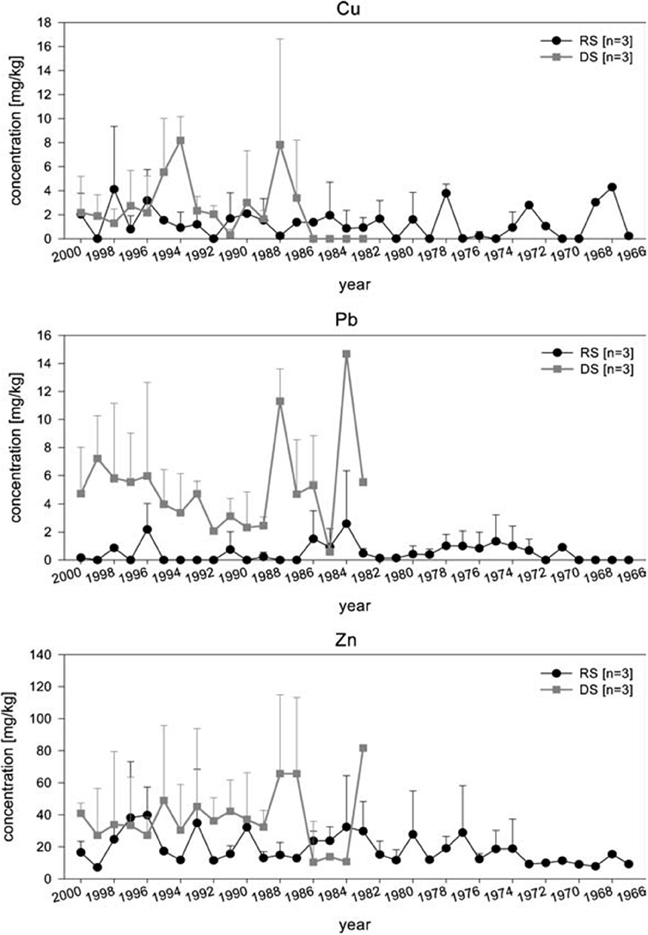
Figure 7. Analysis of Zn, Pb, and Cu concentrations in shells of three specimens, each from the dumping and the reference site were measured using LA–ICP–MS. Applying this method, allows to determine the heavy metal exposure per growth year of A. islandica. Chronological review of heavy metal concentration in the shells of A. islandica from the dumping site (DS) and reference site (RS) in August 2001 using laser ISP–MS. Measurements where performed on the cardinal tooth (Figure from Liehr et al., 2005).
Uranium is less well-studied in bivalve shells; examples include a study of U/Ca in aragonite of the shells of the marine cockle, Cerastoderma edule (Price and Pearce, 1997) and a study of U/Ca ratios in aragonite of freshwater mussel shells as an indicator of uranium pollution through time from a copper–uranium mine in Australia (Markich et al., 2002).
Barite (BaSO4) is a naturally occurring mineral in many sediments and is a major component of almost all drilling muds (Hartley, 1996). Although barite in drilling fluids and PW is usually relatively inert (Neff, 2002), under reducing conditions it can dissolve slowly, releasing Ba into the overlying water column (Boothe and Presley, 1989). It may be possible to use the Ba/Ca ratio in bivalve shells to track the release over time of Ba in the proximity of drill cutting disposal. Marali et al. (2016) have measured Ba/Ca ratios in multicentennial A. islandica chronologies from four sites (Gulf of Maine, Iceland, Faroe Islands, and Isle of Man) to show that Ba/Cashell spikes are synchronous within chronologies, and that this synchroneity is independent of ontogenetic age.
Furthermore, Faubel et al. (2008), Lopes-Lima et al. (2012), Nuñez et al. (2012), and Zuykov et al. (2013) demonstrated that several metals, fabricated metal nanoparticles, and organic contaminants, can bioaccumulate in low concentrations in the extrapallial fluid, and are able to disturb the calcification of shell by forming distinct structures on the internal shell surface.
Physical Variables (Temperature, Salinity)
Temperature and Salinity
Oxygen isotopes. The stable oxygen isotope ratio (δ18Oshell) of carbonate structures reflects both temperature-driven fractionation and the δ18O of the ambient seawater (δ18Osw) from which it is precipitated. The relationship between δ18Oshell and seawater salinity is complicated because of the varying isotopic composition of freshwater and the meridional evaporation/precipitation pattern (e.g., Schmidt et al., 2007). Commonly, in order to calculate temperatures from δ18Oshell, a slightly modified (Sharp, 2007) version of the (Grossman and Ku, 1986) equation is used:
with δ18Oshell calibrated to the Vienna PDB scale and δ18Osw calibrated to the V-SMOW scale. A δ18Oshell change of 1%0 is equivalent to a seawater temperature change of ~4.34°C, assuming constant δ18Osw (and hence constant salinity). As an example, Marsh et al. (1999) used δ18Oshell analysis on annual bands from A. islandica, together with empirical modeling, as proxy evidence for a cold episode in the Gulf of Maine in the 1880s. In a later study, a subseasonal temperature record was established, based on a single A. islandica specimen from the North Sea, which indicated a 1°C warming of SST over a 100 year period (Schöne et al., 2004; Figure 8). However, note that the reconstruction of temperature based on shell δ18O is complicated by likely variability in δ18Osw, particularly in coastal waters. It is therefore important to isolate a reliable and independent proxy for either temperature or salinity so that both variables can be reconstructed without ambiguity.
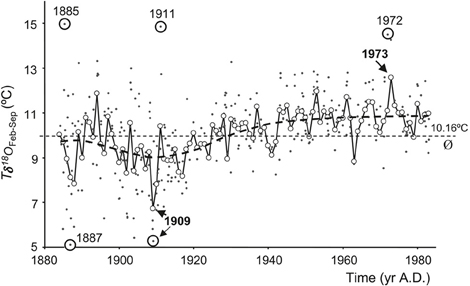
Figure 8. A 100-year temperature record calculated from oxygen isotope values of Arctica islandica, covering the period 1884 to 1983. Individual δ18Oaragonite-derived temperatures (Tδ18O) are plotted as dots. Mean temperatures (open circles) during February through September [Tδ18O (Feb–Sep)] were calculated as weighted averages from individual Tδ18O values. Exceptional Tδ18O and Tδ18O (Feb–Sep) values are highlighted. Long-term mean as indicated by the dashed line is 10.16°C (Figure from Schöne et al., 2004).
Royer et al. (2013) demonstrated that seawater temperatures can be accurately estimated through sclerochronological and δ18O analyses using shells of G. glycymeris. Comparing δ18Oshell-derived temperatures with water temperatures measured at several monitoring stations in the Bay of Brest, the study found that the seasonal pattern of δ18Oshell data indicated a May–October growing season. Furthermore, based on reconstructed temperatures from two different sampling sites, Royer et al. (2013) inferred that shell growth is constrained by a lower thermal threshold of ~13°C.
Potential methods for independent temperature calibration.
Other potential methods for the independent reconstruction of temperatures from calcium carbonate shells include Δ47 clumped isotope thermometry (Ghosh et al., 2006; Henkes et al., 2013) and δ44/40Ca paleothermometry (e.g., Nagler et al., 2006; Hippler et al., 2013). While these techniques show promise, they are limited at present because of the required sample amount and the large analytical uncertainty.
Sr/Ca and Mg/Ca. The partitioning of Sr/Ca and Mg/Ca is strongly affected by a complicated interplay between biological, physio-chemical and kinetic processes during biomineralization and has so far only yielded controversial results in respect of thermometry applications (Toland et al., 2000; Gaetani and Cohen, 2006; Foster et al., 2008, 2009; Schöne et al., 2011b, 2013). Future ultra-high-resolution analyses are needed to test whether particular portions of the aragonitic shell (e.g., near the growth lines) of A. islandica or other bivalves can consistently be used for Sr/Ca or Mg/Ca (paleo)thermometry.
The crystal fabric in A. islandica has been shown to be influenced by environmental variables (Schöne et al., 2013; Stemmer et al., 2013). Heterogeneities in the crystal fabric coincide with heterogeneous distribution of Sr/Ca, Mg/Ca, and Ba/Ca (Schöne et al., 2010, 2013; Shirai et al., 2014). During the formation of the annual growth lines, extremely slow growth occurs and Sr/Ca and Mg/Ca are seemingly incorporated in equilibrium with the ambient seawater. However, during the rest of the growing season, ratios of Sr/Ca and Mg/Ca remained below thermodynamic equilibrium values. The incorporation of Ba does not seem to be related to changes in crystal fabric and appears to be less influenced by vital effects, as Ba/Ca peaks occur erratically at different times of the year.
Evidence so far suggests that Sr/Ca variations are minimally influenced by temperature (e.g., Schöne et al., 2013; Shirai et al., 2014). Schöne et al. (2013) concluded, from results consistent with those of Foster et al. (2009), that crystal size, shape, and orientation are very influential in trace element variability. Similarly, Shirai et al. (2014), using very high analytical resolution showed that the Sr/Ca difference between the outer and middle shell layers were associated with microstructural differences (Figure 9). They concluded that crystal morphology cannot entirely explain the compositional changes of Sr within the shell, since while different Sr/Ca ratios were observed between the irregular prismatic crystals and the adjacent acicular crystals, the Sr/Ca ratio was not correlated with the length and shape of the crystals. It seems that organic composition at the site of calcification is an additional control on Sr/Cashell.
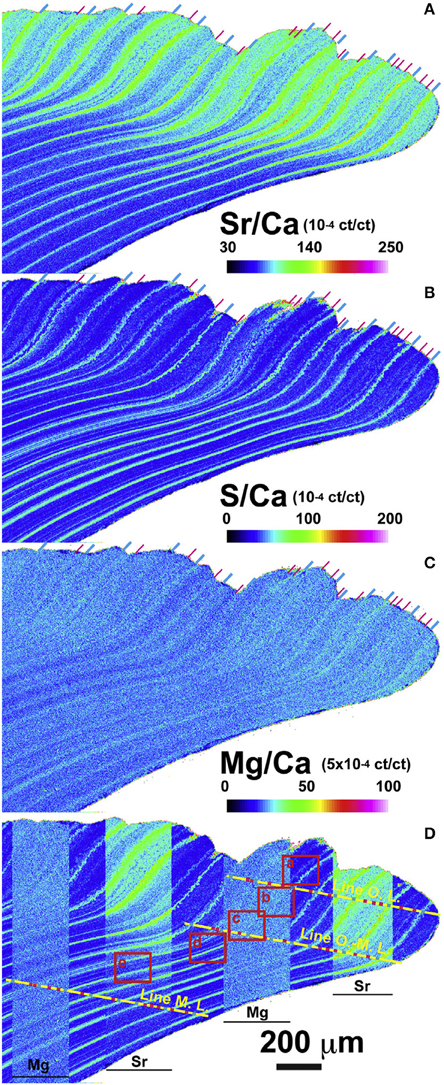
Figure 9. Electron probe microanalyses (EPMA) showing the micrometer-scale distribution of (A) Sr/Ca, (B) S/Ca, (C) Mg/Ca in the shell of Arctica islandica. Signal intensity (cumulative count) ratio is illustrated as a map using a color scale (ct/ct: count/count) shown at lower right of each elemental map. (D) Sr/Ca and Mg/Ca maps are partly overlapped on S/Ca map for the comparison among each elemental map. Position of annual and sub-annual growth lines are clearly recognized and indicated by the blue and pink lines, respectively, at the top of shell signal images and on the yellow lines (Figure from Shirai et al., 2014).
Na/ca. Sodium (Na) has been proposed both as an indicator of post depositional alteration and as a proxy for salinity. As one of the major constituents of sea salt, Na has clear potential as a salinity proxy (the other constituent, like chlorine, occurs at far lower concentrations Kitano et al., 1975). Early research on modern marine mollusc shells suggested that seawater salinity had the strongest control on shell Na content (Rucker and Valentine, 1961; Pilkey and Harris, 1966; Gordon et al., 1970; Kitano et al., 1975), although at the time it was impossible to tell whether Na was bound in the crystal lattice or in microscopic seawater inclusions. However, the very low chlorine concentrations suggest that Na is structurally bound in the crystal lattice (Ishikawa and Ichikuni, 1984). Sodium ions cannot directly substitute for Ca2+ (because of the difference in ion radius and charge) during calcite precipitation (Ishikawa and Ichikuni, 1984). Primarily, Na+ incorporation depends on the activity of Na in seawater, which is a function of its concentration and, to a lesser extent, its activity coefficient (Ishikawa and Ichikuni, 1984). Increasing salinity (and hence [Na+]) increases the activity of Na whereas seawater temperature, over the relevant range, has only a minor effect on the activity coefficient of Na. Hence the effect of temperature on Na incorporation is negligible (Ishikawa and Ichikuni, 1984; Delaney et al., 1985; Lea et al., 1999; Zeebe and Wolf-Gladrow, 2001). Findlater et al. (2014) found a clear relationship between Na concentration and inferred salinity in fossil and modern bivalve shells. It still remains to be tested whether Na incorporation is species-specific, whether the same relationship between Na and salinity occurs in both aragonite and calcite, and how sensitive the potential salinity proxy is.
Crystallography. A recent study investigated the use of the crystal structure of the common cockle, Cerastoderma edule, as a proxy for seawater temperature and salinity (Milano et al., 2016). Living specimens, exhibiting shell growth increments with tidal resolution (Figures 2C–E), were collected after a year of continuous temperature and salinity measurements. Using scanning microscopy, shell microstructures were analyzed, showing that size and shape of the mesocrystals strongly correlated with water temperature during the growing season (May–September). The results suggest that shell microstructures of C. edule may serve as a new, independent proxy for water temperature. Future research will be needed to test whether the relationship between calcite microstructures and temperature can also be observed in other species.
Ecosystem Variables (Trophic Levels, Food Supply, Metagenome)
Food web analysis using stable isotopes in the organic shell material
Stable isotope analysis is frequently used to investigate patterns of productivity and (based on isotopic fractionation) degradation and metabolic transfer of organic matter through the water column (Peterson and Fry, 1987). Carbon and nitrogen isotope ratios record information about the types of primary producers and further alterations as carbon is transferred through the food web. Therefore, carbon and nitrogen isotopes can be used to assess different classes of primary producers or benthic vs. pelagic marine production (DeNiro and Epstein, 1978; Rounick and Winterbourn, 1986; Farquhar et al., 1989).
O'Donnell et al. (2007) showed that proteins in the organic matrix of modern and fossil Mercenaria shells can resolve spatial and temporal changes in dietary carbon sources. However, the organic constituents in shell mineral material do not faithfully record whole-diet carbon isotopic ratios. Controlled feeding experiments have shown that an offset of between 1 and 6‰ can exist between diet and bone collagen bulk δ13C values, depending on the composition of the diet (DeNiro and Epstein, 1978; Howland et al., 2003). Nevertheless, measurements of compound specific δ13C should account for variation in the amino acid makeup of the organic matrix (Howland et al., 2003) and yield results directly reflective of dietary sources (Sykes et al., 1995; Ellis et al., 2014).
Combining stable carbon isotopes with nitrogen isotopes could refine modern and historical trophic assessments and distinguish natural from anthropogenic influences on coastal ecosystems, e.g., coastal nitrogen input, and can ultimately be used to define effects of eutrophication on an ecosystem-level (Carmichael R. H. et al., 2004; Carmichael R. et al., 2004; Valiela, 2009). Delong and Thorp (2006) have used stable carbon and nitrogen isotopes in the shell periostracum to study food web dynamics and changes in trophic complexity over time. Using very high resolution techniques, it may also be possible to analyse stable nitrogen isotopes in the organic fraction of the shell matrix.
Food web analysis using stable isotopes in the inorganic shell material
Studies of long-term (multidecadal to century-long) δ13Cshell timeseries in A. islandica have indicated that age-related vital effects are limited to the shell portions close to the umbo of individuals that have exhibited very rapid growth during early ontogeny (Butler et al., 2011). Using an extensive set of >3500 individual δ13Cshell values, Schöne et al. (2011a) found that shell carbonate is secreted with a constant offset from expected equilibrium (by −1.54 to −2.7 ± 0.2‰), which would correspond, assuming δ13Cfood ~ −25‰, (Erlenkeuser, 1976), to a contribution of metabolic carbon of between 6.2 and 10.8 ± 0.8%. Using data from laboratory and in situ experiments, Beirne et al. (2012) later confirmed these findings. Further, they supported the conclusion that δ13Cshell values of A. islandica provide a robust proxy for seawater dissolved inorganic carbon (DIC) values, since they did not observe an ontogenetic effect or an impact of growth rates on the measured δ13Cshell values. They found the following relationship between δ13Cshell and δ13CDIC:
Microchemical analysis of fish otoliths has recently been suggested as a method of food web analysis, for example by determining the residency of the fish (how long and where they reside e.g., on oil structures; Fowler et al., 2015). Trace elements are incorporated into the calcium carbonate of the otoliths, leaving a record within the otoliths of residency seawater conditions (Campana et al., 2000; Gillanders and Kingsford, 2000; Fowler et al., 2015). It may be possible to observe distinct geochemical signatures in the otolith, characteristic of particular structures, that can potentially be used to evaluate residence times at individual sites and help assess habitat value and contribute to decommissioning decisions.
Although the potential of otolith microchemistry for long-term reconstructions is limited by the relatively short lifespans of fish (usually <25 years), it may be possible to construct otolith chronologies using otoliths of known date held in national fisheries archives. Ultimately, the combination of fish otolith chronologies with centennial scale bivalve chronologies may enable researchers to link pelagic and benthic processes over extended periods.
Mn/Ca
Manganese (Mn2+) has been suggested as a proxy for increased riverine discharge events (Lazareth et al., 2003) and productivity (e.g., Vander Putten et al., 2000; Lazareth et al., 2003). Intra-shell variation in Mn/Ca may reflect the seasonal variation of seawater Mn2+ concentrations (Freitas et al., 2006; Bougeois et al., 2014), indicating that redox processes control its concentration in seawater. One source of marine manganese (Mn2+) is terrestrial input via rivers. Normally it is slowly removed from solution by oxidation to (Mn4+), but reducing conditions in sediments and oxygen depletion within the water column can recycle Mn2+ back into solution. Hence oxygen depletion potentially supports Mn incorporation into the shell. Markich and Jeffree (1994) showed that Mn2+ can be substituted for Ca2+ during uptake from the external medium. Temperature increase may also support incorporation of manganese as a result of increased Mn2+ uptake and transfer to the calcification site (Wada and Fujinuki, 1976).
Freitas et al. (2006) suggested that the intra-annual variation of Mn/Cashell reflects the seasonal variation of seawater Mn2+ concentrations, but they also point out that further research is necessary to test the effect of other variables controlling seawater Mn2+ concentrations (e.g., primary productivity, oxygen concentrations, temperature). Manganese has also been used as a fast chemical marking technique using cathodoluminescence; this might be an additional method to estimate shell growth rate (e.g., Langlet et al., 2006; Barbin et al., 2008; Lartaud et al., 2010). Potentially, Mn/Ca concentrations could be used in monitoring and baseline studies concerned with eutrophication, hypoxia and deep sea mineral exploration (e.g., manganese crusts).
Ba/Ca
Barium is a minor constituent of seawater, found as Ba2+ at very low concentrations (~34 nmol/L). Planktonic organic matter is thought to deliver Ba to the benthos in particulate form, where it is digested by filter feeders. The incorporation of Ba into the shell is therefore linked to primary productivity and metabolic activity (Lazareth et al., 2003; Carré et al., 2006). Nevertheless, a recent study using A. islandica indicated that Ba/Ca peaks (>40 μmol/mol) can occur at different times during the growing season, raising further questions about the factors that influence Ba/Ca concentrations in A. islandica shells (Schöne et al., 2013) and showing the need to better understand the controls and mechanisms of Ba incorporation. Future studies could use multiple shell proxies and culturing experiments to identify the major factors controlling Ba incorporation and to refine proxy calibrations. For example, following a suggestion by Thébault et al. (2009) that the Li/Ca ratio in A. islandica may be associated with Li-rich silicate particles and terrestrial weathering and could ultimately be used as a proxy for river discharge, it may be possible to combine Li/Ca and Ba/Ca measurements to determine the extent to which terrigenous sediment input by river discharge is contributing to the Ba distribution in shell material. There are other studies which suggest that Ba/Ca ratios in bivalve shells track intraannual variability in river discharge (Carroll et al., 2009) or salinity (Gillikin et al., 2006, 2008; Poulain et al., 2015). Poulain et al. (2015) confirmed the conclusion of Barats et al. (2009), working with only the background Ba/Ca signal, that there is a strong inverse correlation between salinity and Ba/Cashell, which suggests that Ba/Cashell could offer a high-resolution proxy for the reconstruction of salinity fluctuations within estuarine and nearshore waters and could also be used to distinguish the salinity and temperature signals in the stable oxygen isotope ratio. They also found a positive correlation between Ba/Cashell and Ba/Casw.
Poulain et al. (2015), using the Manila clam Ruditapes philippinarum, demonstrated that the seawater Ba2+ concentration was reflected at almost daily resolution in Ba/Cashell and might be used as a proxy with very high temporal resolution. The same study also found variable Mg/Cashell ratios, suggesting that the incorporation of magnesium into shell carbonate is strongly regulated by the organism and not by environmental conditions.
Mo/Ca
Molybdenum (Mo) is one of the most abundant transition group metals in seawater, mainly present as the oxyanion in oxygenated environments (Collier, 1985). Mo is considered to be a conservative element in seawater, with low concentrations around 110 nmol/L and little apparent influence of biogeochemical processes on its concentration (Collier, 1985). Coastal Mo distribution is also influenced by freshwater-seawater mixing (Dalai et al., 2005). Some studies, however, have indicated non-conservative behavior in coastal waters, both at the sediment water interface (SWI; Crusius et al., 1996; Adelson et al., 2001; Chaillou et al., 2002; Elbaz-Poulichet et al., 2005) and in the water column (Tuit and Ravizza, 2003; Dellwig et al., 2007). When phytoplankton is decomposed by bacteria, organic compounds are released and Mo-enriched aggregates are subsequently formed which settle on the sediment water interface where they are rapidly decomposed by microbial activity, contributing to a substantial release of Mo in bottom waters (Dellwig et al., 2007). Barats et al. (2010) evaluated ([Mo]/[Ca])shell profiles of Pecten maximus as a potential record of specific biogeochemical processes occurring at the SWI. They found that Mo incorporation is promoted by the significant spring pelagic productivity, although they could not directly link ([Mo]/[Ca])shell maxima with specific phytoplankton species. Barats et al. (2010) found that the background partition coefficient (DMo) indicates anionic Mo precipitation pathways within the calcium carbonate shell but also consider a particulate phase uptake of Mo into the shell to play a role. In order to explain ([Mo]/[Ca])shell maxima in the tropical bivalve species Comptopallium radula, Thébault et al. (2009) suggested the ingestion of N2-fixing cyanobacteria. This seems to be confirmed by the 7-year Mo/Cashell record with late spring maxima, which were not directly related to the spring bloom biomass maximum but rather to a post-bloom period characterized by nutrient depletion (silicic acid and nitrate depletion) and Pseudonitschia spp. dominance. Mo inputs at the SWI can be induced by a diatom biogenic material downward flux and might therefore enrich Mo/Ca ratios of scallop shells at the SWI. Hence, ([Mo]/[Ca])shell records in Pecten maximus may serve as a new proxy for biomonitoring studies of primary production, including eutrophication, in temperate coastal environments.
Ocean Acidification
Crystallography
Increased pCO2 can affect physiology, acid-base homeostasis and biomineralization in bivalves, including decreased growth rates and altered shell structure, composition and mechanical properties (Michaelidis et al., 2005; Gazeau et al., 2007, 2013; Kurihara et al., 2007; Beesley et al., 2008; Kurihara, 2008; Ellis et al., 2009; Dickinson et al., 2012; Ivanina et al., 2013; Fitzer et al., 2014a,b, 2015, 2016).
Milano et al. (2016) (see Biomineralization: Environmental vs. Biological Effects) showed that crystallography strongly correlates with water temperature. However, changes in the carbonate chemistry of ambient seawater (i.e., pH), related to ocean acidification, are also likely to affect calcification and cause changes in the microstructure of shell calcite. A number of studies have recently shown effects of ocean acidification on shell material properties and crystallography (Fitzer et al., 2014a,b, 2015, 2016). Fitzer et al. (2014a) found that M. edulis appears to improve its ability to continue biomineralization, although this occurs at some cost to the structural integrity of the mussel shell. At pCO2 (>1000 μatm) various mechanisms that support biomineralization were observed, including increased protein metabolism, chitinase mRNA and tyrosinase gene expression. Later, Fitzer et al. (2016) confirmed that OA reduces the crystallographic control of shell formation. The study suggests that in order to combat shell damage, more amorphous calcium carbonate formation is induced, lowering the crystallographic control in mussels.
U/Ca
A study by Gillikin and Dehairs (2013) investigated the potential of shell U/Ca as a proxy for ocean acidification (OA). They measured U/Cashell in the aragonitic clam Saxidomus giganteus. Since the elemental analyses were perfectly aligned with δ18O analyses (Gillikin et al., 2005a), it was possible to assign inter- and intra-annual dates using the δ18O profiles, allowing a direct comparison of elemental profiles between two shells. The analyzed shells exhibited seasonal cycles in U/Cashell up to ~6 years of growth, after which the concentration decreased below the detection limit. The authors concluded that U/Ca ratios in S. giganteus shells are not controlled by pH (or []). However, it has to be pointed out that the U/Ca data was only compared to pH and not to other variables in the carbonate system.
Future Application Approaches
Valve-Movement Behavior in Bivalves—A Tool to Calibrate Shell Signatures and Potential Applications for Water Quality Control
Valve movement behavior of bivalves can be used to indicate physiological rate functions (e.g., feeding rate). Studies on A. islandica have shown that the frequency of siphon opening and growth of the shell and tissue were strongly related (Witbaard, 1997). Generally, filter-feeding bivalves reduce their filtration rate by reducing or completely closing their valve-gape (e.g., Riisgård and Larsen, 2015). Video recordings of valve-gape responses of M. edulis to absence or presence of algal cells in the ambient water have revealed that the critical algal concentration (at which concentration the bivalve closes its valves) is between 0.5 and 0.9 mg chl a l−1 (Riisgård et al., 2006; Pascoe et al., 2009).
The principle of a valve position monitor is based on the measurement of electromagnetic field strength between two electronic sensors permanently fixed on the outside of each of the valves and facing each other perpendicularly. Both sensors are connected to a data logger and an energy source (battery). On a pre-set frequency, one of the sensors receives an electric pulse, producing an electro-magnetic field that is detected by the sensor on the opposite valve. What is recorded is the strength of the electro-magnetic field, which depends on the distance between the two sensors (i.e., the valve opening). The distance between the sensors is expressed as the percentage of the signal at maximum valve opening. The time-stamped signals are transferred to the data logger and saved, enabling valve opening behavior to be monitored over time, and allowing it to be related to different a(biotic) parameters (e.g., algal concentrations, sediment turbidity, metal/toxin concentrations in seawater).
High frequency measurements have been carried out to address fine-scale bivalve behavioral physiology (e.g., Wilson et al., 2005) in detail. Such techniques may involve: assessment of valve gape, siphon movements (changes in aperture), filtration and pumping behavior in relation to associated environmental parameters such as depth, light, temperature, particulate matter, food availability, and predator interactions (e.g. Ropert-Coudert and Wilson, 2004).
Several studies (e.g., Manley and Davenport, 1979; Kramer et al., 1989; Huebner and Pynnönen, 1992; Markich et al., 1996; Fdil et al., 2006; Schwartzmann et al., 2011) have confirmed that valve movement behavior can be used to sensitively quantify biological reactions in real-time (Figure 10) for assessing the toxicological effects of metal exposures. The observations found that upon exposure to toxic concentrations of metals, bivalves have the ability to reduce the exposure of their soft tissues for extended periods by closing their valve (Manley and Davenport, 1979; Kramer et al., 1989; Salánki and Balogh, 1989; Huebner and Pynnönen, 1992).
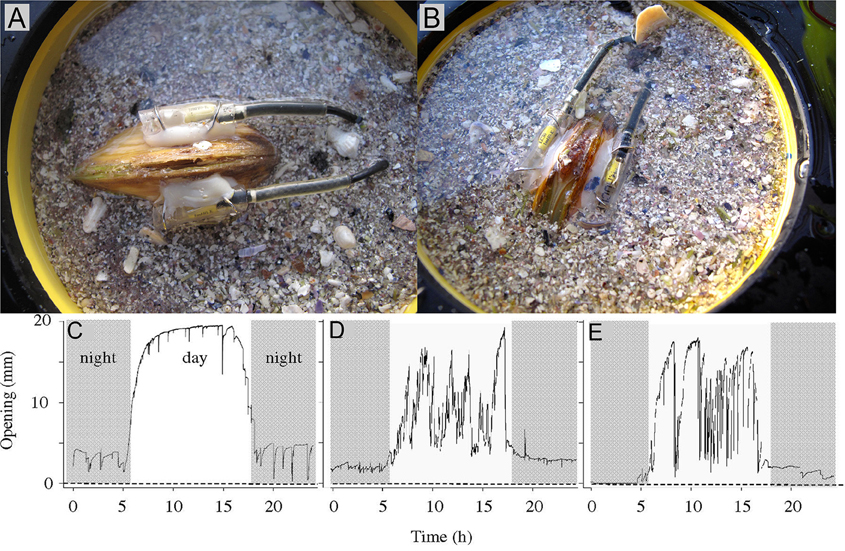
Figure 10. Illustration of the principle of a valve position monitor. Photo attached electric sensors to monitor the valve position on a living specimen of A. islandica with (A) closed valves and (B) open valves. Schwartzmann et al. (2011) recorded typical disturbed daily valve behaviors in giant clams Hippopus hippopus. (C) Typical daily valve behavior in a resting giant clam. (D) Clam during the swell associated with Cyclone Gene (31 January 2008). (E) Clam after 3 weeks at temperatures ranging from 27 to 28°C (02 April 2008; sea surface temperature = 27.7°C) (Photo: Rob Witbaard, Figures C–E from Schwartzmann et al., 2011).
Hence, bivalves are potentially useful as biological early warning systems of water quality (Matthias and Römpp, 1994; de Zwart et al., 1995). Furthermore, observations of bivalve gape and the siphon area might find limited application in areas where turbidity is high or for monitoring burrowing behavior in bivalves (Robson et al., 2009). Although monitoring of valve gape behavior in bivalves has been reported in several studies, no published research has yet focused on connecting shell growth and growth increment patterns with valve gape behavior. If such a connection can be made (Ballesta-Artero et al., 2016, Figure 10), there is potential to use shells to indicate historic changes in levels of nutrient supply, or the presence of threshold levels of toxins/harmful substances in the ambient environment.
Conclusions
We find significant potential in the use of proxy archives in bivalve shells to establish long baseline conditions for environmental monitoring. For very long baselines, this is linked to the longevity and capacity for crossdating of certain species (in particular Arctica islandica and Glycymeris glycymeris), but other, less long-lived species can still be very useful, especially in remote areas such as parts of the Arctic that lack consistent instrumental records (Ambrose et al., 2006; Carroll et al., 2014).
Although the soft tissues in bivalves have long been used in biomonitoring, their use for contamination rate and recovery trend assessment relies on repeated measurements, which are labor intensive and expensive. Furthermore, toxins and heavy metals which are not excreted accumulate in the body tissue, so that concentration changes in the environment are overprinted over time by biological processes, making the recovery of historical changes in these contaminants difficult. Signals in the shell (Figure 11), on the other hand, constitute a stable and temporally sequential archive whose value and significance will likely increase as more sensitive measurement techniques allow analyses to be carried out using smaller amounts of material, and as more linkages are made between shell geochemistry and the ambient environment.
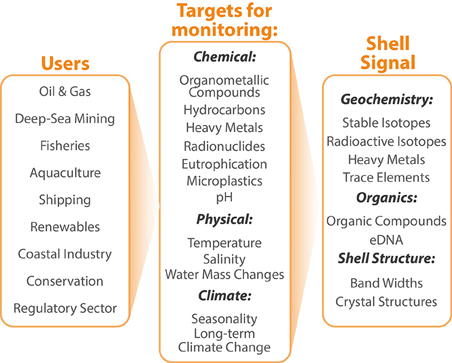
Figure 11. Summary of potential users, monitoring targets and shell signals that can make use of bivalve sclerochronology.
Specifically, the incorporation of elements into the shell matrix can be linked to environmental changes (e.g., variability of elements in seawater, primary productivity, eutrophication; Barats et al., 2008, 2009, 2010; Gillikin et al., 2006; Holland et al., 2014), so that trace element and heavy metal concentrations in bivalve shells can provide suitable bioindicators (Figure 11) for some descriptors of the Marine Framework Strategy Directive. It remains the case that more needs to be known about the relationship between bioavailability and incorporation of elements into the shell calcite, so that the shell concentrations can be robustly interpreted. Detailed investigations of the mechanisms of incorporation into the shell material will be an essential part of future research. For example, by undertaking continuous sampling and analysis of the composition of the extra pallial fluid (EPF) during controlled growth experiments in culture, shell geochemistry could be related to preset culture parameters (e.g., seawater composition), and the composition of the EPF. Another valuable direction of research would be the analysis of heavy metal isotope ratios in order to assign specific sources to metal contamination.
Further insight into the incorporation of trace elements, heavy metals or other contaminants into the shell can be obtained from studies of bivalve behavior in highly fluctuating environments (e.g., Jørgensen, 1988; Wilson et al., 2005, 2008; Robson et al., 2007, 2009; Riisgård and Larsen, 2015). Ultimately, this information will help researchers to understand in detail the physico-chemical interactions between the environment and the animal, and in particular the impact of biological effects and environmental variables on shell geochemistry and growth. For example, techniques are now available for studying valve gape behavior and filtration activity and connecting them to shell growth. Combining observations of long-term valve gape behavior with continuous measurement of seawater chemistry and subsequent measurement of shell geochemistry could help to better understand the processes by which trace elements, heavy metals and other harmful substances are incorporated into the shell and the mechanisms that control the calcification processes.
Other environmental proxies in the shell include the stable isotopes of oxygen, nitrogen and carbon and the shell microstructure (e.g., Raith et al., 1996; Liehr et al., 2005; Lazareth et al., 2006; Welsh et al., 2011), as well as the increment widths themselves which can be an effective indication of nutrients in the environment.
Reduced shell growth can be indicative of less favorable conditions, for example those induced by anthropogenic disturbance (Stott et al., 2010). Increased shell growth can indicate higher nutrient supply (including eutrophication) which can be linked to aquaculture (Stott et al., 2010). Physical damage to larger shells (scars) often reflects impact with fishing gear (Gilkinson et al., 1998; Ramsay et al., 2000), while in smaller shells it is more likely to be a result of factors other than fishing (predator attacks or reburrowing).
The range of applications based on sclerochronology now offers a wide and increasing repertoire of techniques for monitoring natural and anthropogenic environmental variability and distinguishing between them, with applications to a broad range of commercial and regulatory users (Figure 11). Future research should be targeted at understanding the processes of incorporation into the shell, and at developing regionally specific and species specific calibrations to enable the robust interpretation of the shell geochemistry.
Author Contributions
The Leading author, JS was responsible for the conception of the work, information collection, analysis and interpretation, as well as drafting the article. PB and MC were responsible for the conception of the work, the critical revision of the article and the final approval of the version to be published. JH was involved in the conception of the work, the critical revision of the article.
Funding
Funding for this study was kindly provided by the EU within the framework (FP7) of the Marie Curie International Training Network ARAMACC (604802). Additional funding for MLC was provided by the Research Council of Norway (Project # 227046).
Conflict of Interest Statement
The authors declare that the research was conducted in the absence of any commercial or financial relationships that could be construed as a potential conflict of interest.
Supplementary Material
The Supplementary Material for this article can be found online at: http://journal.frontiersin.org/article/10.3389/fmars.2016.00176
References
Addadi, L., Joester, D., Nudelman, F., and Weiner, S. (2006). Mollusk shell formation: a source of new concepts for understanding biomineralization processes. Chem. Eur. J. 12, 980–987. doi: 10.1002/chem.200500980
Adelson, J. M., Helz, G. R., and Miller, C. V. (2001). Reconstructing the rise of recent coastal anoxia; molybdenum in Chesapeake Bay sediments. Geochim. Cosmochim. Acta 65, 237–252. doi: 10.1016/S0016-7037(00)00539-1
Ambrose, W. G., Carroll, M. L., Greenacre, M., Thorrold, S. R., and McMahon, K. W. (2006). Variation in Serripes groenlandicus (Bivalvia) growth in a Norwegian high-Arctic fjord: evidence for local- and large-scale climatic forcing. Glob. Change Biol. 12, 1595–1607. doi: 10.1111/j.1365-2486.2006.01181.x
Ballesta-Artero, I., Witbaard, R., Carroll, M. L., and van der Meer, J. (2016) “Environmental factors driving the valve gape activity of the bivalve Arctica islandica in Northern Norway,” in 4th International Sclerochronology conference (ISC) (Portland, ME).
Barats, A., Amouroux, D., Chauvaud, L., Pécheyran, C., Lorrain, A., Thébault, J., et al. (2009). High frequency Barium profiles in shells of the Great Scallop Pecten maximus: a methodical long-term and multi-site survey in Western Europe. Biogeosciences 6, 157–170. doi: 10.5194/bg-6-157-2009
Barats, A., Amouroux, D., Pécheyran, C., Chauvaud, L., and Donard, O. F. X. (2008). High-frequency archives of manganese inputs to coastal waters (Bay of Seine, France) resolved by the LA−ICP−MS analysis of calcitic growth layers along scallop shells (Pecten maximus). Environ. Sci. Technol. 42, 86–92. doi: 10.1021/es0701210
Barats, A., Amouroux, D., Pécheyran, C., Chauvaud, L., Thébault, J., and Donard, O. F. X. (2010). Spring molybdenum enrichment in scallop shells: a potential tracer of diatom productivity in temperate coastal environments (Brittany, NW France). Biogeosciences 7, 233–245. doi: 10.5194/bg-7-233-2010
Barbin, V., Ramseyer, K., and Elfman, M. (2008). Biological record of added manganese in seawater: a new efficient tool to mark in vivo growth lines in the oyster species Crassostrea gigas. Int. J. Earth Sci. 97, 193–199. doi: 10.1007/s00531-006-0160-0
Bayne, B. L., and Newell, R. C. (1983). “Physiological energetics of marine molluscs,” in The Mollusca, Vol. 4, Physiology, Part 1, eds A. S. M. Saleuddin and K. M. Wilbur (New York, NY: Academic Press), 407–515.
Beesley, A., Lowe, D., Pascoe, C., and Widdicombe, S. (2008). Effects of CO2-induced seawater acidification on the health of Mytilus edulis. Clim. Res. 37, 215–225. doi: 10.3354/cr00765
Beirne, E. C., Wanamaker, A. D. Jr., and Feindel, S. C. (2012). Experimental validation of environmental controls on the δ13C of Arctica islandica (ocean quahog) shell carbonate. Geochim. Cosmochim. Acta 84, 395–409. doi: 10.1016/j.gca.2012.01.021
Beliaeff, B., and Burgeot, T. (2002). Integrated biomarker response: a useful tool for ecological risk assessment. Environ. Toxicol. Chem. 21, 1316–1322. doi: 10.1002/etc.5620210629
Berthou, P., Blanchard, M., Noël, P., and Vergnaud-Grazzini, C. (1986). Stable Isotope Analysis of Shells for Age Determination in Four Bivalve Species from the Normand-Breton Gulf (Western English Channel). Copenhagen: ICES.
Birchenough, S. N. R., Reiss, H., Degraer, S., Mieszkowska, N., Borja, Á., Buhl-Mortensen, L., et al. (2015). Climate change and marine benthos: a review of existing research and future directions in the North Atlantic. Wiley Interdiscip. Rev. Clim. Change 6, 203–223. doi: 10.1002/wcc.330
Boehlert, G. W., and Gill, A. B. (2010). Environmental and ecological effects of ocean renewable energy development: a current synthesis. Oceanography 23, 68–81. doi: 10.5670/oceanog.2010.46
Boothe, P. N., and Presley, B. J. (1989). “Trends in sediment trace element concentrations around six petroleum drilling platforms in the northwestern Gulf of Mexico,” in Proceedings of the 1988 International Conference on Drilling Wastes, eds F. R. Engelhardt, J. P. Ray, A. H. Gillam (Calgary, AB; New York, NY: Elsevier Applied Science), 3–21.
Borja, Á., Franco, J., Valencia, V., Bald, J., Muxika, I., Jesús Belzunce, M., et al. (2004). Implementation of the European water framework directive from the Basque country (northern Spain): a methodological approach. Mar. Pollut. Bull. 48, 209–218. doi: 10.1016/j.marpolbul.2003.12.001
Bougeois, L., de Rafélis, M., Reichart, G.-J., de Nooijer, L. J., Nicollin, F., and Dupont-Nivet, G. (2014). A high resolution study of trace elements and stable isotopes in oyster shells to estimate Central Asian Middle Eocene seasonality. Chem. Geol. 363, 200–212. doi: 10.1016/j.chemgeo.2013.10.037
Bourgoin, B. (1990). Mytilus edulis shell as a bioindicator of lead pollution: considerations on bioavailability and variability. Mar. Ecol. Prog. Ser. 61, 253–262. doi: 10.3354/meps061253
Bowen, R. E., and Depledge, M. H. (2006). Rapid Assessment of Marine Pollution (RAMP). Mar. Pollut. Bull. 53, 631–639. doi: 10.1016/j.marpolbul.2006.09.002
Breitenlechner, E., Hilber, M., Lutz, J., Kathrein, Y., Unterkircher, A., and Oeggl, K. (2010). The impact of mining activities on the environment reflected by pollen, charcoal and geochemical analyses. J. Archaeol. Sci. 37, 1458–1467. doi: 10.1016/j.jas.2010.01.006
Breuer, E., Shimmield, G., and Peppe, O. (2008). Assessment of metal concentrations found within a North Sea drill cuttings pile. Mar. Pollut. Bull. 56, 1310–1322. doi: 10.1016/j.marpolbul.2008.04.010
Brocas, W. M., Reynolds, D. J., Butler, P. G., Richardson, C. A., Scourse, J. D., Ridgway, I. D., et al. (2013). The dog cockle, Glycymeris glycymeris (L.), a new annually-resolved sclerochronological archive for the Irish Sea. Palaeogeogr. Palaeoclimatol. Palaeoecol. 373, 133–140. doi: 10.1016/j.palaeo.2012.03.030
Broeg, K., Westernhagen, H. V., Zander, S., Körting, W., and Koehler, A. (2005). The “bioeffect assessment index” (BAI): a concept for the quantification of effects of marine pollution by an integrated biomarker approach. Mar. Pollut. Bull. 50, 495–503. doi: 10.1016/j.marpolbul.2005.02.042
Brown, M. T., and Depledge, M. H. (1998). “Determinants of trace metal concentrations in marine organisms,” in Metal Metabolism in Aquatic Environments, eds W. J. Langston and M. J. Bebianno (Springer US), 185–217. Available online at: http://link.springer.com/chapter/10.1007/978-1-4757-2761-6_7 (Accessed April 25, 2016).
Buddemeier, R. W., Maragos, J. E., and Knutson, D. W. (1974). Radiographic studies of reef coral exoskeletons: rates and patterns of coral growth. J. Exp. Mar. Biol. Ecol. 14, 179–199. doi: 10.1016/0022-0981(74)90024-0
Butler, P. G., Richardson, C. A., Scourse, J. D., Wanamaker, A. D. Jr., Shammon, T. M., and Bennell, J. D. (2010). Marine climate in the Irish Sea: analysis of a 489-year marine master chronology derived from growth increments in the shell of the clam Arctica islandica. Quat. Sci. Rev. 29, 1614–1632. doi: 10.1016/j.quascirev.2009.07.010
Butler, P. G., Wanamaker, A. D. Jr., Scourse, J. D., Richardson, C. A., and Reynolds, D. J. (2011). Long-term stability of δ13C with respect to biological age in the aragonite shell of mature specimens of the bivalve mollusk Arctica islandica. Palaeogeogr. Palaeoclimatol. Palaeoecol. 302, 21–30. doi: 10.1016/j.palaeo.2010.03.038
Butler, P. G., Wanamaker, A. D. Jr., Scourse, J. D., Richardson, C. A., and Reynolds, D. J. (2013). Variability of marine climate on the North Icelandic Shelf in a 1357-year proxy archive based on growth increments in the bivalve Arctica islandica. Palaeogeogr. Palaeoclimatol. Palaeoecol. 373, 141–151. doi: 10.1016/j.palaeo.2012.01.016
Campana, S. E., Chouinard, G. A., Hanson, J. M., Fréchet, A., and Brattey, J. (2000). Otolith elemental fingerprints as biological tracers of fish stocks. Fish. Res. 46, 343–357. doi: 10.1016/S0165-7836(00)00158-2
Carmichael, R. H., Annett, B., and Valiela, I. (2004). Nitrogen loading to Pleasant Bay, Cape Cod: application of models and stable isotopes to detect incipient nutrient enrichment of estuaries. Mar. Pollut. Bull. 48, 137–143. doi: 10.1016/S0025-326X(03)00372-2
Carmichael, R., Shriver, A. C., and Valiela, I. (2004). Changes in shell and soft tissue growth, tissue composition, and survival of quahogs, Mercenaria mercenaria, and softshell clams, Mya arenaria, in response to eutrophic-driven changes in food supply and habitat. J. Exp. Mar. Biol. Ecol. 313, 75–104. doi: 10.1016/j.jembe.2004.08.006
Carré, M., Bentaleb, I., Bruguier, O., Ordinola, E., Barrett, N. T., and Fontugne, M. (2006). Calcification rate influence on trace element concentrations in aragonitic bivalve shells: evidences and mechanisms. Geochim. Cosmochim. Acta 70, 4906–4920. doi: 10.1016/j.gca.2006.07.019
Carroll, M. L., Ambrose, W. G. Jr., Levin, B. S., Locke, W. L., Henkes, G. A., Hop, H., et al. (2011). Pan-Svalbard growth rate variability and environmental regulation in the Arctic bivalve Serripes groenlandicus. J. Mar. Syst. 88, 239–251. doi: 10.1016/j.jmarsys.2011.04.010
Carroll, M. L., Ambrose, W. G. Jr., Locke, W. L., Ryan, S. K., and Johnson, B. J. (2014). Bivalve growth rate and isotopic variability across the Barents Sea Polar Front. J. Mar. Syst. 130, 167–180. doi: 10.1016/j.jmarsys.2013.10.006
Carroll, M. L., Johnson, B. J., Henkes, G. A., McMahon, K. W., Voronkov, A., Ambrose, W. G. Jr., et al. (2009). Bivalves as indicators of environmental variation and potential anthropogenic impacts in the southern Barents Sea. Mar. Pollut. Bull. 59, 193–206. doi: 10.1016/j.marpolbul.2009.02.022
Carroll, M., and Romanek, C. S. (2008). Shell layer variation in trace element concentration for the freshwater bivalve Elliptio complanata. Geo Mar. Lett. 28, 369–381. doi: 10.1007/s00367-008-0117-3
Carroll, M., Dragsund, E., Pearson, T., Gabrielsen, K. L., Akvaplan-niva, T., Unilab Analyse, T., et al. (2001). Environmental Status of the Norwegian Offshore Sector Based on the Petroleum Regional Monitoring Programme, 1996–1998. Tromsø: Norwegian Oil Industry Association.
Carter, C. J., Wilson, B., and Black, K. (2008). Marine Renewable Energy Devices: A Collision Risk for Marine Mammals? OFFSHORE WIND FARMS. Mar. Mamm. IMPACTS Methodol. Assess. IMPACTS, 60.
CEFAS (2004). Cefas - Homepage. Available online at: https://www.cefas.co.uk/monitoring (Accessed November 17, 2015).
Chaillou, G., Anschutz, P., Lavaux, G., Schäfer, J., and Blanc, G. (2002). The distribution of Mo, U, and Cd in relation to major redox species in muddy sediments of the Bay of Biscay. Mar. Chem. 80, 41–59. doi: 10.1016/S0304-4203(02)00097-X
Chow, T. J., George Snyder, H., and Snyder, C. B. (1976). Mussels (Mytilus sp.) as an indicator of lead pollution. Sci. Total Environ. 6, 55–63. doi: 10.1016/0048-9697(76)90006-1
Claisse, J. T., Pondella, D. J., Love, M., Zahn, L. A., Williams, C. M., Williams, J. P., et al. (2014). Oil platforms off California are among the most productive marine fish habitats globally. Proc. Natl. Acad. Sci. U.S.A. 111, 15462–15467. doi: 10.1073/pnas.1411477111
CMSP NOAA (2015). Coastal and Marine Spatial Planning. Coatal Mar. Spat. Plan. Available online at: http://cmsp.noaa.gov/ (Accessed November 19, 2015).
Collier, R. W. (1985). Molybdenum in the Northeast Pacific Ocean. Limnol Ocean. 30, 1352–1354. doi: 10.4319/lo.1985.30.6.1351
Crusius, J., Calvert, S., Pedersen, T., and Sage, D. (1996). Rhenium and molybdenum enrichments in sediments as indicators of oxic, suboxic and sulfidic conditions of deposition. Earth Planet. Sci. Lett. 145, 65–78. doi: 10.1016/S0012-821X(96)00204-X
Dagnino, A., Allen, J. I., Moore, M. N., Broeg, K., Canesi, L., and Viarengo, A. (2007). Development of an expert system for the integration of biomarker responses in mussels into an animal health index. Biomarkers 12, 155–172. doi: 10.1080/13547500601037171
Dalai, T. K., Nishimura, K., and Nozaki, Y. (2005). Geochemistry of molybdenum in the Chao Phraya River estuary, Thailand: role of suboxic diagenesis and porewater transport. Chem. Geol. 218, 189–202. doi: 10.1016/j.chemgeo.2005.01.002
Dauwe, B., Herman, P. M. J., and Heip, C. H. R. (1998). Community structure and bioturbation potential of macrofauna at four North Sea stations with contrasting food supply. Mar. Ecol. Prog. Ser. 173, 67–83. doi: 10.3354/meps173067
Davenport, C. B. (1938). Growth lines in fossil pectens as indicators of past climates. J. Paleontol. 12, 514–515.
de Zwart, D., Kramer, K. J. M., and Jenner, H. A. (1995). Practical experiences with the biological early warning system “mosselmonitor.” Environ. Toxicol. Water Qual. 10, 237–247. doi: 10.1002/tox.2530100403
Delaney, M. L., Bé, W. H., and Boyle, E. A. (1985). Li, Sr, Mg, and Na in foraminiferal calcite shells from laboratory culture, sediment traps, and sediment cores. Geochim. Cosmochim. Acta 49, 1327–1341. doi: 10.1016/0016-7037(85)90284-4
Dellwig, O., Beck, M., Lemke, A., Lunau, M., Kolditz, K., Schnetger, B., et al. (2007). Non-conservative behaviour of molybdenum in coastal waters: coupling geochemical, biological, and sedimentological processes. Geochim. Cosmochim. Acta 71, 2745–2761. doi: 10.1016/j.gca.2007.03.014
Delong, M. D., and Thorp, J. H. (2006). Significance of instream autotrophs in trophic dynamics of the Upper Mississippi River. Oecologia 147, 76–85. doi: 10.1007/s00442-005-0241-y
DeNiro, M. J., and Epstein, S. (1978). Influence of diet on the distribution of carbon isotopes in animals. Geochim. Cosmochim. Acta 42, 495–506. doi: 10.1016/0016-7037(78)90199-0
Depledge, M. H. (1994). Genotypic toxicity: implications for individuals and populations. Environ. Health Perspect. 102, 101–104. doi: 10.1289/ehp.94102s12101
Diaz, R. J., and Rosenberg, R. (2008). Spreading dead zones and consequences for marine ecosystems. Science 321, 926–929. doi: 10.1126/science.1156401
Dickinson, G. H., Ivanina, A. V., Matoo, O. B., Pörtner, H. O., Lannig, G., Bock, C., et al. (2012). Interactive effects of salinity and elevated CO2 levels on juvenile eastern oysters, Crassostrea virginica. J. Exp. Biol. 215, 29–43. doi: 10.1242/jeb.061481
Douvere, F., and Ehler, C. N. (2009). New perspectives on sea use management: initial findings from European experience with marine spatial planning. J. Environ. Manage. 90, 77–88. doi: 10.1016/j.jenvman.2008.07.004
Drinkwater, K. F. (2006). The regime shift of the 1920s and 1930s in the North Atlantic. Prog. Oceanogr. 68, 134–151. doi: 10.1016/j.pocean.2006.02.011
Dunca, E., Mutvei, H., Göransson, P., Mörth, C.-M., Schöne, B. R., Whitehouse, M. J., et al. (2008). Using ocean quahog (Arctica islandica) shells to reconstruct palaeoenvironment in Öresund, Kattegat and Skagerrak, Sweden. Int. J. Earth Sci. 98, 3–17. doi: 10.1007/s00531-008-0348-6
EC (2015). Good Environmental Status - Marine - Environment - European Commission. Available at: http://ec.europa.eu/environment/marine/good-environmental-status/index_en.htm (Accessed November 18, 2015).
EC MSFD (2010). Commission Decision of 1 September 2010 on Criteria and Methodological Standards on Good Environmental Status of Marine Waters (Notified under Document C(2010) 5956). Available online at: http://eur-lex.europa.eu/legal-content/EN/TXT/PDF/?uri=CELEX:32010D0477(01)&from=EN (Accessed November 19, 2015).
Edge, K. J., Dafforn, K. A., Simpson, S. L., Roach, A. C., and Johnston, E. L. (2014). A biomarker of contaminant exposure is effective in large scale assessment of ten estuaries. Chemosphere 100, 16–26. doi: 10.1016/j.chemosphere.2014.01.001
Elbaz-Poulichet, F., Seidel, J. L., Jézéquel, D., Metzger, E., Prévot, F., Simonucci, C., et al. (2005). Sedimentary record of redox-sensitive elements (U, Mn, Mo) in a transitory anoxic basin (the Thau lagoon, France). Mar. Chem. 95, 271–281. doi: 10.1016/j.marchem.2004.10.001
Elhadj, S., De Yoreo, J. J., Hoyer, J. R., and Dove, P. M. (2006). Role of molecular charge and hydrophilicity in regulating the kinetics of crystal growth. Proc. Natl. Acad. Sci. U.S.A. 103, 19237–19242. doi: 10.1073/pnas.0605748103
Ellis, G. S., Herbert, G., and Hollander, D. (2014). Reconstructing carbon sources in a dynamic estuarine ecosystem using oyster amino Acid δ13C Values from shell and tissue. J. Shellfish Res. 33, 217–225. doi: 10.2983/035.033.0121
Ellis, R. P., Bersey, J., Rundle, S. D., HallSpencer, J. M., and Spicer, J. I. (2009). Subtle but significant effects of CO2 acidified seawater on embryos of the intertidal snail, Littorina obtusata. Aquat. Biol. 5, 41–48. doi: 10.3354/ab00118
Erlenkeuser, H. (1976). 14C and 13C Isotope concentration in modern marine mussels from sedimentary habitats. Naturwissenschaften 63, 338–338. doi: 10.1007/BF00597312
ETNWE (2003). WaveNet - Results from the work of European Thematic Network on Wave Energy. Available online at: http://www.offshorecenter.dk/log/bibliotek/23WaveNet_Full_Report.pdf (Accessed November 19, 2015).
European Parliament (2007). An Energy policy for Europe. Available online at: http://eur-lex.europa.eu/legal-content/EN/TXT/?uri=URISERV:l27067 (Accessed November 19, 2015).
Farquhar, G. D., Ehleringer, J. R., and Hubick, K. T. (1989). Carbon isotope discrimination and photosynthesis. Annu. Rev. Plant Physiol. Plant Mol. Biol. 40, 503–537. doi: 10.1146/annurev.pp.40.060189.002443
Faubel, D., Lopes-Lima, M., Freitas, S., Pereira, L., Andrade, J., Checa, A., et al. (2008). Effects of Cd2+ on the calcium metabolism and shell mineralization of bivalve Anodonta cygnea. Mar. Freshw. Behav. Physiol. 41, 131–146. doi: 10.1080/10236240802194123
Fdil, M. A., Mouabad, A., Outzourhit, A., Benhra, A., Maarouf, A., and Pihan, J. C. (2006). Valve movement response of the mussel Mytilus galloprovincialis to metals (Cu, Hg, Cd and Zn) and phosphate industry effluents from Moroccan Atlantic coast. Ecotoxicology 15, 477–486. doi: 10.1007/s10646-006-0083-3
Findlater, G., Shelton, A., Rolin, T., and Andrews, J. (2014). Sodium and strontium in mollusc shells: preservation, palaeosalinity and palaeotemperature of the Middle Pleistocene of eastern England. Proc. Geol. Assoc. 125, 14–19. doi: 10.1016/j.pgeola.2013.10.005
Fitzer, S. C., Chung, P., Maccherozzi, F., Dhesi, S. S., Kamenos, N. A., Phoenix, V. R., et al. (2016). Biomineral shell formation under ocean acidification: a shift from order to chaos. Sci. Rep. 6:21076. doi: 10.1038/srep21076
Fitzer, S. C., Cusack, M., Phoenix, V. R., and Kamenos, N. A. (2014a). Ocean acidification reduces the crystallographic control in juvenile mussel shells. J. Struct. Biol. 188, 39–45. doi: 10.1016/j.jsb.2014.08.007
Fitzer, S. C., Phoenix, V. R., Cusack, M., and Kamenos, N. A. (2014b). Ocean acidification impacts mussel control on biomineralisation. Sci. Rep. 4:6218. doi: 10.1038/srep06218
Fitzer, S. C., Zhu, W., Tanner, K. E., Phoenix, V. R., Kamenos, N. A., and Cusack, M. (2015). Ocean acidification alters the material properties of Mytilus edulis shells. J. R. Soc. Interface 12:20141227. doi: 10.1098/rsif.2014.1227
Foster, L. C., Allison, N., Finch, A. A., and Andersson, C. (2009). Strontium distribution in the shell of the aragonite bivalve Arctica islandica. Geochem. Geophys. Geosystems 10:Q03003. doi: 10.1029/2007GC001915
Foster, L. C., Finch, A. A., Allison, N., Andersson, C., and Clarke, L. J. (2008). Mg in aragonitic bivalve shells: seasonal variations and mode of incorporation in Arctica islandica. Chem. Geol. 254, 113–119. doi: 10.1016/j.chemgeo.2008.06.007
Fowler, A. M., Macreadie, P. I., Bishop, D. P., and Booth, D. J. (2015). Using otolith microchemistry and shape to assess the habitat value of oil structures for reef fish. Mar. Environ. Res. 106, 103–113. doi: 10.1016/j.marenvres.2015.03.007
Fowler, A. M., Macreadie, P. I., Jones, D. O. B., and Booth, D. J. (2014). A multi-criteria decision approach to decommissioning of offshore oil and gas infrastructure. Ocean Coast. Manag. 87, 20–29. doi: 10.1016/j.ocecoaman.2013.10.019
Franco, J., Borja, Á., Solaun, O., and Pérez, V. (2002). Heavy metals in molluscs from the Basque Coast (Northern Spain): results from an 11-year monitoring programme. Mar. Pollut. Bull. 44, 973–976. doi: 10.1016/S0025-326X(02)00122-4
Freitas, P. S., Clarke, L. J., Kennedy, H., Richardson, C. A., and Abrantes, F. (2006). Environmental and biological controls on elemental (Mg/Ca, Sr/Ca and Mn/Ca) ratios in shells of the king scallop Pecten maximus. Geochim. Cosmochim. Acta 70, 5119–5133. doi: 10.1016/j.gca.2006.07.029
Fuge, R., Hitchon, B., Palmer, T. J., Pearce, N. J. G., William, T., and Perkins (1993). Environmental Geochemistry minor and trace element chemistry of modern shells: a laser ablation inductively coupled plasma mass spectrometry study. Appl. Geochem. 8, 111–116. doi: 10.1016/S0883-2927(09)80020-6
Gaetani, G. A., and Cohen, A. L. (2006). Element partitioning during precipitation of aragonite from seawater: a framework for understanding paleoproxies. Geochim. Cosmochim. Acta 70, 4617–4634. doi: 10.1016/j.gca.2006.07.008
Gazeau, F., Parker, L. M., Comeau, S., Gattuso, J.-P., O'Connor, W. A., Martin, S., et al. (2013). Impacts of ocean acidification on marine shelled molluscs. Mar. Biol. 160, 2207–2245. doi: 10.1007/s00227-013-2219-3
Gazeau, F., Quiblier, C., Jansen, J. M., Gattuso, J.-P., Middelburg, J. J., and Heip, C. H. R. (2007). Impact of elevated CO2 on shellfish calcification. Geophys. Res. Lett. 34:L07603. doi: 10.1029/2006GL028554
GESAMP (1999). Guidelines for Marine Protected Areas. Best Pract. Prot. Area Guidel. Ser. London: IUCN.
Ghosh, P., Adkins, J., Affek, H., Balta, B., Guo, W., Schauble, E. A., et al. (2006). 13C–18O bonds in carbonate minerals: a new kind of paleothermometer. Geochim. Cosmochim. Acta 70, 1439–1456. doi: 10.1016/j.gca.2005.11.014
Gilkinson, K., Paulin, M., Hurley, S., and Schwinghamer, P. (1998). Impacts of trawl door scouring on infaunal bivalves: results of a physical trawl door model/dense sand interaction. J. Exp. Mar. Biol. Ecol. 224, 291–312. doi: 10.1016/S0022-0981(97)00207-4
Gill, A. B. (2005). Offshore renewable energy: ecological implications of generating electricity in the coastal zone. J. Appl. Ecol. 42, 605–615. doi: 10.1111/j.1365-2664.2005.01060.x
Gillanders, B. M., and Kingsford, M. J. (2000). Elemental fingerprints of otoliths of fish may distinguish estuarine‘nursery’habitats. Mar. Ecol. Prog. Ser. 201, 273–286. doi: 10.3354/meps201273
Gillikin, D. P., and Dehairs, F. (2013). Uranium in aragonitic marine bivalve shells. Palaeogeogr. Palaeoclimatol. Palaeoecol. 373, 60–65. doi: 10.1016/j.palaeo.2012.02.028
Gillikin, D. P., De Ridder, F., Ulens, H., Elskens, M., Keppens, E., Baeyens, W., et al. (2005a). Assessing the reproducibility and reliability of estuarine bivalve shells (Saxidomus giganteus) for sea surface temperature reconstruction: implications for paleoclimate studies. Palaeogeogr. Palaeoclimatol. Palaeoecol. 228, 70–85. doi: 10.1016/j.palaeo.2005.03.047
Gillikin, D. P., Dehairs, F., Baeyens, W., Navez, J., Lorrain, A., and André, L. (2005b). Inter- and intra-annual variations of Pb/Ca ratios in clam shells (Mercenaria mercenaria): a record of anthropogenic lead pollution? Mar. Pollut. Bull. 50, 1530–1540. doi: 10.1016/j.marpolbul.2005.06.020
Gillikin, D. P., Dehairs, F., Lorrain, A., Steenmans, D., Baeyens, W., and André, L. (2006). Barium uptake into the shells of the common mussel (Mytilus edulis) and the potential for estuarine paleo-chemistry reconstruction. Geochim. Cosmochim. Acta 70, 395–407. doi: 10.1016/j.gca.2005.09.015
Gillikin, D. P., Lorrain, A., Paulet, Y.-M., André, L., and Dehairs, F. (2008). Synchronous barium peaks in high-resolution profiles of calcite and aragonite marine bivalve shells. Geo Mar. Lett. 28, 351–358. doi: 10.1007/s00367-008-0111-9
Goldberg, E. D., and Bertine, K. K. (2000). Beyond the mussel watch — new directions for monitoring marine pollution. Sci. Total Environ. 247, 165–174. doi: 10.1016/S0048-9697(99)00488-X
Goldberg, E. D., Bowen, V. T., Farrington, J. W., Harvey, G., Martin, J. H., Parker, P. L., et al. (1978). The mussel watch. Environ. Conserv. 5, 101–125. doi: 10.1017/S0376892900005555
Goodwin, D. H., Schöne, B. R., and Dettman, D. L. (2003). Resolution and fidelity of oxygen isotopes as paleotemperature proxies in bivalve mollusk shells: models and observations. Palaios 18, 110–125. doi: 10.1669/0883-1351(2003)18<110:RAFOOI>2.0.CO;2
Gordon, C. M., Carr, R. A., and Larson, R. E. (1970). The influence of environmental factors on the sodium and manganese content of barnacle shells. Limnol. Oceanogr. 15, 461–466. doi: 10.4319/lo.1970.15.3.0461
Grossman, E. L., and Ku, T.-L. (1986). Oxygen and carbon isotope fractionation in biogenic aragonite: temperature effects. Chem. Geol. Isot. Geosci. Sect. 59, 59–74. doi: 10.1016/0168-9622(86)90057-6
Hartley, J. P. (1996). Environmental monitoring of offshore oil and gas drilling discharges—A caution on the use of barium as a tracer. Mar. Pollut. Bull. 32, 727–733. doi: 10.1016/0025-326X(96)00033-1
Henkes, G. A., Passey, B. H., Wanamaker, A. D. Jr., Grossman, E. L., Ambrose, W. G. Jr., and Carroll, M. L. (2013). Carbonate clumped isotope compositions of modern marine mollusk and brachiopod shells. Geochim. Cosmochim. Acta 106, 307–325. doi: 10.1016/j.gca.2012.12.020
Hippler, D., Witbaard, R., van Aken, H. M., Buhl, D., and Immenhauser, A. (2013). Exploring the calcium isotope signature of Arctica islandica as an environmental proxy using laboratory-and field-cultured specimens. Palaeogeogr. Palaeoclimatol. Palaeoecol. 373, 75–87. doi: 10.1016/j.palaeo.2011.11.015
Holland, H. A., Schöne, B. R., Marali, S., and Jochum, K. P. (2014). History of bioavailable lead and iron in the Greater North Sea and Iceland during the last millennium – A bivalve sclerochronological reconstruction. Mar. Pollut. Bull. 87, 104–116. doi: 10.1016/j.marpolbul.2014.08.005
Hooke, R. L. (2000). On the history of humans as geomorphic agents. Geology 28, 843–846. doi: 10.1130/0091-7613(2000)28<843:OTHOHA>2.0.CO;2
Howland, M. R., Corr, L. T., Young, S. M. M., Jones, V., Jim, S., Van Der Merwe, N. J., et al. (2003). Expression of the dietary isotope signal in the compound-specific δ13C values of pig bone lipids and amino acids. Int. J. Osteoarchaeol. 13, 54–65. doi: 10.1002/oa.658
Huebner, J. D., and Pynnönen, K. S. (1992). Viability of glochidia of two species of Anodonta exposed to low pH and selected metals. Can. J. Zool. 70, 2348–2355. doi: 10.1139/z92-315
Huerta-Diaz, M. A., Tessier, A., and Carignan, R. (1998). Geochemistry of trace metals associated with reduced sulfur in freshwater sediments. Appl. Geochem. 13, 213–233. doi: 10.1016/S.0883-2927(97)00060-7
ICES (2007). Report of the Working Group on Biolgocial Effects of Contaminants (WGBEC). Allessandria. Available online at: http://www.ices.dk/sites/pub/CM%20Doccuments/CM-2007/MHC/wgbec07.pdf (Accessed November 16, 2015).
ICES (2008). Report of the Benthos Ecology Working Group (BEWG). Torregrande, Sardinia. Available online at: http://www.ices.dk/sites/pub/Publication%20Reports/Expert%20Group%20Report/mhc/2008/bewg08.pdf (Accessed November, 16, 2015).
IPCC, Pachauri, R. K., Allen, M., Barros, V., Broome, J., Cramer, W., et al. (2014). Climate Change 2014: Synthesis Report. Contribution of Working Groups I, II and III to the Fifth Assessment Report of the Intergovernmental Panel on Climate Change/ R. Pachauri and L. Meyer (editors). Geneva, Switzerland, IPCC. 151.
Ishikawa, M., and Ichikuni, M. (1984). Uptake of sodium and potassium by calcite. Chem. Geol. 42, 137–146. doi: 10.1016/0009-2541(84)90010-X
Ivanina, A. V., Dickinson, G. H., Matoo, O. B., Bagwe, R., Dickinson, A., Beniash, E., et al. (2013). Interactive effects of elevated temperature and CO2 levels on energy metabolism and biomineralization of marine bivalves Crassostrea virginica and Mercenaria mercenaria. Comp. Biochem. Physiol. Part A Mol. Integr. Physiol. 166, 101–111. doi: 10.1016/j.cbpa.2013.05.016
JAMP (1998a). JAMP Guidelines for Contaminant-Specific Biological Effects Monitoring. Oslo: Oslo and Paris Commissions.
JAMP (1998b). JAMP Guidelines for General Biological Effects Monitoring. Oslo: Oslo and Paris Commissions.
Jones, D. S. (1980). Annual cycle of shell growth increment formation in two continental shelf bivalves and its paleoecologic significance. Paleobiology 6, 331–340. doi: 10.1017/S0094837300006837
Jørgensen, C. (1988). The bivalve pump: properties and modelling. Mar. Ecol. Prog. Ser. 45, 205–216. doi: 10.3354/meps045205
Kaufman, D. S., Schneider, D. P., McKay, N. P., Ammann, C. M., Bradley, R. S., Briffa, K. R., et al. (2009). Recent warming reverses long-term arctic cooling. Science 325, 1236–1239. doi: 10.1126/science.1173983
Kitano, Y., Okumura, M., and Idogaki, M. (1975). Incorporation of sodium, chloride and sulfate with calcium carbonate. Geochem. J. 9, 75–84. doi: 10.2343/geochemj.9.75
Klünder, M. H., Hippler, D., Witbaard, R., and Frei, D. (2008). Laser ablation analysis of bivalve shells–archives of environmental information. Geol. Surv. Den. Greenl. Bull. 15, 89–92.
Koide, M., Lee, D. S., and Goldberg, E. D. (1982). Metal and transuranic records in mussel shells, byssal threads and tissues. Estuar. Coast. Shelf Sci. 15, 679–695. doi: 10.1016/0272-7714(82)90079-8
Kramer, K. J. M., Jenner, H. A., and de Zwart, D. (1989). “The valve movement response of mussels: a tool in biological monitoring,” in Environmental Bioassay Techniques and their Application Developments in Hydrobiology, eds M. Munawar, G. Dixon, C. I. Mayfield, T. Reynoldson, and M. H. Sadar (Springer Netherlands), 433–443. Available online at: http://link.springer.com/chapter/10.1007/978-94-009-1896-2_44 (Accessed November 24, 2015).
Krause-Nehring, J., Brey, T., and Thorrold, S. R. (2012). Centennial records of lead contamination in northern Atlantic bivalves (Arctica islandica). Mar. Pollut. Bull. 64, 233–240. doi: 10.1016/j.marpolbul.2011.11.028
Kurihara, H. (2008). Contribution to the theme section “effects of ocean acidification on marine ecosystems” effects of CO2-driven ocean acidification on the early developmental stages of invertebrates. Mar. Ecol. Prog. Ser. 373, 275–284. doi: 10.3354/meps07802
Kurihara, H., Kato, S., and Ishimatsu, A. (2007). Effects of increased seawater pCO2 on early development of the oyster Crassostrea gigas. Aquat. Biol. 1, 91–98. doi: 10.3354/ab00009
Lam, P. K. S., and Gray, J. S. (2003). The use of biomarkers in environmental monitoring programmes. Mar. Pollut. Bull. 46, 182–186. doi: 10.1016/S0025-326X(02)00449-6
Langlet, D., Alunno-Bruscia, M., de Rafélis, M., Renard, M., Roux, M., Schein, E., et al. (2006). Experimental and natural manganese-induced cathodoluminescence in the shell of the Japanese oyster Crassostrea gigas (Thunberg, 1793) from Thau Lagoon (Hérault, France): ecological and environmental implications. Mar. Ecol. Prog. Ser. 317, 143–156. doi: 10.3354/meps317143
Larsen, M. M., Strand, J., Christensen, J. H., Vorkamp, K., Hansen, A. B., and Andersen, O. (2011). Metals and organotins in multiple bivalve species in a one-off global survey. J. Environ. Monit. 13, 1793–1802. doi: 10.1039/c0em00698j
Lartaud, F., de Rafelis, M., Ropert, M., Emmanuel, L., Geairon, P., and Renard, M. (2010). Mn labelling of living oysters: artificial and natural cathodoluminescence analyses as a tool for age and growth rate determination of C. gigas (Thunberg, 1793) shells. Aquaculture 300, 206–217. doi: 10.1016/j.aquaculture.2009.12.018
Lazareth, C. E., Lasne, G., and Ortlieb, L. (2006). Growth anomalies in Protothaca thaca (Mollusca, Veneridae) shells as markers of ENSO conditions. Clim. Res. 30, 263. doi: 10.3354/cr030263
Lazareth, C. E., Putten, E. V., André, L., and Dehairs, F. (2003). High-resolution trace element profiles in shells of the mangrove bivalve Isognomon ephippium: a record of environmental spatio-temporal variations? Estuar. Coast. Shelf Sci. 57, 1103–1114. doi: 10.1016/S0272-7714(03)00013-1
Lea, D. W., Mashiotta, T. A., and Spero, H. J. (1999). Controls on magnesium and strontium uptake in planktonic foraminifera determined by live culturing. Geochim. Cosmochim. Acta 63, 2369–2379. doi: 10.1016/S0016-7037(99)00197-0
Lee, K., Neff, J. M., and DeBlois, E. (2011). “Chapter 1 - produced water: overview of composition, fate, and effects,” in Produced Water: Environmental Risks and Advances in Mitigation Technologies, eds K. Lee and J. Neff (New York, NY: Springer Science & Business Media), 3–54.
Levin, L. A. (2006). Recent progress in understanding larval dispersal: new directions and digressions. Integr. Comp. Biol. 46, 282–297. doi: 10.1093/icb/icj024
Levin, L., Ekau, W., Gooday, A., Jorissen, F., Middelburg, J., Naqvi, S., et al. (2009). Effects of natural and human-induced hypoxia on coastal benthos. Biogeosciences 6, 2063–2098. doi: 10.5194/bg-6-2063-2009
Liehr, G. A., Zettler, M. L., Leipe, T., and Witt, G. (2005). The ocean quahog Arctica islandica L.: a bioindicator for contaminated sediments. Mar. Biol. 147, 671–679. doi: 10.1007/s00227-005-1612-y
Lindeboom, H., Degraer, S., Dannheim, J., Gill, A. B., and Wilhelmsson, D. (2015). Offshore wind park monitoring programmes, lessons learned and recommendations for the future. Hydrobiologia 756, 169–180. doi: 10.1007/s10750-015-2267-4
Lingard, S. M., Evans, R. D., and Bourgoin, B. P. (1992). Method for the estimation of organic-bound and crystal-bound metal concentrations in bivalve shells. Bull. Environ. Contam. Toxicol. 48, 179–184. doi: 10.1007/BF00194369
Linnaeus, C. (1758). Systema Naturae per Regna Tria Naturae, Secundum Classes, Ordines, Genera, Species, Cum Characteribus, Differentiis, Synonymis, Locis, Editio decima, Reformata. Holmiae: Laurentius Salvius.
Linnaeus C. (1767). Caroli Linnaei…Systema Naturae per Regna Tria Naturae: Secundum Classes, Ordines, Genera, Species, Cum Characteribus, Differentiis, Synonymis, Locis, Editio duodecima. 1. Regnum Animale. 1 & 2 Holmiae, Laurentii Salvii. Holmiae [Stockholm]: Laurentii Salvii, 1–532 [1766], 533–1327 [1767].
Lopes-Lima, M., Freitas, S., Pereira, L., Gouveia, E., Hinzmann, M., Checa, A., et al. (2012). Ionic regulation and shell mineralization in the bivalve Anodonta cygnea (swan mussel) following heavy-metal exposure. Can. J. Zool. 90, 267–283. doi: 10.1139/z11-129
Lorrain, A., Paulet, Y.-M., Chauvaud, L., Dunbar, R., Mucciarone, D., and Fontugne, M. (2004). δ13C variation in scallop shells: increasing metabolic carbon contribution with body size?1. Geochim. Cosmochim. Acta 68, 3509–3519. doi: 10.1016/j.gca.2004.01.025
Macreadie, P. I., Fowler, A. M., and Booth, D. J. (2011). Rigs-to-reefs: will the deep sea benefit from artificial habitat? Front. Ecol. Environ. 9:100112. doi: 10.1890/100112
Manley, A. R., and Davenport, J. (1979). Behavioural responses of some marine bivalves to heightened seawater copper concentrations. Bull. Environ. Contam. Toxicol. 22, 739–744. doi: 10.1007/BF02027017
Marali, S., Schöne, B. R., Mertz-Kraus, R., Griffin, S. M., Wanamaker A. D. Jr, Matras, U., et al. (2016). Ba/Ca ratios in shells of Arctica islandica - Potential environmental proxy and crossdating tool. Palaeogeogr. Palaeoclimatol. Palaeoecol. doi: 10.1016/j.palaeo.2015.12.018
Marchitto, T. M. Jr., Jones, G. A., Goodfriend, G. A., and Weidman, C. R. (2000). Precise Temporal Correlation of Holocene Mollusk Shells Using Sclerochronology. Quat. Res. 53, 236–246. doi: 10.1006/qres.1999.2107
Marin, F., Le Roy, N., and Marie, B. (2012). The formation and mineralization of mollusk shell. Front. Biosci. 4, 1099–1125. doi: 10.2741/s321
Marin, F., Luquet, G., Marie, B., and Medakovic, D. (2007). “Molluscan shell proteins: primary structure, origin, and evolution,” in Current Topics in Developmental Biology (Academic Press), 209–276. Available online at: http://www.sciencedirect.com/science/article/pii/S0070215307800068 (Accessed April 2, 2016).
Markich, S. J., and Jeffree, R. A. (1994). Absorption of divalent trace metals as analogues of calcium by Australian freshwater bivalves: an explanation of how water hardness reduces metal toxicity. Aquat. Toxicol. 29, 257–290. doi: 10.1016/0166-445X(94)90072-8
Markich, S. J., Brown, P. L., and Jeffree, R. A. (1996). The use of geochemical speciation modelling to predict the impact of uranium to freshwater biota. Radiochim. Acta 74, 321–326. doi: 10.1524/ract.1996.74.special-issue.321
Markich, S. J., Jeffree, R. A., and Burke, P. T. (2002). Freshwater bivalve shells as archival indicators of metal pollution from a copper−uranium mine in tropical Northern Australia. Environ. Sci. Technol. 36, 821–832. doi: 10.1021/es011066c
Marsh, R., Petrie, B., Weidman, C. R., Dickson, R. R., Loder, J. W., Hannah, C. G., et al. (1999). The 1882 tilefish kill — a cold event in shelf waters off the north-eastern United States? Fish. Oceanogr. 8, 39–49. doi: 10.1046/j.1365-2419.1999.00092.x
Matthias, U., and Römpp (1994). Erprobung des Dreissena-monitors, eines neuen biotestsystems mit der zebramuschel (Dreissena polymorpha), in der Rhein-Gütemeßstation karlsruhe evaluation of the Dreissena-monitor at the river rhine — a new biological monitoring system based on the zebra mussel Dreissena polymorpha. Acta Hydrochim. Hydrobiol. 22, 161–165. doi: 10.1002/aheh.19940220402
Matthiessen, P., and Law, R. J. (2002). Contaminants and their effects on estuarine and coastal organisms in the United Kingdom in the late twentieth century. Environ. Pollut. 120, 739–757. doi: 10.1016/S0269-7491(02)00175-6
McCormack, P., Jones, P., Hetheridge, M. J., and Rowland, S. J. (2001). Analysis of oilfield produced waters and production chemicals by electrospray ionisation multi-stage mass spectrometry (ESI-MSn). Water Res. 35, 3567–3578. doi: 10.1016/S0043-1354(01)00070-7
McLusky, D. S., Bryant, D. M., and Elliott, M. (1992). The impact of land-claim on macrobenthos, fish and shorebirds on the forth estuary, eastern Scotland. Aquat. Conserv. Mar. Freshw. Ecosyst. 2, 211–222. doi: 10.1002/aqc.3270020302
Mette, M. J., Wanamaker, A. D., Carroll, M. L., Ambrose, W. G., and Retelle, M. J. (2016). Linking large-scale climate variability with Arctica islandica shell growth and geochemistry in northern Norway. Limnol. Oceanogr. 61, 748–764. doi: 10.1002/lno.10252
Michaelidis, B., Ouzounis, C., Paleras, A., and Prtner, H. O. (2005). Effects of long-term moderate hypercapnia on acid–base balance and growth rate in marine mussels Mytilus galloprovincialis. Mar. Ecol. Prog. Ser. 293, 109–118. doi: 10.3354/meps293109
Milano, S., Schöne, B. R., and Witbaard, R. (2016). Changes of shell microstructural characteristics of Cerastoderma edule (Bivalvia) — A novel proxy for water temperature. Palaeogeogr. Palaeoclimatol. Palaeoecol. doi: 10.1016/j.palaeo.2015.09.051
Moore, M. N., Depledge, M. H., Readman, J. W., and Paul Leonard, D. R. (2004). An integrated biomarker-based strategy for ecotoxicological evaluation of risk in environmental management. Mutat. Res. Mol. Mech. Mutagen. 552, 247–268. doi: 10.1016/j.mrfmmm.2004.06.028
Morton, B. (2011). The biology and functional morphology of Arctica islandica (Bivalvia: Arcticidae) – A gerontophilic living fossil. Mar. Biol. Res. 7, 540–553. doi: 10.1080/17451000.2010.535833
Nagler, T. F., Hart, S. R., and Hippler, D. (2006). Seasonal Sr/Ca, and 44Ca/40Ca co-variation in Arctica islandica. Available online at: http://inis.iaea.org/Search/search.aspx?orig_q=RN:38111508 (Accessed November 17, 2015).
NCSCO (2004). North Carolina State Climate Office. Available online at: http://www.nc-climate.ncsu.edu/climate/hurricanes.html
Nedwell, J., and Howell, D. (2004). A review of offshore windfarm related underwater noise sources. Cowrie Rep. 544, 1–57.
Neff, J. M. (2002). Bioaccumulation in Marine Organisms: Effect of Contaminants from Oil Well Produced Water. Oxford: Elsevier.
Nuñez, J. D., Laitano, M. V., and Cledón, M. (2012). An intertidal limpet species as a bioindicator: pollution effects reflected by shell characteristics. Ecol. Indic. 14, 178–183. doi: 10.1016/j.ecolind.2011.07.015
O'Donnell, T. H., Macko, S. A., and Wehmiller, J. F. (2007). Stable carbon isotope composition of amino acids in modern and fossil Mercenaria. Org. Geochem. 38, 485–498. doi: 10.1016/j.orggeochem.2006.06.010
Oeschger, R. (1990). Long-term anaerobiosis in sublittoral marine-invertebrates from the Western Baltic Sea-Halicryptus spinulosus (Priapulida), Astarte borealis and Arctica islandica (Bivalvia). Mar. Ecol. Prog. Ser. 59, 133–143. doi: 10.3354/meps059133
OLF (2000). Physical, Chemical, and Biological Characterisation of Offshore Drill Cuttings Piles, Stavanger.
Orme, C. A., Noy, A., Wierzbicki, A., McBride, M. T., Grantham, M., Teng, H. H., et al. (2001). Formation of chiral morphologies through selective binding of amino acids to calcite surface steps. Nature 411, 775–779. doi: 10.1038/35081034
Oschmann, W. (2008). Sclerochronology: editorial. Int. J. Earth Sci. 98, 1–2. doi: 10.1007/s00531-008-0403-3
OSPAR (2010). The Quality Status Report 2010. Available onlne at: http://qsr2010.ospar.org/en/index.html (Accessed November 19, 2015).
Parente, V., Ferreira, D., Moutinho dos Santos, E., and Luczynski, E. (2006). Offshore decommissioning issues: deductibility and transferability. Energy Policy 34, 1992–2001. doi: 10.1016/j.enpol.2005.02.008
Pascoe, P. L., Parry, H. E., and Hawkins, A. J. S. (2009). Observations on the measurement and interpretation of clearance rate variations in suspension-feeding bivalve shellfish. Aquat. Biol. 6, 181–190.
Pearce, N. J. G., and Mann, V. L. (2006). Trace metal variations in the shells of Ensis siliqua record pollution and environmental conditions in the sea to the west of mainland Britain. Mar. Pollut. Bull. 52, 739–755. doi: 10.1016/j.marpolbul.2005.11.003
Perkins, W. T., Fuge, R., and Pearce, N. J. G. (1991). Quantitative analysis of trace elements in carbonates using laser ablation inductively coupled plasma mass spectrometry. J. Anal. At. Spectrom. 6, 445–449. doi: 10.1039/ja9910600445
Peterson, B. J., and Fry, B. (1987). Stable Isotopes in Ecosystem Studies. Annu. Rev. Ecol. Syst. 18, 293–320. doi: 10.1146/annurev.es.18.110187.001453
Pilkey, O. H., and Harris, R. C. (1966). The effect of intertidal environment on the composition of calcareous skeletal material1. Limnol. Oceanogr. 11, 381–385. doi: 10.4319/lo.1966.11.3.0381
Pitts, L. C., and Wallace, G. T. (1994). Lead deposition in the shell of the bivalve, Mya arenaria: an indicator of dissolved lead in seawater. Estuar. Coast. Shelf Sci. 39, 93–104. doi: 10.1006/ecss.1994.1051
Poulain, C., Gillikin, D. P., Thébault, J., Munaron, J. M., Bohn, M., Robert, R., et al. (2015). An evaluation of Mg/Ca, Sr/Ca, and Ba/Ca ratios as environmental proxies in aragonite bivalve shells. Chem. Geol. 396, 42–50. doi: 10.1016/j.chemgeo.2014.12.019
Price, G. D., and Pearce, N. J. G. (1997). Biomonitoring of pollution by Cerastoderma edule from the British Isles: a laser ablation ICP-MS study. Mar. Pollut. Bull. 34, 1025–1031. doi: 10.1016/S0025-326X(97)00098-2
Protasowicki, M., Dural, M., and Jaremek, J. (2007). Trace metals in the shells of blue mussels (Mytilus edulis) from the Poland coast of Baltic sea. Environ. Monit. Assess. 141, 329–337. doi: 10.1007/s10661-007-9899-4
Puente, X., Villares, R., Carral, E., and Carballeira, A. (1996). Nacreous shell of Mytilus galloprovincialis as a biomonitor of heavy metal pollution in Galiza (NW Spain). Sci. Total Environ. 183, 205–211. doi: 10.1016/0048-9697(96)05066-8
Purchase, N. G., and Fergusson, J. E. (1986). Chione (austrovenus) stutchburyi, a New Zealand cockle, as a bio-indicator for lead pollution. Environ. Pollut. Ser. B Chem. Phys. 11, 137–151. doi: 10.1016/0143-148X(86)90040-6
Quitmyer, I. R., Jones, D. S., and Arnold, W. S. (1997). The sclerochronology of hard clams, Mercenaria spp., from the South-Eastern U.S.A.: a Method of elucidating the zooarchaeological records of seasonal resource procurement and seasonality in prehistoric shell middens. J. Archaeol. Sci. 24, 825–840. doi: 10.1006/jasc.1996.0163
Rabalais, N. N., Turner, R. E., Gupta, B. K. S., Boesch, D. F., Chapman, P., and Murrell, M. C. (2007). Hypoxia in the northern Gulf of Mexico: does the science support the plan to reduce, mitigate, and control hypoxia? Estuaries Coasts 30, 753–772. doi: 10.1007/BF02841332
Raith, A., Perkins, W. T., Pearce, N. J. G., and Jeffries, T. E. (1996). Environmental monitoring on shellfish using UV laser ablation ICP-MS. Fresenius J. Anal. Chem. 355, 789–792. doi: 10.1007/s0021663550789
Ramsay, K., Kaiser, M. J., Richardson, C. A., Veale, L. O., and Brand, A. R. (2000). Can shell scars on dog cockles (Glycymeris glycymeris L.) be used as an indicator of fishing disturbance? J. Sea Res. 43, 167–176. doi: 10.1016/S1385-1101(00)00006-X
Reynolds, D. J., Butler, P. G., Williams, S. M., Scourse, J. D., Richardson, C. A., Wanamaker, A. D. Jr., et al. (2013). A multiproxy reconstruction of Hebridean (NW Scotland) spring sea surface temperatures between AD 1805 and 2010. Palaeogeogr. Palaeoclimatol. Palaeoecol. 386, 275–285. doi: 10.1016/j.palaeo.2013.05.029
Richardson, C. A. (2001). “Molluscs as archives of environmental change,” in Oceanography and Marine Biology: An Annual Review, Vol. 39. (London: Aberdeen University Press/Allen & Unwin), 103–164.
Richardson, C. A., Chenery, S. R. N., and Cook, J. M. (2001). Assessing the history of trace metal (Cu, Zn, Pb) contamination in the North Sea through laser ablation : ICP-MS of horse mussel Modiolus modiolus shells. Mar. Ecol. Prog. Ser. 211, 157–167. doi: 10.3354/meps211157
Riisgård, H. U., and Larsen, P. S. (2015). Physiologically regulated valve-closure makes mussels long-term starvation survivors: test of hypothesis. J. Mollus. Stud. 81, 303–307. doi: 10.1093/mollus/eyu087
Riisgård, H. U., Lassen, J., and Kittner, C. (2006). Valve-gape response times in mussels (Mytilus edulis)—effects of laboratory preceding-feeding conditions and in situ tidally induced variation in phytoplankton biomass. J. Shellfish Res. 25, 901–911. doi: 10.2983/0730-8000(2006)25[901:VRTIMM]2.0.CO;2
Risk, M. J., Sayer, B. G., Tevesz, M. J. S., and Karr, C. D. (1996). Comparison of the organic matrix of fossil and recent bivalve shells. Lethaia 29, 197–202. doi: 10.1111/j.1502-3931.1996.tb01876.x
Robson, A., Thomas, G., De Leaniz, C. G., and Wilson, R. (2009). Valve gape and exhalant pumping in bivalves: optimization of measurement. Aquat. Biol. 6, 191–200. doi: 10.3354/ab00128
Robson, A., Wilson, R., and de Leaniz, C. G. (2007). Mussels flexing their muscles: a new method for quantifying bivalve behaviour. Mar. Biol. 151, 1195–1204. doi: 10.1007/s00227-006-0566-z
Rogers, C. E., and McCarty, J. P. (2000). Climate change and ecosystems of the Mid-Atlantic Region. Clim. Res. 14, 235–244. doi: 10.3354/cr014235
Ropert-Coudert, Y., and Wilson, R. P. (2004). Subjectivity in bio-logging science: do logged data mislead. Mem. Natl. Inst. Polar Res. 58, 23–33.
Ropes, J. W., Jones, D., Murawski, S., Serchuk, F., and Jearld, A. (1984). Documentation of annual growth lines in ocean quahogs, Arctica islandica Linne. Fish. Bull. 82, 1–19.
Rosenberg, G. D., and Hughes, W. W. (1991). A metabolic model for the determination of shell composition in the bivalve mollusc, Mytilus edulis. Lethaia 24, 83–96. doi: 10.1111/j.1502-3931.1991.tb01182.x
Rounick, J. S., and Winterbourn, M. J. (1986). Stable carbon isotopes and carbon flow in ecosystems. BioScience 36, 171–177. doi: 10.2307/1310304
Royer, C., Thébault, J., Chauvaud, L., and Olivier, F. (2013). Structural analysis and paleoenvironmental potential of dog cockle shells (Glycymeris glycymeris) in Brittany, northwest France. Palaeogeogr. Palaeoclimatol. Palaeoecol. 373, 123–132. doi: 10.1016/j.palaeo.2012.01.033
Rucker, J. B., and Valentine, J. W. (1961). Salinity response of trace element concentration in Crassostrea virginica. Nature 190, 1099–1100. doi: 10.1038/1901099a0
Salánki, J., and Balogh, K. V. (1989). Physiological background for using freshwater mussels in monitoring copper and lead pollution. Hydrobiologia 188–189, 445–453. doi: 10.1007/BF00027812
Saulnier, I., and Mucci, A. (2000). Trace metal remobilization following the resuspension of estuarine sediments: Saguenay Fjord, Canada. Appl. Geochem. 15, 191–210. doi: 10.1016/S0883-2927(99)00034-7
Schekkerman, H., Meininger, P. L., and Meire, P. M. (1994). Changes in the waterbird populations of the Oosterschelde (SW Netherlands) as a result of large-scale coastal engineering works. Hydrobiologia 282–283, 509–524. doi: 10.1007/BF00024652
Schmidt, G. A., LeGrande, A. N., and Hoffmann, G. (2007). Water isotope expressions of intrinsic and forced variability in a coupled ocean-atmosphere model. J. Geophys. Res. Atmospheres 112, D10103. doi: 10.1029/2006jd007781
Schöne, B. R. (2003). A “clam-ring” master-chronology constructed from a short-lived bivalve mollusc from the northern Gulf of California, USA. Holocene 13, 39–49. doi: 10.1191/0959683603hl593rp
Schöne, B. R. (2013). Arctica islandica (Bivalvia): a unique paleoenvironmental archive of the northern North Atlantic Ocean. Glob. Planet. Change 111, 199–225. doi: 10.1016/j.gloplacha.2013.09.013
Schöne, B. R., Fiebig, J., Pfeiffer, M., Gleβ, R., Hickson, J., Johnson, A. L. A., et al. (2005a). Climate records from a bivalved Methuselah (Arctica islandica, Mollusca; Iceland). Palaeogeogr. Palaeoclimatol. Palaeoecol. 228, 130–148. doi: 10.1016/j.palaeo.2005.03.049
Schöne, B. R., Freyre Castro, A. D., Fiebig, J., Houk, S. D., Oschmann, W., and Kröncke, I. (2004). Sea surface water temperatures over the period 1884–1983 reconstructed from oxygen isotope ratios of a bivalve mollusk shell (Arctica islandica, southern North Sea). Palaeogeogr. Palaeoclimatol. Palaeoecol. 212, 215–232. doi: 10.1016/S0031-0182(04)00311-6
Schöne, B. R., Houk, S. D., Castro, A. D. F., Fiebig, J., Oschmann, W., Kröncke, I., et al. (2005b). Daily growth rates in shells of Arctica islandica: assessing sub-seasonal environmental controls on a long-lived Bivalve mollusk. Palaios 20, 78–92. doi: 10.2110/palo.2003.p03-101
Schöne, B. R., Lega, J. W., Flessa, K., Goodwin, D. H., and Dettman, D. L. (2002). Reconstructing daily temperatures from growth rates of the intertidal bivalve mollusk Chione cortezi (northern Gulf of California, Mexico). Palaeogeogr. Palaeoclimatol. Palaeoecol. 184, 131–146. doi: 10.1016/S0031-0182(02)00252-3
Schöne, B. R., Oschmann, W., Rössler, J., Castro, A. D. F., Houk, S. D., Kröncke, I., et al. (2003). North Atlantic Oscillation dynamics recorded in shells of a long-lived bivalve mollusk. Geology 31, 1037–1040. doi: 10.1130/G20013.1
Schöne, B. R., Pfeiffer, M., Pohlmann, T., and Siegismund, F. (2005c). A seasonally resolved bottom-water temperature record for the period AD 1866–2002 based on shells of Arctica islandica (Mollusca, North Sea). Int. J. Climatol. 25, 947–962. doi: 10.1002/joc.1174
Schöne, B. R., Radermacher, P., Zhang, Z., and Jacob, D. E. (2013). Crystal fabrics and element impurities (Sr/Ca, Mg/Ca, and Ba/Ca) in shells of Arctica islandica - Implications for paleoclimate reconstructions. Palaeogeogr. Palaeoclimatol. Palaeoecol. 373, 50–59. doi: 10.1016/j.palaeo.2011.05.013
Schöne, B. R., Wanamaker, A. D. Jr., Fiebig, J., Thébault, J., and Kreutz, K. (2011a). Annually resolved δ13Cshell chronologies of long-lived bivalve mollusks (Arctica islandica) reveal oceanic carbon dynamics in the temperate North Atlantic during recent centuries. Palaeogeogr. Palaeoclimatol. Palaeoecol. 302, 31–42. doi: 10.1016/j.palaeo.2010.02.002
Schöne, B. R., Zhang, Z., Jacob, D., Gillikin, D. P., Tütken, T., Garbe-Schönberg, D., et al. (2010). Effect of organic matrices on the determination of the trace element chemistry (Mg, Sr, Mg/Ca, Sr/Ca) of aragonitic bivalve shells (Arctica islandica)—Comparison of ICP-OES and LA-ICP-MS data. Geochem. J. 44, 23–37. doi: 10.2343/geochemj.1.0045
Schöne, B. R., Zhang, Z., Radermacher, P., Thébault, J., Jacob, D. E., Nunn, E. V., et al. (2011b). Sr/Ca and Mg/Ca ratios of ontogenetically old, long-lived bivalve shells (Arctica islandica) and their function as paleotemperature proxies. Palaeogeogr. Palaeoclimatol. Palaeoecol. 302, 52–64. doi: 10.1016/j.palaeo.2010.03.016
Schroeder, D. M., and Love, M. S. (2004). Ecological and political issues surrounding decommissioning of offshore oil facilities in the Southern California Bight. Ocean Coast. Manag. 47, 21–48. doi: 10.1016/j.ocecoaman.2004.03.002
Schwartzmann, C., Durrieu, G., Sow, M., Ciret, P., Lazareth, C. E., and Massabuau, J.-C. (2011). In situ giant clam growth rate behavior in relation to temperature: a one-year coupled study of high-frequency noninvasive valvometry and sclerochronology. Limnol. Oceanogr. 56, 1940–1951. doi: 10.4319/lo.2011.56.5.1940
Scourse, J., Richardson, C., Forsythe, G., Harris, I., Heinemeier, J., Fraser, N., et al. (2006). First cross-matched floating chronology from the marine fossil record: data from growth lines of the long-lived bivalve mollusc Arctica islandica. Holocene 16, 967–974. doi: 10.1177/0959683606hl987rp
Seitz, R. D., Dauer, D. M., Llansó, R. J., and Long, W. C. (2009). Broad-scale effects of hypoxia on benthic community structure in Chesapeake Bay, USA. J. Exp. Mar. Biol. Ecol. 381(Suppl.), S4–S12. doi: 10.1016/j.jembe.2009.07.004
Serban, A., Engel, M. H., and Macko, S. A. (1988). Proceedings of the 13th International Meeting on Organic Geochemistry The distribution, stereochemistry and stable isotopic composition of amino acid constituents of fossil and modern mollusk shells. Org. Geochem. 13, 1123–1129. doi: 10.1016/0146-6380(88)90298-7
Sharp, Z. (2007). Principles of Stable Isotope Geochemistry. Upper Saddle River, NJ: Pearson Prentice Hall.
Shirai, K., Schöne, B. R., Miyaji, T., Radarmacher, P., Krause, R. A. Jr., and Tanabe, K. (2014). Assessment of the mechanism of elemental incorporation into bivalve shells (Arctica islandica) based on elemental distribution at the microstructural scale. Geochim. Cosmochim. Acta 126, 307–320. doi: 10.1016/j.gca.2013.10.050
Shumchenia, E. J., Smith, S. L., McCann, J., Carnevale, M., Fugate, G., Kenney, R. D., et al. (2012). An adaptive framework for selecting environmental monitoring protocols to support ocean renewable energy development, an adaptive framework for selecting environmental monitoring protocols to support ocean renewable energy development. Sci. World J. Sci. World J. 2012:e450685. doi: 10.1100/2012/450685
Steimle, F. W., Boehm, P. D., Zdanowicz, V. S., and Bruno, R. A. (1986). Organic and trace metal levels in ocean Quahog, Arctica Islandica Linne, from the Northwestern Atlantic. Fish Bull U. S. 84:1. Available at: http://www.osti.gov/scitech/biblio/7245082 (Accessed November 17, 2015).
Stemmer, K., Nehrke, G., and Brey, T. (2013). Elevated CO 2 levels do not affect the shell structure of the Bivalve Arctica islandica from the Western Baltic. PLoS ONE 8:e70106. doi: 10.1371/journal.pone.0070106
Stephenson, A. E., DeYoreo, J. J., Wu, L., Wu, K. J., Hoyer, J., and Dove, P. M. (2008). Peptides enhance magnesium signature in calcite: insights into origins of vital effects. Science 322, 724–727. doi: 10.1126/science.1159417
Stott, K. J., Austin, W. E. N., Sayer, M. D. J., Weidman, C. R., Cage, A. G., and Wilson, R. J. S. (2010). The potential of Arctica islandica growth records to reconstruct coastal climate in northwest Scotland, UK. Quat. Sci. Rev. 29, 1602–1613. doi: 10.1016/j.quascirev.2009.06.016
Strahl, J., Dringen, R., Schmidt, M. M., Hardenberg, S., and Abele, D. (2011). Metabolic and physiological responses in tissues of the long-lived bivalve Arctica islandica to oxygen deficiency. Comp. Biochem. Physiol. Part A Mol. Integr. Physiol. 158, 513–519. doi: 10.1016/j.cbpa.2010.12.015
Swan, E. F. (1957). The meaning of strontium-calcium ratios. Deep Sea Res. 4, 71. doi: 10.1016/0146-6313(56)90036-3
Sykes, G. A., Collins, M. J., and Walton, D. I. (1995). The significance of a geochemically isolated intracrystalline organic fraction within biominerals. Org. Geochem. 23, 1059–1065. doi: 10.1016/0146-6380(95)00086-0
Szefer, P., and Szefer, K. (1990). Metals in molluscs and associated bottom sediments of the southern baltic. Helgoländer Meeresunters 44, 411–424. doi: 10.1007/BF02365477
Taylor, A. C. (1976). Burrowing behaviour and anaerobiosis in the bivalve Arctica islandica (L.). J. Mar. Biol. Assoc. U.K. 56, 95–109. doi: 10.1017/S0025315400020464
Thain, J. E., Vethaak, A. D., and Hylland, K. (2008). Contaminants in marine ecosystems: developing an integrated indicator framework using biological-effect techniques. ICES J. Mar. Sci. J. Cons. 65, 1508–1514. doi: 10.1093/icesjms/fsn120
Thébault, J., Chauvaud, L., L'Helguen, S., Clavier, J., Barats, A., Jacquet, S. É., et al. (2009). Barium and molybdenum records in bivalve shells: geochemical proxies for phytoplankton dynamics in coastal environments? Limnol. Oceanogr. 54, 1002–1014. doi: 10.4319/lo.2009.54.3.1002
Tibbetts, P. J. C., Buchanan, I. T., Gawel, L. J., and Large, R. (1992). “A comprehensive determination of produced water composition,” in Produced Water Environmental Science Research, eds. J. P. Ray and F. R. Engelhardt (Springer), 97–112. Available at: http://link.springer.com/chapter/10.1007/978-1-4615-2902-6_9 (Accessed April 2, 2016).
Toland, H., Perkins, B., Pearce, N., Keenan, F., and Leng, M. J. (2000). A study of sclerochronology by laser ablation ICP-MS. J. Anal. At. Spectrom. 15, 1143–1148. doi: 10.1039/b002014l
Tuit, C. B., and Ravizza, G. (2003). The marine distribution of molybdenum. Geochim. Cosmochim. Acta Suppl. 67, 495.
van der Oost, R., Beyer, J., and Vermeulen, N. P. E. (2003). Fish bioaccumulation and biomarkers in environmental risk assessment: a review. Environ. Toxicol. Pharmacol. 13, 57–149. doi: 10.1016/S1382-6689(02)00126-6
Vander Putten, E. V., Dehairs, F., Keppens, E., and Baeyens, W. (2000). High resolution distribution of trace elements in the calcite shell layer of modern Mytilus edulis: environmental and biological controls. Geochim. Cosmochim. Acta 64, 997–1011. doi: 10.1016/S0016-7037(99)00380-4
Wada, K, and Fljjinuki, T. (1976). “Biomineralization in bivalve molluscs with emphasis on the chemical composition of the extrapallial fluid,” in The Mechanisms of Mineralization in the Invertebrates and Plants, eds N. Watabe and K. M. Wilbur (University of South Carolina Press), I75–190.
Weidman, C. R., Jones, G. A., and Lohmann, K. C. (1994). The long-lived mollusc Arctica islandica: a new paleoceanographic tool for the reconstruction of bottom temperatures for the continental shelves of the northern North Atlantic Ocean. J. Geophys. Res. Oceans 99, 18305–18314. doi: 10.1029/94JC01882
Wells, P. S. (2011). European prehistory: A survey., ed. S. Milisauskas Springer Science & Business Media.
Welsh, K., Elliot, M., Tudhope, A., Ayling, B., and Chappell, J. (2011). Giant bivalves (Tridacna gigas) as recorders of ENSO variability. Earth Planet. Sci. Lett. 307, 266–270. doi: 10.1016/j.epsl.2011.05.032
Westermark, T., Carell, B., Mutvei, H., Forberg, S., and Kulakowski, E. (1996). Elemental content in shells of the ocean quahog, Arctica islandica L. (Mollusca: Bivalvia), from NW Europe as marine environmental archives. in Bulletin de l'Institut océanographique (Musée océanographique), 105–111. Available at: http://cat.inist.fr/?aModele=afficheN&cpsidt=2505512 (Accessed July 2, 2015).
Wilson, R. P., Shepard, E., and Liebsch, N. (2008). Prying into the intimate details of animal lives: use of a daily diary on animals. Endanger. Species Res. 4, 123–137. doi: 10.3354/esr00064
Wilson, R., Reuter, P., and Wahl, M. (2005). Muscling in on mussels: new insights into bivalve behaviour using vertebrate remote-sensing technology. Mar. Biol. 147, 1165–1172. doi: 10.1007/s00227-005-0021-6
Witbaard, R. (1997). Tree of the Sea: The Use of the Internal Growth Lines in the Shell of Arctica Islandica (Bivalvia, Mollusca) for the Retrospective Assessment of Marine Environmental Change. Doctoral thesis, University of Groningen.
Witbaard, R., Duineveld, G. C. A., and Bergman, M. (2001). The effect of tidal resuspension on benthic food quality in the southern North Sea. Senckenberg. Maritima 31, 225–234. doi: 10.1007/BF03043031
Zeebe, R., and Wolf-Gladrow, D. (2001). CO2 in Seawater: Equilibrium, Kinetics, Isotopes, Vol. 65. Amsterdam: Elsevier Oceanography Book Series.
Keywords: bivalve, shell, environmental monitoring, baseline, sclerochronology
Citation: Steinhardt J, Butler PG, Carroll ML and Hartley J (2016) The Application of Long-Lived Bivalve Sclerochronology in Environmental Baseline Monitoring. Front. Mar. Sci. 3:176. doi: 10.3389/fmars.2016.00176
Received: 26 May 2016; Accepted: 31 August 2016;
Published: 23 September 2016.
Edited by:
Angel Borja, AZTI, SpainReviewed by:
Jose M. Riascos, Universidad del Valle, ColombiaEugenio Alberto Aragon-Noriega, Centro de Investigaciones Biológicas del Noroeste S C, Mexico
Copyright © 2016 Steinhardt, Butler, Carroll and Hartley. This is an open-access article distributed under the terms of the Creative Commons Attribution License (CC BY). The use, distribution or reproduction in other forums is permitted, provided the original author(s) or licensor are credited and that the original publication in this journal is cited, in accordance with accepted academic practice. No use, distribution or reproduction is permitted which does not comply with these terms.
*Correspondence: Juliane Steinhardt, anVsaWFuZS5zdGVpbmhhcmR0QGdtYWlsLmNvbQ==