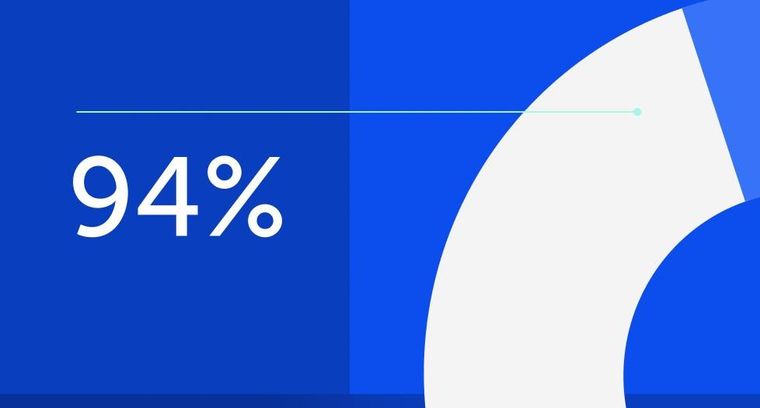
94% of researchers rate our articles as excellent or good
Learn more about the work of our research integrity team to safeguard the quality of each article we publish.
Find out more
REVIEW article
Front. Mar. Sci., 19 May 2016
Sec. Deep-Sea Environments and Ecology
Volume 3 - 2016 | https://doi.org/10.3389/fmars.2016.00072
Although initially viewed as oases within a barren deep ocean, hydrothermal vents and methane seep chemosynthetic communities are now recognized to interact with surrounding ecosystems on the sea floor and in the water column, and to affect global geochemical cycles. The importance of understanding these interactions is growing as the potential rises for disturbance of the systems from oil and gas extraction, seabed mining and bottom trawling. Here we synthesize current knowledge of the nature, extent and time and space scales of vent and seep interactions with background systems. We document an expanded footprint beyond the site of local venting or seepage with respect to elemental cycling and energy flux, habitat use, trophic interactions, and connectivity. Heat and energy are released, global biogeochemical and elemental cycles are modified, and particulates are transported widely in plumes. Hard and biotic substrates produced at vents and seeps are used by “benthic background” fauna for attachment substrata, shelter, and access to food via grazing or through position in the current, while particulates and fluid fluxes modify planktonic microbial communities. Chemosynthetic production provides nutrition to a host of benthic and planktonic heterotrophic background species through multiple horizontal and vertical transfer pathways assisted by flow, gamete release, animal movements, and succession, but these pathways remain poorly known. Shared species, genera and families indicate that ecological and evolutionary connectivity exists among vents, seeps, organic falls and background communities in the deep sea; the genetic linkages with inactive vents and seeps and background assemblages however, are practically unstudied. The waning of venting or seepage activity generates major transitions in space and time that create links to surrounding ecosystems, often with identifiable ecotones or successional stages. The nature of all these interactions is dependent on water depth, as well as regional oceanography and biodiversity. Many ecosystem services are associated with the interactions and transitions between chemosynthetic and background ecosystems, for example carbon cycling and sequestration, fisheries production, and a host of non-market and cultural services. The quantification of the sphere of influence of vents and seeps could be beneficial to better management of deep-sea environments in the face of growing industrialization.
It has been 40 years since the discovery of deep-sea chemosynthetic ecosystems fueled by fluid emissions from within the earth's crust. Hydrothermal vents hosting giant tubeworms, extensive mussel and clam beds, and dense shrimp and crab aggregations—and then cold methane seeps with related faunas—proved to be highly productive ecosystems reliant on microbes that use chemical energy (rather than light energy) to fix organic carbon (Tunnicliffe et al., 2003). Scientists now recognize that there are many hundreds of hydrothermal vents and thousands of hydrocarbon seeps in deep water (Beaulieu et al., 2013; Skarke et al., 2014; Beaulieu et al., 2015). These ecosystems occur in a large range of geological settings, from mid ocean ridges to seamounts, back-arc basins, margins and trenches, and include high-temperature flow from black smoker chimneys and diffuse flow from cracks in basalt pavement, cold brine lakes, mud volcanoes, carbonate pinnacles, and hot muds on continental margins.
Some chemosynthetic systems have already been damaged by human activities or are now targeted for exploitation (Ramirez-Llodra et al., 2011). The vulnerability of vents and seeps to human disturbance has motivated the authors to address how vents and seeps interact with surrounding ecosystems. As mining of hydrothermal vents for copper, zinc, lead, gold, and silver becomes a reality, many questions remain regarding the dynamics of these ecosystems, and what it might mean for the ocean to lose them. While less visible, the risk to the methane seep communities that colonize nearly all of the world's continental margins has escalated from increased bottom trawling activity and oil and gas drilling in deep waters. Human impacts affect both active and less known inactive chemosynthetic ecosystems, including organic falls, as well as the surrounding sea floor. Understanding how vent and seep ecosystems transition from active to inactive, and how they interact with non-chemosynthetic ecosystems is needed for environmental management and protection of these systems and services.
Ecologists have largely focused on characteristics associated with the vents and seeps themselves, often treating these environments in a binary fashion: vent and non-vent, seep and non-seep. However, the boundaries between vent and seep ecosystems and the background deep sea are not as sharp in space or time as often depicted, but rather extend through spatial and temporal transitions in environmental conditions, including transitions in concentrations of trace elements, carbon fluxes, compositions and biomass of biological assemblages, and ecological interactions and behaviors (e.g., feeding, reproduction).
Transition zones that connect different ecosystems along environmental gradients, also called ecotones, are common globally, and have been recognized on land at least since the early 1900s (Risser, 1995; Kark and Van Rensburg, 2006). Ecotones may function as critical transition zones that act as conduits for transfer of energy, water, nutrients, particles, organic matter and organisms; such transitions are exemplified at the interface of land and ocean (Levin et al., 2001). They may also function as genetic and taxonomic diversity hotspots and centers of evolutionary novelty (Kark and Van Rensburg, 2006). Transition zones among deep-sea ecosystems that have distinct environmental conditions and communities are widespread. Research on the species associated with inactive and transition habitats at vents and seeps has intensified, motivated by increased threat from extraction activities and the potential that background species interacting with vents and seeps may offer important insights into consequences of and adaptation to environmental stressors (e.g., Erickson et al., 2009; Levin et al., 2009).
In this literature review, we explore interactions between chemosynthetic ecosystems and the surrounding benthic and pelagic ecosystems, spatial and temporal characteristics of transition zones, and the role of transition zones in ecosystem function and service. Our focus is on (i) elemental cycling and energy flux, (ii) habitat use, (iii) trophic interactions, and (iv) connectivity of metapopulations and metacommunities. We draw on a deep knowledge of the literature, much of which is based on research using human- and remotely operated vehicles, camera and video observations, as well as molecular and stable isotope tools. The authors bring to the problem hundreds of years of collective experience (and hundreds of publications) developed from working at hydrothermal vents, seeps, and background communities.
Elucidation of the four-dimensional sphere of influence of chemosynthetic ecosystems will facilitate more accurate predictions of the consequences of human activities on these systems, especially as industry moves forward toward extraction of resources associated with hydrothermal vents and hydrocarbon seeps (Ramirez-Llodra et al., 2011). This knowledge will help to inform management activities such as impact assessment, valuation of ecosystem services and spatial planning, and for predicting the consequences of climate change for these systems and their services.
Vent and seep habitats, while often viewed as spatially restricted, have an expanded pelagic and benthic geochemical footprint beyond those sites that manifest on the seafloor surface. The fluxes of energy and chemicals from these sites alter local environments, and constitute important sources and sinks in global biogeochemical and heat budgets. The impact on the surrounding deep sea differs between vents and seeps as a result of the geological forces generating the efflux. As a first order distinction, seeps are the surface manifestation of the release of hydrocarbons (often dominated by methane) from the seafloor and form buoyant vertical bubble plumes in areas of flux sufficient to escape the biological filter formed by bacteria and archaea that consume methane in and on the seafloor (Reeburgh, 2007). In the subsurface, anaerobic methane oxidation leads to the generation of hydrogen sulfide, adding another reduced compound to the hydrocarbon-rich seeping fluids. In contrast, vents release a mixture of chemical compounds, the composition of which varies greatly among regions and geologic settings and often co-occurs with extremely high temperature (exceeding 350°C). These compounds include sulfide, methane, and hydrogen that fuel chemosynthetic production (Petersen et al., 2011) as well as many forms of iron, radionuclides, and mineral-rich particulates (Lilley et al., 1995). The rate of fluid flow from seeps can be highly variable, ranging from millimeters to 100 s of cm yr−1, with outliers being 3–6 m yr−1 (Torres et al., 2002; Cordes et al., 2005; de Beer et al., 2006) in contrast to flows at vents, which can be greater by eight orders of magnitude at maximum flow from active chimney structures (> 2.3 m s−1) (Tivey et al., 1995). Within any individual site, there can be both diffuse and high flow areas that together generate a wide area of benthic and pelagic influence.
Chemosynthetic primary production (carbon fixation) relies on the capture of energy from reactions that include the oxidation of sulfide and methane (at both seeps and vents) and hydrogen (at vents). These processes generate increased biomass relative to the surrounding deep sea while altering the overall cycles of S, O, and C in the oceans. At seeps, a recent review of existing data estimated that 0.02 Gt of methane-C is consumed annually in the sediment with an additional 0.02 Gt methane-C escaping annually into the hydrosphere (Boetius and Wenzhoefer, 2013). Aerobic methane oxidation, reviewed in Valentine (2011), results in a drawdown of oxygen; in soft-sediment seep systems this ranges from 4 to 1450 mmol O2 m−2 day−1 (Boetius and Wenzhoefer, 2013). Similar estimates are hard to calculate at vents because of their rocky, rather than soft-sediment structures, however degradation of the global vent primary production, which is estimated to be 1013 grams year−1 (McCollom and Shock, 1997), would also represent a significant oxygen sink in the deep sea.
Both vents and seeps transmit chemical and heat energy to the water column via plumes. At seeps, however, the vast majority of energy is trapped within the seafloor. While there is flux of energy from areas of high seepage (e.g., de Beer et al., 2006), commonly over 80% of methane is oxidized prior to its reaching the surface of the sediment (Boetius and Wenzhoefer, 2013), and sulfide concentrations are typically below detection limits above the seafloor (Cordes et al., 2005, 2006). This results in a widespread benthic footprint at seeps but a limited water column influence. Where bubble plumes rapidly transport methane through the water column, differences in the partial pressure of gasses in the water cause methane to diffuse out of the bubbles and into the water column (McGinnis et al., 2006), leaving a trail of reduced compounds in its wake. This methane is then oxidized by aerobic methanotrophs in the overlying water. Globally, up to 1175 mmol CH4 m−2 d−1 or 0.02 Gt CH4-C are estimated to be released into the water column from seeps (reviewed in Boetius and Wenzhoefer, 2013). At the scale of a single seep at Hydrate Ridge, it has been estimated that 20 Mt CH4 yr−1 is oxidized in the water column post methane emission. In addition to methane, fluxes of other sources of energy from seeps occur, such as dissolved organic carbon. Pohlman et al. (2011) estimated DOC flux to be 0.2–20.3 Tg C annually from seeps globally.
At vents, much of the fluid flux from the seafloor forms expansive neutrally buoyant plumes that disperse throughout large areas of the ocean. The fluids released from vents are chemically altered seawater, circulating from the ridge flanks through the crust near shallow magmatic intrusions at elevated temperature and pressure. These fluids contain substantial amounts of gases, including CO2, H2S, H2, and occasionally CH4, and the composition is influenced by temperature and pressure. However, a main impact of this hydrothermal cycling occurs in the crust where the source water is modified prior to expulsion at the vent proper through removal of elements including Mg, Mn, Li, Rb, and Cs (Von Damm et al., 1985), thus altering the total budget of these materials in the ocean (Edmond et al., 1979). Concurrently, other compounds are released from the crust, including iron, silica, and other metals (Alt, 2003; Tagliabue et al., 2010). The impact of these processes is large, often exceeding 100% of the global riverine flux of these compounds (Von Damm et al., 1985; Lilley et al., 1995; Resing et al., 2015). These compounds therefore provide excellent tracers for the extent of the plumes since Si, P, V, Mn, Fe, Cu, and Zn are enriched by up to a factor of 20 relative to the suspended matter of the deep ocean. Vent plumes spread out along isopycnal surfaces and, in some cases, can disperse tens to thousands of kilometers from the ridge crest where they are released (Lupton and Craig, 1981; Baker et al., 1995).
Hydrothermal vent plumes are also areas of active chemical cycling by unique bacterial and viral communities (Juniper et al., 1998; Ortmann and Suttle, 2005). Zooplankton graze directly above the plume (Burd and Thomson, 1994; Vereshchaka and Vinogradov, 1999; Cowen et al., 2001) on particulate organic carbon (POC) that is entrained in the fluid (Bennett et al., 2011). Active chemoautotrophic microbial taxa involved in the N and Mn cycles occur in the plumes themselves (Dick and Tebo, 2010; Baker et al., 2012), thus hydrothermal plumes introduce pelagic biogeochemical heterogeneity through creation of redox gradients in an otherwise homogenous environment with effects at spatial scales large enough to alter global cycles.
While the centralized areas of strong fluid flow at vents and seeps drive the pelagic footprint of venting and seeping, slower, more diffuse flow over wider areas leads to a seabed footprint that includes altered geochemistry, active carbonate precipitation, and abundant and unique fauna of all size classes. Diffuse flow areas can occupy the majority of spatial extent at vents and seeps. For example, the Haakon Mosby Mud Volcano is a structure ~1 km2 in size with the diffuse venting area covering 84% of the region (Niemann et al., 2006; Jerosch et al., 2007). Since no animal fauna is found in temperatures that remain consistently above 48°C (Girguis and Lee, 2006) and very few bacteria can survive those temperatures, the vast majority of microbially mediated production at vents (both by symbiont-bearing fauna and free-living fauna) occurs in these diffuse areas. At seeps, the anaerobic oxidation of methane (AOM) increases the alkalinity in the sediment leading to precipitation of carbonate according to the formula:
These areas of in situ precipitated hard substrate can be expansive; Builders Pencil, a methane seep located off New Zealand, covers 70,000 m2 (Baco et al., 2010), and the vast majority of the hard substrata of the entire northern Gulf of Mexico, the Hikurangi Margin in New Zealand, (Barnes et al., 2010) and the Gulf of Cadiz (Magalhães et al., 2012) consists of authigenic carbonates resulting from seepage. The rocks at vents and seeps are also active components in the cycling of elements (Schrenk et al., 2003; Marlow et al., 2014b). Both hard and soft substrates at vents and seeps host a diversity of fauna that harness the chemical energy released from the seafloor (Grassle, 1985; Van Dover, 2000; Levin, 2005; Levin et al., 2015). Conditions in areas of diffuse flow are less stressful than those in areas of focused flow, allowing the persistence of microbes and animals that capture and transfer the energy more effectively into the food web.
At both vents and seeps, complex structures are created by animals, but the most extensive and enduring substrata are large sulfide chimneys at vents and extensive pavements of carbonates at seeps. These surfaces are often colonized by “background” fauna, which use them for attachment substrata, shelter, and access to food via grazing or through position in the current (Table 1). Foundation species, whose structure and activities modify the environment at chemosynthetic habitats include siboglinid tubeworms, large bivalves and snails, the shells or tubes of which add habitat complexity that remains after the cessation of fluid flow and their death (Cordes et al., 2010). Shells may last one or two decades in deep Pacific vent habitats (Killingley et al., 1980) but over 20,000 years in the ultramafic setting of vents near the mid-Atlantic Ridge (Lartaud et al., 2010). Biogenic surfaces are particularly important for endemic epifauna around vigorous venting fluids, but are also utilized in regions of intermediate or low fluid flow (Govenar, 2010). Shells (graveyards) of large buccinid gastropods (Neptunea contraria) and bathymodiolin mussels accumulate among carbonate slabs and on mud volcano craters (e.g., Meknès and Darwin MVs, Gulf of Cadiz) and host mostly amphipods, but also other small crustaceans, polychaetes and ophiuroids (Rodrigues, 2009). In the western Pacific, dense colonies of large provannid snails (Ifremeria, Alviniconcha) cluster on chimneys and their shells accumulate at the chimney bases and host many scavengers such as buccinid gastropods, amphipods, and asteroids (Galkin, 1992). The less mineralized structures of siboglinid tubeworms have a duration of only a few years (Ravaux et al., 2003), but the complex bushes provide access to decaying organics for a transition fauna that includes a mix of endemic and deep-sea species at vents (Marcus and Tunnicliffe, 2002) and may support some distinctive species such as siphonophores (Shank et al., 1998). At seeps, heavily chitinized tubes of siboglinids (vestimentiferan Lamellibrachia, Escarpia, and Seepiophila; frenulates Lamellisabella, Spirobrachia, and monoliferan Sclerolinum) may represent rare perches above a sea of sediment for small suspension feeders such as hydroids, actinarians, and even foraminifera (Bergquist et al., 2003; Cordes et al., 2005).
Table 1. Habitats generated by chemosynthetic processes that are used by peripheral and background fauna.
Inactive chimneys at vents provide substratum that projects through the benthic boundary layer, providing suspension feeders with access to a higher volume flux of particulates. However, there have been few opportunities to observe this habitat despite the high interest in exploitation of inactive sulfide deposits. Where noted, inactive chimneys host many sessile taxa and form an assemblage dominated by sponges, cnidarians including corals, and echinoderms (Tunnicliffe et al., 1986; Galkin, 1997). To the extent that these taxa accumulate on inactive sulfides, Boschen et al. (2015) suggest that such assemblage on inactive, sulfide-rich chimneys may be distinctive. Such habitats are long-lived with ages ranging up to 100,000 years (Jamieson et al., 2014), thus forming sites of high biodiversity that can support the large corals and sponges noted by Galkin (1997).
Similarly, the carbonates that are precipitated by archaeal-bacterial consortia at methane seeps provide habitat for a broad range of chemosynthetic and heterotrophic microbes and fauna (Marlow et al., 2014a; Case et al., 2015; Levin et al., 2015). When fluid flow ceases, they may still host a few species typically found at seeps, but also attract diverse assemblages of background species including cold-water corals (Cordes et al., 2008; Bowden et al., 2013; Quattrini et al., 2015). Sedimentation rates can be high on continental margins where seeps are common, so the establishment of carbonate boulders, mounds, and pavements promotes an interaction between seep and background communities that may last for centuries or longer. In the Gulf of Cadiz, the highest species richness of gastropods is found on the upper slope (500–1200 m) where carbonate crusts and slabs are widespread. In particular, 17 species (27% of the total recorded) were found at Darwin mud volcano whose crater is completely covered by carbonate slabs (Génio et al., 2013). Carbonates near Costa Rica seeps that appear inactive host many amphipods, ophiuroids, gastropods, cnidarians, and polychaetes (Levin et al., 2015). On the New Zealand margin, old carbonates are colonized by sponges, scleractinian and antipatharian corals, and crinoids but lack seep-endemic species (Bowden et al., 2013). Authigenic carbonates found in seeps along the NE US Atlantic Margin provided substrate for octocorals and scleractinian cup corals (Quattrini et al., 2015). Carbonates and empty siboglinid tubes also provide sites for the attachment of egg capsules for both invertebrates (e.g., Neptunea, octopus) and fish (sculpin, sharks, rays, and skates; Drazen et al., 2003; Treude et al., 2011).
Active vents and seeps can alter pelagic habitats by adding particulate substrates in deep water about 100–350 m above the bottom. The hydrothermal plume above fields of high temperature venting contains notably higher biomass of bacteria than background seawater (Winn et al., 1986). As the plume ages, exopolymers increase, indicating production within the plume; these polymers bind particles and create organic-rich aggregates that provide surfaces for heterotrophic bacteria and feeding copepods (Shackelford and Cowen, 2006).
In some scenarios, significant changes in the microbial community have been demonstrated throughout the water column overlying an active seep, including in the photic zone. These include elevated abundances of methane oxidizing microbes, but also elevated abundance of Prochlorococcus phylotypes (Rakowski et al., 2015). Although many of these changes remain unexplained, these findings offer a first order approximation of the great distance of influence; the rapid rise of the bubbles may enhance localized upwelling and relieve nutrient limitation at the surface, particularly in oligotrophic regions.
It is estimated that 3% of the total flux of organic carbon to the deep seabed is derived from chemoautotrophic production at hydrothermal vents (Jannasch, 1995; McCollom and Shock, 1997; Van Dover, 2000). If we assume global seep primary production is at least double that of vents (given that there is likely an order of magnitude more seeps than vents), then the total contribution of chemoautotrophic production to the deep sea may exceed 10% of the total flux of organic carbon. This is likely a minimum value because improved detection methods using Eh combined with backscatter sensors suggest that vent sites are likely two to six times more abundant than currently documented (Baker et al., 2015). This chemoautotrophic production is not uniformly distributed throughout the deep ocean, but instead is likely distributed along exponentially decreasing, three-dimensional export gradients from the center of a chemoautotrophic ecosystem. On continental margins, productivity of seeps has been postulated to be an important contributor to local fisheries productivity (Levy and Lee, 1988), and a commercial crab species (Chaceon sp.) has been observed feeding on mussels at the Menez Gwen vents close to the Azores (Colaço et al., 2002). Seep productivity has also been implicated as a source of carbon for pelagic bacterial assemblages in the Gulf of Mexico (Kelley et al., 1998).
Export of chemoautotrophic production can follow multiple pathways, including benthopelagic coupling through advection of particulate organic carbon, trophic transfer by predators and scavengers, and release of gametes into the water column. Vertical, benthopelagic coupling is evident on local scales at hydrothermal vents, where, for example, amphipod swarms occur above diffuse flow zones at vents on the East Pacific Rise, presumably feeding on chemoautotrophic bacteria (Kaartvedt et al., 1994). Elevated zooplankton biomass and abundance (including meroplankton) in near-field plankton samples has also been attributed to vent productivity (Wiebe et al., 1988; Burd and Thomson, 1994; Mullineaux et al., 1995). Buoyant and neutrally buoyant hydrothermal plumes are advective agents of chemoautotrophic production from vents (Dick et al., 2013). Transfer and fate of microbes and reducing compounds into the water column above chemoautotrophic ecosystems is an area of intensive research, stimulated by new sampling capabilities that permit high spatial resolution of microbial community characteristics (Sheik et al., 2014).
Epifaunal species peripheral to chemoautotrophic ecosystems benefit also from horizontal advection of particulate organic material from vents and seeps. For example, bamboo corals and other non-vent organisms with sulfur isotopic compositions consistent with a diet of particulate organic material derived from microbial chemoautotrophy were found near vents in Manus Basin (Erickson et al., 2009), and dense assemblages of suspension-feeding and microcarnivorous epifauna adjacent to symbiont-hosting invertebrates have also been inferred or documented to rely on chemoautotrophically derived particulate organic matter. Examples include serpulid worms and anemones at Galapagos vents (Hessler et al., 1988), serpulid worms Neovermilia sp. at warm seeps near Barbados, Peru and Costa Rica (Sibuet and Olu, 1998; Levin et al., 2012), and the octocoral Callogorgia delta with increased abundance near seeps in the northern Gulf of Mexico (Quattrini et al., 2013).
Vertical connectivity between vent systems and the pelagic environment has been demonstrated first through chemical fluxes, and later the microbial flux and the associated zooplankton. Recently, input of photosynthetically produced carbon to the diet of vent animals has also been shown. Through analysis of fatty acid biomarkers as proxy of microalgae production it was shown by Colaço et al. (2009), that the vent mussel from the Menez Gwen vent field at the Azores triple junction profits from the particulate matter. Diatom skeletons were also found in their guts, proving that they were ingesting those organisms (Riou et al., 2010). Fatty acids, carotene pigments and stable isotopes also showed that R. exoculata juveniles have reserves of organic matter with a photosynthetic origin (Pond et al., 1997; Copley et al., 1998; Gebruk et al., 2000). In the western Pacific Ocean, evidence of photosynthetic input into the diet of vent-obligate invertebrates occurs at the relatively shallow vents on East Diamante (Stevens et al., 2015).
Heterotrophic infauna, which can be very abundant at and near seeps and some vents globally, often derive their nutrition from mixotrophy—a mix of chemosynthetically and photosynthetically derived organic matter in sediments or overlying waters (Levin and Michener, 2002; Levin et al., 2009, 2010; Demopoulos et al., 2010; Pape et al., 2011; Thurber et al., 2012, 2013; Rodrigues et al., 2013). Mixotrophy enables some seep species to endure variations in hydrocarbon-rich fluids, to persist over time under decaying activity or to occupy more peripheral areas at seeps. This nutritional pattern also has been documented for many polychaete families (e.g., maldanids, nereids, ampharetids, nephtyids, onuphids), amphipods, tanaids, cnidarians, and nematodes, with increased use of photosynthetically-derived carbon in transitional sediments with diffuse flow or less active seepage. The depleted δ13C values of nematodes sampled at increasing distance from a local seepage site at the Darwin Mud volcano, Gulf of Cadiz, suggested that thiotrophic C is part of the nematode diet at least up to 10 m away from the seep site where no signs of AOM were measured in the sediments (Pape et al., 2011). At seeps on the northern California shelf (30–50 m), use of photosynthetic carbon by infauna is the norm (Levin et al., 2000); only 1 in 14 species exhibited isotope signatures reflecting methane-derived carbon. Such systems represent one end member in a gradient of trophic interactions between chemosynthetic and photosynthetically fueled ecosystems. At bathyal seeps in the NE Pacific where 25–50% of seep infauna also occur in background (non-seep) sediments (Levin, 2003; Levin et al., 2010), the combined use of photosynthetic and chemosynthetic nutritional sources and likely exchange of larvae among populations suggests a combination of energy, carbon, biomass and genetic flows between background and seep habitats. The consumption of the infauna by mobile predators such as galatheid and lithodid crabs strengthens these interactions (Demopoulos et al., 2010; Niemann et al., 2013).
Trophic transfer of chemoautotrophically derived production from vents and seeps by vagrant predators and scavengers is well documented but largely unquantified. Typical marauding predators that move back and forth between chemoautotrophic and non-chemoautotrophic environments include crabs, fish, seastars, and octopus (Tunnicliffe and Jensen, 1987; Marques and Porteiro, 2000; Voight, 2000; MacAvoy et al., 2002, 2003, 2008). At Gulf of Mexico seeps, predators such as galatheid crabs collected up to 2 km distant from a known seep derived 12 to 40% of their carbon from seep productivity (MacAvoy et al., 2008). However, other predatory taxa near seeps in the Gulf of Mexico, including hagfish (Eptatretus) and giant isopods (Bathynomous giganteus), were shown to derive the majority of their nutrition from photosynthetic material (MacAvoy et al., 2002, 2003, 2008). The lithodid crab, Paralomis sp., is generally considered to be a predator, but at an active mud volcano of the Costa Rica subduction zone, a combination of behavioral observations, stable isotope analyses, and genetic analyses of gut contents indicates it may also function as a grazer of thiotrophic microbial mats (Niemann et al., 2013). The role of predators in transfer of chemoautotrophic carbon to the surrounding seabed may decrease with depth, as observed at seeps in the Sea of Okhotsk where predator (crab and sea star) abundance decreased dramatically with depth from 160 to 1600 m. In addition to the transfer of chemoautotrophic production to the surrounding deep sea, predators contribute to variations in community structure in chemoautotrophic ecosystems through consumption and through indirect effects (e.g., modification of habitat quality Micheli et al., 2002). In some circumstances, endemic vent taxa themselves can be transfer agents for chemoautotrophic carbon to the surrounding deep sea. For example, brooding vent crabs Kiwa tyleri move to the periphery of the vent field (Marsh et al., 2015), although the extent to which they contribute to carbon transfer is unknown.
Release of gametes may represent an important, yet unquantified source of export of particulate carbon from chemosynthetic ecosystems, given the high fecundity of biomass-dominant endemic taxa with dispersive larval stages (Tyler and Young, 1999). Some of the best data on reproductive output comes from in situ observations of seep vesicomyid clams in Sagami Bay, where the clams release 5.8 x 108 eggs m−2 yr−1 (Fujikura et al., 2007). Gametes and developing larvae could serve as food for a variety of pelagic organisms and, given evidence that larvae of vent (Pond et al., 1997) and seep (Arellano et al., 2014) organisms may be found in the euphotic zone, the vertical transfer of organic material from chemoautotrophic ecosystems can be on the order of a kilometer or more. Horizontal export of chemoautotrophic production via dispersive larvae can be even more extensive, potentially hundreds of km (Adams et al., 2012; Young et al., 2012; Hilário et al., 2015).
Transfer of chemoautotrophic production to surrounding deep-sea ecosystems may also increase during waning of hydrothermal or seep activity through increased ability of organisms to invade as environmental gradients weaken (Marcus and Tunnicliffe, 2002; Van Dover, 2002; Bergquist et al., 2003). Carbonate outcrops and their attendant autotrophic microbial communities may also serve as conduits for carbon export to the surrounding deep-sea organisms (Levin et al., 2015).
Chemosynthetic ecosystems clearly export organic material to the surrounding environment through multiple transfer pathways, but fluxes of these materials and relative strengths of the pathways are, for the most part, poorly quantified, as are the circumstances and processes that control these fluxes. The environmental acquisition of free-living stages of microbes as symbionts (as in siboglinids) represents another potential area of interaction. These topics of research are ripe for innovative ecosystem approaches and critical to understanding the role of chemoautotrophic ecosystems in deep-sea services.
The degree of exchange of individuals among populations, habitats or ecosystems is termed connectivity, and depending on the life-history characteristics of a species can involve the dispersal of larval propagules and recruits, and migration of adults. Connectivity, in turn, influences the dynamics, spatial distribution and persistence, particularly of spatially fragmented populations, as well their genetic structure and evolution. Dispersal is difficult to quantify in even the shallowest marine environments (e.g., Cowen and Sponaugle, 2009); in the deep sea estimates of dispersal have to be inferred using indirect methods; options include biophysical models to predict larval dispersal trajectories by combining ocean circulation models with biological parameters (e.g., Metaxas and Saunders, 2009), and inference of dispersal from measures of population genetic structure (e.g., Kinlan and Gaines, 2003).
Dispersal trajectories have been predicted using biophysical models for four species associated with cold seeps in the Gulf of Mexico: the siboglinid tubeworm Lamellibrachia luymesi, the bivalve Bathymodiolus childressi, the gastropod Bathynerita naticoidea, and the asteroid Sclerasterias tanneri (Young et al., 2012). The dispersal distance of these species ranged between ~ 100 and 1100 km, and varied with pelagic larval duration (PLD) and depth in the water column, with greater potential dispersal distances at shallower depths. These distances suggest that dispersal of individual larvae across seep locations as well as to non-chemosynthetic habitats can occur within their lifetime.
For hydrothermal vents on ocean ridge crests, an additional challenge to generating realistic and accurate models is that the circulation is altered by the complex topography created by vents (Thomson et al., 2003). Use of progressive vector models based on Eulerian current measurements from moored current meters in combination with PLDs suggest that larval Riftia pachyptila tubeworms could potentially disperse as far as ~ 100 km over their lifetime, placing distant vent fields as well as non-chemosynthetic habitats within reach (Marsh et al., 2015). Simulations by McGillicuddy et al. (2010) also suggest that larvae with a 30 day PLD can be transported >100 km along a ridge crest, but less than 20 km away from it. Patterns of dispersal varied with time of year and the height above the bottom at which the larvae were released.
Employing a population genetic approach, Baco et al. (in press) reviewed 51 studies and derived dispersal estimates from 42 deep-sea species (15 species from chemosynthetic ecosystems, 11 non-chemosynthetic ecosystem invertebrates, and 16 fishes). The range of dispersal estimates obtained ranged from 0.24 to 2028 km (geometric mean = 33 km, median 34 km), with fishes being much more dispersive than chemosynthetic or non-chemosynthetic invertebrates. Deep-sea dispersal estimates were comparable to or slightly greater than the dispersal estimates for shallow-water species.
The estimates of dispersal distance both from biophysical modeling and population genetic approaches exceed the diameter of the average chemosynthetic site, implying that propagules from these ecosystems reach non-chemosynthetic habitats, providing the potential for connectivity of chemosynthetic ecosystems to transition zones and into background environments. A key intermediary habitat for the connectivity of chemosynthetic ecosystems to background communities on both ecological and evolutionary time scales may be organic remains. Numerous species, genera, and families of invertebrates have been found to be shared across reducing environments (e.g., Tunnicliffe et al., 1998; Bernardino et al., 2012; Vrijenhoek, 2013), including at vents associated with mid-ocean ridges, back-arc spreading centers, seamounts, and serpentinite systems, at seeps associated in various settings (petroleum seeps, mud volcanoes, salt diapirs, brine pools, methane hydrates), and sometimes at whale falls, wood falls, and other organic deposits. Whale falls were first proposed to act as stepping stones for the dispersal of a vent and seep species based on shared morphotypes (Smith et al., 1989) and later confirmed for a few species with molecular analyses (Feldman et al., 1998; Baco et al., 1999). The distribution of whale falls is thought to be much wider than that of vents and seeps, and may be common enough on the seafloor to allow for dispersal (Smith, 2006). Vesicomyid clams can have broad (e.g., trans-Pacific) distributions consistent with their use of organic drift deposits of multiple types, which are found with relatively high frequency along continental margins (Audzijonyte et al., 2012). Among the macrofauna and meiofauna, it is likely that some polychaete, gastropod and nematode species overlap occurs among chemoautotrophic habitat types and with the surrounding seabed ecosystems (Pape et al., 2011; Cunha et al., 2013a; Hilário et al., 2015), but these groups have received less attention than the megafauna of vents, seeps, and whalefalls.
Key to the ecological connectivity of whale falls and various organic remains with other chemoautotrophic ecosystems is the slow, sustained breakdown of organic material over large time periods leading to the development of a reducing habitat with measureable concentrations of hydrogen sulfide, thus providing suitable habitat for sulphophilic species (Smith and Baco, 2003). For example, siboglinid tube worms and bathymodiolin mussels have been observed on sunken shipwrecks, with the same species also observed on nearby cold seeps (Dando et al., 1992; Hughes and Crawford, 2008; Southward et al., 2011). In total, at least 21 taxa are known to occur on sunken wood that also occur at either vents or seeps (Metaxas and Kelly, 2010). Kelp holdfasts and other plant materials also produce ephemeral reducing conditions, and harbor at least 7 taxa that occur at vent and/or seeps (Metaxas and Kelly, 2010). However, sampling of whale falls, sunken wood, kelp and other types of organic remains still remain sparse and existing samples come from carcasses or wood panels experimentally implanted and sampled over relatively short time periods (< 3 years). Community composition shifts with succession in these habitat types (Smith and Baco, 2003) and overlapping species may only occur during the brief window of one successional stage, allowing them to be easily missed during limited sampling opportunities. Notably, among the reviewed papers, there is not a single paper that looks specifically at dispersal of chemosynthetic ecosystem species to non-chemosynthetic ecosystems or vice versa.
Shared genera and families across reducing ecosystems indicate connectivity on an evolutionary scale among different reducing ecosystems. Biogeographic and evolutionary relationships among metazoan taxa of chemoautotrophic ecosystems have been reviewed descriptively (Tunnicliffe et al., 1998) and phylogenetically (Vrijenhoek, 2013). Most of the dominant taxa of chemoautotrophic ecosystems are of relatively recent origin, dating from repopulation events following global deepwater anoxic events (i.e., < 65 Myr old) (Vrijenhoek, 2013; Yang et al., 2013). Dominant symbiont-hosting invertebrates at hydrothermal vents arose from continental margin cold-seep (or wood-fall) ancestors, possibly multiple times [siboglinid tubeworms (McMullin et al., 2003; Hilário et al., 2011; Li et al., 2015); vesicomyid clams (Peek et al., 1997; Baco et al., 1999; Decker et al., 2012); mytilid (bathymodolin) mussels (Distel et al., 2000; Jones et al., 2006; Thubaut et al., 2013); decapods (Yang et al., 2013); yeti crabs (Roterman et al., 2013)]. Cross-ecosystem evolutionary connectivity in other dominant megafaunal groups such as Alviniconcha snails (Johnson et al., 2015b) have yet to be found.
Macrofaunal taxa characteristic of chemoautotrophic ecosystems also show evolutionary connectivity, although the final patterns are not always resolved. For example, vent polychaete species in the genus Archinome form a clade that is sister to the single known seep species, or the seep species was derived from vent-dwelling ancestors (Borda et al., 2013). For Amphisamytha, the single seep-dwelling species was found to be a sister group to a Mid-Atlantic Ridge vent species, and there was a complex relationship among the various Pacific vent-dwelling species (Stiller et al., 2013). Other studies on macrofaunal taxa, such as limpets (Johnson et al., 2006, 2008) and dorvilleid polychaetes (Thornhill et al., 2012; Wiklund et al., 2012) have revealed cryptic species, but broader scale sampling and analysis are required to interpret cross-ecosystem ecological and evolutionary connectivity.
The use of DNA barcoding can be a critical tool for establishing the degree of species overlap among larger-bodied taxa between habitat types as well as verifying the presence of chemosynthetic ecosystem species in non-reducing habitats (e.g., Baco et al., 1999). For smaller-bodied meiofauna, emerging tools such as metabarcoding will allow sediment-dwelling meiofauna to be screened among habitat types for a more rapid assessment of species overlap (e.g., Fonseca et al., 2010). Nematodes and Foraminifera, which exhibit limited evolutionary specialization at vents and seeps at the genus level may be good candidates for high connectivity with background systems (Van Gaever et al., 2009). However, recent phylogenetic analysis for Halomonhystera disjuncta, a nematode species reported from both shallow-water habitats and a deep-water cold seep (Van Gaever et al., 2006) showed that the deep-sea populations comprised an endemic lineage compared to the shallow-water relatives (Van Campenhout et al., 2014).
Similarly for microbes, next generation sequencing of SSU rRNA genes allows for screening of species overlap and metagenomics allows for screening of overlap in functional genes among the microbes of various habitat types (e.g., Huber et al., 2007; Jansson, 2013). Once overlap in taxa among habitat types is established, population genomics methods will allow levels of ecological and evolutionary connectivity between vents, seeps, and other ecosystems to be determined.
Clines in physical environmental conditions from point source to background are associated with ecotones along which dominance shifts from chemoautotrophic species close to the source to background heterotrophic species at some distance away from it. Clines at both vents and seeps are three-dimensional, with chemical and temperature gradients extending across and into the seabed and upwards through the water column. While the seabed extent of chemosynthetic faunas and their spatial zonation have been studied for both vents and seeps, at least for epifauna and bacterial mats visible in camera transects, much less is known about the area of influence on infaunal and water column ecosystems.
Fluids rich in methane and dissolved metals from seeps and vents, respectively, disperse vertically up to ~500 m above the seabed and similar distances laterally in the water column as buoyant or neutrally buoyant plumes (Baker et al., 1995; Skebo et al., 2006; Law et al., 2010), and thus have potential effects on biological processes in the water column distant from their seabed sources (Skebo et al., 2006). Elevated biomass of bacterioplankton occurs throughout the water column above sites of shallow (< ca. 200 m depth) seep, hydrothermal, and volcanic activity in the southwest Pacific (Sorokin et al., 1998), and zooplankton abundances in the first several meters above vent sites in the Gulf of California can be approximately four times higher than at background sites (Berg and Vandover, 1987). Non-vent holoplankton are found in high concentrations immediately above vent openings in the western and northeast Pacific (Metaxas, 2004, 2011). Increased concentrations of crustaceans and gelatinous zooplankton have been recorded in a layer 300 m above hydrothermal vents at Endeavour Seamount (Burd and Thomson, 1994, 1995) but distributions of zooplankton and nekton at 20 m above the seabed at this site probably result from taxon-specific aggregation in response to water column gradients, rather than biomass enhancement (Skebo et al., 2006).
Studies of cold seeps at ~1000 m depth in the Gulf of Mexico suggest that planktonic microbial communities can be influenced by seep-derived methane from the seabed upwards into the euphotic zone (Rakowski et al., 2015). On the Hikurangi Margin, New Zealand, persistent methane flares imaged by ship-based echosounders extend from the seabed at ~1000 m depth up to an apparent ceiling at ~500 m depth (Faure et al., 2010; Greinert et al., 2010) and are advected laterally below this depth (Law et al., 2010). Law et al. (2010) conclude that seeps on this margin have a negligible impact on ambient water column nutrient, particulate, and sediment biogeochemistry but are a potential source of bacteria, including methanotrophs, to the water column. Likewise, similar plumes have been detected along the US Atlantic (~180–1700 m) and Cascadia Margins, with plume heights emanating >100 m above bottom (Skarke et al., 2014; Johnson et al., 2015a). Approximately 440 seeps were mapped along a 950 km track from Cape Hatteras to Georges Bank. While the degree of lateral advection of this methane remains unknown, these seeps could serve as a significant, yet unquantified source of seabed methane (Skarke et al., 2014), fueling microbial communities both in the water column and seafloor over large spatial areas.
At the seabed, perceptions of ecotone extent are dependent on faunal size spectra. At seeps (Sahling et al., 2002; Van Dover et al., 2003; Fischer et al., 2012; Bowden et al., 2013; Grupe et al., 2015) and vents (Podowski et al., 2009), studies of epifauna visible in video surveys show habitat patches generally of small spatial extent, typically 1–100 m scale, with hard boundaries and often with conspicuous zonation of the dominant taxa (Barry et al., 1997; Olu-LeRoy et al., 2004). However, sites may consist of many such patches in close proximity and at differing successional stages, such that the overall site extends 1 km or more on its longest axis (Jones et al., 2010; Bowden et al., 2013; Wagner et al., 2013). Vent-associated mobile mega-epifauna may be the first organisms visible around vents and have been used to predict the presence of vents at scales of up to 500 m (Van Dover et al., 1987). At seeps, accumulations of seep-associated bivalve shells may extend over much greater areas than the live fauna (Olu-LeRoy et al., 2004; Baco et al., 2010). Macro-infauna (>300 μm) show broader and more diffuse boundaries (Menot et al., 2010). For meiofauna, changes in composition along the seep transition zone differ from very abrupt (Van Gaever et al., 2006, 2009) to rather gradual with only changes in relative proportions of taxa, depending on the seep characteristics such as seepage intensity (Zeppilli et al., 2012), size of the seep (Pape et al., 2011) and regional context (Guilini et al., 2012). There is also evidence that ecotones associated with seeps decrease in size with decreasing depth, which may be a consequence of the increased availability of photosynthetically vs. chemosynthetically derived production (Sahling et al., 2003).
The many studies that have measured and examined the factors that regulate settlement and colonization at hydrothermal vents and at cold seeps (e.g., Shea et al., 2008; Metaxas and Kelly, 2010; Arellano and Young, 2011; Cunha et al., 2013a,b; Génio et al., 2013) reveal species-specific habitat requirements for settlement and colonization (i.e., survival post-settlement). Such requirements are related to the chemical composition of the fluids and/or biotic factors, such as successional stage, or the presence of other species that determine suitability of different chemosynthetic habitats. Alternatively, the transition zones between the reducing environment and the background deep sea may impact the settlement of larvae. Along transition zones, neither settlement nor colonization have been measured systematically. It is clear that transitional habitats may have higher local diversity by blending taxa endemic to reducing habitats and those that are tolerant to the stressors that occur there (Cordes et al., 2009; Bernardino et al., 2012; Cunha et al., 2013b; Levin et al., 2015). Yet to be determined are the spatial and temporal scales at which these differences become evident, or whether the proximity to other types of hardgrounds affects the importance of seep carbonates as hard substrate habitat.
The transitions between chemosynthetic habitats and the background of the deep sea occur in time as well as space, and are particularly well studied at vents (Table 2). The transition is often governed by changes in the fluxes and geochemistry of fluids and accompanying shifts in the properties of foundation species (e.g., Figure 6 in Bernardino et al., 2012). When chemosynthetic habitats first appear, released fluids are typically anoxic and rich in hydrogen sulfide, which is toxic to the majority of species that dwell in background sediments (Somero et al., 1989). In fact, large explosive releases of fluids and gases associated with volcanic events can cause mortality of nekton above the site, and dead and dying animals can be consumed by vent inhabitants (Staudigel et al., 2006; Tunnicliffe et al., 2013). Emergent fluid also brings microbes flushed from subsurface habitats, and the broad deposition of microbial organics released in some events (Meyer et al., 2013) may benefit background deposit or filter feeders. In these early stages, the transition zone is usually sharp and narrow, both vertically and horizontally, with strong gradients in temperature and/or chemical concentration relative to the background setting (Govenar et al., 2005; Cordes et al., 2006). In the Juan de Fuca vent system, the colonist fauna at vigorous flows on chimneys consists primarily of alvinellid polychaetes, with Ridgeia picesae tubeworms coming in afterwards (Sarrazin et al., 1997), while alvinellid colonization at new vents on lavas depends upon prior tubeworm settlement (Marcus et al., 2009).
Over time, the flux of fluids to the seafloor and the overlying water column declines as minerals and/or authigenic carbonate precipitate in the subsurface thus occluding fluid pathways and altering fluid composition. In vent systems, this process occurs on the order of one to a few decades (Desbruyères, 1998; Shank et al., 1998), although shorter time-scale variations may occur and the successional sequences may revert with alterations in fluid flow through porous chimney structures (Cuvelier et al., 2011). On longer time scales, magmatic influences can recede, reducing fluid circulation (e.g., Butterfield et al., 1997). In addition, the presence of large quantities of iron in some hydrothermal systems results in the sequestration of dissolved sulfide (Govenar et al., 2005). These processes may diminish the sphere of influence of the individual site on the surrounding deep-sea ecosystem, but they also create more hospitable conditions for background macrofaunal species (Desbruyères et al., 2001). In tubeworm assemblages at Pacific hydrothermal vents, fluid flux and tubeworm architecture influence access to background species (Shank et al., 1998; Marcus and Tunnicliffe, 2002). Senescent vents appear about as diverse as active vents on Juan de Fuca Ridge, however, species distributions are more even and include typical deep-sea taxa such as spider crabs, isopods, tanaids, ophiuroids, hydrozoans, and sponges (Tsurumi and Tunnicliffe, 2003) (Figure 1).
Methane seeps exhibit similar successional changes in structure and faunal assemblages over extended time periods, although changes can also occur abruptly (Table 3). At the hydrocarbon seeps of the Gulf of Mexico, the first fauna inhabiting hard substrata are the bathymodiolin mussels (Cordes et al., 2009) that require reduced chemicals at the seafloor (Childress and Fisher, 1992). In the Hikurangi Margin in New Zealand, ampharetid polychaete beds are associated with the highest flux zones, and facilitate the settlement of later successional stages (Bowden et al., 2013). These early-stage biogenic habitats are dominated by endemic species, while in most circumstances the background deep-sea fauna is relegated to the periphery of the more active habitats (Sarrazin et al., 1997; Bergquist et al., 2003; Bowden et al., 2013), although mobile fauna such as crabs and octopus and less-mobile filter feeders may appear during early successional stages.
In the Gulf of Mexico seeps, successional changes are documented over decades to centuries for assemblages associated with tubeworms (Lamellibrachia luymesi and Seepiophilia jonesi). These large biogenic structures attract increasing numbers of background grazers and, eventually, predators as fluid flux declines and they grow upwards and away from sources of sulfide and methane (Bergquist et al., 2003; Cordes et al., 2005). As sulfide levels decline, bacterial biomass, endemic species and productivity all decline but the representation of mobile crustaceans, hagfish, eels and overall biodiversity increases, potentially persisting for hundreds of years (Cordes et al., 2006, 2009). A similar successional sequence has also been described from the Hikurangi Margin off the coast of New Zealand, with mussels and clams giving way to tubeworm aggregations that may persist for centuries (Bowden et al., 2013). On the NE Pacific margin, seep sediments at 500 and 800 m exhibit a gradual decline in macrofaunal endemicity with decreasing fluid fluxes. Highly sulfidic microbial mats support few background species; better-oxygenated, less sulfidic vesicomyid clam beds share only 45–51% of microbial mat species, but many species with near-seep sediments that appear inactive (80% off CA and 96% off OR). More distant sites at similar depths shared fewer but still substantial numbers of species with seep clam-bed sites (70% at 500 m off CA; 35% in the oxygen minimum zone [OMZ] off OR). Because of the mixing of background species and seep endemics, alpha diversity tended to be highest in clam bed or near-seep sediments (Levin et al., 2010).
As the flux of fluids continues to diminish, the productivity of the sites decline and faunal composition changes. A significant process at this stage is the export of productivity by the large, mobile fauna that may now enter the site due to the amelioration of toxicity and increased oxygen availability (MacAvoy et al., 2003). When fluid flux ceases entirely, the sites become inactive and are often only identified through shell hash and the persistence of authigenic carbonates (Vanreusel et al., 2009). However, microbial activity may persist for long periods within the authigenic precipitates even at sites that appear to be visually inactive (Génio et al., 2008; Marlow et al., 2014a). Finally, the transition zone is reduced to the effects of substrate provision as described above. During these phases the fauna may become highly diverse, with many background species present in surrounding sediments.
Many vent and seep sites occur from shallow to bathyal depths; some vents are very shallow on arc volcanoes while seeps can occur at abyssal to hadal depths in subduction trenches. The impact of chemosynthetic sites on the surrounding communities is a function of water depth (affecting export of surface productivity), the regional oceanography (primarily in terms of the amount of surface productivity), water mass influence (in terms of connectivity), and regional biodiversity. Because specialist, chemosynthetic habitat-endemic faunas are rare at very shallow (< 200 m) vents and seeps, there is a general assumption that the contribution of chemosynthetic production is less important in productive, shallow settings.
The biogeography of vent sites has been compiled by Tunnicliffe et al. (1998); Van Dover et al. (2002) and Bachraty et al. (2009), and these studies describe very different dominant taxa in different regions. In contrast, the provincial biogeography of the global bathyal benthic fauna is still not well known, however, a scheme delineating benthic provinces has been proposed (UNESCO, 2009; Watling et al., 2013). While the biogeography of vent communities seems to parallel that of the bathyal communities on ridges, it is likely that both the vent and surrounding bathyal communities are products of their individual, but shared, geological histories (Watling et al., 2013). Thus, vent communities in the Atlantic, with the exception of the vent fields close to the Azores archipelago, are dominated by shrimp harboring commensal bacteria on their gills while similar sites in the East Pacific are characterized by tubeworms and bivalves also hosting symbiotic chemosynthetic bacteria. The shallowest vents close to the Azores are dominated by the vent mussel B. azoricus, which hosts a dual symbiosis in their gills, but also assimilates photosynthetically-derived carbon (Colaço et al., 2009; Riou et al., 2010). In addition to mussels, vents in the W. Pacific and Indian Ocean support large symbiont-bearing snails. The degree to which the vent communities from different areas impact the surrounding bathyal benthos will likely be a function of the composition of the vent community, the composition of the surrounding bathyal community, and the depth-related productivity.
Chemosynthetic communities, as we have seen, rely on symbiotic or free-living chemosynthetic bacteria for their productivity. The surrounding bathyal communities, however, are dependent on settling organic particles from the overlying water column. Since bathyal communities underlie both highly productive as well as oligotrophic waters, depending on their location, their biodiversity and productivity will correspondingly vary. In oligotrophic regions or deeper waters, organic matter contributions from vent communities may result in enhanced benthic animal density and productivity in the surrounding area. In areas where there are high levels of water column organic particulate input, such as at high latitudes, the additional input from vent communities might have less influence on the surrounding benthos. Eckelbarger and Watling (1995) showed that the ability of species to reproduce rapidly in response to sudden inputs of organic matter was determined by the phylogenetic history of the species. Animals that were related to opportunistic species in shallow water most likely would have the metabolic capability of converting ingested organic matter to eggs in a relatively short time period, whereas species without that capability would be unable to take advantage of the increased input. Under oligotrophic waters, however, the additional input of organic matter to the sediments from chemosynthetic communities could provide the boost needed to locally enhance animal density by allowing successful recruitment of larvae.
In some areas, mesoscale eddies that pass over vents can result in episodic pulses of food to vents while exporting the produced carbon and larvae away from the vent areas. Surface generated mesoscale eddies were observed to influence the transport of hydrothermal vent efflux and vent larvae away from the North East Pacific Rise (Adams et al., 2011). The eddies that reach the deep sea provide a mechanism to disperse propagules hundreds of kilometers away from the ridge crest. Mesoscale and submesoscale vortices at depths ranging from 0 to 1000 m are frequent (Ciani et al., 2014). In this case, vent fields at bathyal depths can have larger influence on the surrounding environments. Similar phenomena have not been studied at seeps.
Overlying hydrography may also influence the extent to which seep and background communities share species or interact. In the Gulf of Cadiz, there is a transition at 1200 m depth that coincides with changes in the oceanographic, geologic and biogeochemical settings. This depth also separates shallower mud volcanoes where background fauna are more prevalent and deeper mud volcanoes that exhibit a much higher degree of endemism (Cunha et al., 2013b). At bathyal depths of 500–800 m in the NE Pacific, a well-developed OMZ creates a stress-tolerant background community; there, the macrofauna and meiofauna exhibit significant overlap between seep and non-seep sediments (Levin et al., 2010; Guilini et al., 2012). The fauna on transition substrates (inactive carbonates) along the Costa Rica margin also reflect the influence of overlying oxygenation, whereas those on carbonates at active seepage sites do not (Levin et al., 2015).
Extraction of living and non-living resources and waste disposal in the deep sea, as well as inadvertent pollutant and debris inputs have the potential to influence the interactions and transitions described above (See Box 1). Those activities with known impacts on chemosynthetic ecosystems and their surroundings are bottom trawl fisheries, mining and oil and gas extraction (Baker et al., 2010). The increasing number of scientific sampling expeditions and potential alteration of these ecosystems by scientific work must also be considered (Godet et al., 2011). For example, the InterRidge data base reports 874 cruises between 1976 and 2012. We know of no such compilation for seeps. Scientific activity at hydrothermal vents is regulated by a voluntary code of conduct, the Inter-Ridge “Statement of commitment to responsible research practices at deep-sea hydrothermal vents” (InterRidge, 2006). While scientific activities must comply with national laws, there are no binding laws in areas beyond national jurisdiction.
Box 1. Human Influence on Chemosynthetic Ecotones and Surrounding Ecosystems.
Bottom Trawling: Causes physical disturbance and removal of 3D biogenic structures on margins and seamounts. Widespread interference with seeps is likely, affecting habitat provision, trophic subsidies and connectivity. Less clear influence on vents.
Oil, gas or hydrate exploitation: Physical disturbance from structures, anchors, noise, cuttings, and leakage. Most likely to involve seeps; interaction with vents is limited. Possible strong influence on elemental fluxes, minor influence on habitat provision, trophic interactions, and connectivity. May disturb background community in ways that affect interactions with chemosynthetic systems.
Seabed mining: Mining of seafloor massive sulfides or gas hydrates will involve removal of substrate, plumes, noise, and associated contaminants. This may have the greatest impact on inactive vents and seeps, transition habitats and adjacent background communities
Whaling and logging: May reduce availability of organic falls, which serve as habitat or stepping stones for species reliant on chemosynthetic energy sources.
Scientific research: Sample collection, instrumentation, ship, sub, ROV disruption occur with minor effects on fluxes, habitat, trophic exchange and connectivity.
Waste disposal: Munitions dumping, sewage, debris and litter is common on continental margins and may be most likely to affect seeps. Impacts are likely to be minor; organic debris and sunken ships with organic cargo may provide new habitat or stepping stones.
Pollution: Pervasive contamination will reach all ecosystems near human sources, but no studies have examined effects on vent or seep species.
Climate change: Warming may dissociate gas hydrates. Ocean acidification and ocean deoxygenation are likely on margins, affecting bathyal seeps. Vent, seep, and transition zone species may be more tolerant of some environmental change.
As resource exploitation increases in the deep sea (Box 1), there is growing interest in understanding the ecosystem functions and services that may be vulnerable to resulting disturbance (Armstrong et al., 2012; Thurber et al., 2014). Rarely, however, is the focus on the services linked to the exchange of energy, elements, individuals, and biomass between deep-sea ecosystems. Here we identify those ecosystem services associated with the interactions and transitions between chemosynthetic and background ecosystems.
On a global scale, hydrothermal vents and methane seeps are involved in the biogeochemical cycling and elemental transformation of carbon, sulfur, and nitrogen (Hinrichs and Boetius, 2002; Dekas et al., 2009; Boetius and Wenzhoefer, 2013) and contribute to the huge diversity of deep-sea organisms and habitats. What makes these ecosystems unique is the use of chemical energy from hydrogen, methane, hydrogen sulfide, ammonium or iron to fix inorganic carbon and generate increased microbial and faunal biomass. These exchanges enhance trophic and structural complexity relative to the surrounding deep sea and provide the setting for complex trophic interactions (e.g., Levin, 2005; Sellanes et al., 2008; Cordes et al., 2010; Govenar, 2010). All these ecological functions, often described as intermediate (Haines-Young and Potschin, 2013) or supporting services (Millenium Ecosystem Assessment., 2005), are the underpinning structures and processes necessary for the production of all other provisioning, regulating and cultural ecosystem services and it is unquestionable that, although distant from humans in space and time, they create value (Armstrong et al., 2012; Thurber et al., 2014).
Regulating services include the role of biogeochemical cycling of elements in climate regulation and carbon sequestration, as well as lifecycle maintenance and bioremediation potential of pollutant stressors. As much iron is delivered to the ocean by diffuse venting as from riverine sources (Toner et al., 2009) and this iron can be stabilized in nano particles allowing it to disperse widely and remain bioavailable (Yücel et al., 2011). As discussed earlier in this review, the microbial communities found at vents and seeps contribute to the global cycling of carbon, nitrogen, sulfur, and potentially heavy metals such as iron and arsenic (Nauhaus et al., 2002; Meyer-Dombard et al., 2012). AOM and associated precipitation of authigenic carbonate at cold seeps constitutes a major carbon sink in the deep sea (Ritger et al., 1987; Reeburgh, 2007). Recent discovery of active AOM in seemingly old, inactive carbonates (Marlow et al., 2014a), and the ability of fauna to benefit from substrate and food provisions within these carbonates (Stakes et al., 1999; Levin et al., 2015) suggests that C sequestration during transition phases of seeps may be more extensive in space and time than previously thought. The consumption of sulfide or methane by bacteria and archaea at seeps and vents also acts as a biological filter that prevents climate-relevant substances from freely entering the hydrosphere and acting as potential greenhouse gas sources (Jørgensen and Boetius, 2007; Boetius and Wenzhoefer, 2013). Bio-remediation potential of vent and seep microorganisms and animals is only now being explored. Use of microbes for degrading oil spills (Mason et al., 2014) or as a biocatalytic agent for industrial scrubbing of CO2 (Mahon et al., 2015) are recent examples. Lifecycle maintenance, habitat and gene pool protection are provided by coral and sponge aggregations, which often occur at elevated densities in the vicinity of vent and seep sites (Cordes et al., 2008; Quattrini et al., 2014). In addition to protection of the gene pool for these species, vents and seeps maintain habitats for refugia and reproduction of background deep-sea species (Drazen et al., 2003; Thurber et al., 2010; Treude et al., 2011).
Provisioning services are provided by both biotic and abiotic resources at vents and seeps with market values through their interaction with background systems or conditions. There is increasing evidence that trophic subsidies from seeps and vents may influence fisheries but quantification of such subsidies and further documentation of possible habitat relationships with fishery species are still lacking (Grupe et al., 2015). Several commercially exploited crustacean and fish species typically associated with background habitats are often observed at chemosynthetic ecosystems around the world. These include Patagonian toothfish, (Sellanes et al., 2008, 2012), orange roughy (Bowden et al., 2013), thornyheads, sablefish, and Pacific Dover Sole (Grupe et al., 2015; Levin et al., 2016), and lithodid decapods (Niemann et al., 2013) (Figure 2). On the Hikurangi Margin demersal fish associated with active and inactive seep sites are directly targeted by commercial trawl fisheries (Baco et al., 2010). In part, this is because acoustic flares at seep sites have been persistently misinterpreted by fishers as fish aggregations, but some fish are caught nonetheless (because the flares co-occur with carbonate structures where fish aggregate) so the practice continues. The broad range of temperature, pH and toxicity conditions in chemosynthetic ecosystems make them an obvious potential source of compounds with novel biochemical properties (e.g., Pettit, 2011). While some of these compounds have been isolated from hydrothermal vent organisms and were found to be potentially useful for industrial processes (Leis et al., 2015; Mahon et al., 2015) and pharmaceutics (Martins et al., 2013), the transition zones have yet to be targeted by bioprospectors. Methane hydrates, which are often of biogenic origin, may be used as a new energy resource and several nations are investing into technology development to quantify and unlock this reserve and harvest natural gas from methane hydrates (project SUGAR, Germany). Environmental regulation and impact assessment for exploitation of these new resources must incorporate an accurate understanding of the host ecosystems and their services.
Figure 2. Seep habitat use by “background” species of commercial value: (A) California King crab and thornyheads (B) Lithodid crabs (C) sablefish (Anoplopoma fimbria) and (D) Pacific Dover Sole (Microstomus pacificus).
Cultural services are all the non-material, and normally non-consumptive, outputs of ecosystems that affect physical and mental states of people. As we learn about the interconnection of chemosynthetic ecosystems with surrounding habitats and species the existence value (the value of knowing something exists regardless of whether or not you use it), option value (the value of knowing you have the option later), inspirational value (e.g., for art, film or literature), bequest value, and stewardship value placed on these systems may grow. Certainly scientific interest, already strong (Godet et al., 2011), will expand as living resource managers gain interest. Other intellectual and representative interactions with chemosynthetic ecosystems may highlight the interactions between vent, seep and non-chemosynthetic systems via educational, entertainment (ex-situ viewing and experience through different media) and purely aesthetic (e.g., inspiration for art and design) contexts. Within the last decade, deep-sea tourism providing in situ experiential interaction with animals and seascapes has become established at certain vent fields (Leary, 2007). Deep-sea exploration via telepresence, promoted by the US NOAA Office of Ocean Exploration and Research (NOAA Ship Okeanos Explorer) and the Ocean Exploration Trust (EV Nautilus) is greatly raising public awareness of and enthusiasm for chemosynthetic ecosystems as features interacting with the broader deep sea (e.g., Carey et al., 2015; Quattrini et al., 2015; Levin et al., 2016).
Valuation of the ecosystem services outlined above is complicated by limited knowledge and ability to determine the actual benefits that people experience. For the services associated with chemosynthetic transitions and exchanges, challenges are posed by the fact that they (a) provide services that are public goods (i.e., they may be enjoyed by any number of people without affecting other people's enjoyment), (b) no one person has an incentive to pay to maintain the good and collective action is required, (c) are affected by negative externalities or uncompensated side effects of human actions, and (d) stakeholders suffering the loss (e.g., scientists, civil society, future generations) generally are not compensated for the damages. Because no one person or group “owns” the resource (whether it is in national or international waters), open access can lead to severe overexploitation and potentially severe declines in the resource provision over time.
(1) Evidence supports the existence of transition zones, or ecotones, in space and time between vent and seep chemosynthetic habitats and the background deep-sea communities. These influence elemental cycling and energy flux, trophic linkages, provision of habitat and genetic exchange, and extend spatially along the seafloor and into the water column (Figure 3).
(2) The extent and scales over which chemosynthetic influence is exerted varies at vents and seeps. Along the seafloor, hydrothermal vents exhibit sharper gradients but smaller magnitude of influence on surrounding ecosystems than for seeps, whereas the reverse may be true for pelagic gradients and interactions.
(3) Trophic linkages between cold seeps and the background benthic community may be stronger than at vents, potentially due to relative similarity in substrata (sediments) and lack of physical and temperature barriers at seeps. In contrast, while background assemblages near hard-substrate vents may benefit from increased particles in the water column and on the seafloor, they are often less diverse and less abundant, with slow-growing, low mobility species that would have fewer direct interactions with the vent habitat. At sedimented vents, the scale of ecotones and trophic linkages could be more similar to those in seeps.
(4) The magnitude of influence on the surrounding water column is likely greater at hydrothermal vents than at seeps, although seeps are less well studied in this respect. The great vertical and horizontal reach of hydrothermal plumes acts to increase microbial activity and plankton concentrations, as well as structural and functional diversity. For some elemental fluxes such as iron, the sphere of influence of the vents can be global. The vertical and lateral extent of seep fluid influence to the water column requires more study.
(5) At both hydrothermal vents and cold seeps the chemical environment becomes less toxic and primary production decreases over time, making biogenic and geologic structures more readily available for colonization. Inactive chimneys at vents and carbonate structures at seeps can be present for 1000 s of years providing stable hard substrata for species from the background community. This increased structural habitat diversity promotes greater overall biodiversity (Cordes et al., 2010; Govenar, 2010).
Figure 3. Schematic of seep and vent interactions with surrounding deep-sea ecosystems. The y axis is meters above bottom on a log scale. DOC, Dissolved Organic Carbon; POC, Particulate Organic Carbon; SMS, Seafloor Massive Sulfide.
Despite this basic conceptual understanding (Figure 3), there are many areas where there are simply no data, or where current knowledge derives from anecdotal, non-quantitative observations. We identify 3 major themes where scientific research can help to advance our ability to accurately quantify, constrain and incorporate into management the interactions of vent and seep ecosystems with their surroundings.
What is the influence of vent and seep plumes on local and global water column biogeochemical cycles, processes, microbiology and biota? Questions remain about the magnitude of carbon, trace elements and nutrients exported from vents and seeps to the deep sea in dissolved, particulate and animal forms and the consequences for global elemental cycling.
How do fauna use inactive chemosynthetic habitats and how and over what time scales do they transition to background settings? What is the genetic connectivity between chemosynthetic and non-chemosynthetic ecosystems? Genetic exchange has yet to be examined in any systematic way, despite the fact that a number of species are known to occupy both background and reducing habitats. With recent concerted efforts to study vents and seeps in close proximity (e.g., Watanabe et al., 2010; Portail et al., 2015), including likely stepping stone habitats (wood, whale, kelp) and deep-sea coral communities (e.g., Cordes et al., 2008; Becker et al., 2009), more quantitative estimates of the co-occurrence of species have been made; these efforts will be enhanced by molecular barcoding of fauna from background and other chemosynthetic systems.
What are the transfer mechanisms of chemosynthetic and photosynthetically-derived carbon, nitrogen and sulfur into and out of vents and seeps and how does this vary with location and depth? Chemosynthetic organic matter may prime the degradation of photosynthetically produced but otherwise refractory compounds that make up the rain of production to the deep. Novel trophic approaches, beyond tracking of isotopically distinct methane, are needed to evaluate nutritional subsidies, particularly at vents.
As with much of the deep sea, megafauna and microbes are often the focus of faunal research at vents and seeps. Metazoan and protozoan meiofauna, as well as larval stages of these and other taxa, also are important to address for the transitions discussed here. The benefits of addressing these knowledge gaps concerning the chemosynthetic sphere of influence may continue to grow with an expanding human footprint in the deep sea and the need for intensified spatial planning and impact assessment.
The manuscript was initially conceived and finally edited by LAL, with conceptual framework and writing contributions by all authors.
The authors declare that the research was conducted in the absence of any commercial or financial relationships that could be construed as a potential conflict of interest.
We thank the JM Kaplan fund for financial support and the Center for Marine Biodiversity and Conservation for the logistical support of the Chemosynthesis Crossroads Workshop, held at Scripps Institution of Oceanography in Sept. 2015. LAL was supported by NSF-EAR 1324095. CLVD was supported by NSF-OCE 1031050. AC is supported by Program Investigador FCT (IF/00029/2014/CP1230/ CT0002) from FCT and through the strategic project UID/MAR/04292/2013 granted to MARE. Thanks to Cheyenne Payne for help with the bibliography. Two anonymous reviewers made helpful recommendations to an earlier version of this manuscript. This paper is a direct outcome of discussions at the workshop, and a product of the Deep-Ocean Stewardship Initiative. Any use of trade, product, or firm names is for descriptive purposes only and does not imply endorsement by the U.S. Government.
Adams, D., McGillicuddy, D. J. Jr., Zamudio, L., Thurnherr, A. M., Liang, X., Rouxell, O., et al. (2011). Surface-generated mesoscale eddies transport deep-sea products from hydrothermal vents. Science 332, 580–583. doi: 10.1126/science.1201066
Adams, D. K., Arellano, S. M., and Govenar, B. (2012). Larval dispersal: vent life in the water column. Oceanography 25, 256–268. doi: 10.5670/oceanog.2012.24
Alt, J. C. (2003). Stable isotopic composition of upper oceanic crust formed at a fast spreading ridge, ODP Site 801. Geochem. Geophys. Geosyst. 4:8908. doi: 10.1029/2002gc000400
Arellano, S., Van Gaest, A., Johnson, S., Vrijenhoek, R., and Young, C. (2014). Larvae from deep-sea methane seeps disperse in surface waters. Proc. R. Soc. B 281:20133276. doi: 10.1098/rspb.2013.3276
Arellano, S. M., and Young, C. M. (2011). Temperature and salinity tolerances of embryos and larvae of the deep-sea mytilid mussel Bathymodiolus childressi. Mar. Biol. 158, 2481–2493. doi: 10.1007/s00227-011-1749-9
Armstrong, C. W., Foley, N. S., Tinch, R., and van den Hove, S. (2012). Services from the deep: steps towards valuation of deep sea goods and services. Ecosyst. Serv. 2, 2–13. doi: 10.1016/j.ecoser.2012.07.001
Audzijonyte, A., Krylova, E. M., Sahling, H., and Vrijenhoek, R. C. (2012). Molecular taxonomy reveals broad trans-oceanic distributions and high species diversity of deep-sea clams (Bivalvia: Vesicomyidae: Pliocardiinae) in chemosynthetic environments. Syst. Biodivers. 10, 403–415. doi: 10.1080/14772000.2012.744112
Bachraty, C., Legendre, P., and Desbruyeres, D. (2009). Biogeographic relationships among deep-sea hydrothermal vent faunas at global scale. Deep Sea Res. Part I 56, 1371–1378. doi: 10.1016/j.dsr.2009.01.009
Baco, A., Smith, C., Peek, A., Roderick, G., and Vrijenhoek, R. (1999). The phylogenetic relationships of whale-fall vesicomyid clams based on mitochondrial COI DNA sequences. Mar. Ecol. Prog. Ser. 182, 137–147. doi: 10.3354/meps182137
Baco, A. R., Etter, R., Beerli, P., Ribiero, P., Von Der Heyden, S., and Kinlan, B. (in press). A synthesis of genetic connecitivity in deep-sea fauna implications for marine reserve design. Mol. Ecol.
Baco, A. R., Rowden, A. A., Levin, L. A., Smith, C. R., and Bowden, D. A. (2010). Initial characterization of cold seep faunal communities on the New Zealand Hikurangi margin. Mar. Geol. 272, 251–259. doi: 10.1016/j.margeo.2009.06.015
Baker, E., Resing, J., Haymon, R., Tunnicliffe, V., Martinez, R., Ferrini, V., et al. (2015). How many vent fields? New estimates of vent field populations on ocean ridges from precise mapping of hydrothermal discharge locations. Earth Planet. Sci. Lett. 47–71. doi: 10.1029/GM091p0047
Baker, E. T., German, C. R., and Elderfield, H. (1995). Hydrothermal plumes over spreading-center axes: global distributions and geological inferences, in Seafloor Hydrothermal Systems: Physical, Chemical, Biological and Geological Interactions, eds S. E. Humphris, R. A. Zierenberg, L. S. Mullineaux, and R. E. Thomson (Washington, DC: American Geophysical Union), 47–71. doi: 10.1029/GM091p0047
Baker, J. B., Lesniewski, R. A., and Dick, G. J. (2012). Genome-enabled transcriptomics reveals archaeal populations that drive nitrification in a deep-sea hydrothermal plume. ISME J. 6, 2269–2279. doi: 10.1038/ismej.2012.64
Baker, M., Ramirez-Llodra, E., Tyler, P., German, C., Boetius, A., Cordes, E., et al. (2010). Biogeography, ecology and vulnerability of chemosynthetic ecosystems in the deep sea, in Life in the World's Oceans, ed A. D. McIntyre (Oxford: Blackwell Publishing Ltd.), 161–182.
Barnes, P. M., Lamarche, G., Bialas, J., Henrys, S., Pecher, I., Netzeband, G. L., et al. (2010). Tectonic and geological framework for gas hydrates and cold seeps on the Hikurangi subduction margin, New Zealand. Mar. Geol. 272, 26–48. doi: 10.1016/j.margeo.2009.03.012
Barry, J. P., Kochevar, R. E., and Baxter, C. H. (1997). The influence of pore-water chemistry and physiology on the distribution of vesicomyid clams at cold seeps in Monterey Bay: implications for patterns of chemosynthetic community organization. Limnol. Oceanogr. 42, 318–328. doi: 10.4319/lo.1997.42.2.0318
Beaulieu, S. E., Baker, E. T., and German, C. R. (2015). Where are the undiscovered hydrothermal vents on oceanic spreading ridges? Deep Sea Res. II Top. Stud. Oceanogr. 121, 202–212. doi: 10.1016/j.dsr2.2015.05.001
Beaulieu, S. E., Baker, E. T., German, C. R., and Maffei, A. (2013). An authoritative global database for active submarine hydrothermal vent fields. Geochem. Geophys. Geosyst. 14, 4892–4905. doi: 10.1002/2013GC004998
Becker, E., Cordes, E., Macko, S., and Fisher, C. (2009). Importance of seep primary production to Lophelia pertusa and associated fauna in the Gulf of Mexico. Deep Sea Res. I 56, 786–800. doi: 10.1016/j.dsr.2008.12.006
Bennett, S. A., Statham, P. J., Green, D. R. H., Bris, N. L., McDermot, J. M., Prado, F., et al. (2011). Dissolved and particulate organic carbon export from seafloor hydrothermal venting: east Pacific Rise, 9°50'N. Deep Sea Res. Part I Oceanogr. Res. Papers 58, 922–931. doi: 10.1016/j.dsr.2011.06.010
Berg, C. J., and Vandover, C. L. (1987). Benthopelagic macrozooplankton communities at and near deep-sea hydrothermal vents in the eastern Pacific ocean and the Gulf of California. Deep Sea Res. Part A Oceanogr. Res. Papers 34, 379–401. doi: 10.1016/0198-0149(87)90144-0
Bergquist, D. C., Fleckenstein, C., Szalai, E. B., Knisel, J., and Fisher, C. R. (2004). Environment drives physiological variability in the cold seep mussel Bathymodiolus childressi. Limnol. Oceanogr. 49, 706–715. doi: 10.4319/lo.2004.49.3.0706
Bergquist, D. C., Ward, T., Cordes, E. E., McNelis, T., Howlett, S., Kosoff, R., et al. (2003). Community structure of vestimentiferan-generated habitat islands from Gulf of Mexico cold seeps. J. Exp. Mar. Biol. Ecol. 289, 197–222. doi: 10.1016/S0022-0981(03)00046-7
Bernardino, A., Levin, L., Thurber, A., and Smith, C. (2012). Comparative composition, diversity and trophic ecology of sediment macrofauna at vents, seeps and organic falls. PLoS ONE 7:e33515. doi: 10.1371/journal.pone.0033515
Boetius, A., and Wenzhoefer, F. (2013). Seafloor oxygen consumption fuelled by methane from cold seeps. Nat. Geosci. 6, 725–734. doi: 10.1038/ngeo1926
Borda, E., Kudenov, J. D., Chevaldonné, P., Blake, J. A., Desbruyères, D., Fabri, M. C., et al. (2013). Cryptic species of Archinome (Annelida: Amphinomida) from vents and seeps. Proc. R. Soc. Lond. B 280:20131876. doi: 10.1098/rspb.2013.1876
Boschen, R. E., Rowden, A. A., Clark, M. R., Barton, S. J., Pallentin, A., and Gardner, J. P. A. (2015). New Zealand seamounts: implications for seafloor massive sulfide mining. Mar. Ecol. Prog. Ser. 523, 1–14. doi: 10.3354/meps11239
Bowden, D. A., Rowden, A. A., Thurber, A. R., Baco, A. R., Levin, L. A., and Smith, C. R. (2013). Cold seep epifaunal communities on the Hikurangi Margin, New Zealand: composition, succession, and vulnerability to human activities. PLoS ONE 8:e76869. doi: 10.1371/journal.pone.0076869
Burd, B. J., and Thomson, R. E. (1994). Hydrothermal venting at Endeavor Ridge – effect on zooplankton biomass throughout the water column. Deep Sea Res. Part I Oceanogr. Res. Papers 41, 1407–1423. doi: 10.1016/0967-0637(94)90105-8
Burd, B. J., and Thomson, R. E. (1995). Dstribution of zooplankton associated with the Endeavor Ridge hydrothermal plume. J. Plankton Res. 17, 965–997. doi: 10.1093/plankt/17.5.965
Butterfield, D. A., Jonasson, I. R., Massoth, G. J., Feely, R. A., Roe, K. K., Embley, R. E., et al. (1997). Seafloor eruptions and evolution of hydrothermal fluid chemistry. Philos. Trans. R. Soc. Lond. A Math. Phys. Eng. Sci. 355, 369–386. doi: 10.1098/rsta.1997.0013
Carey, S., Bell, K. L. C., Roman, C., Dondin, F., Robertson, R., Gobin, J., et al. (2015). Exploring Kick'em Jenny submarine volcano and the Barbados cold seep province, southern Lesser Antilles. Oceanography 28(Suppl. 1), 38–39.
Case, D. H., Pasulka, A. L., Marlow, J. J., Grupe, B. M., and Levin, L. A. (2015). Methane seep carbonates host distinct, diverse, and dynamic microbial assemblages. mBio 6:e01348-15. doi: 10.1128/mBio.01348-15
Childress, J., and Fisher, C. (1992). The biology of hydrothermal vent animals: physiology, biochemistry, and autotrophic symbioses. Oceanogr. Mar. Biol. Ann. Rev. 30, 337–441.
Ciani, D., Carton, X. J., Chapron, B., and Bashmachnikov, I. (2014). Surface signature of subsurface-intensified vortices. Am. Geophys. Union Fall Meeting 2014 abstract #OS43C–1298.
Colaço, A., Dehairs, F., and Desbruyeres, D. (2002). Nutritional relations of deep-sea hydrothermal fields at the Mid-Atlantic Ridge: a stable isotope approach. Deep Sea Res. Part I 49, 395–412. doi: 10.1016/S0967-0637(01)00060-7
Colaço, A., Prieto, C., Martins, A., Figueiredo, M., Lafon, V., Monteiro, M., et al. (2009). Seasonal variations in lipid composition of the hydrothermal vent mussel Bathymodiolus azoricus from the Menez Gwen vent field. Mar. Environ. Res. 67, 146–152. doi: 10.1016/j.marenvres.2008.12.004
Copley, J. T. P., Jorgensen, P. B. K., and Sohn, R. A. (2007). Assessment of decadal-scale ecological change in the shrimp Rimicaris exoculata. J. Mar. Biol. Assoc. UK 84, 859–867. doi: 10.1017/S0025315407056512
Copley, C. E. A., Tyler, P. A., and Varney, M. S. (1998). Lipid profiles of hydrothermal vent shrimps, in International Symposium on Deep-Sea Hydrothermal Vent Biology No 1 (Funchal, Madeira).
Cordes, E., Bergquist, D., Predmore, B., Dienes, P., Jones, C., Telesnicki, G., et al. (2006). Alternate unstable states: convergent paths of succession in hydrocarbon-seep tubeworm-associated communities. J. Exp. Mar. Biol. Ecol. 339, 159–176. doi: 10.1016/j.jembe.2006.07.017
Cordes, E. E., Bergquist, D. C., and Fisher, C. R. (2009). Macro-ecology of Gulf of Mexico cold seeps. Ann. Rev. Mar. Sci. 1, 143–168. doi: 10.1146/annurev.marine.010908.163912
Cordes, E. E., Cunha, M. R., Galéron, J., Mora, C., Olu-Le Roy, K., Sibuet, M., et al. (2010). The influence of geological, geochemical, and biogenic habitat heterogeneity on seep biodiversity. Mar. Ecol. 31, 51–65. doi: 10.1111/j.1439-0485.2009.00334.x
Cordes, E. E., Hourdez, S., Predmore, B. L., Redding, M. L., and Fisher, C. R. (2005). Succession of hydrocarbon seep communities associated with the long-lived foundation species Lamellibrachia luymesi. Mar. Ecol. Prog. Ser. 305, 17–29. doi: 10.3354/meps305017
Cordes, E. E., McGinley, M. P., Podowski, E. L., Becker, E. L., Lessard-Pilon, S. A., Viada, S. T., et al. (2008). Coral communities of the deep Gulf of Mexico. Deep Sea Res. Part I. 55, 777–787. doi: 10.1016/j.dsr.2008.03.005
Corliss, J. B., Dymond, J., Gordon, L. I., Edmond, J. M., von Herzen, R. P., Ballard, R. D., et al. (1979). Submarine thermal springs on the Galapagos Rift. Science 203, 1073–1083. doi: 10.1126/science.203.4385.1073
Cowen, J. P., Bertram, M. A., Wakeham, S. G., Thomson, R. E., Lavelle, J. W., Baker, E. T., et al. (2001). Ascending and descending particle flux from hydrothermal plumes at Endeavour Segment, Juan de Fuca Ridge. Deep Sea Res. Part I 48, 1093–1120. doi: 10.1016/S0967-0637(00)00070-4
Cowen, R. K., and Sponaugle, S. (2009). Larval dispersal and marine population connectivity. Ann. Rev. Mar. Sci. 1, 443–466. doi: 10.1146/annurev.marine.010908.163757
Cunha, M., Matos, F., Génio, L., Hilário, A., Moura, C., Ravara, A., et al. (2013a). Are organic falls bridging reduced environments in the deep sea? – Results from colonization experiments in the Gulf of Cadiz. PLoS ONE 8:e76688. doi: 10.1371/journal.pone.0076688
Cunha, M., Rodrigues, C., Génio, L., Hilário, A., Ravara, A., and Pfannkuche, O. (2013b). Macrofaunal assemblages from mud volcanoes in the Gulf of Cadiz: abundance, biodiversity and diversity partitioning across spatial scales. Biogeosciences 10, 2553–2568. doi: 10.5194/bg-10-2553-2013
Cuvelier, D., Sarrazin, J., Colaço, A., Copley, J. T., Glover, A. G., Tyler, P. A., et al. (2011). Community dynamics over 14 years at the Eiffel Tower hydrothermal edifice on the Mid-Atlantic Ridge. Limnol. Oceanogr. 56, 1624–1640. doi: 10.4319/lo.2011.56.5.1624
Dando, P., Southward, A., and Southward, E. (1992). Shipwrecked tube worms. Nature 356, 667. doi: 10.1038/356667a0
de Beer, D., Sauter, E., Niemann, H., Kaul, N., Foucher, J. P., Witte, U., et al. (2006). In situ fluxes and zonation of microbial activity in surface sediments of the Håkon Mosby Mud Volcano. Limnol. Oceanogr. 51, 1315–1331. doi: 10.4319/lo.2006.51.3.1315
Decker, C., Olu, K., Cunha, R. L., and Arnaud-Haond, S. (2012). Phylogeny and diversification patterns among vesicomyid bivalves. PLoS ONE 7:e33359. doi: 10.1371/journal.pone.0033359
Dekas, A. E., Poretsky, R. S., and Orphan, V. J. (2009). Methane-consuming microbial consortia deep-sea archaea fix and share nitrogen in methane-consuming microbial consortia. Science 326, 422–426. doi: 10.1126/science.1178223
Demopoulos, A., Gualtieri, D., and Kovacs, K. (2010). Food-web structure of seep sediment macrobenthos from the Gulf of Mexico. Deep Sea Res. Part II 57, 1972–1981. doi: 10.1016/j.dsr2.2010.05.011
Desbruyères, D. (1998). Temporal variations in the vent communities on the East Pacific Rise and Galapagos Spreading Centre: a review of present knowledge. Cah. de Biol. Mar. 39, 241–244.
Desbruyères, D., Biscoito, M., Caprais, J.-C., Colaço, A., Comtet, T., Crassous, P., et al. (2001). Variations in deep-sea hydrothermal vent communities on the Mid-Atlantic Ridge near the Azores plateau. Deep Sea Re. Part I 48, 1325–1346. doi: 10.1016/S0967-0637(00)00083-2
Dick, G. J., Anantharaman, K., Baker, B. J., Li, M., Reed, D. C., and Sheik, C. S. (2013). The microbiology of deep-sea hydrothermal vent plumes: ecological and biogeographic linkages to seafloor and water column habitats. Front. Microbiol. 4:124. doi: 10.3389/fmicb.2013.00124
Dick, G. J., and Tebo, B. M. (2010). Microbial diversity and biogeochemistry of the Guaymas Basin deep-sea hydrothermal plume. Environ. Microbiol. 12, 1334–1347. doi: 10.1111/j.1462-2920.2010.02177.x
Distel, D. L., Baco, A. R., Chuang, E., Morrill, W., Cavanaugh, C., and Smith, C. R. (2000). Marine ecology: do mussels take wooden steps to deep-sea vents? Nature 403, 725–726. doi: 10.1038/35001667
Drazen, J. C., Goffredi, S. K., Schlining, B., and Stakes, D. S. (2003). Aggregations of egg-brooding deep-sea fish and cephalopods on the Gorda Escarpment: a reproductive hot spot. Biol. Bull. 205, 1–7. doi: 10.2307/1543439
Eckelbarger, K. J., and Watling, L. (1995). Role of phylogenetic constraints in determining reproductive patterns in deep-sea invertebrates. Inverteb. Biol. 114, 256–269. doi: 10.2307/3226880
Edmond, J. M., Measures, C., McDuff, R. E., Chan, L. H., Collier, R., and Grant, B. (1979). Ridge crest hydrothermal activity and the balances of the major and minor elements in the ocean; the Galapagos data. Earth Planet. Sci. Lett. 46, 1–18. doi: 10.1016/0012-821X(79)90061-X
Erickson, K. L., Macko, S. A., and Dover, C. L. V. (2009). Evidence for a chemoautotrophically based food web at inactive hydrothermal vents (Manus Basin). Deep Sea Res. II 56, 1577–1585. doi: 10.1016/j.dsr2.2009.05.002
Faure, K., Greinert, J., von Deimling, J. S., McGinnis, D. F., Kipfer, R., and Linke, P. (2010). Methane seepage along the Hikurangi Margin of New Zealand: geochemical and physical data from the water column, sea surface and atmosphere. Mar. Geol. 272, 170–188. doi: 10.1016/j.margeo.2010.01.001
Feldman, R. A., Shank, T. M., Black, M. B., Baco, A. R., Smith, C. R., and Vrijenhoek, R. C. (1998). Vestimentiferan on a whale fall. Biol. Bull. 194, 116–119. doi: 10.2307/1543041
Fischer, D., Sahling, H., Noethen, K., Bohrmann, G., Zabel, M., and Kasten, S. (2012). Interaction between hydrocarbon seepage, chemosynthetic communities, and bottom water redox at cold seeps of the Makran accretionary prism: insights from habitat-specific pore water sampling and modeling. Biogeosciences 9, 2013–2031. doi: 10.5194/bg-9-2013-2012
Fonseca, V. G., Carvalho, G. R., Sung, W., Johnson, H. F., Power, D. M., Neill, S. P., et al. (2010). Second-generation environmental sequencing unmasks marine metazoan biodiversity. Nat. Commun. 1, 98. doi: 10.1038/ncomms1095
Fujikura, K., Amaki, K., Barry, J., Fujiwara, Y., Furushima, Y., Iwase, R., et al. (2007). Long-term in situ monitoring of spawning behavior and fecundity in Calyptogena spp. Mar. Ecol. Prog. Ser. 333, 185–193. doi: 10.3354/meps333185
Fustec, A., Desbruyères, D., and Juniper, K. S. (1987). Deep-sea hydrothermal vent communities at 13°N on the East Pacific Rise: microdistribution and temporal variations. Biol. Oceanogr. 4, 121–164.
Galkin, S. V. (1992). The benthic fauna of hydrothermal vents in the Manus Basin. Oceanologyia 32, 768–774.
Galkin, S. V. (1997). Megafauna associated with hydrothermal vents in the Manus Back-Arc Basin (Bismarck Sea). Mar. Geol. 142, 197–206. doi: 10.1016/S0025-3227(97)00051-0
Gebruk, A. V., Southward, E. C., Kennedy, H., and Southward, A. J. (2000). Food sources, behaviour, and distribution of hydrothermal vent shrimps at the Mid-Atlantic Ridge. J. Mar. Biol. Assoc. UK 80, 485–499. doi: 10.1017/S0025315400002186
Génio, L., Johnson, S., Vrijenhoek, R., Cunha, M., Tyler, P., Kiel, S., et al. (2008). New record of “Bathymodiolus” mauritanicus Cosel from Gulf of Cadiz (NE Atlantic) mud volcanoes. J. Shellfish Res. 27, 53–61. doi: 10.2983/0730-8000(2008)27[53:NROBMC]2.0.CO;2
Génio, L., Waren, A., Matos, F. L., and Cunha, M. R. (2013). The snails' tale in deep-sea habitats in the Gulf of Cadiz (NE Atlantic). Biogeosciences 10, 5159–5170. doi: 10.5194/bg-10-5159-2013
Girguis, P. R., and Lee, R. W. (2006). Thermal preference and tolerance of alvinellids. Science 312, 231. doi: 10.1126/science.1125286
Godet, L., Zelnio, K. A., and Van Dover, C. L. (2011). Scientists as stakeholders in conservation of hydrothermal vents. Conserv. Biol. 25, 214–222. doi: 10.1111/j.1523-1739.2010.01642.x
Govenar, B. (2010). Shaping vent and seep communities: habitat provision and modification by foundation species, in The Vent and Seep Biota: Aspects from Microbes to Ecosystems, ed S. Kiel (Dordrecht: Springer), 403–432. doi: 10.1007/978-90-481-9572-5_13
Govenar, B., LeBris, N., Gollner, S., Glanville, J., Aperghis, A. B., Hourdez, S., et al. (2005). Epifaunal community structure associated with Riftia pachyptila in chemically different hydrothermal vent habitats. Mar. Ecol. Prog. Ser. 305, 67–77. doi: 10.3354/meps305067
Grassle, J. F. (1985). Hydrothermal vent animals: distribution and biology. Science 229, 713–717. doi: 10.1126/science.229.4715.713
Greinert, J., Lewis, K. B., Bialas, J., Pecher, I. A., Rowden, A., Bowden, D. A., et al. (2010). Methane seepage along the Hikurangi Margin, New Zealand: overview of studies in 2006 and 2007 and new evidence from visual, bathymetric and hydroacoustic investigations. Mar. Geol. 272, 6–25. doi: 10.1016/j.margeo.2010.01.017
Grupe, B. M., Krach, M. L., Pasulka, A. L., Maloney, J. M., Levin, L. A., and Frieder, C. A. (2015). Methane seep ecosystem functions and services from a recently discovered southern California seep. Mar. Ecol. 36, 91–108. doi: 10.1111/maec.12243
Guilini, K., Levin, L. A., and Vanreusel, A. (2012). Cold seep and oxygen minimum zone associated sources of margin heterogeneity affect benthic assemblages, diversity and nutrition at the Cascadian margin (NE Pacific Ocean). Prog. Oceanogr. 96, 77–92. doi: 10.1016/j.pocean.2011.10.003
Haines-Young, R., and Potschin, M. (2013). Common International Classification of Ecosystem Services (CICES): Consultation on Version 4. Report to the European Environmental Agency, EEA Framework Contract no: EEA/IEA/09/003. (August–December 2012).
Haymon, R. M., Fornari, D. J., Von Damm, K. L., Lilley, M. D., Perfit, M. R., Edmond, J. M., et al. (1993). Volcanic eruption of the mid-ocean ridge along the East Pacific Rise crest at 9°45-52'N: direct submersible observations of seafloor phenomena associated with an eruption event in April, 1991. Earth Planet. Sci. Lett. 119, 85–101. doi: 10.1016/0012-821X(93)90008-W
Hessler, R. R., Smithey, W. M., Boudrias, M. A., Keller, C. H., Lutz, R. A., and Childress, J. J. (1988). Temporal change in megafauna at the Rose Garden hydrothermal vent (Galapagos Rift; eastern tropical Pacific). Deep Sea Res. Part A 35, 1681–1709. doi: 10.1016/0198-0149(88)90044-1
Hessler, R. R., Smithey, W. M., and Keller, C. H. (1985). Spatial and temporal variation of giant clams, tubeworms and mussels at deep-sea hydrothermal vents. Bull. Biol. Soc. Wash. 6, 411–428.
Hilário, A., Capa, M., Dahlgren, T. G., Halanych, K. M., Little, C. T., Thornhill, D. J., et al. (2011). New perspectives on the ecology and evolution of siboglinid tubeworms. PLoS ONE 6:e16309. doi: 10.1371/journal.pone.0016309
Hilário, A., Metaxas, A., Gaudron, S., Howell, K., Mercier, A., Mestre, N., et al. (2015). Estimating dispersal distance in the deep sea: challenges and applications to marine reserves. Front. Mar. Sci. 2:6. doi: 10.3389/fmars.2015.00006
Hinrichs, K., and Boetius, A. (2002). The anaerobic oxidation of methane: new insights in microbial ecology and biogeochemistry, in Ocean Margin Systems, eds G. Wefer, D. Billett, D. Hebbeln, B. Jorgensen, M. Schulter, and T. Van Weering (Berlin; Heidelberg: Springer-Verlag), 457–477.
Huber, J. A., Mark Welch, D. B., Morrison, H. G., Huse, S. M., Neal, P. R., Butterfield, D. A., et al. (2007). Microbial population structures in the deep marine biosphere. Science 318, 97–100. doi: 10.1126/science.1146689
Hughes, D. J., and Crawford, M. (2008). A new record of the vestimentiferan Lamellibrachia sp.(Polychaeta: Siboglinidae) from a deep shipwreck in the eastern Mediterranean. Mar. Biodivers. Rec. 1:e21. doi: 10.1017/s1755267206001989
InterRidge (2006). Statement of Commitment to Responsible Research Practices at Deep-Sea Hydrothermal Vents. Kiel: Underwater Mining Institute.
Jamieson, J. W., Clague, D. A., and Hannington, M. D. (2014). Hydrothermal sulfide accumulation along the Endeavour Segment, Juan de Fuca Ridge. Earth Planet. Sci. Lett. 395, 136–148. doi: 10.1016/j.epsl.2014.03.035
Jannasch, H. W. (1995). Microbial interactions with hydrothermal fluids. Seafloor Hydrotherm. Syst. 273–296. doi: 10.1029/gm091p0273
Jansson, J. (2013). Omics for understanding microbial functional dynamics. Environm. Microbiol. 14, 1–3. doi: 10.1111/j.1462-2920.2011.02518.x
Jerosch, K., Schlüter, M., Foucher, J.-P., Allais, A.-G., Klages, M., and Edy, C. (2007). Spatial distribution of mud flows, chemoautotrophic communities, and biogeochemical habitats at Haakon Mosby Mud Volcano. Mar. Geol. 243, 1–17. doi: 10.1016/j.margeo.2007.03.010
Johnson, P., Miller, U. K., and Solomon, E. A. (2015a). Analysis of bubble plume distributions to evaluate methane hydrate decomposition on the continental slope. Geochem. Geophys. Geosyst.16, 3825–3839. doi: 10.1002/2015GC005955
Johnson, S. B., Warén, A., Tunnicliffe, V., Dover, C. V., Wheat, C. G., Schultz, T. F., et al. (2015b). Molecular taxonomy and naming of five cryptic species of Alviniconcha snails (Gastropoda: Abyssochrysoidea) from hydrothermal vents. System. Biodivers. 13, 278–295. doi: 10.1080/14772000.2014.970673
Johnson, S. B., Warén, A., and Vrijenhoek, R. C. (2008). DNA barcoding of Lepetodrilus limpets reveals cryptic species. J. Shellfish Res. 27, 43–51. doi: 10.2983/0730-8000(2008)27[43:DBOLLR]2.0.CO;2
Johnson, S. B., Young, C. R., Jones, W. J., Warén, A., and Vrijenhoek, R. C. (2006). Migration, isolation, and speciation of hydrothermal vent limpets (Gastropoda; Lepetodrilidae) across the Blanco Transform Fault. Biol. Bull. 210, 140–157. doi: 10.2307/4134603
Jones, A. T., Greinert, J., Bowden, D. A., Klaucke, I., Petersen, C. J., Netzeband, G. L., et al. (2010). Acoustic and visual characterisation of methane-rich seabed seeps at Omakere Ridge on the Hikurangi Margin, New Zealand. Mar. Geol. 272, 154–169. doi: 10.1016/j.margeo.2009.03.008
Jones, W., Won, Y., Maas, P., Smith, P., Lutz, R., and Vrijenhoek, R. (2006). Evolution of habitat use by deep-sea mussels. Mar. Biol. 148, 841–851. doi: 10.1007/s00227-005-0115-1
Jørgensen, B. B., and Boetius, A. (2007). Feast and famine—microbial life in the deep-sea bed. Nat. Rev. Microbiol. 5, 770–781. doi: 10.1038/nrmicro1745
Juniper, S. K., Bird, D. F., Summit, M., Vong, M. P., and Baker, E. T. (1998). Bacterial and viral abundances in hydrothermal event plumes over northern Gorda Ridge. Deep Sea Res. Part II 45, 2739–2749. doi: 10.1016/S0967-0645(98)00091-5
Kaartvedt, S., Dover, C. L. V., Mullineaux, L. S., Wiebe, P. H., and Bollens, S. M. (1994). Amphipods on a deep-sea hydrothermal treadmill. Deep Sea Res. Part I 41, 179–195. doi: 10.1016/0967-0637(94)90032-9
Kark, S., and Van Rensburg, B. J. (2006). Ecotones: marginal or central areas of transition? Isr. J. Ecol. Evol. 52, 29–53. doi: 10.1560/IJEE.52.1.29
Kelley, C. A., Coffin, R. B., and Cifuentes, L. A. (1998). Stable isotope evidence for alternative bacterial carbon sources in the Gulf of Mexico. Limnol. Oceanogr. 43, 1962–1969.
Killingley, J. S., Berger, W. H., MacDonald, K. C., and Newman, W. A. (1980). 18O/16O variations in deep-sea carbonate shells from the Rise hydrothermal field. Nature 287, 218–221. doi: 10.1038/287218a0
Kinlan, B. P., and Gaines, S. D. (2003). Propagule dispersal in marine and terrestrial environments: a community perspective. Ecology 84, 2007–2020. doi: 10.1890/01-0622
Lartaud, F., de Rafelis, M., Oliver, G., Krylova, E., Dyment, J., Ildefonse, B., et al. (2010). Fossil clams from a serpentinite-hosted sedimented vent field near the active smoker complex Rainbow, MAR, 36°13′N: insight into the biogeography of vent fauna. Geochem. Geophys. Geosyst. 11:Q0AE01. doi: 10.1029/2010gc003079
Law, C. S., Nodder, S. D., Mountjoy, J. J., Marriner, A., Orpin, A., Pilditch, C. A., et al. (2010). Geological, hydrodynamic and biogeochemical variability of a New Zealand deep-water methane cold seep during an integrated three-year time-series study. Mar. Geol. 272, 189–208. doi: 10.1016/j.margeo.2009.06.018
Leary, D. K. (2007). International Law and the Genetic Resources of the Deep Sea. Leiden: Martinus Nijhoff Publishers.
Leis, B., Heinze, S., Angelov, A., Pham, V. T. T., Thurmer, A., Jebbar, M. W. L., et al. (2015). Functional screening of hydrolytic activities reveals an extremely thermostable cellulase from a deep-sea archaeon. Front. Bioeng. Biotechnol. 3:95. doi: 10.3389/fbioe.2015.00095
Levesque, C., Juniper, S. K., and Limen, H. (2006). Spatial organization of food webs along habitat gradients at deep-sea hydrothermal vents on Axial Volcano, Northeast Pacific. Deep Sea Res. I 53, 726–739. doi: 10.1016/j.dsr.2006.01.007
Levin, L. A. (2003). Oxygen minimum zone benthos: adaptation and community response to hypoxia. Oceanogr. Mar. Biol. 41, 1–45.
Levin, L. A. (2005). Ecology of cold seep sediments: interactions of fauna with flow, chemistry, and microbes. Oceanogr. Mar. Biol. Ann. Rev. 43, 1–46. doi: 10.1201/9781420037449.ch1
Levin, L. A., Boesch, D. F., Covich, A., Dahm, C., Erseus, C., Ewel, K., et al. (2001). The function of marine critical transition zones and the role of sediment biodiversity. Ecosystems 4, 430–451. doi: 10.1007/s10021-001-0021-4
Levin, L. A., Girguis, P. R., German, C. R., Brennan, M. L., Tüzün, S., Wagner, J., et al. (2016). Exploration and discovery of methane seeps and associated communities in the California Borderland, in New Frontiers in Ocean Exploration: The E/V Nautilus and NOAA Ship Okeanos Explorer 2015 Field Season, eds K. L. C. Bell, M. L. Brennan, J. Flanders, N. A. Raineault, and K. Wagner (Rockville, MD: The Oceanography Society), 40–43. doi: 10.5670/oceanog.2016.supplement.01
Levin, L. A., James, D. W., Martin, C. M., Rathburn, A., Harris, L., and Michener, R. (2000). Do methane seeps support distinct infaunal assemblages? Observations on community structure and nutrition from the northern California slope and shelf. Mar. Ecol. Prog. Ser. 208, 21–39. doi: 10.3354/meps208021
Levin, L. A., Mendoza, G. F., Gonzalez, J., and Thurber, A. (2010). Diversity of bathyal macrobenthos on the northeastern Pacific margin: the influence of methane seeps and oxygen minimum zones. Mar. Ecol. 31, 94–110. doi: 10.1111/j.1439-0485.2009.00335.x
Levin, L. A., Mendoza, G. F., Grupe, B. M., Gonzalez, J. P., Jellison, B., Rouse, G. W., et al. (2015). Biodiversity on the Rocks: macrofauna inhabiting authigenic carbonate at Costa Rica methane seeps. PLoS ONE 10:e0136129. doi: 10.1371/journal.pone.0136129
Levin, L. A., Mendoza, G. F., Konotchick, T., and Lee, R. (2009). Community structure and trophic relationships in Pacific hydrothermal sediments. Deep Sea Res. II 56, 1632–1648. doi: 10.1016/j.dsr2.2009.05.010
Levin, L. A., and Michener, R. (2002). Isotopic evidence of chemosynthesis-based nutrition of macrobenthos: the lightness of being at Pacific methane seeps. Limnol. Oceanogr. 47, 1336–1345. doi: 10.4319/lo.2002.47.5.1336
Levin, L. A., Orphan, V. J., Rouse, G. W., Ussler, W., Rathburn, A. E., Cook, G. S., et al. (2012). A hydrothermal seep on the Costa Rica margin: middle ground in a continuum of reducing ecosystems. Proc. R. Soc. B 279, 2580–2588. doi: 10.1098/rspb.2012.0205
Levy, E. M., and Lee, K. (1988). Potential contribution of natural hydrocarbon seepage to benthic productivity and the fisheries of Atlantic Canada. Can. J. Fish. Aquatic Sci. 35, 349–352. doi: 10.1139/f88-041
Li, Y., Kocot, K. M., Schander, C., Santos, S. R., Thornhill, D. J., and Halanych, K. M. (2015). Mitogenomics reveals phylogeny and repeated motifs in control regions of the deep-sea family Siboglinidae (Annelida). Mol. Phylogenet. Evol. 85, 221–229. doi: 10.1016/j.ympev.2015.02.008
Lilley, M. D., Feely, R. A., and Trefry, J. H. (1995). Chemical and biochemical transformations in hydrothermal plumes, in Seafloor Hydrothermal Systems: Physical, Chemical, Biological, and Geological Interactions, eds S. E. Humphris, R. A. Zierenberg, L. S. Mullineaux, and R. E. Thomson (Washington, DC: American Geophysical Union), 369–391. doi: 10.1029/gm091p0369
Lupton, J., and Craig, H. (1981). A Major Helium-3 Source at 15°S on the East Pacific Rise. Science 214, 13–18. doi: 10.1126/science.214.4516.13
MacAvoy, S. E., Carney, R. S., Fisher, C. R., and Macko, S. A. (2002). Use of chemosynthetic biomass by large, mobile, benthic predators in the Gulf of Mexico. Mar. Ecol. Prog. Ser. 225, 65–78. doi: 10.3354/meps225065
MacAvoy, S. E., Carney, R. S., Morgan, E., and Macko, S. A. (2008). Stable isotope variation among the mussel Bathymodiolus childressi and associated heterotrophic fauna at four cold-seep communities in the Gulf of Mexico. J. Shellfish Res. 27, 147–151. doi: 10.2983/0730-8000(2008)27[147:SIVATM]2.0.CO;2
MacAvoy, S. E., Macko, S. A., and Carney, R. S. (2003). Links between chemosynthetic production and mobile predators on the Louisiana continental slope: stable carbon isotopes of specific fatty acids. Chem. Geol. 201, 229–237. doi: 10.1016/S0009-2541(03)00204-3
MacDonald, I. R., Guinasso, N. L., Reilly, J. F., Brooks, J. M., Callender, W. R., and Gabrielle, S. G. (1990). Gulf of Mexico seep communities: VI. Patterns in community structure and habitat. Geo-Mar. Let.10, 244–252.
Magalhães, V. H., Pinheiro, L. M., Ivanov, M. K., Kozlova, E., Blinova, V., Kolganova, J., et al. (2012). Formation processes of methane-derived authigenic carbonates from the Gulf of Cadiz. Sediment. Geol. 243–244, 155–168. doi: 10.1016/j.sedgeo.2011.10.013
Mahon, B. P., Bhatt, A., Vulla, D., Supuran, C. T., and McKenna, R. (2015). Exploration of anionic inhibition of the α-carbonic anhydrase from Thiomicrospira crunogena XCL- 2 gammaproteobacterium: a potential bio-catalytic agent for industrial CO2 removal. Chem. Eng. Sci. 138, 575–580. doi: 10.1016/j.ces.2015.07.030
Marcus, J., and Tunnicliffe, V. (2002). Living on the edges of diffuse vents on the Juan de Fuca Ridge. Cah. Biol. Mar. 43, 263–266.
Marcus, J., Tunnicliffe, V., and Butterfield, D. A. (2009). Post-eruption succession of macrofaunal communities at diffuse flow hydrothermal vents on Axial Volcano, Juan de Fuca Ridge, Northeast Pacific. Deep Sea Res. II 56, 1586–1598. doi: 10.1016/j.dsr2.2009.05.004
Marlow, J. J., Steele, J. A., Case, D. H., Connon, S. A., Levin, L. A., and Orphan, V. J. (2014a). Microbial abundance and diversity patterns associated with sediments and carbonates from the methane seep environments of Hydrate Ridge, OR. Front. Mar. Sci. Aquat. Microbiol. 1:44. doi: 10.3389/fmars.2014.00044
Marlow, J. J., Steele, J. A., Ziebis, W., Thurber, A. R., Levin, L. A., and Orphan, V. J. (2014b). Carbonate hosted methanotrophy: an unrecognized methane sink in the deep sea. Nat. Commun. 5, 5094. doi: 10.1038/ncomms6094
Marques, A., and Porteiro, F. (2000). Hydrothermal vent mussel Bathymodiolus sp. (Mollusca: Mytilidae): diet item of Hydrolagus affinis (Pisces: Chimaeridae). Copeia 2000, 806–807. doi: 10.1643/0045-8511(2000)000[0806:HVMBSM]2.0.CO;2
Marsh, L., Copley, J. T., Tyler, P. A., and Thatje, S. (2015). In hot and cold water: differential life-history traits are key to success in contrasting thermal deep-sea environments. J. Anim. Ecol. 84, 898–913doi: 10.1111/1365-2656.12337
Martins, A., Tenreiro, T., Andrade, G., Gadanho, M., Chaves, S., Abrantes, M., et al. (2013). Photoprotective bioactivity present in a unique marine bacteria collection from Portuguese deep sea hydrothermal vents. Mar. Drugs 11, 1506–1523. doi: 10.3390/md11051506
Mason, O. U., Scott, N. M., Gonzalez, A., Robbins-Pianka, A., Bælum, J., Kimbrel, J., et al. (2014). Metagenomics reveals sediment microbial community response to Deepwater Horizon oil spill. ISME J. 8, 1464–1475. doi: 10.1038/ismej.2013.254
McCollom, T., and Shock, E. (1997). Geochemical constraints on chemolithoautotrophic metabolism by microorganisms in seafloor hydrothermal systems. Geochim. Cosmochim. Acta 61, 4375–4391. doi: 10.1016/S0016-7037(97)00241-X
McGillicuddy, D. J., Lavelle, J. W., Thurnherr, A. M., Kosnyrev, V. K., and Mullineaux, L. S. (2010). Larval dispersion along an axially symmetric mid-ocean ridge. Deep Sea Res. Part I 57, 880–892. doi: 10.1016/j.dsr.2010.04.003
McGinnis, D. F., Greinert, J., Artemov, Y., Beaubien, S. E., and Wüest, A. (2006). Fate of rising methane bubbles in stratified waters: how much methane reaches the atmosphere? J. Geophys. Res. 111:C09007. doi: 10.1029/2005JC003183
McMullin, E. R., Hourdez, S., Schaeffer, S. W., and Fisher, C. R. (2003). Phylogeny and biogeography of deep sea vestimentiferan tubeworms and their bacterial symbionts. Symbiosis 34, 1–41.
Menot, L., Galeron, J., Olu, K., Caprais, J.-C., Crassous, P., Khripounoff, A., et al. (2010). Spatial heterogeneity of macrofaunal communities in and near a giant pockmark area in the deep Gulf of Guinea. Mar. Ecol. Evol. Perspect. 31, 78–93. doi: 10.1111/j.1439-0485.2009.00340.x
Metaxas, A. (2004). Spatial and temporal patterns in larval supply at hydrothermal vents on the northwest Pacific Ocean. Limnol. Oceanogr. 49, 1949–1956. doi: 10.4319/lo.2004.49.6.1949
Metaxas, A. (2011). Spatial patterns of larval abundance at hydrothermal vents on seamounts: evidence for recruitment limitation? Mar. Ecol. Prog. Ser. 437, 103–117. doi: 10.3354/meps09283
Metaxas, A., and Kelly, N. E. (2010). Do larval supply and recruitment vary among chemosynthetic environments of the deep sea. PLoS ONE 5:e11646. doi: 10.1371/journal.pone.0011646
Metaxas, A., and Saunders, M. (2009). Quantifying the “bio-” components in biophysical models of larval transport in marine benthic invertebrates: advances and pitfalls. Biol. Bull. 216, 257–272. doi: 10.2307/25548159
Meyer, J. L., Akerman, N. H., Proskurowski, G., and Huber, J. A. (2013). Microbiological characterization of post-eruption “snowblower” vents at Axial Seamount, Juan de Fuca Ridge. Front. Microbiol. 4:153. doi: 10.3389/fmicb.2013.00153
Meyer-Dombard, D. R., Shock, E. L., and Amend, J. P. (2012). Effects of trace element concentrations on culturing thermophiles. Extremophiles 16, 317–331. doi: 10.1007/s00792-012-0432-5
Micheli, F., Peterson, C. H., Mullineaux, L. S., Fisher, C. R., Mills, S. W., Sancho, G., et al. (2002). Predation structures communities at deep-sea hydrothermal vents. Ecol. Monogr. 72, 365–382. doi: 10.1890/0012-9615(2002)072[0365:PSCADS]2.0.CO;2
Millenium Ecosystem Assessment. (2005). Ecosystems and Human Well-Being: Synthesis. Washington, DC: Island Press.
Mullineaux, L. S., Wiebe, P. H., and Baker, E. T. (1995). Larvae of benthic invertebrates in hydrothermal vent plumes over Juan de Fuca Ridge. Mar. Biol. 122, 585–596. doi: 10.1007/BF00350680
Nauhaus, K., Boetius, A., Krüger, M., and Widdel, F. (2002). In vitro demonstration of anaerobic oxidation of methane coupled to sulphate reduction in sediment from a marine gas hydrate area. Environ. Microbiol. 4, 296–305. doi: 10.1046/j.1462-2920.2002.00299.x
Niemann, H., Linke, P., Knittel, K., and MacPherson, E. (2013). Methane-carbon flow into the benthic food web at cold seeps—A case study from the Costa Rica subduction zone. PLoS ONE 8:e74894. doi: 10.1371/journal.pone.0074894
Niemann, H., Lösekann, T., de Beer, D., Elvert, M., Nadalig, T., Knittel, K., et al. (2006). Novel microbial communities of the Haakon Mosby mud volcano and their role as a methane sink. Nature 443, 854–858. doi: 10.1038/nature05227
Nye, V., Copley, J. T., and Tyler, P. A. (2013). Spatial variation in the population structure and reproductive biology of Rimicaris hybisae (Caridea: Alvinocarididae) at hydrothermal vents on the Mid-Cayman spreading centre. PLoS ONE 8:e60319. doi: 10.1371/journal.pone.0060319
Olu-LeRoy, K., Sibuet, M., Fiala-Medioni, A., Gofas, S., Salas, C., Mariotti, A., et al. (2004). Cold seep communities in the deep eastern Mediterranean Sea: composition, symbiosis and spatial distribution on mud volcanoes. Deep Sea Res. I 51, 1915–1936. doi: 10.1016/j.dsr.2004.07.004
Ortmann, A., and Suttle, C. (2005). High abundances of viruses in a deep-sea hydrothermal vent system indicates viral mediated microbial mortality. Deep Sea Res. Part I 52, 1515–1527. doi: 10.1016/j.dsr.2005.04.002
Pape, E., Campinas Bezerra, T. N., Vanneste, H., Heeschen, K., Moodley, L., Leroux, F., et al. (2011). Community structure and feeding preference of nematodes associated with methane seepage at the Darwin mud volcano (Gulf of Cadiz). Mar. Ecol. Prog. Ser. 438, 71–83. doi: 10.3354/meps09278
Peek, A. S., Gustafson, R. G., Lutz, R. A., and Vrijenhoek, R. C. (1997). Evolutionary relationships of deep-sea hydrothermal vent and cold-water seep clams (Bivalvia: Vesicomyidae): results from the mitochondrial cytochrome oxidase subunit I. Mar. Biol. 130, 151–161. doi: 10.1007/s002270050234
Petersen, J. M., Zielinski, F. U., Pape, T., Seifert, R., Moraru, C., Amann, R., et al. (2011). Hydrogen is an energy source for hydrothermal vent symbioses. Nature 476, 176–180. doi: 10.1038/nature10325
Pettit, R. K. (2011). Culturability and secondary metabolite diversity of extreme microbes: expanding contribution of deep sea and deep-sea vent microbes to natural product discovery. Mar. Biotechnol. 13, 1–11. doi: 10.1007/s10126-010-9294-y
Podowski, E. L., Moore, T. S., Zelnio, K. A., and Luther, G. W. III, Fisher, C. R. (2009). Distribution of diffuse flow megafauna in two sites on the Eastern Lau Spreading Center, Tonga. Deep Sea Res. I 56, 2041–2056. doi: 10.1016/j.dsr.2009.07.002
Pohlman, J. W., Bauer, J. E., Waite, W. F., Osburn, C. L., and Chapman, N. R. (2011). Methane hydrate-bearing seeps as a source of aged dissolved organic carbon to the oceans. Nat. Geosci. 4, 37–41. doi: 10.1038/ngeo1016
Pond, D. W., Segonzac, M., Bell, M. V., Dixon, D. R., Fallick, A. E., and Sargent, J. R. (1997). Lipid and lipid carbon stable isotope composition of the hydrothermal vent shrimp Mirocaris fortunata: evidence for nutritional dependence on photosynthetically fixed carbon. Mar. Ecol. Prog. Ser. 157, 221–231. doi: 10.3354/meps157221
Portail, M., Olu, K., Escobar-Briones, E., Caprais, J. C., Menot, L., Waeles, M., et al. (2015). Comparative study of vent and seep macrofaunal communities in the Guaymas Basin. Biogeosciences 12, 5455–5479. doi: 10.5194/bg-12-5455-2015
Quattrini, A., Georgian, S., Byrnes, L., Stevens, A., Falco, R., and Cordes, E. (2013). Niche divergence by deep-sea octocorals in the genus Callogorgia across the continental slope of the Gulf of Mexico. Mol. Ecol. 22, 4123–4140. doi: 10.1111/mec.12370
Quattrini, A. M., Etnoyer, P. J., Doughty, C., English, L., Falco, R., Remon, N., et al. (2014). A phylogenetic approach to octocoral community structure in the deep Gulf of Mexico. Deep Sea Res. II 99, 92–102. doi: 10.1016/j.dsr2.2013.05.027
Quattrini, A. M., Nizinski, M. S., Chaytor, J. D., Demopoulos, A. W. J., Roark, E. B., France, S. C., et al. (2015). Exploration of the canyon-incised continental margin of the northeastern United States reveals dynamic habitats and diverse communities. PLoS ONE 10:e0139904. doi: 10.1371/journal.pone.0139904
Rakowski, C., Magen, C., Bosman, S., Gillies, L., Rogers, K., Chanton, J., et al. (2015). Methane and microbial dynamics in the Gulf of Mexico water column. Front. Mar. Sci. 2:69. doi: 10.3389/fmars.2015.00069
Ramirez-Llodra, E., Tyler, P. A., Baker, M. C., Bergstad, O. A., Clark, M. R., Escobar, E., et al. (2011). Man and the last great wilderness: human impact on the deep sea. PLoS ONE 6:e22588. doi: 10.1371/journal.pone.0022588
Ravaux, J., Zbinden, M., Voss-Foucart, M. F., Compere, P., Goffinet, G., and Gaill, F. (2003). Comparative degradation rates of chitinous exoskeletons from deep-sea environments. Mar. Biol. 143, 405–412. doi: 10.1007/s00227-003-1086-8
Reeburgh, W. S. (2007). Oceanic methane biogeochemistry. Chem. Rev. 107, 486–513. doi: 10.1021/cr050362v
Resing, J. A., Sedwick, P. N., German, C. R., Jenkins, W. J., Moffett, J. W., Sohst, B. M., et al. (2015). Basin-scale transport of hydrothermal dissolved metals across the South Pacific Ocean. Nature 523, 200–203. doi: 10.1038/nature14577
Riou, V., Colaco, A., Bouillon, S., Khripounoff, A., Dando, P., Mangion, P., et al. (2010). Mixotrophy in the deep sea: a dual endosymbiotic hydrothermal mytilid assimilates dissolved and particulate organic matter. Mar. Ecol. Prog. Ser. 405, 187–201. doi: 10.3354/meps08515
Risser, P. G. (1995). The status of the science examining ecotones. Bioscience 45, 318–325. doi: 10.2307/1312492
Ritger, S., Carson, B., and Suess, E. (1987). Methane-derived authigenic carbonates formed by subduction-induced pore-water expulsion along the Oregon/Washington margin. Geol. Soc. Am. Bull. 98, 147–156.
Roberts, H. H., and Carney, R. S. (1997). Evidence of episodic fluid, gas, and sediment venting on the northern Gulf of Mexico continental slope.Econ. Geol.92, 863–879. doi: 10.2113/gsecongeo.92.7-8.863
Rodrigues, C. F. (2009). Macrofaunal Assemblages from Mud Volcanoes in the Gulf of Cadiz. Universidade de Aveiro. PhD thesis, Universidade de Aveiro, Aveiro, Portugal. 314pp + annexes.
Rodrigues, C. F., Hilario, A., and Cunha, M. R. (2013). Chemosymbiotic species from the Gulf of Cadiz (NE Atlantic): distribution, life styles and nutritional patterns. Biogeosciences 10, 2569–2581. doi: 10.5194/bg-10-2569-2013
Roterman, C., Copley, J., Linse, K., Tyler, P., and Rogers, A. (2013). The biogeography of the yeti crabs (Kiwaidae) with notes on the phylogeny of the Chirostyloidea (Decapoda: Anomura). Proc. R. Soc. Lond. B 280, 20130718. doi: 10.1098/rspb.2013.0718
Sahling, H., Galkin, S. V., Salyuk, A., Greinert, J., Foerstel, H., Piepenburg, D., et al. (2003). Depth-related structure and ecological significance of cold-seep communities – a case study from the Sea of Okhotsk. Deep Sea Res. Part I Oceanogr. Res. Papers 50, 1391–1409. doi: 10.1016/j.dsr.2003.08.004
Sahling, H., Rickert, D., Lee, R. W., Linke, P., and Suess, E. (2002). Macrofaunal community structure and sulfide flux at gas hydrate deposits from the Cascadia convergent margin, NE Pacific. Mar. Ecol. Prog. Ser. 231, 121–138. doi: 10.3354/meps231121
Saldanha, L., and Biscoito, M. (1997). Fishes from the Lucky Strike and Menez Gwen hydrothermal vent sites (Mid-Atlantic Ridge). Bol. Museu Mun. Funch. 49, 189–206.
Sarrazin, J., and Juniper, S. K. (1999). Biological characteristics of a hydrothermal edifice mosaic community. Mar. Ecol. Prog. Ser. 185, 1–19. doi: 10.3354/meps185001
Sarrazin, J., Robigou, V., Juniper, S. K., and Delaney, J. (1997). Biological and geological dynamics over four years on a high-temperature sulfide structure at the Juan de Fuca Ridge hydrothermal observatory. Mar. Ecol. Prog. Ser. 153, 5–24. doi: 10.3354/meps153005
Schrenk, M. O., Kelley, D. S., Delaney, J. R., and Baross, J. A. (2003). Incidence and diversity of microorganisms within the walls of an active deep-sea sulfide chimney. Appl. Environ. Microbiol., 69, 3580–3592. doi: 10.1128/AEM.69.6.3580-3592.2003
Sellanes, J., Pedraza, M. J., and Zapata Hernandez, G. (2012). Las areas de filtracion de metano constituyen zonas de agregacion del bacalao de profundidad (Dissostichus eleginoides) frente a Chile central. Lat. Am. J. Aquat. Res. 40, 980–991. doi: 10.3856/vol40-issue4-fulltext-14
Sellanes, J., Quiroga, E., and Neira, C. (2008). Megafauna community structure and trophic relationships at the recently discovered Concepcion Methane Seep Area, Chile, 36° S. ICES J. Mar. Sci. 65, 1102–1111. doi: 10.1093/icesjms/fsn099
Shackelford, R., and Cowen, J. P. (2006). Transparent exopolymer particles (TEP) as a component of hydrothermal plume particle dynamics. Deep Sea Res. Part I 53, 1677–1694. doi: 10.1016/j.dsr.2006.08.001
Shank, T. M., Fornari, D. J., Von Damm, K. L., Lilley, M. D., Haymon, R. M., and Lutz, R. A. (1998). Temporal and spatial patterns of biological community development at nascent deep-sea hydrothermal vents (9°50'N, East Pacific Rise). Deep Sea Res. Part II. 45, 465–515. doi: 10.1016/S0967-0645(97)00089-1
Shea, K., Metaxas, A., Young, C. R., and Fisher, C. R. (2008). Processes and interactions in macrofaunal assemblages at hydrothermal vents: a modeling perspective, in Magma to Microbe, eds R. P. Lowell, J. S. Seewald, A. Metaxas, and M. R. Perfit (Washington, DC: American Geophysical Union), 259–274. doi: 10.1029/178GM13
Sheik, C. S., Anantharaman, K., Breier, J. A., Sylvan, J. B., Edwards, K. J., and Dick, G. J. (2014). Spatially resolved sampling reveals dynamic microbial communities in rising hydrothermal plumes across a back-arc basin. ISME J. 9, 1434–1445. doi: 10.1038/ismej.2014.228
Sibuet, M., and Olu, K. (1998). Biogeography, biodiversity and fluid dependence of deep-sea cold-seep communities at active and passive margins. Deep Sea Res. Part II 45, 517–567. doi: 10.1016/S0967-0645(97)00074-X
Skarke, A., Ruppel, C., Kodis, M., Brothers, D., and Lobecker, E. (2014). Widespread methane leakage from the sea floor on the northern US Atlantic margin. Nat. Geosci. 7, 657–661doi: 10.1038/ngeo2232
Skebo, K., Tunnicliffe, V., Berdeal, I. G., and Johnson, H. P. (2006). Spatial patterns of zooplankton and nekton in a hydrothermally active axial valley on Juan de Fuca Ridge. Deep Sea Res. Part I Oceanogr. Res. Papers 53, 1044–1060. doi: 10.1016/j.dsr.2006.03.001
Smith, C., Kukert, H., Wheatcroft, R., Jumars, P., and Deming, J. (1989). Vent fauna on whale remains. Nature 341, 27–28. doi: 10.1038/341027a0
Smith, C. R. (2006). Bigger is better: the role of whales as detritus in marine ecosystems, in Whales, Whaling, and Ocean Ecosystems, eds J. A. Estes, D. P. DeMaster, D. F. Doak, T. M. Williams, and R. Brownell Jr. (Berkeley: University of California Press), 286–300.
Smith, C. R., and Baco, A. R. (2003). Ecology of whale falls at the deep-sea floor. Oceanogr. Mar. Biol. 41, 311–354.
Somero, G. N., Childress, J. J., and Anderson, A. E. (1989). Transport, metabolism, and detoxification of hydrogen sulfide in animals from sulfide-rich marine environments. CRC Crit. Rev. Aquatic Sci. 1, 591–614.
Sorokin, Y. I., Sorokin, P. Y., and Zakuskina, O. Y. (1998). Microplankton and its functional activity in zones of shallow hydrotherms in the Western Pacific. J. Plankton Res. 20, 1015–1031. doi: 10.1093/plankt/20.6.1015
Southward, E. C., Andersen, A. C., and Hourdez, S. (2011). Lamellibrachia anaximandri n. sp., a new vestimentiferan tubeworm (Annelida) from the Mediterranean, with notes on frenulate tubeworms from the same habitat. Zoosystema 33, 245–279. doi: 10.5252/z2011n3a1
Stakes, D. S., Orange, D., Paduan, J. B., Salamy, K. A., and Maher, N. (1999). Cold-seeps and authigenic carbonate formation in Monterey Bay, California. Mar. Geol. 159, 93–109. doi: 10.1016/S0025-3227(98)00200-X
Staudigel, H., Hart, S. R., Pile, A., Bailey, B. E., Baker, E. T., Brooke, S., et al. (2006). Vailulu'u Seamount, Samoa: life and death on an active submarine volcano. Proc. Natl. Acad. Sci. U.S.A. 103, 6448–6453. doi: 10.1073/pnas.0600830103
Stevens, C. J., Juniper, S. K., Limen, H., Pond, D. W., Metaxas, A., and Gelinas, Y. (2015). Obligate hydrothermal vent fauna at East Diamante submarine volcano (Mariana Arc) exploit photosynthetic and chemosynthetic carbon sources. Mar. Ecol. Prog. Ser. 525, 25–39. doi: 10.3354/meps11229
Stiller, J., Rousset, V., Pleijel, F., Chevaldonné, P., Vrijenhoek, R. C., and Rouse, G. W. (2013). Phylogeny, biogeography and systematics of hydrothermal vent and methane seep Amphisamytha (Ampharetidae, Annelida), with descriptions of three new species. Syst. Biodivers. 11, 35–65. doi: 10.1080/14772000.2013.772925
Tagliabue, A., Bopp, L., Dutay, J.-C., Bowie, A. R., Chever, F., Jean-Baptiste, P., et al. (2010). Hydrothermal contribution to the oceanic dissolved iron inventory. Nat. Geosci. 3, 252–256. doi: 10.1038/ngeo818
Thomson, R. E., Mihaly, S. F., Rabinovich, A. B., Mcduff, R. E., Veirs, S. R., and Stahr, F. R. (2003). Constrained circulation at Endeavour Ridge facilitates colonization by vent larvae. Nature 424, 545–549. doi: 10.1038/nature01824
Thornhill, D., Struck, T. H., Ebbe, B., Lee, R. W., Mendoza, G. F., Levin, L. A., et al. (2012). Adaptive radiation in extremophilic Dorvilleidae (Annelida): diversification of a single colonizer or multiple independent lineages? Ecol. Evol. 2, 1958–1970. doi: 10.1002/ece3.314
Thubaut, J., Puillandre, N., Faure, B., Cruaud, C., and Samadi, S. (2013). The contrasted evolutionary fates of deep-sea chemosynthetic mussels (Bivalvia, Bathymodiolinae). Ecol. Evol. 3, 4748–4766. doi: 10.1002/ece3.749
Thurber, A. R., Kröger, K., Neira, C., Wiklund, H., and Levin, L. A. (2010). Stable isotope signatures and methane use by New Zealand cold seep benthos. Mar. Geol. 272, 260–269. doi: 10.1016/j.margeo.2009.06.001
Thurber, A. R., Levin, L. A., Orphan, V. J., and Marlow, J. (2012). Archaea in metazoan diets: implications for food webs and biogeochemical cycling. ISME J. 6, 1602–1612. doi: 10.1038/ismej.2012.16
Thurber, A. R., Levin, L. A., Rowden, A., Sommer, S., Linke, P., and Kroger, K. (2013). Microbes, macrofauna and methane: a novel seep community fueled by aerobic methanotrophy. Limnol. Oceanogr. 58, 1640–1656. doi: 10.4319/lo.2013.58.5.1640
Thurber, A. R., Sweetman, A. K., Narayanaswamy, B. E., Jones, D. O. B., Ingels, J., and Hansman, R. L. (2014). Ecosystem function and services provided by the deep sea. Biogeosciences 11, 3941–3963. doi: 10.5194/bg-11-3941-2014
Tivey, M. K., Humphris, S. E., Thompson, G., Hannington, M. D., and Rona, P. A. (1995). Deducing patterns of fluid-flow and mixing within the tag active hydrothermal mound using mineralogical and geochemical data. J. Geophys. Res. Solid Earth 100,527-12,555. doi: 10.1029/95jb00610
Toner, B. M., Fakra, S. C., Manganini, S. J., Santelli, C. M., Marcus, M. A., Moffett, J. W., et al. (2009). Preservation of iron (II) by carbon-rich matrices in a hydrothermal plume. Nat. Geosci. 2, 197–201. doi: 10.1038/ngeo433
Torres, M. E., McManus, J., Hammond, D. E., de Angelis, M. A., Heeschen, K. U., Colbert, S. L., et al. (2002). Fluid and chemical fluxes in and out of sediments hosting methane hydrate deposits on Hydrate Ridge, OR. I: hydrological provinces. Earth Planet. Sci. Lett. 201, 525–540. doi: 10.1016/S0012-821X(02)00733-1
Treude, T., Kiel, S., Linke, P., Peckmann, J., and Goedert, J. L. (2011). Elasmobranch egg capsules associated with modern and ancient cold seeps: a nursery for marine deep-water predators. Mar. Ecol. Prog. Ser. 437, 175–181. doi: 10.3354/meps09305
Tsurumi, M., and Tunnicliffe, V. (2001). Characteristics of a hydrothermal vent assemblage on a volcanically active segment of Juan de Fuca Ridge, Northeast Pacific. Can. J. Fish. Aquatic Sci. 58, 530–542. doi: 10.1139/f01-005
Tsurumi, M., and Tunnicliffe, V. (2003). Tubeworm-associated communities at hydrothermal vents on the Juan de Fuca Ridge, northeast Pacific. Deep Sea Res. Part I 50, 611–629. doi: 10.1016/S0967-0637(03)00039-6
Tunnicliffe, V., Botros, M., De Burgh, M. E., Dinet, A., Johnson, H. P., Juniper, S. K., et al. (1986). Hydrothermal vents of Explorer Ridge, northeast Pacific. Deep Sea Res. 33, 401–412. doi: 10.1016/0198-0149(86)90100-7
Tunnicliffe, V., Embley, R. W., Holden, J. F., Butterfield, D. A., Massoth, G. J., and Juniper, S. K. (1997). Biological colonization of new hydrothermal vents following an eruption on Juan de Fuca Ridge. Deep Sea Res. Part I 44, 1627–1644. doi: 10.1016/S0967-0637(97)00041-1
Tunnicliffe, V., and Jensen, R. G. (1987). Distribution and behaviour of the spider crab Macroregonia macrochira Sakai (Brachyura) around the hydrothermal vents of the northeast Pacific. Can. J. Zool. 65, 2443–2449. doi: 10.1139/z87-369
Tunnicliffe, V., Juniper, S. K., and Sibuet, M. (2003). Reducing environments of the deep-sea floor, in Ecosystems of the Deep Oceans, ed P. A. Tyler. (Amsterdam: Elsevier), 81–110.
Tunnicliffe, V., Mcarthur, A. G., and Mchugh, D. (1998). A biogeographical perspective of the deep-sea hydrothermal vent fauna. Adv. Mar. Biol. 34, 353–442. doi: 10.1016/S0065-2881(08)60213-8
Tunnicliffe, V., Tyler, J., and Dower, J. F. (2013). Population ecology of the tonguefish Symphurus thermophilus (Pisces; Pleuronectiformes; Cynoglossidae) at sulphur-rich hydrothermal vents on volcanoes of the northern Mariana Arc. Deep Sea Res. Part II. 92, 172–182. doi: 10.1016/j.dsr2.2013.01.026
Tyler, P. A., and Young, C. M. (1999). Reproduction and dispersal at vents and cold seeps. J. Mar. Biol. Associat. 79, 193–208. doi: 10.1017/S0025315499000235
UNESCO (2009). Global Open Oceans and Deep Seabed (GOODS)—Biogeographic Classification. Paris: UNESCO. UNESCO-IOC (IOC Technical Series, 84).
Valentine, D. L. (2011). Emerging topics in marine methane biogeochemistry. Ann. Rev. Mar. Sci. 3, 147–171. doi: 10.1146/annurev-marine-120709-142734
Van Campenhout, J., Derycke, S., Tchesunov, A., Portnova, D., and Vanreusel, A. (2014). The Halomonhystera disjuncta population is homogeneous across the Håkon Mosby mud volcano (Barents Sea) but is genetically differentiated from its shallow-water relatives. J. Zool. System. Evolution. Res. 52, 203–216. doi: 10.1111/jzs.12054
Van Dover, C. (2000). The Ecology of Deep-Sea Hydrothermal Vents. Princeton, NJ: Princeton University Press.
Van Dover, C., Franks, P. J. S., and Ballard, R. D. (1987). Prediction of hydrothermal vent locations from distributions of brachyuran crabs. Limnol. Oceanogr. 32, 1006–1010. doi: 10.4319/lo.1987.32.4.1006
Van Dover, C. L. (2002). Community structure of mussel beds at deep-sea hydrothermal vents. Mar. Ecol. Prog. Ser. 230, 137–158. doi: 10.3354/meps230137
Van Dover, C. L., Aharon, P., Bernhard, J. M., Caylor, E., Doerries, M., Flickinger, W., et al. (2003). Blake Ridge methane seeps: characterization of a soft-sediment, chemo synthetically based ecosystem. Deep Sea Res. Part I Oceanogr. Res. Papers 50, 281–300. doi: 10.1016/S0967-0637(02)00162-0
Van Dover, C. L., German, C. R., Speer, K. G., Parson, L. M., and Vrijenhoek, R. C. (2002). Evolution and biogeography of deep-sea vent and seep invertebrates Science 295, 1253–1257. doi: 10.1126/science.1067361
Van Gaever, S., Galéron, J., Sibuet, M., and Vanreusel, A. (2009). Deep-sea habitat heterogeneity influence on meiofaunal communities in the Gulf of Guinea. Deep Sea Res. Part I Oceanogr. Res. Papers 56, 2259–2269. doi: 10.1016/j.dsr2.2009.04.008
Van Gaever, S., Moodley, L., de Beer, D., and Vanreusel, A. (2006). Meiobenthos at the Arctic Håkon Mosby Mud Volcano, with a parental-caring nematode thriving in sulphide-rich sediments. Ecol. Progr. Ser. 321, 143–155. doi: 10.3354/meps321143
Vanreusel, A., Andersen, A., Boetius, A., Connelly, D., Cunha, M., Decker, C., et al. (2009). Biodiversity of cold seep ecosystems along the European margins. Oceanography 22, 110–127. doi: 10.5670/oceanog.2009.12
Vereshchaka, A., and Vinogradov, G. (1999). Visual observations of the vertical distribution of plankton throughout the water column above Broken Spur vent field, Mid-Atlantic Ridge. Deep Sea Res. Part I 46, 1615–1632. doi: 10.1016/S0967-0637(99)00018-7
Voight, J. R. (2000). A deep-sea octopus (Graneledone cf. boreopacifica) as a shell-crushing hydrothermal vent predator. J. Zool. 252, 335–341. doi: 10.1111/j.1469-7998.2000.tb00628.x
Von Damm, K. L., Edmond, J. M., Grant, B., Measures, C. I., Walden, B., and Weiss, R. F. (1985). Chemistry of submarine hydrothermal solutions at 21°N, East Pacific Rise. Geochim. Cosmochim. Acta 49, 2197–2220. doi: 10.1016/0016-7037(85)90222-4
Vrijenhoek, R. C. (2013). On the instability and evolutionary age of deep-sea chemosynthetic communities. Deep Sea Res. Part II 92, 189–200. doi: 10.1016/j.dsr2.2012.12.004
Wagner, J. K. S., McEntee, M. H., Brothers, L. L., German, C. R., Kaiser, C. L., Yoerger, D. R., et al. (2013). Cold-seep habitat mapping: high-resolution spatial characterization of the Blake Ridge Diapir seep field. Deep Sea Res. Part II 92, 183–188. doi: 10.1016/j.dsr2.2013.02.008
Watanabe, H., Fujikura, K., Kojima, S., Miyazaki, J. I., and Fujiwara, Y. (2010). Japan: vents and seeps in close proximity, in The Vent and Seep Biota: Aspects from Microbes to Ecosystems, ed S. Kiel (Dordrecht: Springer), 379–401. doi: 10.1007/978-90-481-9572-5_12
Watling, L., Guinotte, J., Clark, M. R., and Smith, C. R. (2013). A proposed biogeography of the deep ocean floor. Prog. Oceanogr. 111, 91–112. doi: 10.1016/j.pocean.2012.11.003
Wiebe, P. H., Copley, N., Van Dover, C. L., Tamse, A., and Manrique, F. (1988). Deep-water zooplankton of the Guaymas Basin hydrothermal vent field. Deep Sea Res. 35, 985–1013. doi: 10.1016/0198-0149(88)90072-6
Wiklund, H., Altamira, I., Glober, A., Smith, C., Baco, A., and Dahlgren, T. (2012). Systematics and biodiversity of Ophryotrocha (Annelida, Dorvilleidae) with descriptions of six new species from deep-sea whale-fall and wood-fall habitats in the north-east Pacific. System. Biodiv. 10, 243–259. doi: 10.1080/14772000.2012.693970
Winn, C. D., Karl, D. M., and Massoth, G. J. (1986). Microorganisms in deep-sea hydrothermal plumes. Nature 320, 744–746. doi: 10.1038/320744a0
Yang, J. S., Lu, B., Chen, D. F., Yu, Y., Yang, F., Nagasawa, H., et al. (2013). When did decapods invade hydrothermal vents? Clues from the Western Pacific and Indian Oceans. Mol. Biol. Evolut. 30, 305–309. doi: 10.1093/molbev/mss224
Young, C. M., He, R., Emlet, R. B., Li, Y., Qian, H., Arellano, S. M., et al. (2012). Dispersal of deep-sea larvae from the intra-American seas: simulations of trajectories using ocean models. Integr. Comp. Biol. 52, 483–496. doi: 10.1093/icb/ics090
Yücel, M., Gartman, A., Chan, C. S., and Luther, G. W. (2011). Hydrothermal vents as a kinetically stable source of iron-sulphide-bearing nanoparticles to the ocean. Nat. Geosci. 4, 367–371. doi: 10.1038/ngeo1148
Keywords: chemosynthetic ecosystem, ecosystem services, connectivity, deep sea, ecotone, elemental cycling, habitat use, trophic interactions
Citation: Levin LA, Baco AR, Bowden DA, Colaco A, Cordes EE, Cunha MR, Demopoulos AWJ, Gobin J, Grupe BM, Le J, Metaxas A, Netburn AN, Rouse GW, Thurber AR, Tunnicliffe V, Van Dover CL, Vanreusel A and Watling L (2016) Hydrothermal Vents and Methane Seeps: Rethinking the Sphere of Influence. Front. Mar. Sci. 3:72. doi: 10.3389/fmars.2016.00072
Received: 03 March 2016; Accepted: 25 April 2016;
Published: 19 May 2016.
Edited by:
Cinzia Corinaldesi, Polytechnic University of Marche, ItalyReviewed by:
Rachel Narehood Austin, Columbia University in the City of New York, USACopyright © 2016 Levin, Baco, Bowden, Colaco, Cordes, Cunha, Demopoulos, Gobin, Grupe, Le, Metaxas, Netburn, Rouse, Thurber, Tunnicliffe, Van Dover, Vanreusel and Watling. This is an open-access article distributed under the terms of the Creative Commons Attribution License (CC BY). The use, distribution or reproduction in other forums is permitted, provided the original author(s) or licensor are credited and that the original publication in this journal is cited, in accordance with accepted academic practice. No use, distribution or reproduction is permitted which does not comply with these terms.
*Correspondence: Lisa A. Levin, bGxldmluQHVjc2QuZWR1
Disclaimer: All claims expressed in this article are solely those of the authors and do not necessarily represent those of their affiliated organizations, or those of the publisher, the editors and the reviewers. Any product that may be evaluated in this article or claim that may be made by its manufacturer is not guaranteed or endorsed by the publisher.
Research integrity at Frontiers
Learn more about the work of our research integrity team to safeguard the quality of each article we publish.