- Jena School for Microbial Communication, Institute for Inorganic and Analytical Chemistry, Friedrich Schiller University Jena, Jena, Germany
The roles of organic matter in seawater have often been discussed from the aspect of metal toxicity and bioavailability in seawater. In fact, organic ligands, as part of the organic matter, can work as a trace metal ion buffer system. At the same time, however, the release of well-defined metal chelators as exudates by, for example, marine bacteria is necessary to compete with natural metal complexes and sustain the metal acquisition required for several processes including nitrogen fixation. The identification, isolation, and structure elucidation of chelators is, thus, essential to our understanding of metal stress management in the natural habitat and role of these chelators on cellular process. The isolation of an organic ligand from its chemosphere is a challenging task. The purpose of this paper is, therefore, to give an additional perspective on how the effective application of stable isotope pairs of a metal of interest (both cations and oxoanions) combined with mass spectrometric analyses can pave the way to discovering new organic ligands (i.e., metallophores) and the chelating characteristics of dissolved organic matter (DOM): Pairs of isotopes, such as 54Fe and 58Fe (or any other pair of available isotopes of a given metal), can be used to create easily detectable unique isotopic signatures in mass spectra when they are bound by chelators. The identification of organic ligands is outlined for a proposed model system of mutualistic interactions between the green macroalga Ulva (Chlorophyta) and associated bacteria, as well as discussed briefly for DOM along land-sea gradients. Overall, the characterization of a broader spectrum of chelators in aquatic systems will open a new window to decipher the eco-physiological functions of organic ligands as a metal ion buffer and metallophores in metal cycling in marine ecosystems.
A Short Introduction: Metal Acquisition in (Macro)Algae and Associated Bacteria
Maintaining a sufficient internal concentration of essential macro- and micronutrients, despite extracellular environmental stress, is an essential function for any organisms (i.e., nutrient homeostasis). If a given nutrient is insufficiently available and its concentration drops below a critical threshold, growth becomes limited, as demonstrated for several algal species. Conversely, when the external concentration of nutrients increases higher than an acceptable value, growth is impaired because the nutrient becomes toxic. Overall, algal metal homeostasis has to balance between two extremes, enabling the adequate amounts of micronutrients to maintain metal-requiring processes in macroalgae, but not becoming toxic. Heavy metals are, thus, a good example of the necessity of a well-controlled micronutrient uptake and storage in marine algae (Sunda et al., 2005; Hopkinson and Morel, 2009).
The achievement of metal homeostasis requires a well-controlled acquisition of trace metals that both allows improving metal availability (e.g., Fe, Zn, and Mo) under metal limiting conditions and even controls metal entry under metal toxic conditions (e.g., Cu, Cd). The acquisition of metal is, hence, a complex multiple step process, whereby chelators are potentially involved in various steps. Originally known as iron carriers (i.e., siderophores), many chelating agents have an adaptable coordination chemistry not only for cations like Fe, but also for oxoanions, which might be particularly relevant in understanding metal-ligand speciation in ocean waters that are abundant in V(V) and Mo(VI) (Butler, 1998; Soe et al., 2015).
In the terrestrial world, besides important studies on metal acquisition of bacteria (Andrews et al., 2003; Ma et al., 2009), major contributions were made to the understanding of metal homeostasis of plant-microbe interactions and nitrogen-fixing bacteria (Clemens et al., 2002; González-Guerrero et al., 2014). In this context, several model studies were carried out with the nitrogen-fixing soil bacterium Azotobacter vinelandii releasing the tris-chatecholate siderophore protochelin (Cornish and Page, 1995; Kraepiel et al., 2009; Harrington et al., 2012). Protochelin is utilized for Fe(III)-, Mo(VI)-, and V(V)-uptake and detoxifies W(VI) (Bellenger et al., 2008; Wichard et al., 2008), demonstrating the multiple functions of one and the same ligand (= metallophore). Overall, the metallophores are exuded by the organism and mobilize the target metal from either a mineral or organic nutrient source by ligand exchange. The target metal–metallophore complex formed is subsequently taken up by the organism through a specific uptake system (Kraepiel et al., 2009; Kraemer et al., 2015).
In marine systems, the trace metal acquisition strategies of the multicellular macroalgae remain largely unknown, although these organisms represent important ecological niches in the coastal marine environment (Miller et al., 2014). This perspective paper, thus, highlights the identification of both algal/bacterial metallophores and organic ligands as part of the dissolved organic matter (DOM) (Figure 1), with a specific focus on the chemosphere of the marine macroalga Ulva and its associated bacteria (Figure 2). Both alga and bacteria contribute to the “soup of organic ligands” which finally increase the bioavailability of metals due to various ligand exchanges with DOM, in the first place, or between each other in a sequestered recruitment process (Figure 2A) when the complex has a sufficiently low enough thermodynamic stability to undergo ligand-exchange reactions (Kraemer et al., 2006). Weak organic ligands can even increase the bioavailability of various metals in the presence of strong organic ligands as shown for Cu, Zn, and Cd (Aristilde et al., 2012; Walsh et al., 2015). Finally, the metallophores transport the scavenged trace metal into the cell mediated by e.g., sideroreceptors (Morrissey and Bowler, 2012). The identification of metal organic ligands in natural samples will pave the way for understanding the organic ligand-mediated metal acquisition (Strategy II) shuttled from DOM to alga (via bacterial metallophores). Hereby, chelator-mediated uptake of iron can also occur indirectly by in situ photolysis of the ligand delivering the essential nutrients to the algae, which was proven for dinoflagellates (Amin et al., 2009a).
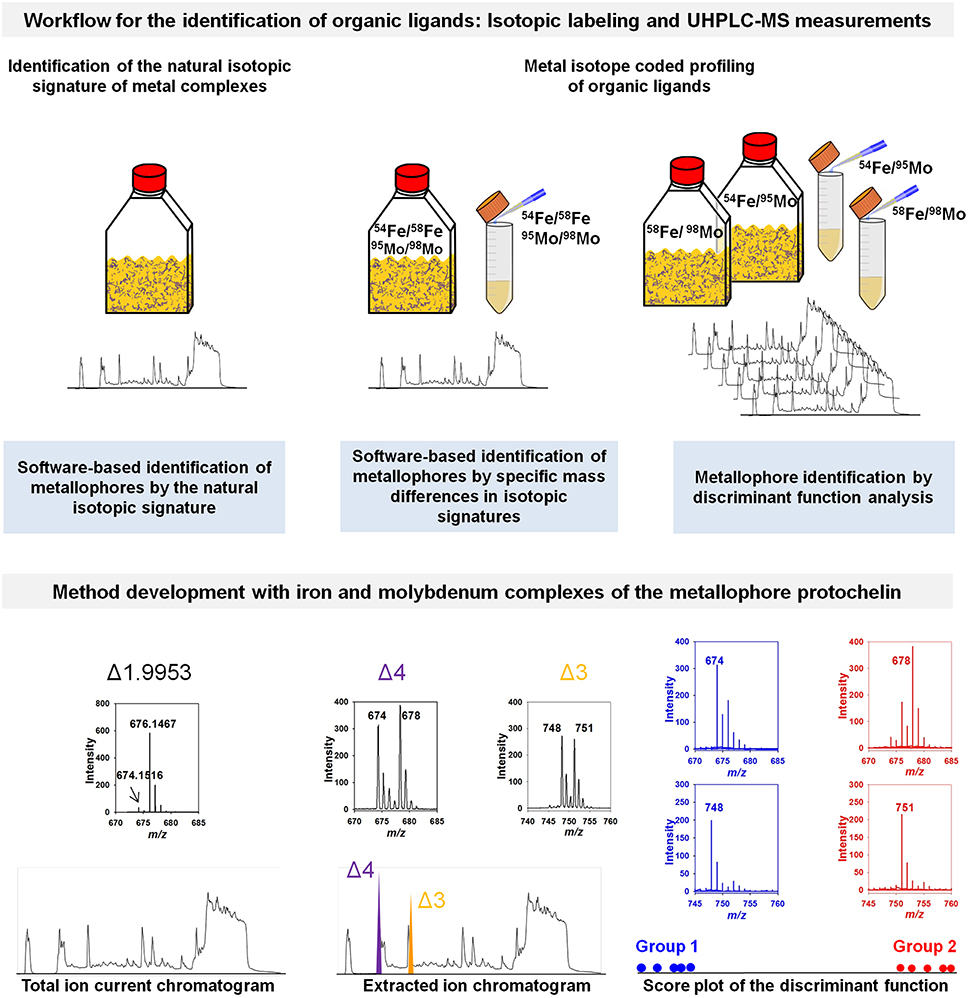
Figure 1. Conceptual workflow for the identification of organic ligands. Three approaches are shown using the isotopic signature of metal complexes for the identification of organic ligands and metallophores (exemplarily demonstrated for the metallophore protochelin). Using high resolution mass spectrometry allows one to identify the natural iron isotopic pattern. Unique isotopic signatures of potential candidates in mass spectra can be identified upon labeling potential chelators with isotopically pure pairs of, for example, 54Fe and 58Fe or 95Mo and 98Mo in natural samples or extracts with an improved signal-to-noise ratio (Deicke et al., 2014). The inserts show mass spectra (of the respective treatments above) recorded in negative ion mode either by Q-Exactive Orbitrap (Thermo Scientific, Germany) or by Q-ToF (Waters, UK) mass spectrometers coupled to an ultra-performance liquid chromatography (UHPLC). Most importantly, the chelating properties of potential chelators must be preserved during the whole analytical process (Deicke et al., 2013). Data processing can be achieved by identification of specific mass differences in the isotopic signatures or discriminant function analysis as outlined by Deicke et al. (2014). Plots are reproduced from Deicke et al. (2014) with permission from the Royal Society of Chemistry.
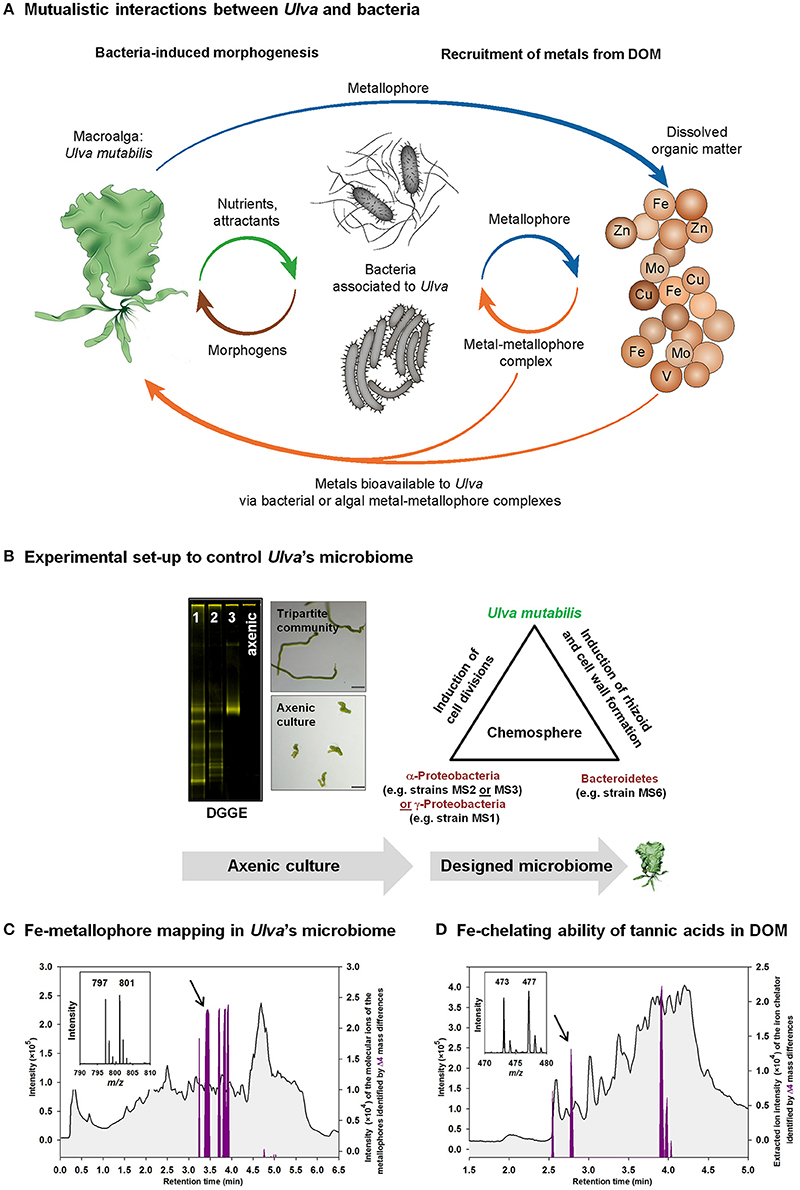
Figure 2. Studying metal acquisitions in Ulva's chemosphere. (A) Mutualistic interactions are vital for Ulva and bacteria by releasing morphogens, nutrients, and attractants, and by controlling the metal acquisition by ligand exchange processes (strategy II) from particulate or dissolved metal-organic matter complexes within the chemosphere. (B) Axenic cultures of Ulva have to be established to achieve reproducible and repeatable conditions for laboratory studies. These conditions need to be proven rigorously by, for example, PCR-based techniques such as denaturing gradient gel electrophoresis (DGGE) of the supernatant of axenic cultures. The successful purification process can be monitored by progressive purification stages from lane 1 to 3 until axenic cultures are achieved (DGGE-gel) (see also Wichard, 2015). Afterwards, the impact of a designed microbiome can be reliably tested using tri- or multiple-partite systems of U. mutabilis and algal morphogenesis-inducing bacteria. Microscopic pictures show an axenic callus-like culture (bottom panel) in comparison to the normal development of 2- to 3-week-old germlings under conditions of Ulva's tripartite community (top panel) (magnification bars = 100 μm). (C,D) Upon labeling with 54Fe and 58Fe, the extracted chromatograms (purple line), identified by the specific Δ4 mass differences of the molecular ions of the iron complexing ligands, are shown on top of the total ion current chromatogram (TIC): (C) Identification of Fe-metallophores produced by bacteria associated to Ulva within the tripartite community. (D) Mapping of tannic acids bound to iron as a part of dissolved organic matter (DOM) (Deicke et al., 2014).
Toward the Identification of Organic Ligands in Marine Dissolved Organic Matter
Marine DOM contains metal-binding functional groups (such as carboxylates, phenols, amines, and thiols) with metal-binding affinities and ligand densities spanning many orders of magnitude (Aiken et al., 2011). DOM comprises thus organic ligands that can work as “metal ion buffers” or metallophores. However, their prevalence among marine DOM is poorly established due to the few attempts to determine metal complexes using mass spectrometric approaches. Consequently, the picture of the recruitment processes, starting with the identification of the respective metal speciation till the acquisition and uptake, is pretty incomplete and important pieces of the mosaic are missing for the understanding of the complete metal biogeochemical cycles.
Many of the first-row transition metal ions in seawater are (partially) complexed by undefined organic ligands. Complexing characteristics have been studied widely by, for example, electrochemical (Van Den Berg, 1984; Kramer, 1986; Hering et al., 1987; Buffle, 1988; Gledhill and van den Berg, 1994), chromatographic (Mills et al., 1987; Baffi et al., 1992) and chemiluminescent (Sunda and Huntsman, 1991) techniques for decades and, more recently, on the scale of explorative field surveys (e.g., during GEOTRACES mission). The presence of carboxylate and phenolate groups gives, for example, the humic acids, as part of DOM, the ability to form complexes not only with cations such as Fe(II) and Fe(III), but also with oxoanions such as molybdate and vanadate (Ross et al., 2000; Deicke et al., 2014) measured by mass spectrometry. Despite all these advances in methodology, “much work is still be done in coupling voltammetry, mass spectrometry techniques, and process studies to better characterize the nature and cycling of Fe-binding ligands in the marine environment” as Gledhill and Buck (2012) have recently concluded in their review.
In marine waters of the photic zone it was demonstrated that most ferric ion is almost quantitatively complexed under iron-limited conditions by organic ligands (Donat and Bruland, 1995). These iron complexes (FeL) have pretty high conditional stability constants in seawater, ranging from Log 19 to Log 23, which is similar to the stability of bacterial siderophore complexes (Rue and Bruland, 1995). Whether, these strong ligands are indeed siderophores is difficult to establish due to their low concentrations, making the purification and further eco-physiological uptake experiments with the target organism difficult. Nevertheless, other than the stability constant of metal-ligand complexes, several groups succeeded in elucidating the structure of several ligands released by marine bacteria in culture (Reid et al., 1993; Butler and Martin, 2005; Amin et al., 2009b; Sandy and Butler, 2009).
There have only been few reports about the direct identification of metallophores and other organic ligands in the DOM of natural marine samples. Sequestration and decomposition processes of DOM are very dynamic, particularly in the presence of bacteria and sunlight. Thus, the determination of (low concentrated) organic ligands becomes challenging which is essential to understand ligand exchange processes with bacterial or algal metallophores. Up to now strong hydroxamate siderophore such as the ferrioaxamines were identified in pre-concentrated water samples collected in the Atlantic Ocean using high performance liquid chromatography coupled to electrospray ionization mass spectrometry (HPLC-ESI-MS) (Gledhill et al., 2004; Mawji et al., 2008). Exploring siderophores in complex matrices can be also achieved upon HPLC-separation by ionizing the samples with inductively coupled plasma-mass spectrometry (ICP-MS). Eluated compounds need to be subsequently analyzed by HPLC-ESI-MS to identify the organic ligand complexing with iron (Mawji et al., 2011; Boiteau et al., 2013). Whereas, the ICP-MS combined with LC allows the easy quantification of metals (and metal-complexes) at very low concentration, ESI-MS measurements provide additional valuable information for structure elucidation, particularly if MS/MS experiments are applied. Using high-resolution-ESI-MS (HR-ESI-MS), hydroxamate-type ligands have been directly identified in seawater samples (Velasquez et al., 2011) or e.g., in fungi (Lehner et al., 2013; Pourhassan et al., 2014) via their natural iron-isotope signature. This approach was improved by the application of a newly developed MatLab based program for the identification of natural isotope patterns of metal complexes (Baars et al., 2014). Using this algorithm, Baars and co-workers could also determine novel metallophores in the culture medium of A. vinelandii (Baars et al., 2016). In addition, ESI-MS/MS experiments were suggested to identify metal-ligand complexes through the release of metal species from its respective complex (Tsednee et al., 2016).
With this perspective paper, I like to suggest a targeted analysis of low-molecular-weight organic ligands based on fast UHPLC-ESI-MS measurements (Figure 1). Metal isotope coded profiling (MICP) of metallophores can reveal organic metal-binding ligands in complex matrices through the identification of specific isotopic signatures. Replacement of, for example, natural iron or molybdenum with isotopically pure 54Fe/58Fe (ratio 1:1) or 95Mo/98Mo (ratio 1:1) causes easily detectable unique isotopic signatures in the mass spectra of potential metal-complexing ligands (Deicke et al., 2013, 2014) (Figure 1). This can be achieved under laboratory conditions not only in growth media, but also by spiking directly seawater or solid-phase extracts. Importantly, as the relative affinity of the metallophores for e.g., Mo or Fe is dependent on the pH, all experiments needs to be conducted under pH-controlled conditions. The sensitivity of the measurements often increases due to the additional complexation of available ligands. Moreover, the improved ionization efficiency of some metal complexes helps to enhance the signal-to-noise ratio compared to the free ligand at the same chromatographic conditions (Deicke et al., 2013). The methodology does not necessarily depend on HR-ESI-MS measurements and can be applied to any mass spectrometer. However, more sensitive measurements, for example by UHPLC-HR-Q-Exactive Orbitrap mass spectrometry (Thermo-Fisher), might detect even low concentrated metal complexes without solid-phase extraction and further perturbation of the sample. Besides the fact that the methodology cannot be applied to monoisotopic elements, experimenters have to be always conscious about several parameters such as, for example, the selectivity of solid-phase extractions, the ionization efficiency of ESI as well as pH changes and ligand exchanges during the analytical process (see also Di Marco and Bombi, 2006; Keith-Roach, 2010).
The chemical exploration of both low-molecular-weight organic ligands and the metallophore production is a crucial prerequisite to study metal acquisition and homeostasis of an organism. With MICP it was shown that, besides the metallophore protochelin, tannic acids bind molybdate and iron and, thus, contribute to the metal bioavailability (Deicke et al., 2014; Jouogo et al., 2016). A. vinelandii produced a higher amount of metallophores in the presence of tannic acids, which coincides with an active, regulated, and concomitant acquisition of molybdenum and vanadium (Jouogo et al., 2016). The data suggest that tannic acid contribute to the metal bioavailability by both preventing iron precipitation and by decreasing the amount of easily available molybdate. In this way with MICP, two birds can be killed with one stone: (i) the identification of metallophores for metal uptake and (ii) organic ligands which solely work as metal buffer in DOM. Upon partly purification, the stable isotope-labeled samples can be then directly applied to short-term-uptake experiments monitored by ICP-MS to test if an essential metal can be recruited from a newly identified metallophore and accumulated by the organism.
Perspective Applications
As speciation and uptake of trace metals seems to be controlled by organic ligands, our laboratory suggests two strategies to unveil the eco-physiological functions of ligands: (i) controlled mechanistic studies of metal management of the biota, and (ii) explorative targeting surveys of any organic ligand for a given metal. I propose two research lines for laboratory and field-based studies below.
Model System: Ulva and Its Associated Bacteria
The marine macroalga Ulva is a cosmopolitan seaweed that causes green tides, for example, in shallow costal environments. This phenomenon is particularly studied in the Yellow Sea (China), using field samples to unveil, for example, growth and development under eutrophic coastal conditions. In our laboratory, we have established a model system where Ulva grows under strictly controlled conditions starting with an axenic feedstock of gametes inoculated with specific bacteria inducing the algal morphogenesis in artificial seawater medium (Wichard and Oertel, 2010; Spoerner et al., 2012) (Figure 2B). Hereby, bacteria are essential, as Ulva develops into a callus-like morphotypes under axenic conditions (Wichard, 2015). The combination of two specific bacteria from the Rhodobacteraceae (or Halomonadaceae) and Flavobacteriaceae can completely recover the growth and morphogenesis of axenic Ulva mutabilis cultures forming a symbiotic tripartite community by releasing morphogenetic compounds (Spoerner et al., 2012), which can also be determined in natural lagoon waters (Grueneberg et al., 2016). The acquisition of trace metals can, thus, be easily simulated in the tripartite community under standardized conditions, along with explorative chemical studies and characterization of new organic ligands used as metallophores for metal recruitment or “metal buffers” in the habitat as a result of, for example, environmental changes. Indeed, the fast growing Ulva might face risk of nitrogen and iron limitations in eutrophic waters, which may also affect its microbiome directly, which is, vice versa, essential for Ulva's proper growth, development and morphogenesis. It was shown recently that macroalgae blooms favor heterotrophic diazotrophic bacteria even in nitrogen-rich coastal surface waters, due potentially to the fast uptake of nitrogen by Ulva (Zhang et al., 2015). Given the role of trace metals in the production of Mo-, V-, and/or Fe-dependent nitrogenases, green tides may, thus, trigger metallophore production to increase the bioavailability of trace elements. All these natural conditions can be simplified under controlled laboratory conditions with a designed microbiome starting with axenic cultures of Ulva (Figure 2B).
Laboratory experiments are necessary to understand the dynamics of metal bioavailability and recruitment in macroalgae. Model experiments will help to integrate the chemist's and biologist's perspective of “the impact of microbial metabolism on marine dissolved organic matter” (Kujawinski, 2011). To follow up the intriguing ideas expressed in Kujawinski's review, our perspective research line aims, in particular, at investigating the metallome (= all biologically active compounds that contain a metal atom) and the dynamics of metal acquisition within a well-defined community of the green macroalga U. mutabilis and an experimentally designed microbiome. Pilot studies have recently revealed that bacteria tightly associated to U. mutabilis release unknown organic ligands which complex iron (Figure 2B). Released microbial bacterial siderophores become, therefore, part of the organic matter in the chemosphere and contribute to the recruitment of, for example, iron bound to organic matter and other sources that can now be studied exemplarily within the tripartite community in U. mutabilis (Figure 2C). In fact, it has already been demonstrated that nitrate accumulation in Ulva thalli in nitrate-rich lagoon waters (Sacca di Goro, Italy) was inversely related to Fe uptake, indicating an influence of Fe limitation on N acquisition (Viaroli et al., 2005). Fast growing Ulva might, thus, experience limiting conditions due to the low concentration or poor availability of iron. I hereby hypothesize that Ulva benefits from its associated microbiome governing the Fe recruitment. Bacterial siderophores would be shared as public goods (Dumas and Kuemmerli, 2012) within a bacterial-algal mutualism, where heterotrophic bacteria are fed by the algae through the release of carbon sources. Carbohydrates released by Ulva might also enhance the iron bioavailability as shown for saccharides in phytoplankton (Hassler et al., 2011). In addition, algal and bacterial exopolymeric substances (EPS) in biofilms can be a source of weaker and stronger ligands in the range from log = 10.4 to log = 12.0 (Hassler et al., 2015). The protocol of MICP has to be further developed for the upcoming research field of metals associated with macromolecules.
Ulva's growth medium can also be modulated by the addition of humic or fulvic acids or any other potential ligands which affect the bioavailability of metals directly and mimic natural conditions, where metals speciation is controlled by organic matter. Laboratory experiments will allow us to measure (i) quantitative and (ii) qualitative changes of the metallophore profile due to environmentally relevant changes in the growth conditions of the bacterial-macroalgal community (Wichard et al., 2009; Jouogo et al., 2016).
The Role of Land-Sea Processes in Modulating the Abundance and Prevalence of Chelators: Finding the Needle in the Haystack
It was suggested that only small amounts of bioavailable iron exist for macroalgae in costal seawater not affected by inflow from land and that a significant quantity of dissolved iron is complexed to organic ligands, which was demonstrated by 59Fe-labeled iron uptake experiments (Suzuki et al., 1995). With an increasing amount of organic ligands, algae growth declined markedly due to the reduced bioavailable iron concentrations because of complexation (Laglera and van den Berg, 2009; Lee et al., 2009). Combined approaches are, thus, necessary to obtain a complete picture of global element cycles comprising both terrestrial and oceanic components (Butler, 1998). While a lot of investigations dealt originally with the vertical distribution of DOM (Hirose and Tanoue, 1998), there are an increasing number of studies which explore the prevalence and changes of the DOM pool along the estuarine gradient (Sleighter and Hatcher, 2008; Dubinenkov et al., 2015; Medeiros et al., 2015). Hereby, it will be still be challenging to focus on the perspective organic ligands due to the heterogeneous DOM composition (Kujawinski, 2011). However, a comparison of various solid phases has already revealed reliable extraction protocols of DOM (Dittmar et al., 2008; Waska et al., 2015) that can be combined with the isotopic coded profiling proposed (Figures 1, 2D). As DOM contains strong organic ligands also as a signal of biological fresh matter (Hirose, 2000), the identification of organic ligands might be particularly successful in areas where fresh biological material is available. Proxies for terrestrial DOM, for instance, have revealed that terrestrial DOM fluxes, which are often derived from the decomposition of vascular land plants and mobilized nearby river systems, contribute significantly to the total DOM in estuaries and coastal waters (Osburn et al., 2015).
Recent studies continue to focus on the determination and pattern of dissolved matter facilitated by either chromatographic methods (Sandron et al., 2015) or by directly injected solid-phase- extracted compounds using Fourier transform ion cyclotron resonance mass spectrometry (FT-ICR-MS) without additional pre-procedural steps (Hertkorn et al., 2006; Dittmar et al., 2008; Herzsprung et al., 2015). Indeed, the chemical identity of DOM will be the key to the most urgent questions regarding the cycling of DOM in the ocean (Koch et al., 2005) and the subsequent identification of its chelating ability. Overall, hydroxyl functional groups, which can be found predominantly in humic acids (Thurman, 1985), hydroxamic acids, α-hydroxy carboxylic acids, carboxylic acids, oxazolone, and thiazolidine groups are of special interest depending on the metal and its oxidation states from the perspective of coordination chemistry (Springer and Butler, 2016). Some studies have already assigned the specific molecular identity of DOM in seawater samples to carboxyl-rich alicyclic molecules, which are expected to be candidates for metal binding and multiple coordination across cations that also supports aggregation processes (Hertkorn et al., 2006), others identified carbohydrates, lipids, amino acids, amino sugars, and tannins (Sleighter and Hatcher, 2008). In any case, as the DOM pool is changing continuously and mixing can only partly account for changes along the estuarine gradient (Asmala et al., 2016), the various transformation processes at the land-sea interface will be not predictable. The search for potential ligands or metal complexes along the estuarine gradient becomes, therefore, like “finding the needle in the haystack.”
Land-sea processes are particular intriguing as the abundance and fingerprint of organic ligands might vary adjacent to the original source from, for example, terrestrial, permafrost soil, riverine, or marine waters. The source-specific patterns of chelators can change distinctly differently from land to open sea. The FT-ICR-MS studies on Arctic DOM have already demonstrated latitude, pH and water-dependent compositions (Roth et al., 2013; Dubinenkov et al., 2015).
Whereas DOM has often been used as a proxy for bio-productivity in terms of a carbon source, its specific impact as a “metal buffer” for biological processes should be investigated as well. Upon the administration of individual isotopes or isotopic pairs, for example, 54Fe/58Fe, 63Cu/65Cu, 66Zn/68Zn, or 95Mo/98Mo and subsequent measurements by UHPLC-MS or even by direct-inlet FT-ICR-MS organic ligands might be identified that contribute potentially to the regulation of bioavailability and toxicity of metals in the water column.
Conclusion
Both controlled laboratory and explorative field studies are necessary to obtain a complete picture of the element cycles in the chemosphere of the suggested model community of Ulva and its bacteria. Certainly genome and transcriptome data as well as the establishment of stable transformants in metal homeostasis dysfunctions will help to close the gap of our understanding of Ulva's metal homeostasis. An orchestrated approach is, thus, needed to combine explorative molecular studies (targeted and “omics” analysis, i.e., metabolomics and transcriptomics) with the essential biological and oceanographic metadata and upcoming genomic analysis of Ulva (Wichard et al., 2015). Perspective integrated studies that include DOM analysis and the determination of chelators/metallophores will contribute to an improved understanding of DOM-microbe-algal interactions that serve as the base of the metal biogeochemical cycle and affect their own bio-productivity.
Author Contributions
TW conceived, designed, and wrote the paper.
Conflict of Interest Statement
The author declares that the research was conducted in the absence of any commercial or financial relationships that could be construed as a potential conflict of interest.
Acknowledgments
I apologize to those colleagues whose work could not be cited owing to space constraints. I want to thank the Deutsche Forschungsgemeinschaft (CRC 1127, ChemBioSys), which supported the sub-project “Metallophores as mediators for metal cycling: development of libraries for metal ion buffering and as redox carriers as well as profiling of metallophores.” The project presented in the paper has also received funding from the European Union's Horizon 2020 research and innovation programme under the Marie Sklodowska-Curie grant agreement No. 642575. I thank Prof. Dr. Georg Pohnert (University Jena) for his great support. I appreciate the efforts of the reviewers and their useful comments and suggestions for improving the manuscript.
References
Aiken, G. R., Hsu-Kim, H., and Ryan, J. N. (2011). Influence of dissolved organic matter on the environmental fate of metals, nanoparticles, and colloids. Environ. Sci. Technol. 45, 3196–3201. doi: 10.1021/es103992s
Amin, S. A., Green, D. H., Hart, M. C., Kuepper, F. C., Sunda, W. G., and Carrano, C. J. (2009a). Photolysis of iron-siderophore chelates promotes bacterial-algal mutualism. Proc. Natl. Acad. Sci. U.S.A. 106, 17071–17076. doi: 10.1073/pnas.0905512106
Amin, S. A., Green, D. H., Küpper, F. C., and Carrano, C. J. (2009b). Vibrioferrin, an unusual marine siderophore: iron binding, photochemistry, and biological implications. Inorg. Chem. 48, 11451–11458. doi: 10.1021/ic9016883
Andrews, S. C., Robinson, A. K., and Rodríguez-Quiñones, F. (2003). Bacterial iron homeostasis. FEMS Microbiol. Rev. 27, 215–237. doi: 10.1016/S0168-6445(03)00055-X
Aristilde, L., Xu, Y., and Morel, F. M. M. (2012). Weak organic ligands enhance zinc uptake in marine phytoplankton. Environ. Sci. Technol. 46, 5438–5445. doi: 10.1021/es300335u
Asmala, E., Kaartokallio, H., Carstensen, J., and Thomas, D. N. (2016). Variation in riverine inputs affect dissolved organic matter characteristics throughout the estuarine gradient. Front. Mar. Sci. 2:125. doi: 10.3389/fmars.2015.00125
Baars, O., Morel, F. M. M., and Perlman, D. H. (2014). ChelomEx: isotope-assisted discovery of metal chelates in complex media using high-resolution LC-MS. Anal. Chem. 86, 11298–11305. doi: 10.1021/ac503000e
Baars, O., Zhang, X., Morel, F. M. M., and Seyedsayamdost, M. R. (2016). The siderophore metabolome of Azotobacter vinelandii. Appl. Environ. Microbiol. 82, 27–39. doi: 10.1128/AEM.03160-15
Baffi, F., Ianni, M. C., Cardinale, A. M., Magi, E., Frache, R., and Ravera, M. (1992). Study of reversed-phase C18-silica in liquid-chromatography for the determination of free dissolved amino-acids and copper(II) amino-acid complexes at the picomole level in marine matrices. Anal. Chim. Acta 260, 99–106. doi: 10.1016/0003-2670(92)80132-q
Bellenger, J. P., Wichard, T., Kustka, A. B., and Kraepiel, A. M. L. (2008). Uptake of molybdenum and vanadium by a nitrogen-fixing soil bacterium using siderophores. Nat. Geosci. 1, 243–246. doi: 10.1038/ngeo161
Boiteau, R. M., Fitzsimmons, J. N., Repeta, D. J., and Boyle, E. A. (2013). Detection of iron ligands in seawater and marine cyanobacteria cultures by high-performance liquid chromatography-inductively coupled plasma-mass spectrometry. Anal. Chem. 85, 4357–4362. doi: 10.1021/ac3034568
Buffle, J. (1988). Complexation Reactions in Aquatic Systems; An Analytical Approach. Chichester: Ellis Horwood Publisher.
Butler, A. (1998). Acquisition and utilization of transition metal ions by marine organisms. Science 281, 207–210. doi: 10.1126/science.281.5374.207
Butler, A., and Martin, J. D. (2005). The marine biogeochemistry of iron. Met. Ions Biol. Syst. 44, 21–46.
Clemens, S., Palmgren, M. G., and Krämer, U. (2002). A long way ahead: understanding and engineering plant metal accumulation. Trends Plant Sci. 7, 309–315. doi: 10.1016/S1360-1385(02)02295-1
Cornish, A. S., and Page, W. J. (1995). Production of the triacetecholate siderophore protochelin by Azotobacter vinelandii. Biometals 8, 332–338.
Deicke, M., Bellenger, J.-P., and Wichard, T. (2013). Direct quantification of bacterial molybdenum and iron metallophores with ultra-high-performance liquid chromatography coupled to time-of-flight mass spectrometry. J. Chromatogr. A 1298, 50–60. doi: 10.1016/j.chroma.2013.05.008
Deicke, M., Mohr, J. F., Bellenger, J.-P., and Wichard, T. (2014). Metallophore mapping in complex matrices by metal isotope coded profiling of organic ligands. Analyst 139, 6096–6099. doi: 10.1039/c4an01461h
Di Marco, V. B., and Bombi, G. G. (2006). Electrospray mass spectrometry (ESI-MS) in the study of metal-ligand solution equilibria. Mass Spectrom. Rev. 25, 347–379. doi: 10.1002/mas.20070
Dittmar, T., Koch, B., Hertkorn, N., and Kattner, G. (2008). A simple and efficient method for the solid-phase extraction of dissolved organic matter (SPE-DOM) from seawater. Limnol. Oceanogr. Methods 6, 230–235. doi: 10.4319/lom.2008.6.230
Donat, J. R., and Bruland, K. W. (1995). “Trace elements in the oceans,” in Trace Elements in Natural Waters, eds B. Salbu and E. Steinnes (Boca Raton, FL: CRC Press), 247–281.
Dubinenkov, I., Flerus, R., Schmitt-Kopplin, P., Kattner, G., and Koch, B. P. (2015). Origin-specific molecular signatures of dissolved organic matter in the Lena Delta. Biogeochemistry 123, 1–14. doi: 10.1007/s10533-014-0049-0
Dumas, Z., and Kuemmerli, R. (2012). Cost of cooperation rules selection for cheats in bacterial metapopulations. J. Evol. Biol. 25, 473–484. doi: 10.1111/j.1420-9101.2011.02437.x
Gledhill, M., and Buck, K. N. (2012). The organic complexation of iron in the marine environment: a review. Front. Microbiol. 3:69. doi: 10.3389/fmicb.2012.00069
Gledhill, M., McCormack, P., Ussher, S., Achterberg, E. P., Mantoura, R. F. C., and Worsfold, P. J. (2004). Production of siderophore type chelates by mixed bacterioplankton populations in nutrient enriched seawater incubations. Mar. Chem. 88, 75–83. doi: 10.1016/j.marchem.2004.03.003
Gledhill, M., and van den Berg, C. M. G. (1994). Determination of complexation of iron(III) with natural organic complexing ligands in seawater using cathodic stripping voltammetry. Mar. Chem. 47, 41–54. doi: 10.1016/0304-4203(94)90012-4
González-Guerrero, M., Matthiadis, A., Saez, Á., and Long, T. A. (2014). Fixating on metals: new insights into the role of metals in nodulation and symbiotic nitrogen fixation. Front. Plant Sci. 5:45. doi: 10.3389/fpls.2014.00045
Grueneberg, J., Engelen, A. H., Costa, R., and Wichard, T. (2016). Macroalgal morphogenesis induced by waterborne compounds and bacteria in coastal seawater. PLoS ONE 11:e0146307. doi: 10.1371/journal.pone.0146307
Harrington, J. M., Bargar, J. R., Jarzecki, A. A., Roberts, J. G., Sombers, L. A., and Duckworth, O. W. (2012). Trace metal complexation by the triscatecholate siderophore protochelin: structure and stability. Biometals 25, 393–412. doi: 10.1007/s10534-011-9513-7
Hassler, C. S., Norman, L., Mancuso Nichols, C. A., Clementson, L. A., Robinson, C., Schoemann, V., et al. (2015). Iron associated with exopolymeric substances is highly bioavailable to oceanic phytoplankton. Mar. Chem. 173, 136–147. doi: 10.1016/j.marchem.2014.10.002
Hassler, C. S., Schoemann, V., Nichols, C. M., Butler, E. C. V., and Boyd, P. W. (2011). Saccharides enhance iron bioavailability to Southern Ocean phytoplankton. Proc. Natl. Acad. Sci. U.S.A. 108, 1076–1081. doi: 10.1073/pnas.1010963108
Hering, J. G., Sunda, W. G., Ferguson, R. L., and Morel, F. M. M. (1987). A field comparison of 2 methods for the determination of copper complexation - bacterial bioassay and fixed-potential amperometry. Mar. Chem. 20, 299–312. doi: 10.1016/0304-4203(87)90064-8
Hertkorn, N., Benner, R., Frommberger, M., Schmitt-Kopplin, P., Witt, M., Kaiser, K., et al. (2006). Characterization of a major refractory component of marine dissolved organic matter. Geochim. Cosmochim. Acta 70, 2990–3010. doi: 10.1016/j.gca.2006.03.021
Herzsprung, P., Tümpling, W. V., Hertkorn, N., Harir, M., Friese, K., and Schmitt-Kopplin, P. (2015). High-field FTICR-MS data evaluation of natural organic matter: are chon5s2 molecular class formulas assigned to 13C isotopic m/z and in reality CHO components? Anal. Chem. 87, 9563–9566. doi: 10.1021/acs.analchem.5b02549
Hirose, K. (2000). “Strong organic ligands in seawater: peculiar functional groups in oceanic organic matter-synthesis,” in Dynamics and Characterization of Marine Organic Matter, eds N. Handa, E. Tanoue, and T. Hama (Tokyo: Springer), 339–382.
Hirose, K., and Tanoue, E. (1998). The vertical distribution of the strong ligand in particulate organic matter in the North Pacific. Mar. Chem. 59, 235–252. doi: 10.1016/s0304-4203(97)00095-9
Hopkinson, B. M., and Morel, F. M. M. (2009). The role of siderophores in iron acquisition by photosynthetic marine microorganisms. Biometals 22, 659–669. doi: 10.1007/s10534-009-9235-2
Jouogo, N. C., Pourhassan, N., Darnajoux, R., Deicke, M., Wichard, T., Burrus, V., et al. (2016). Effect of organic matter on nitrogenase metal cofactors homeostasis in Azotobacter vinelandii under diazotrophic conditions. Environ. Microbiol. Rep. 8, 76–84. doi: 10.1111/1758-2229.12353
Keith-Roach, M. J. (2010). A review of recent trends in electrospray ionisation-mass spectrometry for the analysis of metal-organic ligand complexes. Anal. Chim. Acta 678, 140–148. doi: 10.1016/j.aca.2010.08.023
Koch, B. P., Witt, M., Engbrodt, R., Dittmar, T., and Kattner, G. (2005). Molecular formulae of marine and terrigenous dissolved organic matter detected by electrospray ionization Fourier transform ion cyclotron resonance mass spectrometry. Geochim. Cosmochim. Acta 69, 3299–3308. doi: 10.1016/j.gca.2005.02.027
Kraemer, S. M., Crowley, D. E., and Kretzschmar, R. (2006). Geochemical aspects of phytosiderophores-promoted iron acquisition by plants. Adv. Agron. 91, 1–46. doi: 10.1016/S0065-2113(06)91001-3
Kraemer, S. M., Duckworth, O. W., Harrington, J. M., and Schenkeveld, W. D. C. (2015). Metallophores and Trace Metal Biogeochemistry. Aquat. Geochem. 21, 159–195. doi: 10.1007/s10498-014-9246-7
Kraepiel, A. M. L., Bellenger, J. P., Wichard, T., and Morel, F. M. M. (2009). Multiple roles of siderophores in free-living nitrogen-fixing bacteria. Biometals 22, 573–581. doi: 10.1007/s10534-009-9222-7
Kramer, C. J. M. (1986). Apparent copper complexation capacity and conditional stability constants in North-Atlantic waters. Mar. Chem. 18, 335–349. doi: 10.1016/0304-4203(86)90016-2
Kujawinski, E. B. (2011). “The impact of microbial metabolism on marine dissolved organic matter,” in Annual Review of Marine Science, Vol. 3, eds C. A. Carlson and S. J. Giovannoni (Palo Alto, CA: Annual Reviews), 567–599.
Laglera, L. M., and van den Berg, C. M. G. (2009). Evidence for geochemical control of iron by humic substances in seawater. Limnol. Oceanogr. 54, 610–619. doi: 10.4319/lo.2009.54.2.0610
Lee, J., Park, J. H., Shin, Y. S., Lee, B. C., Chang, N. I., Cho, J., et al. (2009). Effect of dissolved organic matter on the growth of algae, Pseudokirchneriella subcapitata, in Korean lakes: the importance of complexation reactions. Ecotoxicol. Environ. Saf. 72, 335–343. doi: 10.1016/j.ecoenv.2008.01.013
Lehner, S. M., Atanasova, L., Neumann, N. K. N., Krska, R., Lemmens, M., Druzhinina, I. S., et al. (2013). Isotope-assisted screening for iron-containing metabolites reveals a high degree of diversity among known and unknown siderophores produced by Trichoderma spp. Appl. Environ. Microbiol. 79, 18–31. doi: 10.1128/AEM.02339-12
Ma, Z., Jacobsen, F. E., and Giedroc, D. P. (2009). Coordination chemistry of bacterial metal transport and sensing. Chem. Rev. 109, 4644–4681. doi: 10.1021/cr900077w
Mawji, E., Gledhill, M., Milton, J. A., Tarran, G. A., Ussher, S., Thompson, A., et al. (2008). Hydroxamate siderophores: occurrence and importance in the Atlantic Ocean. Environ. Sci. Technol. 42, 8675–8680. doi: 10.1021/es801884r
Mawji, E., Gledhill, M., Milton, J. A., Zubkov, M. V., Thompson, A., Wolff, G. A., et al. (2011). Production of siderophore type chelates in Atlantic Ocean waters enriched with different carbon and nitrogen sources. Mar. Chem. 124, 90–99. doi: 10.1016/j.marchem.2010.12.005
Medeiros, P. M., Seidel, M., Ward, N. D., Carpenter, E. J., Gomes, H. R., Niggemann, J., et al. (2015). Fate of the Amazon River dissolved organic matter in the tropical Atlantic Ocean. Global Biogeochem. Cycles 29, 677–690. doi: 10.1002/2015gb005115
Miller, E. P., Boettger, L. H., Weerasinghe, A. J., Crumbliss, A. L., Matzanke, B. F., Meyer-Klaucke, W., et al. (2014). Surface-bound iron: a metal ion buffer in the marine brown alga Ectocarpus siliculosus? J. Exp. Bot. 65, 585–594. doi: 10.1093/jxb/ert406
Mills, G. L., McFadden, E., and Quinn, J. G. (1987). Chromatographic studies of dissolved organic-matter and copper organic-complexes isolated from estuarine waters. Mar. Chem. 20, 313–325. doi: 10.1016/0304-4203(87)90065-x
Morrissey, J., and Bowler, C. (2012). Iron utilization in marine cyanobacteria and eukaryotic algae. Front. Microbiol. 3:43. doi: 10.3389/fmicb.2012.00043
Osburn, C. L., Boyd, T. J., Montgomery, M. T., Coffin, R. B., Bianchi, T. S., and Paerl, H. W. (2015). Optical proxies for terrestrial dissolved organic matter in estuaries and coastal waters. Front. Mar. Sci. 2:127. doi: 10.3389/fmars.2015.00127
Pourhassan, N., Gagnon, R., Wichard, T., and Bellenger, J.-P. (2014). Identification of the hydroxamate siderophore ferricrocin in Cladosporium cladosporioides. Nat. Prod. Commun. 9, 539–540.
Reid, R. T., Live, D. H., Faulkner, D. J., and Butler, A. (1993). A siderophore from a marine bacterium with an exceptional ferric ion affinity constant. Nature 366, 455–458. doi: 10.1038/366455a0
Ross, A. R. S., Ikonomou, M. G., and Orians, K. J. (2000). Characterization of dissolved tannins and their metal-ion complexes by electrospray ionization mass spectrometry. Anal. Chim. Acta 411, 91–102. doi: 10.1016/s0003-2670(00)00746-7
Roth, V.-N., Dittmar, T., Gaupp, R., and Gleixner, G. (2013). Latitude and pH driven trends in the molecular composition of DOM across a north south transect along the Yenisei River. Geochim. Cosmochim. Acta 123, 93–105. doi: 10.1016/j.gca.2013.09.002
Rue, E. L., and Bruland, K. W. (1995). Complexation of iron(III) by natural organic-ligands in the central north pacific as determined by a new competitive ligand equilibration adsorptive cathodic stripping voltammetric method. Mar. Chem. 50, 117–138. doi: 10.1016/0304-4203(95)00031-l
Sandron, S., Rojas, A., Wilson, R., Davies, N. W., Haddad, P. R., Shellie, R. A., et al. (2015). Chromatographic methods for the isolation, separation and characterisation of dissolved organic matter. Environ. Sci. 17, 1531–1567. doi: 10.1039/c5em00223k
Sandy, M., and Butler, A. (2009). Microbial iron acquisition: marine and terrestrial siderophores. Chem. Rev. 109, 4580–4595. doi: 10.1021/cr9002787
Sleighter, R. L., and Hatcher, P. G. (2008). Molecular characterization of dissolved organic matter (DOM) along a river to ocean transect of the lower Chesapeake Bay by ultrahigh resolution electrospray ionization Fourier transform ion cyclotron resonance mass spectrometry. Mar. Chem. 110, 140–152. doi: 10.1016/j.marchem.2008.04.008
Soe, C. Z., Telfer, T. J., Levina, A., Lay, P. A., and Codd, R. (2015). Simultaneous biosynthesis of putrebactin, avaroferrin and bisucaberin by Shewanella putrefaciens and characterisation of complexes with iron(III), molybdenum(VI) or chromium(V). J. Inorg. Biochem. doi: 10.1016/j.jinorgbio.2016.06.015. [Epub ahead of print].
Spoerner, M., Wichard, T., Bachhuber, T., Stratmann, J., and Oertel, W. (2012). Growth and thallus morphogenesis of Ulva mutabilis (Chlorophyta) depends on a combination of two bacterial species excreting regulatory factors. J. Phycol. 48, 1433–1447. doi: 10.1111/j.1529-8817.2012.01231.x
Springer, S. D., and Butler, A. (2016). Microbial ligand coordination: consideration of biological significance. Coord. Chem. Rev. 306(Pt 2), 628–635. doi: 10.1016/j.ccr.2015.03.013
Sunda, W. G., and Huntsman, S. A. (1991). The use of chemiluminescence and ligand competition with edta to measure copper concentration and speciation in seawater. Mar. Chem. 36, 137–163.
Sunda, W. G., Price, N. M., and Morel, F. M. M. (2005). “Trace metal ion buffers and their use in culture studies,” in Algal Culturing Techniques, ed R. A. Andersen (Amsterdam: Elsevier), 35–63.
Suzuki, Y., Kuma, K., and Matsunaga, K. (1995). Bioavailable iron species in seawater measured by macroalga (Laminaria japonica) uptake. Mar. Biol. 123, 173–178. doi: 10.1007/bf00350337
Tsednee, M., Huang, Y.-C., Chen, Y.-R., and Yeh, K.-C. (2016). Identification of metal species by ESI-MS/MS through release of free metals from the corresponding metal-ligand complexes. Sci. Rep. 6:26785. doi: 10.1038/srep26785
Van Den Berg, C. M. G. (1984). Direct determination of sub-nanomolar levels of zinc in sea-water by cathodic stripping voltammetry. Talanta 31, 1069–1073. doi: 10.1016/0039-9140(84)80252-0
Velasquez, I., Nunn, B. L., Ibisanmi, E., Goodlett, D. R., Hunter, K. A., and Sander, S. G. (2011). Detection of hydroxamate siderophores in coastal and Sub-Antarctic waters off the South Eastern Coast of New Zealand. Mar. Chem. 126, 97–107. doi: 10.1016/j.marchem.2011.04.003
Viaroli, P., Bartoli, M., Azzoni, R., Giordani, G., Mucchino, C., Naldi, M., et al. (2005). Nutrient and iron limitation to Ulva blooms in a eutrophic coastal lagoon (Sacca di Goro, Italy). Hydrobiologia 550, 57–71. doi: 10.1007/s10750-005-4363-3
Walsh, M. J., Goodnow, S. D., Vezeau, G. E., Richter, L. V., and Ahner, B. A. (2015). Cysteine enhances bioavailability of copper to marine phytoplankton. Environ. Sci. Technol. 49, 12145–12152. doi: 10.1021/acs.est.5b02112
Waska, H., Koschinsky, A., Chancho, M. J. R., and Dittmar, T. (2015). Investigating the potential of solid-phase extraction and Fourier-transform ion cyclotron resonance mass spectrometry (FT-ICR-MS) for the isolation and identification of dissolved metal-organic complexes from natural waters. Mar. Chem. 173, 78–92. doi: 10.1016/j.marchem.2014.10.001
Wichard, T. (2015). Exploring bacteria-induced growth and morphogenesis in the green macroalga order Ulvales (Chlorophyta). Front. Plant Sci. 6:86. doi: 10.3389/fpls.2015.00086
Wichard, T., and Oertel, W. (2010). Gametogenesis and gamete release of Ulva mutabilis and Ulva lactuca (Chlorophyta): regulatory effects and chemical characterization of the “swarming inhibitor.” J. Phycol. 46, 248–259. doi: 10.1111/j.1529-8817.2010.00816.x
Wichard, T., Bellenger, J. P., Loison, A., and Kraepiel, A. M. L. (2008). Catechol siderophores control tungsten uptake and toxicity in the nitrogen-fixing bacterium Azotobacter vinelandii. Environ. Sci. Technol. 42, 2408–2413. doi: 10.1021/es702651f
Wichard, T., Charrier, B., Mineur, F., Bothwell, J. H., De Clerck, O., and Coates, J. C. (2015). The green seaweed Ulva: a model system to study morphogenesis. Front. Plant Sci. 6:72. doi: 10.3389/fpls.2015.00072
Wichard, T., Mishra, B., Myneni, S. C. B., Bellenger, J. P., and Kraepiel, A. M. L. (2009). Storage and bioavailability of molybdenum in soils increased by organic matter complexation. Nat. Geosci. 2, 625–629. doi: 10.1038/ngeo589
Keywords: dissolved organic matter, green algae, macroalgae, metal chelation, metal homeostasis, metal uptake, isotopic labeling, siderophores
Citation: Wichard T (2016) Identification of Metallophores and Organic Ligands in the Chemosphere of the Marine Macroalga Ulva (Chlorophyta) and at Land-Sea Interfaces. Front. Mar. Sci. 3:131. doi: 10.3389/fmars.2016.00131
Received: 26 March 2016; Accepted: 11 July 2016;
Published: 29 July 2016.
Edited by:
Sylvia Gertrud Sander, University of Otago, New ZealandReviewed by:
Antonio Cobelo-Garcia, Spanish National Research Council, SpainOliver Baars, Princeton University, USA
Copyright © 2016 Wichard. This is an open-access article distributed under the terms of the Creative Commons Attribution License (CC BY). The use, distribution or reproduction in other forums is permitted, provided the original author(s) or licensor are credited and that the original publication in this journal is cited, in accordance with accepted academic practice. No use, distribution or reproduction is permitted which does not comply with these terms.
*Correspondence: Thomas Wichard, thomas.wichard@uni-jena.de