- Department of Biomedical Science and Environmental Biology, Kaohsiung Medical University, Kaohsiung, Taiwan
Nanotoxicity has become of increasing concern since the rapid development of metal nanoparticles (NPs). Aquatic nanotoxicity depends on crucial qualitative and quantitative properties of nanomaterials that induce adverse effects on subcellular, tissue, and, organ level. The dose-response effects of size-dependent metal NPs, however, are not well investigated in aquatic organisms. In order to determine the uptake and elimination rate constants for metal NPs in the metabolically active/detoxified pool of tissues, a one-compartmental toxicokinetic model can be applied when subcellular partitioning of metal NPs data would be available. The present review is an attempt to describe the nano-characteristics of toxicokinetics and subcellular partitioning on aquatic organisms with the help of the mechanistic modeling for NP size-dependent physiochemical properties and parameters. Physiologically-based pharmacokinetic (PBPK) models can provide an effective tool to estimate the time course of NP accumulation in target organs and is useful in quantitative risk assessments. NP accumulation in fish should take into account different effects of different NP sizes to better understand tissue accumulative capacities and dynamics. The size-dependent NP partition coefficient is a crucial parameter that influences tissue accumulation levels in PBPK modeling. Further research is needed to construct the effective systems-level oriented toxicokinetic model that can provide a useful tool to develop quantitatively the robustly approximate relations that convey a better insight into the impacts of environmental metal NPs on subcellular and tissue/organ responses in aquatic organisms.
Introduction
Nanotoxicity has become of increasing concern since the rapid development of metal nanoparticles (NPs) and their wide use in industrial and biomedical applications. Metal nanomaterials have been widely used in drug delivery, sunscreens, electronics, computer processors, conductive coatings, anti-aging creams, and skin conditioners (Shaw and Handy, 2011). These broad applications have led to direct or indirect release of NP into environment including aquatic and oceanic ecosystems. The large specific surface areas and reactive activity of nanomaterial properties makes them efficient in practical applications. However, they also reported to induce different toxic modes of action depending on their nano-characteristics, especially related to particle size (Shaw and Handy, 2011).
In view of the biological factors, nanotoxicity to aquatic organisms is exerted through two mechanisms: (i) the accumulative capacity of NPs through absorption, distribution, metabolism, and excretion (i.e., toxicokinetics, TK) and (ii) the accumulated nanomaterial-causing adverse effects at the site of action or target site (i.e., toxicodynamics, TD). When chemical induces the adverse effect to the organism, the critical effect concentration is not dependent on the external concentration. The appropriate determination should be involved the toxic properties of chemical, exposure pathways, endpoint of toxic effect response, and internal/external effect concentration. Toxicokinetic mechanism represents the time course of chemical and metabolite level in the target organs/tissues of aquatic organism, quantifying the relationships between external concentration of chemical in aquatic ecosystems and internal dose of chemical in target organs or tissues of exposed aquatic organism.
Wang and Rainbow (2006) suggested that specific subcellular distribution of chemicals could reflect their toxic reactions. Recently, most studies have shown negative effects and tissue accumulations of metal NPs among aquatic organisms (Zhao et al., 2011; Farmen et al., 2012; Shaw et al., 2012; Maes et al., 2014). However, little is known, about the subcellular distribution and the mechanistic modeling for describing the nano-characteristics of toxicokinetics and subcellular partitioning in aquatic organisms, especially for NP size-dependent physiochemical properties and parameters.
Here we explore how the size-dependent metal NP affects aquatic ecotoxicities based on subcellular and tissue residues. We particularly demarcate challenges provided by NP toxico- and pharmacokinetic modeling based on NP size-specific toxicity at the subcellular, tissue, and physiological levels. The present review is an attempt to describe the nano-characteristics of toxicokinetics and subcellular partitioning in aquatic organisms with the help of the mechanistic modeling for NP size-dependent physiochemical properties and parameters.
Size-dependent Toxicity
Most studies indicate that nanotoxicity depends on size, surface reactivity, crystallinity, aggregation, and dissolution of nanomaterials (Kasemets et al., 2009; Zhao et al., 2011). However, Zhao et al. (2007) showed that particle size is directly correlated with relevant factors that affect nanotoxicity in organisms. The characteristics of waterborne metal NP have been reported in several studies by using the single particle sized surface area, aggregation, and zeta potential (Zhao et al., 2011; Shaw et al., 2012; Isani et al., 2013). However, each study has inherent limitations about how the different NP sizes can induce a variety of particle properties that subsequently affect nanotoxicity.
How size of waterborne NP affects their toxicity on aquatic organisms remains an important challenge. Most studies focus on single size of NP to construct the relationship between metal NP concentration and their adverse effects. Recently, several studies have paid attention on investigation of behavior, mortality, development, and histopathological effects of iron, zinc, copper, and titanium NPs on zebrafish, medaka, and rainbow trout (Griffitt et al., 2008, 2009; Chen et al., 2012, 2013; Shaw et al., 2012; Isani et al., 2013).
To date, the studies regarding the physiological effects of nano-sized waterborne CuNP on fish included 26.7 nm-zebrafish (Danio rerio), 20–40 nm-carp (Cyprinus carpio), and 87- and 164 nm-rainbow trout (Oncorhynchus mykiss) systems (Griffitt et al., 2008, 2009; Zhao et al., 2011; Shaw et al., 2012; Al-Bairuty et al., 2013; Isani et al., 2013). Dose-response effects of size-dependent metal NPs were also found in in vitro osteoblastic MC3T3-E1 cells and PC12 pheochromocytoma cell studies, indicating that the smaller metal NPs remained more toxic than larger ones on cell toxicity (Carlson et al., 2008; Kim et al., 2012; Prasad et al., 2013). Therefore, it is suggested that nanotoxicological studies should take into account size effects and surface properties of NP.
Modeling of Subcellular Partitioning
Previous studies indicated that subcellular distribution can be used to describe the complex binding of metal ions in different subcellular compartments with different metal ion-binding ligands (Wang and Rainbow, 2006; Buchwalter et al., 2008; Seebaugh and Wallace, 2009; Huang et al., 2010). They pointed out that metal ions binding to target subcellular compartments could reflect the metal toxicity and detoxification. The critical sites of toxic action in the subcellular fraction include a metabolically active pool (MAP) comprising organelles and heat sensitive proteins and a metabolically detoxified pool (MDP) comprising metal rich granules and metallothionein-like proteins. The MAP is the target compartment that induces toxic effects, whereas MDP controls the detoxifying capacity. However, the subcellular partitioning of size-dependent NP concentration has not been well investigated on toxic effects to aquatic organisms. There are few studies on assessing the subcellular effects of waterborne metal NPs in fish liver. Recently, Fan et al. (2013) indicated that NP concentration distributions in metal rich granules were the main target for ZnNPs, whereas Zn concentration in organelles increased significantly after Zn ion exposure.
If subcellular partitioning data of metal NP are available, a one-compartmental toxicokinetic model can be used to determine the size-dependent uptake and elimination rate constants of metal NPs in MAP or MDP of tissue. On the other hand, the estimated key toxicokinetic parameters of size-dependent metal NPs are capable of describing NP accumulation kinetics over time in MAP or MDP. Croteau and Luoma (2009) have recently proposed a metal influx threshold (MIT) concept to determine the kinetics of detoxification. MIT occurs when metal influx is equal or larger than the combined rate of metal loss in MAP exceeding the MAP influx threshold, resulting in detoxification (Figure 1) (Croteau and Luoma, 2009). In light of the MIT concept, the size-dependent metal NP detoxification in aquatic organisms can be fully quantified.
Moreover, several researches indicated that metal subcellular distributions controlled the assimilation efficiencies of metals in predators fed with different prey (i.e., metal trophic transfer level) (Dubois and Hare, 2009; He et al., 2010; Guo et al., 2013). This implicated that metal subcellular distribution of aquatic fish could also influence the human health risk from metal-contaminated seafood consumptions due to the trophic transfer of metals. The main subcellular distributions of trophically available metals usually include organelles, heat sensitive proteins, and metallothionein-like proteins that can also be used to predict the trophic transfer fraction (Wallace et al., 2003). Cheung and Wang (2005) indicated that different metal characteristics could be concentrated in insoluble or soluble fractions. However, to our knowledge, there is no available information concerning NP subcellular-related trophic transfer level of freshwater fish.
Shaw et al. (2012) and Zhao et al. (2011) indicated that accumulative levels have been found in different target tissues under waterborne metal NPs and ion exposures. The subcellular distribution could provide more clear information for understanding the abilities of toxicity and detoxification of target tissues between nano- and non-nano metal exposures. If the critical subcellular distribution of metal NPs would be available, the cellular causes of biomagnification in food chains could be better understood.
Physiologically-based Pharmacokinetic Modeling-Kinetics of Tissue Distribution
Shaw et al. (2012) and Isani et al. (2013) indicated that gill and liver of rainbow trout were the target organs for accumulating CuNP. De Boeck et al. (2003), Peyghan et al. (2003), and Soedarini et al. (2012) pointed out that liver was the key site for homeostasis of accumulating Cu. However, gill could uptake higher CuNP levels than liver (Griffitt et al., 2007; Zhao et al., 2011). Notably, Kashiwada (2006) indicated that NP could be capable of passing the blood-brain barrier and causing brain damage in medaka. Health risk is significantly related to the potential accumulation of NPs in individual factors. Until now, most studies in dealing with NP accumulation in aquatic organisms focused on waterborne exposure and tissue- specific accumulation (Shaw et al., 2012; Isani et al., 2013). They did not investigate the internal NP accumulation based on systemic circulation to reach the tissues and organs. The NP distributions within different organ systems by different exposure routes need to be identified. Here, the toxicokinetic approaches incorporated with physiological and biochemical parameters are capable of describing the complete dynamics of NPs, especially for size-dependent nanotoxic effect under sublethal exposures.
Physiologically-based pharmacokinetic (PBPK) models can provide an effective tool to estimate the time course of chemical accumulation in target organs/tissues of fish and could be useful in quantitative risk assessment. The PBPK models can integrate the physiological structures of organism and physicochemical properties of toxicants and can quantitatively describe the kinetic processes of absorption, distribution, metabolism, and excretion (Krishnan and Peyret, 2009). The PBPK models have been used in several aquatic fish species subjected to various chemicals; e.g., cadmium in rainbow trout (Salmo gairdneri), dioxin in brook trout Salvelinus fontinalis (Nichols et al., 1998), 1,1,2,2-tetrachloroethane, pentachloroethane, and hexachloroethane in lake trout (Salvelinus namaycush) (Lien et al., 2001), and arsenic and copper in tilapia (Oreochromis mossambicus) (Liao et al., 2005; Chen and Liao, 2014). However, those developed PBPK models did not investigate the NP accumulation in fish.
In terms of metal NPs, a PBPK model is generally based on the following assumptions: (i) each compartment is well-mixed and homogenous in the distribution of toxicants and their chemical speciation, (ii) all transport processes are mediated by blood flow, (iii) uptake and elimination processes are described as a first-order reaction, and (iv) there is a complete equilibrium of metal NP between the dissolved phase and tissue. The essence of almost all PBPK models can be described by a state-space equation as:
where {Ci(t)} is the state variable vector describing metal NPs in each target tissue (μg g−1), {u(t)} is the input vector of metal NPs in the aquatic environment (μg L−1), [A] is the state matrix describing the exchange rate between target tissues (L d−1), and [B] is the constant input matrix describing the exchange rate into target tissues (L d−1). The principle feature of the tissue compartment model is schematized in Figure 2.
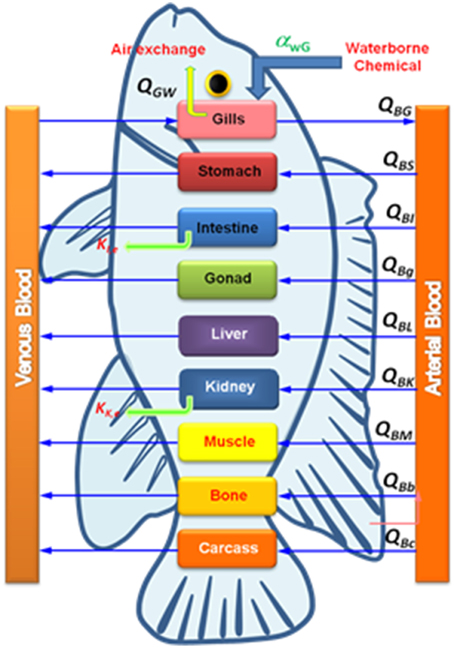
Figure 2. Scheme of a PBPK model for NPs in fish where QGw is the gill-water exchange rate, QBi is the blood-tissue exchange rate, and ki is the elimination rate.
The essential physiological and physicochemical parameters are needed to be estimated for constructing a metal NP-fish PBPK model. Physiological parameters include blood volume and organ/tissue weight that can be obtained from experimental data in that exchange rates between tissues and blood compartment are calculated as the fraction of cardiac output. One of the crucial physicochemical parameter is the tissue partition coefficientdefined as the area under the curve (AUC) of metal NP in tissue/AUC of metal NP in blood for specific tissue. The partition coefficient affects tissue accumulation levels in a PBPK model (Brown et al., 1997).
Zhao et al. (2011) pointed out that the whole body accumulation of waterborne CuNPs in the size range 20–40 nm was higher than that of 1000–2000 nm Cu bulk exposure. Thus, NPs accumulation in fish should take into account the different nano-sized effects to better understand the tissue accumulative capacities and their dynamics. Lankveld et al. (2010) performed the partition coefficients of size-dependent silver NP (20, 80, and 100 nm) in a rat PBPK model. However, this model did not reveal the relationship between NP size and tissue accumulation. To date, there are limited PBPK parameters for metal NPs in aquatic organisms. Thus, we need to build a suitable model involving particle size, surface charge, and tissue kinetic characteristics that could well-describe the toxicokinetics of metal NPs in aquatic organisms.
As there are very few studies on an integrated approach using metal NPs characteristics, physiological, physicochemical, and subcellular partitioning related parameters to assess health risks in the aquatic environment. Specific parameters are required to be used and quantified in experimental approaches, including environmental to individual, organ-system to subcellular levels. Thorough understanding of NPs at tissue, cell and subcellular level is essentially required in the light of differential diffusion property of different NPs through blood-brain barrier.
Conclusion
A comprehensive evaluation of aquatic nanotoxicology should perform suitable experiments to obtain baseline data in order to develop the mechanistic models for predicting the potential accumulation and toxicities of NPs. It is equally important to determine the relationships among metal NPs exposure, tissue accumulation, and the fractions of the MAP and MDP in aquatic organisms. The mechanisms representing adverse effects of metal NPs on aquatic organisms usually include three elements: (i) size-dependent exposure, (ii) toxicological susceptibility, and (iii) recovery capacity. Generally, toxicological susceptibility includes bioaccumulation (uptake and elimination) and coping mechanism (detoxification, storage, and antioxidant).
To date there are limited studies that pursue an integrated approach by using metal NPs characteristics, physiological, physicochemical, and subcellular partitioning related parameters to assess health risks in the aquatic environment. Specific parameters should be used and quantified in experimental approaches that include environmental to individual, organ-system to subcellular levels. Further research is needed to employ the effective systems-level oriented toxicokinetic model that determines in a fully quantitative way to develop robustly approximate relations, leading to new nanoecotoxicological modeling opportunities. These efforts open up new possibilities for assessing how environmental metal NPs characteristics affect subcellular and tissue/organ responses in aquatic organisms.
Conflict of Interest Statement
The author declares that the research was conducted in the absence of any commercial or financial relationships that could be construed as a potential conflict of interest.
Acknowledgments
This study was supported by a grant from the Ministry of Science and Technology of the Republic of China (MOST 103-2313-B-037-003; MOST 104-2311-B-037-001-MY2).
References
Al-Bairuty, G. A., Shaw, B. J., Handy, R. D., and Henry, T. B. (2013). Histopathological effects of waterborne copper nanoparticles and copper sulphate on the organs of rainbow trout (Oncorhynchus mykiss). Aquat. Toxicol. 126, 104–115. doi: 10.1016/j.aquatox.2012.10.005
Brown, R. P., Delp, M. D., Lindstedt, S. L., Rhomberg, L. R., and Beliles, R. P. (1997). Physiological parameter values for physiologically based pharmacokinetic models. Toxicol. Ind. Health 13, 407–484. doi: 10.1177/074823379701300401
Buchwalter, D. B., Cain, D. J., Martin, C. A., Xie, L., Luoma, S. N., and Garland, T. (2008). Aquatic insect ecophysiological traits reveal phylogenetically based differences in dissolved cadmium susceptibility. Proc. Natl. Acad. Sci. U.S.A. 105, 8321–8326. doi: 10.1073/pnas.0801686105
Carlson, C., Hussain, S. M., Schrand, A. M., Braydich-Stolle, L. K., Hess, K. L., Jones, R. L., et al. (2008). Unique cellular interaction of silver nanoparticles: size-dependent generation of reactive oxygen species. J. Phys. Chem. B 112, 13608–11619. doi: 10.1021/jp712087m
Chen, P. J., Tan, S. W., and Wu, W. L. (2012). Stabilization or oxidation of nanoscale zerovalent iron at environmentally relevant exposure changes bioavailability and toxicity in medaka fish. Environ. Sci. Technol. 46, 8431–8439. doi: 10.1021/es3006783
Chen, P. J., Wu, W. L., and Wu, K. C. (2013). The zerovalent iron nanoparticle causes higher developmental toxicity than its oxidation products in early life stages of medaka fish. Water Res. 47, 3899–3909. doi: 10.1016/j.watres.2012.12.043
Chen, W. Y., and Liao, C. M. (2014). Interpreting copper bioaccumulation dynamics in tilapia using system-level explorations of pulsed acute/chronic exposures. Ecotoxicology 23, 1124–1136. doi: 10.1007/s10646-014-1255-1
Cheung, M. S., and Wang, W. X. (2005). Influence of subcellular metal compartmentalization in different prey on the transfer of metal to a predatory gastropod. Mar. Ecol. Prog. Ser. 286, 155–166. doi: 10.3354/meps286155
Croteau, M. N., and Luoma, S. N. (2009). Predicting dietborne metal toxicity from metal influxes. Environ. Sci. Technol. 43, 4915–4921. doi: 10.1021/es9007454
De Boeck, G., Ngo, T. T., Van Campenhout, K., and Blust, R. (2003). Differential metallothionein induction patterns in three freshwater fish during sublethal copper exposure. Aquat. Toxicol. 65, 413–424. doi: 10.1016/S0166-445X(03)00178-4
Dubois, M., and Hare, L. (2009). Subcellular distribution of cadmium in two aquatic invertebrates: change over time and relationship to Cd assimilation and loss by a predatory insect. Environ. Sci. Technol. 43, 356–361. doi: 10.1021/es801406r
Fan, W., Li, Q., Yang, X., and Zhang, L. (2013). Zn subcellular distribution in liver of goldfish (Carassius auratus) with exposure to zinc oxide nanoparticles and mechanism of hepatic detoxification. PLoS ONE 8:e78123. doi: 10.1371/journal.pone.0078123
Farmen, E., Mikkelsen, H. N., Evensen, O., Einset, J., Heier, L. S., Rosseland, B. O., et al. (2012). Acute and sub-lethal effects in juvenile Atlantic salmon exposed to low μg/L concentrations of Ag nanoparticles. Aquat. Toxicol. 108, 78–84. doi: 10.1016/j.aquatox.2011.07.007
Griffitt, R. J., Luo, J., Gao, J., Bonzongo, J. C., and Barber, D. S. (2008). Effects of particle composition and species on toxicity of metallic nanometerials in aquatic organism. Environ. Toxicol. Chem. 27, 1972–1978. doi: 10.1897/08-002.1
Griffitt, R. J., Weil, R., Hyndman, K. A., Denslow, N. D., Powers, K., Taylor, D., et al. (2007). Exposure to copper nanoparticles causes gill injury and acute lethality in zebrafish (Danio rerio). Environ. Sci. Technol. 41, 8178–8186. doi: 10.1021/es071235e
Grifftt, R. J., Hyndman, K., Denslow, N. D., and Barber, D. S. (2009). Comparison of molecular and histological changes in zebrafish gill exposed to metallic nanoparticles. Toxicol. Sci. 107, 404–415. doi: 10.1093/toxsci/kfn256
Guo, F., Yao, J., and Wang, W. X. (2013). Bioavailability of purified subcellular metals to marine fish. Environ. Toxicol. Chem. 21, 2109–2116. doi: 10.1002/etc.2286
He, M., Ke, C. H., and Wang, W. X. (2010). Effect of cooking and subcellular distribution on the bioaccessibility of trace elements in two marine fish species. J. Agric. Food Chem. 58, 3517–3523. doi: 10.1021/jf100227n
Huang, X., Guo, F., Ke, C., and Wang, W. X. (2010). Responses of abalone Haliotis diversicolor to sublethal exposure of waterborne and dietary silver and cadmium. Ecotox. Environ. Safe 73, 1130–1137. doi: 10.1016/j.ecoenv.2010.05.018
Isani, G., Falcioni, M. L., Barucca, G., Sekar, D., Andreani, G., Carpenè, E., et al. (2013). Comparative toxicity of CuO nanoparticles and CuSO4 in rainbow trout. Ecotox. Environ. Safe 97, 40–46. doi: 10.1016/j.ecoenv.2013.07.001
Kasemets, K., Ivask, A., Dubourguier, H. C., and Kahru, A. (2009). Toxicity of nanoparticles of ZnO, CuO, and TiO2 to yeast Saccharomyces cerevisiae. Toxicol. Vitro 23, 1116–1122. doi: 10.1016/j.tiv.2009.05.015
Kashiwada, S. (2006). Distribution of nanoparticles in the see - through medaka (Oryzias latipes). Environ. Health Perspect. 114, 1697–1702. doi: 10.1289/ehp.9209
Kim, T. H., Kim, M., Park, H. S., Shin, U. S., Gong, M. S., and Kim, H. W. (2012). Size-dependent cellular toxicity of silver nanoparticles. J. Biomed. Mater. Res. Part A 100A, 1033–1043. doi: 10.1002/jbm.a.34053
Krishnan, K., and Peyret, T. (2009). “Physiologically based toxicokinetic (PBTK) modeling in ecotoxicology,” in Ecotoxicology Modeling, ed J. Devillers (New York, NY: Springer), 145–175. doi: 10.1007/978-1-4419-0197-2_6
Lankveld, D. P. K., Oomen, A. G., Krystek, P., Neigh, A., and Troost-de Jong, A. (2010). The kinetics of the tissue distribution of silver nanoparticles of different sizes. Biomaterials 31, 8350–8361. doi: 10.1016/j.biomaterials.2010.07.045
Liao, C. M., Liang, H. M., Chen, B. C., Singh, S., Tsai, J. W., Chou, Y. H., et al. (2005). Dynamical coupling of PBPK/PD and AUC-based toxicity models for arsenic in tilapia Oreochromis mossambicus from a blackfoot disease area in Taiwan. Environ. Pollut. 135, 221–233. doi: 10.1016/j.envpol.2004.11.005
Lien, G. J., McKim, J. M., Hoffman, A. D., and Jenson, C. T. (2001). A physiologically based toxicokinetic model for lake trout (Salvelinus namaycush). Aquat. Toxicol. 51, 335–350. doi: 10.1016/S0166-445X(00)00117-X
Maes, H. M., Stibany, F., Giefers, S., Daniels, B., Deutschmann, B., Baumgartner, W., et al. (2014). Accumulation and distribution of multiwalled carbon nanotubes in zebrafish (Danio rerio). Environ. Sci. Technol. 48, 12256–12264. doi: 10.1021/es503006v
Nichols, J. W., Jensen, K. M., Tietge, J. E., and Johnson, R. D. (1998). Physiologically based toxicokinetics model for maternal transfer of 2,3,7,8-tetrachlorodibenzo-p-dioxin in brook trout (Salvelinus fontinalis). Environ. Toxicol. Chem. 17, 2422–2434. doi: 10.1002/etc.5620171208
Peyghan, R., Razijalaly, M., Baiat, M., and Rasekh, A. (2003). Study of bioaccumulation of copper in liver and muscle of common carp Cyprinus carpio after copper sulfate bath. Aquacult. Int. 11, 597–604. doi: 10.1023/B:AQUI.0000013323.69018.ff
Prasad, R. Y., McGee, J. K., Killius, M. G., Suarez, D. A., Blackman, C. F., DeMarini, D. M., et al. (2013). Investigating oxidative stress and inflammatory responses elicited by silver nanoparticles using high-throughput reporter genes in HepG2 cells: effect of size, surface coating, and intracellular uptake. Toxicol. Vitro 27, 2013–2021. doi: 10.1016/j.tiv.2013.07.005
Seebaugh, D. R., and Wallace, W. G. (2009). Assimilation and subcellular partitioning of elements by grass shrimp collected along an impact gradient. Aquat. Toxicol. 93, 107–115. doi: 10.1016/j.aquatox.2009.04.010
Shaw, B. J., Al-Bairuty, G., and Handy, R. D. (2012). Effects of waterborne copper nanoparticles and copper sulphate on rainbow trout (Oncorhynchus mykiss): physiology and accumulation. Aquat. Toxicol. 116–117, 90–101. doi: 10.1016/j.aquatox.2012.02.032
Shaw, B. J., and Handy, R. D. (2011). Physiological effects of nanoparticles on fish: a comparison of nanometals versus metal ions. Environ. Int. 37, 1083–1097. doi: 10.1016/j.envint.2011.03.009
Soedarini, D., Klaver, L., Roessink, I., Widianarko, B., van Straalen, N. M., and van Gestel, C. A. M. (2012). Copper kinetics and internal distribution in the marbled crayfish (Procambarus sp.). Chemosphere 87, 333–338. doi: 10.1016/j.chemosphere.2011.12.017
Wallace, W. G., Lee, B. G., and Luoma, S. N. (2003). Subcellular compartmentalization of Cd and Zn in two bivalves. I. Significance of metal-sensitive fractions (MSF) and biologically detoxified metal (BMD). Mar. Ecol. Prog. Ser. 249, 183–197. doi: 10.3354/meps249183
Wang, W. X., and Rainbow, P. S. (2006). Subcellular partitioning and the prediction of cadmium to toxicity to aquatic organisms. Environ. Chem. 3, 395–399. doi: 10.1071/EN06055
Keywords: waterborne nanoparticles, size-dependent, subcellular, toxicokinetic model, PBPK, nanotoxicity
Citation: Chen W-Y (2016) Toxicokinetic Modeling Challenges for Aquatic Nanotoxicology. Front. Mar. Sci. 2:114. doi: 10.3389/fmars.2015.00114
Received: 09 July 2015; Accepted: 10 December 2015;
Published: 05 January 2016.
Edited by:
Jiang-Shiou Hwang, National Taiwan Ocean University, TaiwanReviewed by:
Periyadan K. Krishnakumar, King Fahd University of Petroleum and Minerals, Saudi ArabiaRathinam Arthur James, Bharathidasan University, India
Copyright © 2016 Chen. This is an open-access article distributed under the terms of the Creative Commons Attribution License (CC BY). The use, distribution or reproduction in other forums is permitted, provided the original author(s) or licensor are credited and that the original publication in this journal is cited, in accordance with accepted academic practice. No use, distribution or reproduction is permitted which does not comply with these terms.
*Correspondence: Wei-Yu Chen, d3ljaGVuQGttdS5lZHUudHc=; Zjk2NjIyMDE2QG50dS5lZHUudHc=