- 1Aix Marseille Université, Centre National de la Recherche Scientifique/INSU, Université de Toulon, IRD, Mediterranean Institute of Oceanography, Marseille, France
- 2Mediterranean Institute of Oceanography – IRD/Centre National de la Recherche Scientifique/Aix-Marseille University, IRD, Noumea, New Caledonia
The dinitrogen (N2) fixed by diazotrophs and released as dissolved nitrogen (DN) has been compared in batch cultures of four marine diazotrophic cyanobacteria: the colony forming Trichodesmium IMS101 and the unicellular strains Cyanothece ATCC51142, Crocosphaera watsonii WH8501 and WH0003. Two approaches were conducted for this purpose. The first approach consisted in the comparison of the total accumulation of fixed N2 in the culture medium (both in the dissolved and particulate pools) with the net N2 fixation rates (i.e., the fixed N2 incorporated only in the particulate fraction after 15N2 incubation). The difference between the two measures accounted for the fixed N2 released as DN. The second approach consisted in the direct measure of the 15N-enrichment of ammonium (NH) and dissolved organic N (DON) following 15N2 incubations. The fixed N2 released as DN accounted for ~0–20% and ~1% of the fixed N2 after 24 h in the first and second approach, respectively. We show that the recent methodological improvements in the net N2 fixation determination applied in this study tend to reconcile the two approaches that formerly led to contrasted values. However, the large analytical uncertainties of the first approach limit its reliability. Thus, the direct determination of the 15N-enrichment of the dissolved pool remains the best tool to assess the fixed N2 released in the DN pool, in particular as it allows shorter incubation times. There were no clear patterns detected between the filamentous Trichodesmium and unicellular strains, neither in terms of the amount of fixed N2 released as DN nor in terms of the proportion of NH relative to DON. This suggests that the release of fixed N2 is a process shared among the filamentous and free living diazotrophs.
Introduction
The biological conversion of dinitrogen (N2) to ammonium (NH) -referred to as N2 fixation- is performed by organisms called diazotrophs, and represents the main external source of bioavailable nitrogen (N) to the global ocean (Deutsch et al., 2007; Gruber, 2008). This new N is thought to fuel up to 50% of new primary production in the tropical North Atlantic (Capone et al., 2005), North Pacific (Karl et al., 1997), and South Pacific (Moutin et al., 2008; Raimbault and Garcia, 2008) Oceans. The filamentous diazotrophic cyanobacterium Trichodesmium sp. has been the focus of most of the research on N2 fixation until the 2000s, as it is conspicuous and easy to collect (Capone et al., 1997). However, molecular inquiries have revealed a wider diversity of marine diazotrophs (Zehr et al., 1998, 2001, 2003; Moisander et al., 2010). In particular, unicellular cyanobacterial diazotrophs (UCYN) are more abundant than Trichodesmium sp. at the global scale (Luo et al., 2012) and contribute at least as much as Trichodesmium sp. to N2 fixation in several oceanic basins (Montoya et al., 2004; Bonnet et al., 2009; Benavides et al., 2011).
While important progress has been made over the last decades on determining the biogeographical distribution and controlling factors of diazotrophs in the global ocean, little is known about the fate of the recently fixed N2 in marine ecosystems, its release into the dissolved pool, and its potential transfer to the pelagic food web. Most of the N2 fixation estimates have been performed by measuring the 15N-enrichment of the particulate organic N (PON) pool after incubations with 15N2 (hereafter referred to as “net” N2 fixation rates) according to the protocols described in Montoya et al. (1996), or more recently in Mohr et al. (2010) and Großkopf et al. (2012). In these methods, the filtrate is discarded, and the 15N2 fixed and released in the dissolved pool as dissolved N (DN) is not taken into account. Previous field studies where 15N-enrichments were measured both in the PON and DN pools (leading to “gross” N2 fixation rates) indicate that the amount of fixed N2 released in the DN pool at the end of the incubation accounts for 10% to more than 50% of gross N2 fixation (Glibert and Bronk, 1994; Konno et al., 2010; Benavides et al., 2013b). This N release directly affects the ecosystems as the surrounding planktonic community can access to the DN released as ammonium (NH) or dissolved organic N (DON). As an example, in a semi-controlled competition experiment, Cyanothece sp. Miami BG 043511 transferred up to ~90% of the recently fixed N2 toward a non-N2 fixing cyanobacteria (Agawin et al., 2007). The transfer of recently fixed N2 by Trichodesmium sp. has also been evidenced in natural planktonic assemblage (Lee Chen et al., 2011; Bonnet et al., under revision) and is thought to support recurrent blooms of the harmful dinoflagellate Karenia brevis (Lenes and Heil, 2010; Mulholland et al., 2014). Ultimately, 15N isotopic signature reveals the presence of diazotrophs derived N in DON (Meador et al., 2007), zooplankton (Montoya et al., 2002; McClelland et al., 2003; Mompeán et al., 2013) and in sediment traps of the oligotrophic open ocean (Karl et al., 1997), demonstrating the potential biogeochemical importance of diazotrophs in these vast oceanic regions.
The recently fixed N2 released as DN reported in field studies has been related to dying diazotrophic cells, mainly through viral lysis (Fuhrman, 1999), sloppy feeding (O'Neil and Roman, 1992), and programmed cell death on decaying blooms of Trichodesmium sp. (Berman-Frank et al., 2004). However, studies performed on unialgal exponentially growing cultured diazotrophs reported that Trichodesmium sp. releases up to 80% of gross N2 fixation, suggesting an endogenous active release or excretion mechanisms (Mulholland et al., 2004; Mulholland and Bernhardt, 2005; Benavides et al., 2013a). This active release of N appears to be counterintuitive due to the high energetic cost of N2 fixation as compared to nitrate (NO) assimilation (Falkowski, 1983). Several explanations are detailed in the literature, such as the supply of N toward cells lacking the nitrogenase enzyme in the Trichodesmium sp. colonies through NH (Mulholland and Capone, 2000; Mulholland et al., 2004) or amino acids excretion (Carpenter et al., 1992; Capone et al., 1994; Mulholland and Capone, 1999), or an extracellular N storage in the mucilage of the small colonies of Gloeothece (Flynn and Gallon, 1990). While these processes appear valuable for colonial diazotrophs, they are counterintuitive for the free living UCYN, as large N release would represent a net loss of N. Thus, contrasting patterns may be expected between UCYN and colony forming diazotrophs regarding the amount and dynamics of N released as DN. However, comparative studies are still lacking.
Interestingly, the literature reports a wide range of fixed N2 released by diazotrophs, i.e., from ~0% to more than 80% of the gross N2 fixation. The lowest values are generally obtained by the direct measure of the 15N-enrichment of both particulate and dissolved pools, while the highest values are generally obtained by an alternative indirect method consisting in comparing net and gross N2 fixation rates, where the difference accounts for the fixed N2 released in the DN. However, it has been recently shown that the method used to estimate net N2 fixation rates were underestimated by a factor of 2–6 due to an incomplete and non-instantaneous dissolution of the 15N2 gas used as a tracer (Mohr et al., 2010; Großkopf et al., 2012; Wilson et al., 2012). As a result, the proportion of the fixed N2 released in the DN pool compared to the gross N2 fixation is probably overestimated by the same extent. New experiments using actualized methods are thus needed to reassess the magnitude of the fixed N2 released as DN and to reconcile the different approaches.
Here, the fixed N2 released as DN has been measured and compared in four strains of unicellular and filamentous marine diazotrophs representing some of the major contributors to global N2 fixation (Luo et al., 2012) grown in batch cultures. Two independent methodological approaches were used. In the first experiment the accumulation of total N (TN) in the culture medium over a period of 6 days, accounting for the gross N2 fixation, was compared with net N2 fixation using the actualized 15N2 dissolution method. In the second experiment, the fixed N2 released along a diel cycle was directly quantified in the filtrate by measuring the 15N-enrichment of the DON and NH pools after 15N2-incubation. The two methods are compared and discussed together with the differences and similarities observed between the strains.
Materials and Methods
Culture Conditions and Experimental Setup
Four unialgal strains were studied: Trichodesmium erythraeum IMS101 (hereafter referred to as Trichodesmium), isolated in the North Tropical Atlantic (Prufert-Bebout et al., 1993), Cyanothece sp. ATCC51142 (hereafter referred to as Cyanothece), isolated in an intertidal marsh (Reddy et al., 1993), and two strains of Crocosphaera watsonii: WH8501, isolated in the South Atlantic Ocean (Waterbury and Willey, 1988), and WH0003, isolated in the North Pacific Ocean (Webb et al., 2009). The culture medium was composed of natural seawater collected in the oligotrophic South West Pacific Ocean and characterized by low dissolved inorganic N (DIN) and DON concentrations (lower than 0.1 and 4 μmol N L−1, respectively). After collection, seawater was filtered (0.2 μm), autoclaved and amended with nutrients in the same proportion as for the YBC II medium (Chen et al., 1996), except for phosphate (PO), which concentration was set at 10 μmol P L−1 instead of 50 μmol P L−1 in the original YBCII. Cultures were maintained in this medium in exponential growth at 27°C and 120 μmol photon m−2 s−1 on a 12 h light: 12 h dark cycle for at least 10 generations. Cultures were not axenic but standard sterilization procedures of all laboratory material and systematic manipulation under a laminar flow hood were done in order to keep a minimum level of bacterial contamination. This contamination was checked using flow cytometry three times during the experiment (days 3, 6, and 11) for each strain. Heterotrophic bacterial populations were generally more than 3 orders of magnitude less abundant than the diazotrophs populations and did not accumulate during the experiment. Assuming a bacterial cell content of 5.8 fg N cell−1 (Fukuda et al., 1998), bacterial N biomass represented on average less than 0.05% (n = 12, range 0.01–0.5%) of the cultivated strains N biomass.
At the start of the monitoring (day 0), each of the four strains was distributed in three sterile polycarbonate 4.5 L bottles leading to a culture volume of 2.4 L in each bottle. In vivo chlorophyll a fluorescence was monitored every day at 2:30 pm local time for 13 days using a Trilogy fluorometer (Turner Designs) to determine the growth rates of each strain. Cells were gently mixed every day in order to avoid their adhesion to the sides of the bottles. Two different experiments (hereafter referred to as exp 1 and exp 2) were undertaken in order to quantify the recently fixed N2 released in the DN pool. Exp 1 consisted in the comparison of the cumulative net N2 fixation (using 24 h based 15N2 incubations) with the total N (TN) accumulation (accounting for the cumulative gross N2 fixation) where TN is defined as the sum of PON, DON, NH, and NOx (nitrate + nitrite). Exp 1 lasted for 6 days (from day 0 to day 6) in order to be able to measure significant deviation between net and gross N2 fixation. Every day, 110 mL of culture from each of the 4.5 L bottle were collected and apportioned as follows: 60 mL were devoted to the measure of N2 and carbon (C) fixation rates and the concentration of particulate organic C (POC) and PON, 20 mL were devoted to the measure of DON, 10 mL to the measure of NOx and PO and 20 mL to the measure of NH.
Exp 2 consisted in measuring the 15N-enrichment of the NH and DON pools following 15N2 incubations over a diel cycle. It was performed in the middle of the exponential growth phase, i.e., at day 6 for Cyanothece and C. watsonii WH0003 and at day 11 for Trichodesmium and C. watsonii WH8501 (Figure 1). The start of the monitoring was performed at the beginning of the N2 fixing period (light period for Trichodesmium and dark period for UCYN strains). Just before the start of the N2 fixing period, about 1.3 L from each triplicate culture vessel was distributed in nine sterile polycarbonate culture flasks (160 mL) resulting in 27 flasks for each strain. Each flask was 15N2-labeled according to the method described below and incubated under the same conditions as the parent culture. Every 3 h, a set of triplicate flasks was sacrificied for the following measurements: in vivo chlorophyll a fluorescence, inorganic nutrients, PON, DON, N2, and C fixation rates, and 15N-enrichment of the NH and DON pools.
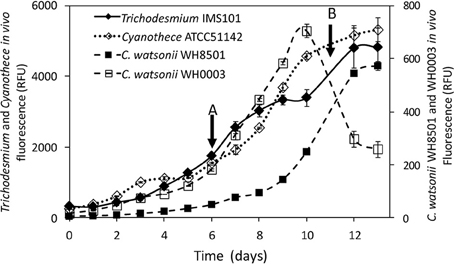
Figure 1. Evolution of the in vivo Chl a fluorescence (in relative fluorescence units) in Trichodesmium IMS101, Cyanothece ATCC51142, C. watsonii WH8501 and WH0003 cultures. Error bars represent the standard deviation of triplicate measurements and the propagated analytical error. Black arrows represent the start of the diel cycle experiments (exp 2): on day 6 in Cyanothece and C. watsonii WH0003 cultures (A), on day 11 in Trichodesmium and C. watsonii WH8501 cultures (B).
It has to be noticed that these experiments quantify the amount of N2 that has been fixed and then released as DN within the time of incubation (< 24 h), such that DN accumulation resulting from this recent flux could be discriminated from bulk DN. Thus, the measure has to be discriminated from the total DN accumulation in the culture medium.
Dissolved Organic and Inorganic Nutrients Measurements
Samples for nitrite (NO), NO, and PO determination were collected in HCl-washed 20 mL vials, poisoned with HgCl2 to a final concentration of 10 μg L−1, and stored at 4°C until analyses performed on a segmented flow auto-analyzer (Aminot and Kérouel, 2007). The detection limit was 0.05 μmol N L−1 for NOx and 0.01 μmol P L−1 for PO. Samples for NH determination were collected in 40 mL glass vials and directly analyzed according to Holmes et al. (1999) on a Trilogy fluorometer (Turner Designs). The detection limit was 0.01 μmol N L−1. Samples for DON were collected in 40 mL glass vials, filtered on pre-combusted (450°C, 4 h) GF/F filters, stored at −20°C until analysis by the wet-oxidation method according to Pujo-Pay and Raimbault (1994). DON concentrations were corrected from the contribution of NH and NOx.
N2 Fixation and Primary Production Rates
Net N2 fixation rates were measured using the 15N2-enriched seawater method (Großkopf et al., 2012). The enriched seawater was prepared using the culture medium described above, which was degassed for 1 h by circulating it through a degassing membrane (mini-module®, Membrana) connected to a vacuum pump (< 850 mbar) at a rate of 280 mL min−1. The degassed medium was then transferred to a 2 L gas tight Tedlar® bag using silicon tubing. 10 mL of 15N2 (98.3 atom% 15N, Cambridge Isotope Laboratories) was added to the bag and the bubble was “physically broken” until its complete dissolution. 15N2 enriched medium was then added (5% vol:vol) to the 60 mL bottles for exp 1 and to the experimental 160 mL culture flasks for exp 2. In order to measure the 15N2 enrichment of the medium, samples were collected from Tedlar® bags in Exetainer® vials previously He-purged in order to avoid contact with atmospheric N2. These samples were analyzed on a Membrane Inlet Mass Spectrometer (MIMS) for the determination of the 30N/28N ratio (Kana et al., 1994) using natural seawater at equilibrium with the atmosphere as a reference. The measured 15N2 enrichment in the Tedlar® bags was 84 ± 8% resulting in a final enrichment of 4.2 ± 0.4 atom% in the incubation bottles. Net primary production (C fixation) was measured using the 13C labeling method by adding a H13CO solution to the experimental culture bottles together with 15N2 enriched medium, resulting in a final calculated 13C enrichment of 10 atom%. For exp 1, the experimental bottles were incubated for 24 h and filtered on precombusted (450°C, 4 h) GF/F glass fiber filters (Whatman®). For exp 2, the triplicate experimental bottles were filtered every 3 h along the diel cycle and treated as described above. The PON and POC contents and the 15N and 13C enrichment of the cells were measured on an elemental analyzer coupled to an isotope ratio mass spectrometer (EA-IRMS, Integra CN) calibrated using IAEA standards. The analytical precision associated with mass determination ranged between 0.2 and 2.8% of PON and between 0.8 and 4.8% of POC. The analytical precision associated with 15N and 13C enrichment was ± 0.0010 atom% and ± 0.0003 atom% for a measured mass of 0.7 μmol-N and 6.7 μmol-C, respectively. All the results were corrected from the blank contribution. Net N2 fixation (ρN2, net) and net C fixation (ρCnet) rates were calculated as follows:
with ΔRPON and ΔRPOC the differences in 15N and 13C atom% measured in the particulate matter between two time points, RN2 and RC the 15N and 13C atom% in seawater during the incubation, [PON] and [POC] the PON and POC concentrations (μmol L−1) at the given time point, and Δt the time between each sampling point. For exp 1, the cumulated net N2 fixation was calculated as the sum of all the daily-based ρN2, net. For exp 2, ρN2, net and ρCnet were divided by the number of cells in the cultures in order to express the cellular N2 fixation rates. The final analytical precision was calculated as the analytical precision of each term accumulated according to the propagation of errors law.
15N-enrichment of NH and DON Pools
The 15N-enrichment of the NH and DON pools during exp 2 was measured using the two steps ammonium diffusion method modified from Slawyk and Raimbault (1995) and Raimbault et al. (1999). At each of the 9 time points over the diel cycle, 100 mL of the filtrate from every 160 mL flask were collected in polyethylene tubes, poisoned with HgCl2 (20 μg mL−1 final concentration), stored at 4°C in the dark, and transferred to 500 mL borosilicate bottles just before analysis. Briefly, during the first step, all the NH was converted into NH3 by adding MgO (baked at 450°C for 4 h) and then trapped on an acidified GF/C glass filters (50 μL H2SO4 0.5 N) suspended above the sample using a stainless hook attached to the cap. 1 μmol-N of non-labeled NH was added as a carrier to provide enough PN for mass spectrometry analyses. After 1 week of incubation at 55°C with daily agitation, filters were recovered, dried at 60°C for 24 h and stored in precombusted (450°C, 4 h) glass vials until analysis by EA-IRMS as described above for N2 fixation. During the second step, the DON was converted to nitrate (NO) by wet oxidation. 10 mL of a digestion mixture (60 g of K2S2O8 dissolved in 1 L of NaOH 1.5 N) was added to the borosilicate bottles, which were then autoclaved at 120°C for 30 min. The NO resulting from the oxidation was reduced in NH by adding 200 mg of Devarda's alloy (baked at 450°C for 4 h). The NH was then recovered by repeating the first step adding 1.5 mL of NaOH 12.5 N instead of MgO. In this second step the use of a strong base (NaOH) compensated the acidity brought by the Devarda's alloy and insured basic conditions. NONO were also recovered during this second step, but as they are not likely released by diazotrophs (thus not 15N-enriched) and considering their low concentrations (< 0.2 μmol N L−1) relative to DON in the culture medium, they were not discriminated from the DON pool. All the results were corrected from the blank contribution. For each incubation time of exp 2, the total amount of N2 fixed and incorporated as PON () or released as NH and DON () was calculated as:
with ΔRN, the 15N-enrichment of the PON, NH, or DON pools and [N], the PON, DON, or NH concentrations. The recovery of the 15N in the dissolved pool allowed the calculation of the gross N2 fixation for each incubation time as:
In order to evaluate the accuracy of the method, two tests were conducted. First, the ability of the method to recover expected N content and 15N-enrichment of the whole cultures was tested. For this purpose, the culture medium containing Cyanothece and C. watsonii WH0003 cells was sampled after 12 h and 24 h of 15N2-incubation. The N content of the samples was extracted as described in the second step of the diffusion method (Slawyk and Raimbault, 1995). The resulting TNdiffused concentration was compared to the TN obtained from the sum of PON, DON, NH, and NOx concentrations individually determined. In addition, the 15N-enrichment recovered allowed the direct determination of the gross N2 fixation as follows:
with ΔRTN the 15N-enrichment of the diffused TN pool and [TN] the sum of the PON, DON, NH, and NOx concentrations. The was compared to the ∑N2, gross calculated at the same times points.
The second test consisted in the evaluation of the ability of the method to isolate the NH pool from the alkali-labile DON compounds. For this purpose several organic substrates (urea, creatine, and glutamic acid) were dissolved in 100 ml of filtered (0.2 μm) seawater (30 μmol N L−1 final concentrations) and processed as described in the first step of the diffusion method. The recovery of these compounds was compared to the recovery of NH diffused in the same conditions.
An isotopic fractionation occurs during the diffusion of the NH which tends to decrease the final 15N-enrichment (Holmes et al., 1998). According to Holmes et al. (1998), the high N recovery during the NH diffusion step (>95%) and the relatively small volumes used for the diffusion in our study limited the isotopic fractionation. The latter was thus neglected.
Results
The four strains exhibited an increase of in vivo chlorophyll a fluorescence along the 13 days of monitoring (Figure 1). The average growth rates calculated for each strain during the exponential growth phase were 0.30 d−1, 0.26 d−1, 0.37 d−1, and 0.38 d−1 for Trichodesmium, Cyanothece, C. watsonii WH8501, and WH0003 cultures, respectively.
Fixed N2 Released as DN Estimated from the Mass Balance Approach (Exp 1)
PON and DON concentrations continuously increased in all cultures during exp 1, while NH concentrations remained below 0.5 μmol N L−1 during the 6 days of the experiment and did not show any clear pattern (Figure 2). The resulting increase of TN after the 6 days of the experiment was 82.7 ± 3.8 μmol N L−1, 102.3 ± 2.5 μmol N L−1, 37.0 ± 2.4 μmol N L−1, and 88.9 ± 4.5 μmol N L−1 in Trichodesmium, Cyanothece, C. watsonii WH8501, and WH0003 cultures, respectively. In the meantime, net N2 fixation rates cumulated over the 6 days of exp 1 reached 78.3 ± 9.9 μmol N L−1, 84.2 ± 9.7 μmol N L−1, 30.8 ± 5.2 μmol N L−1, and 80.2 ± 11.0 μmol N L−1 in the cultures as cited above. Even though the two parameters followed the same pattern, the accumulation of TN was higher than the cumulated net N2 fixation at the end of exp 1 in all strains (Figure 3). The difference accounted for the fixed N2 released as DN during the 24 h of incubation and represented 5 ± 13, 18 ± 12, 17 ± 18, and 10 ± 15% of the gross N2 fixation in Trichodesmium, Cyanothece, C. watsonii WH8501, and WH0003 cultures, respectively (Table 1). At the end of exp1, due to the large analytical uncertainties, the proportion of fixed N2 released as DN was significantly higher than zero only in the Cyanothece culture (one-tailed t-test, α = 0.05, n = 3). Furthermore, there were no statistically significant differences in fixed N2 released as DN between the four strains (two-tailed t-test, α = 0.05).
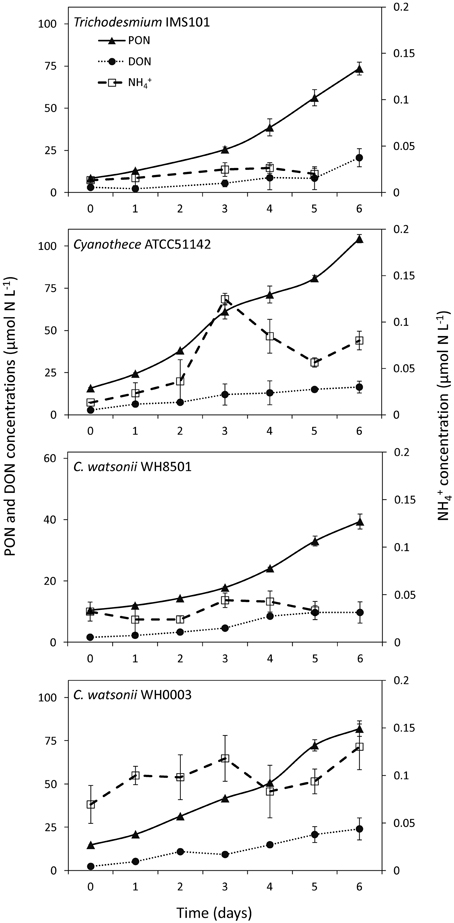
Figure 2. Evolution of Particulate Organic Nitrogen (PON), Dissolved Organic Nitrogen (DON) and ammonium (NH) concentrations during exp 1. Error bars represent the standard deviation of triplicate measurements and the propagated analytical error.
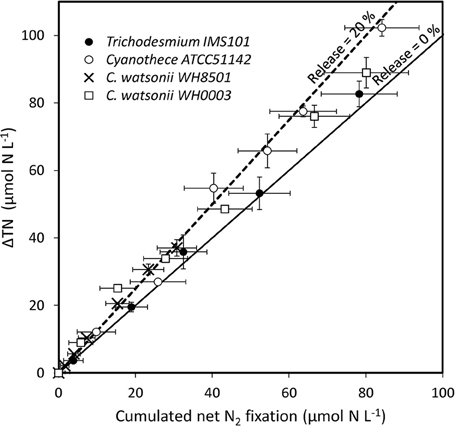
Figure 3. TN accumulation (ΔTN), accounting for gross N2 fixation, as a function of cumulated incorporation of N2 in the PON, accounting for net N2 fixation, in the culture media during exp 1. Error bars represent the standard deviation of triplicate measurements and the propagated analytical error. The lines show the evolution of the system assuming a proportion of fixed N2 released as DN accounting for 0% (solid line) and 20% (dotted line) of the gross N2 fixation.
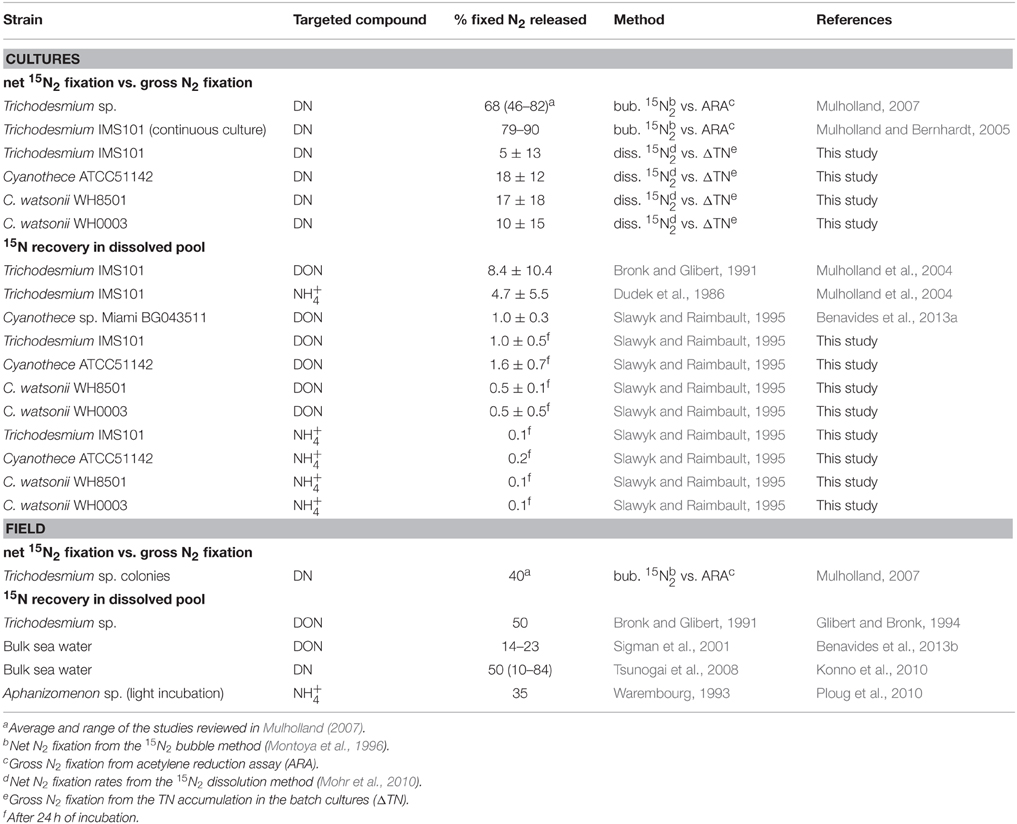
Table 1. Summary of the fixed N2 released as DN, NH, or DON reported for diazotrophs in the literature and in this study (in percentage of the gross N2 fixation) as a function of the methodology used.
During exp 1, the cellular C:N ratios in the studied strains were relatively stable and averaged 4.7, 7.7, 9.2, and 12.3 in Trichodesmium, Cyanothece, C. watsonii WH8501, and WH0003 cultures, respectively (Table 2). The C:N2 fixation ratio clearly increased for all strains by a factor of up to 4, during the 6 days of incubation (Table 2).
Fixed N2 Released as DN Measured from the 15N-enrichment of DN (Exp 2)
During exp 2, C and N2 fixation rates were followed over the diel cycle. N2 fixation started at the beginning of the light period in Trichodesmium culture, peaked at midday at 8.5 ± 1.0 fmol N cell−1 h−1 (assuming 100 cells per trichome), and stopped at the beginning of the dark period (Figure 4). C fixation followed a similar pattern and peaked around midday at 223.0 ± 65.0 fmol C cell−1 h−1 (Figure 4). Conversely, N2 and C fixation rates in the UCYN cultures were time-decoupled. In Cyanothece, C. watsonii WH8501 and WH0003 cultures, N2 fixation started at the beginning of the dark period and peaked between 6 and 9 h at 1.3 ± 0.1 fmol N cell h−1, 0.6 ± 0.1 fmol N cell h−1 and 2.5 ± 0.7 fmol N cell h−1, respectively (Figure 4). C fixation occurred during the light period and peaked at 44.2 ± 16.1 fmol C cell h−1, 14.2 ± 3.0 fmol C cell h−1, and 68.6 ± 24.0 fmol C cell h−1 in Cyanothece, C. watsonii WH8501, and WH0003 cultures, respectively (Figure 4).
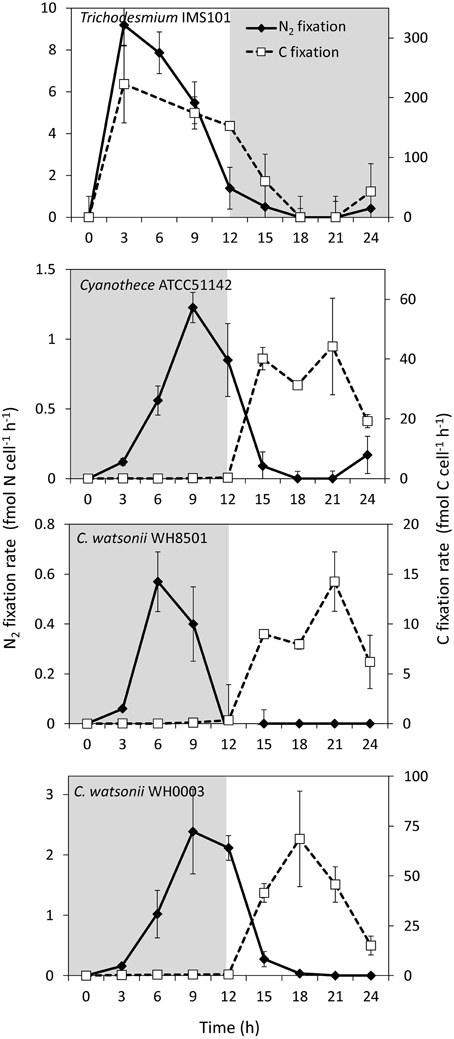
Figure 4. Net N2 and C fixation rates during exp 2 for each strain studied. Error bars represents the standard deviation of the triplicates cumulated with the analytical precision according to the propagation of errors. Gray areas represent the dark periods.
Although variable, DON concentrations did not show any clear pattern along the diel cycle with concentrations ranging from 6 to 19 μmol N L−1 (Figure 5). NH concentrations ranged from 0.09 to 0.19 μmol N L−1, respectively in all cultivated strains, except in the C. watsonii WH0003 culture, where NH concentrations peaked after the beginning of the N2 fixing period (dark) at 0.43 ± 0.29 μmol N L−1 (Figure 5). In the Trichodesmium culture, the 15N-enrichments of the DON (RDON) and NH (RNH4) pools during the diel cycle followed the enrichment of the PON pool (RPON), i.e., it increased during the N2 fixing period and remained stable during the non N2 fixing period (Table S1). However, RDON remained 6–20 times lower than RPON, whereas RNH4 reached and exceeded the value of RPON at the end of the N2 fixing period. Similarly, in the UCYN cultures, the 15N-enrichment of the dissolved pools also increased along the diel cycle and RDON remained lower than the RPON, whereas RNH4 equaled or exceeded RPON (Table S1). For all the strains, due to the low NH concentrations, the fixed N2 released as NH represented less than 0.2% of gross N2 fixation after 24 h of incubation (Figure 5, Table S2). Meanwhile, the fixed N2 released as DON did not exceed 1.6% of gross N2 fixation (Figure 5, Table S2). Most of the fixed N2 released as DN (sum of DON and NH) was in the form of DON (76–90%) in all cultures. These results indicate that while the proportion of the fixed N2 released increased over the diel cycle in the Trichodesmium culture, it was maximum for UCYN strains at the beginning of the N2 fixing period (in particular for Cyanothece, Figure 5) and tended to decrease afterwards. Nevertheless, these peaks have to be moderated by their low values and large uncertainties. No statistical significant differences were found between the strains, neither in terms of fixed N2 released as DN (two-tailed t-test, α = 0.05, n = 6) nor in terms of proportion of fixed N2 released as NH vs. DON (two-tailed t-test, α = 0.05, n = 6) after 24 h of incubation. Our results also indicate that the proportion of fixed N2 released as DN after 24 h of incubation for each of the strains studied was not significantly different between exp 1 and exp 2 (two-tailed t-test, α = 0.05, n = 6).
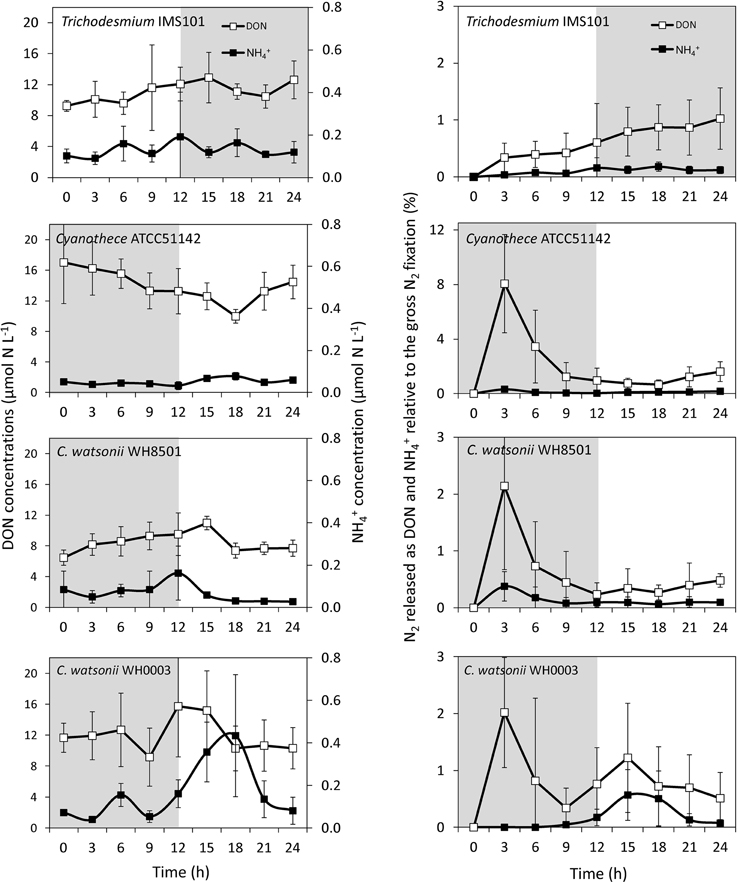
Figure 5. Evolution of DON and NH concentrations along the diel cycle (left panel) and proportion of fixed N2 released as DON and NH relative to gross N2 fixation (right panel) as a function of the incubation time in each culture during exp 2. Error bars represent the standard deviation of triplicate measurements and the propagated analytical error. Gray areas represent the dark periods.
Accuracy of the Diffusion Method
The tests conducted to evaluate the accuracy of the diffusion method indicate that more than 95% of the NH were recovered after the first step of the diffusion (Table 3). The recovery of the whole N culture content after all steps (wet oxidation, conversion of NO into NH3, and diffusion on the filter) ranged from 67 to 91% (78% on average; Figure 6). The method reproduces well the expected 15N-enrichment as, on average, the gave similar results than the ∑N2, gross (Figure 6). The first step of the diffusion method had a limited impact on the hydrolysis and further diffusion of the various DON compounds tested (Table 3). Indeed, while the recovery of NH was >95%, < 12% of the DON compounds tested were hydrolyzed and diffused.
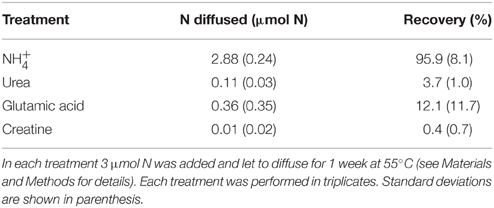
Table 3. N recovered after the first step of the diffusion method for ammonium (NH) and three organic molecules solutions.
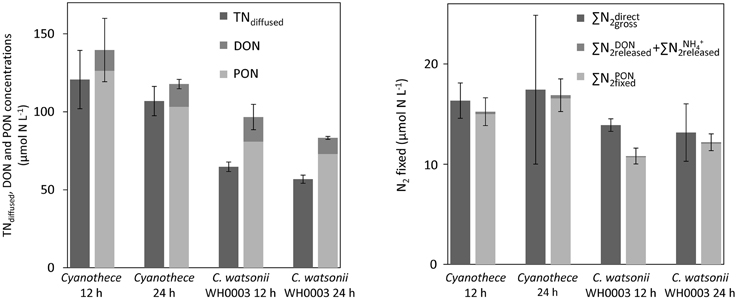
Figure 6. Concentrations of TN recovered using the diffusion method (TNdiffused) and sum of PON and DON concentrations at the 12 h and 24 h time points in Cyanothece and C. watsonii WH8501 cultures (left). Gross N2 fixation measured using the 15N-enrichment recovered from the diffusion of the whole culture medium () and as the sum of N2 incorporated in PON () and released as DON () and NH () after 12 h and 24 h of incubation for Cyanothece and C. watsonii WH8501 (right). Error bars represent the standard deviation of triplicate measurements and the propagated analytical error.
Discussion
Diazotrophs can fix atmospheric N2 at high rates, and introduce new N into nutrient depleted waters of the ocean. The release of a high proportion of recently fixed N2 to the DN pool has long been suggested based on the observed accumulation of DN during Trichodesmium sp. blooms (Devassy et al., 1979; Karl et al., 1997; Lenes et al., 2001). Most of the culture experiments have focused on Trichodesmium sp., but very few data are available for UCYN (Table 1). Here, are discussed and compared the results of fixed N2 released in the DN pool obtained using two independent approaches in four cultures of representative marine diazotrophs grown under identical conditions.
Methodological Considerations Regarding the Measure of the Fixed N Release
The measure of the fixed N2 released by diazotrophs is affected by a number of methodological issues that needs to be mentioned prior to any physiological or ecological interpretation. In the literature, the values of fixed N2 released as DN appear to be closely related to the methodological approach used (Table 1). The methods used can be separated into two categories: 1/ the comparison of net and gross N2 fixation rates assessed by acetylene reduction assays (ARA) or the accumulation of TN in the batch cultures and 2/ the direct measurement of 15N-enrichment in the DN pool following 15N2 incubations (Mulholland et al., 2004; Benavides et al., 2013a, this study). Both approaches have methodological issues that are discussed below, together with recent insights taken into account in our study that tended to reconcile them.
The release estimated by the comparison of the gross and net N2 fixation generally yield higher values as compared to the 15N isotopic determination of DN (Table 1). Recent insights showed that the 15N2 bubble method widely used (Montoya et al., 1996) underestimates net fixation rates by a factor of 2–6 (Mohr et al., 2010; Großkopf et al., 2012; Wilson et al., 2012) that may overestimate the release to the same extent. The large proportion of fixed N2 released as DN obtained from previous comparisons of the gross and net N2 fixation rates reported in Table 1 (70–90% of the gross N2 fixation) would be reduced to 21–27% if we consider a dissolution of 30% of the 15N2 bubble during the 12 h of the fixing period as shown by Mohr et al. (2010). Another issue is the uncertainty of the gross rate when estimated from the ARA as the conversion factor between acetylene and N2 is subjected to debate (from 3 to 4), which prevents any precise evaluation of the fixed N2 released as DN (Mulholland, 2007). Consequently, the values of fixed N2 released as DN estimated from the comparison of gross and net N2 fixation rates should be considered with caution.
The recovery of the 15N-enrichment in the DN pool (DON and NH) following 15N2 incubations is the only way to directly measure the recently fixed N2 released as DN. Two methods have been used to recover the DON: the DON separation from DIN using an ion retardation column (Bronk and Glibert, 1991), and the diffusion method (Slawyk and Raimbault, 1995, see Materials and Methods Section for details). Theoretically, both methods should be able to recover an important and representative fraction of the DON pool. However, none of the methods show 100% accuracy. Changes made in the manufacture of the retardation ion column have resulted in a variable DON isolation efficiency (McCarthy and Bronk, 2008), leading to the possibility of contaminations by DIN when using the new-build ions retardation resins. Similarly, the high temperature conditions (55°C) used for the extraction of the NH associated with the diffusion method (Slawyk and Raimbault, 1995) hydrolyzes part of the labile DON pool leading to a biased estimate of the enrichment (McCarthy and Bronk, 2008). The tests conducted in this study showed that the hydrolysis of labile DON compounds during the NH diffusion step occurs, albeit relatively low (< 12%, Table 1). Further investigations should be conducted to estimate to which extent the accumulation of small fraction of hydrolyzed DON compounds may ultimately affect the measured 15N-enrichment of the NH pool. The isotopic signature recovered in both methods may thus not be equal, which hinders the direct comparison of the fixed N2 released as DON between studies. Nevertheless, our results of fixed 15N2 released as DON and NH (1–8% of the gross N2 fixation) are in the same range than the two studies that used the recovering 15N in the dissolved pool for Cyanothece (~1%) and Trichodesmium cultures (~10%) (Mulholland et al., 2004; Benavides et al., 2013a).
In this study we used the 15N2-enriched seawater method and measured the 15N2 enrichment of the incubated medium (RN2) in order to provide accurate estimates of net N2 fixation rates. As a result, the differences between the fixed N2 released as DN values calculated from the mass balanced approach (exp 1) used here and the 15N recovery in the dissolved pool (exp 2) were reduced as compared to previous studies (Table 1). However, uncertainties in the determination of the RN2 and the propagations of the errors during the 6 days of exp 1 resulted in high uncertainties associated with the final estimates of fixed N2 released as DN (about ±10% of the gross N2 fixation). Alternatively, the direct recovery of the 15N in the DN pool allows short incubation times and thus limits the propagation of errors. Furthermore, the final proportion of fixed N2 released as DN compared to the gross N2 fixation is not sensitive to the RN2. Indeed, when calculating the proportion, Equation (3) is divided by Equation (4) and the RN2 is divided by itself. Thus, despite the recognized methodological bias, the 15N recovery method appears to be the most accurate way to quantify the fixed N2 released as DN both in culture and in field studies.
C and N Dynamics
The growth rates measured in this study are in good agreement with those reported in the literature for Trichodesmium sp. (LaRoche and Breitbarth, 2005), Cyanothece sp. (Feng et al., 2010), and C. watsonii sp. (Webb et al., 2009). During the diel cycle (exp 2), Trichodesmium net N2 fixation rates peaked 3 h after the beginning of the light period, which is slightly earlier and in the lower end of rates (determined using ARA) previously reported (Mulholland and Bernhardt, 2005; Wannicke et al., 2009; Knapp et al., 2012). The magnitude of net N2 fixation rates measured in the UCYN cultures are in the range of those reported in the literature determined using ARA (Dekaezemacker and Bonnet, 2011; Knapp et al., 2012; Masuda et al., 2013). The temporal uncoupling between C fixation (photosynthesis) and N2 fixation observed over the diel cycle both in the cellular C:N ratio and fixation rates is well documented for Cyanothece (Sherman et al., 1998) and C. watsonii (Dron et al., 2012; Mohr et al., 2013). This decoupling prevents the inactivation of the nitrogenase by oxygen produced during photosynthesis (Fay, 1992; Gallon, 1992). The averaged cellular C:N ratios were different among the strains. Trichodesmium showed the lowest cellular C:N ratio (4.7 ± 0.6), which is at the lower end of the values reported in the literature for both culture and field experiments (LaRoche and Breitbarth, 2005; Mulholland, 2007; Holl and Montoya, 2008; Wannicke et al., 2009). In contrast, the relatively high cellular C:N ratio of the UCYN cultivated in this study (Table 2) is in the range of published ratios for Cyanothece (Benavides et al., 2013a) and in the high end for the two C. watsonii strains (Webb et al., 2009; Dekaezemacker and Bonnet, 2011).
Diazotrophs are known to exhibit a higher C:N2 fixation ratio than that expected from their cellular C:N ratio or to the Redfield ratio of 6.6 (Mulholland, 2007). As an example, C:N2 fixation ratio are on average 7 times higher than the Redfield ratio in the Sargasso Sea (Orcutt et al., 2001). Some investigators have suggested that the high C:N2 fixation ratio in Trichodesmium colonies may be explained by an over C fixation to serve as carbohydrate for the ballasting effect (Villareal and Carpenter, 1990; Romans et al., 1994; White et al., 2012), or to reduce by respiration the oxygen concentration that would inhibit the nitrogenase activation. Alternatively, the discrepancy between fixation and biomass C:N ratios may be due to substantial fixed N2 release into dissolved pool (Mulholland et al., 2004). Here, the use of the actualized 15N2 net fixation rate method tended to bring closer the two ratios (Table 2) as compared to previous studies. This reduces the importance of the processes cited above, in particular the hypothesis of large fixed N2 releases which is comforted by the low values reported here. Nevertheless, while the cellular C:N ratios of all the strains monitored here were relatively stable, the C:N2 fixation ratios increased along exp 1, indicating a shift in the metabolism of N, C or both assimilation processes (Table 2). This has already been observed in batch cultures of Trichodesmium IMS101 (Mulholland and Capone, 2001) and Cyanothece sp. BG 043511 (Benavides et al., 2013a). It is possible that in response to the increase in DON availability (Figure 2), the organisms partly use this alternative source of N, leading to an increase in the C:N2 fixation ratio. This is supported by studies that have measured significant uptake rates of DN compounds by Trichodesmium sp. (Mulholland and Capone, 1999; Mulholland et al., 1999; Orcutt et al., 2001; Holl et al., 2005) and Aphanizomenon sp. (Berman, 1999) cultures. Furthermore, changes in the ratio of N2 fixation relative to NH or DON uptakes with the different growth stages confirm that diazotrophs may adapt their N metabolism as a function of their environment (Mulholland and Capone, 2000, 2001).
Strong Similarities in the Release between the Filamentous and Unicellular Strains
Large differences in the N metabolism between the colony forming Trichodesmium and the free living UCYN strains were suspected to generate differences in the fixed N2 release dynamics. Indeed, the slight but continuous increase of fixed N2 release observed in the Trichodesmium culture contrasts with the peak observed at the beginning of the N2 fixing period in the UCYN ones. It is possible that part of the recently fixed N2 by UCYN during the dark period is stored in the extracellular pool before being metabolized during the photosynthesis period. However, the peaks of fixed N2 release occurred at the very beginning of the N2 fixation period, when the rates were still low (Figure 4). Thus, the absolute amount of fixed N2 released is low compared to the total amount of N2 fixed along the diel cycle and does not significantly differ from Trichodesmium after 24 h of incubation.
The low fixed N2 release rates reported here seem to contradict the efficient transfer of fixed N2 from diazotroph to non-diazotrophs observed in culture experiments (Agawin et al., 2007; Lee Chen et al., 2011). Nevertheless, the absence of 15N accumulation in the DN pool does not neccesarly preclude intense N fluxes to transit through the dissolved pool. The measure of the fixed N2 released as DN is the result of the recently fixed N2 excreted out from the cells (from the intra- to the extra-cellular pools) but also of its subsequent uptake that would occur during the incubation time. Indeed, high NH uptake rates are reported in Trichodesmium sp. (Mulholland and Capone, 1999, 2001; Mulholland et al., 2004) and C. watsonii cultures (Masuda et al., 2013). This might explain the absence of NH accumulation in the DN pool in our cultures and the low release rates measured, but it also implies high NH regeneration which is in good agreement with the high enrichment of the NH pool measured in the present study (Table S2). The absence of significant differences in the release between the strains studied here suggests that the N release and regeneration occurs both for colonial/filamentous and free living diazotrophs. Thus, the release and the subsequent uptake of the recently fixed N2 may be a way to exchange N not only for filamentous but also for unicellular and free living diazotrophs (Foster et al., 2013; Thompson and Zehr, 2013). It could also be due to the nitrogenase apparatus that is complex to modulate; the energetic cost of its flexibility could be higher than the cost of over-fixing N2 (Dron et al., 2012). In addition, it is possible that the NH regeneration is due to an unavoidable counter diffusion, i. e. the unionized NH3, in equilibrium with NH, accumulated in the intracellular pool may diffuse toward the extracellular pool (Kleiner, 1985; Van Dommelen et al., 1998). High NH uptake ability would thus prevent the diazotrophs to run out of N. Another factor that may explain efficient N2 transfer despite low extracellular fixed N2 releases rates is a high production of extracellular polysaccharides (EPS) as reported for C. watsonii (Sohm et al., 2011). Theses exudates, in which the cells are embeded, are retained on GF/F filters and may limit the diffusion of the released compounds toward the dissolved pool resulting in low fixed N2 release as DN. However, in the field, the formation of aggregates through high EPS production may facilitate the transfer of N2 from diazotrophs to non-diazotrophs through their spacial proximity (Paerl and Priscu, 1998; Foster et al., 2013; Klawonn et al., 2015).
Most of the fixed N2 released as DN measured in this study was in the organic form (DON ~80–90%). This suggests that a significant part of the fixed N2 could be advantageous for heterotrophs through the microbial loop or phytoplankton capable of mixotrophy (Bronk et al., 2007). These results have to be taken with caution as the cultures were not axenic. Even though the bacterial contamination was limited (< 0.05% of the total biomass in average), it could have played a potential role in the N turnover in the culture medium. It is possible that the released DON compounds were recycled as NH, which might prevent the accumulation of DON and thus reduce the release rates. Furthermore, part of the bacteria can pass through the GFF filter and affect the fixed N2 released as DON. Nevertheless, despite present, no heterotrophic bacterial accumulations were observed during the course of the experiment and their low biomasses strongly suggest that the bacterial presence had a limited impact on the main conclusions of this study, namely low fixed N2 releases in the DN.
The fixed N2 released by diazotrophs reported in the literature is generally higher for field studies compared to culture studies (Table 1). Aside from the methodological bias, the physiological status of the cells is probably one of the main factors explaining this difference. Culture studies are generally performed during the exponential growth phase of cells, whereas in field studies, communities are probably composed of cells experiencing different phases of their cellular cycle that may affect the fixed N2 released as DN (Mulholland et al., 2004). The release is also sensitive to a variety of exogenous factors occurring in the field such as intense viral lysis (Fuhrman, 1999; Hewson et al., 2004) and sloppy feeding (O'Neil and Roman, 1992; O'Neil et al., 1996), which are absent (or strongly limited) in cultures. Additionally, in the field, cells are not maintained in optimal growth conditions of temperature, nutrient and light availability, which may influence N2 fixation rates and the quantity and quality of fixed N released. As an example, Wannicke et al. (2009) showed an increase of extracellular NH concentrations in Trichodesmium IMS101 cultures exposed to drastic changes of light intensity, suggesting an increase of the fixed N2 released as NH.
Conclusions
This study provides new insights on the recently fixed N2 released as DN by different strains of diazotrophs and shows that, in culture, the release does not depend on the different diazotrophs strains considered. Indeed, no clear differences were observed between filamentous and unicellular strains both in terms of quantity of fixed N2 released as DN, and in terms of quality (DON vs. NH). The absence of significant differences suggests that release processes are shared among free living and filamentous diazotrophs. The review of previously published experiments shows that the two approaches commonly used lead to very different values of fixed N2 released as DN. The more accurate determination of N2 fixation rates applied here tended to reduce the gaps between both approaches and shows that the proportion of fixed N2 released as DN is likely < 10% of the gross N2 fixation in diazotroph culture. Nevertheless, the N flux excreted out of the diazotroph cells might be higher due to probable NH re-uptake. In natural assemblages, fixed N2 excreted as DN may be efficiently used by the surrounding planktonic community, which is confirmed by multiple examples of cellular interactions between various diazotrophs and their close environment (Thompson and Zehr, 2013).
Conflict of Interest Statement
The authors declare that the research was conducted in the absence of any commercial or financial relationships that could be construed as a potential conflict of interest.
Acknowledgments
We gratefully acknowledge I. Berman-Frank, M. Eichner and E. Webb for providing the diazotrophic strains used in this study. HB was supported by a PhD scholarship from the French Ministry of Education and Research. Funding for this research was provided by the Agence Nationale de la Recherche (ANR starting grant VAHINE ANR-13-JS06-0002) to SB, INSU/LEFE/CYBER program and the TRICHOTOX (P2R) project. SB was funded by IRD. We are grateful to M. Benavides and the reviewers for the valuable comments on the manuscript.
Supplementary Material
The Supplementary Material for this article can be found online at: http://journal.frontiersin.org/article/10.3389/fmars.2015.00080
References
Agawin, N. S. R., Rabouille, S., Veldhuis, M. J. W., Servatius, L., Hol, S., van Overzee, H. M. J., et al. (2007). Competition and facilitation between unicellular nitrogen-fixing cyanobacteria and non-nitrogen-fixing phytoplankton species. Limnol. Oceanogr. 52, 2233–2248. doi: 10.4319/lo.2007.52.5.2233
Aminot, A., and Kérouel, R. (2007). Dosage Automatique des Nutriments dans les Eaux Marines. Plouzané: Ifremer.
Benavides, M., Agawin, N., Arístegui, J., Ferriol, P., and Stal, L. (2011). Nitrogen fixation by Trichodesmium and small diazotrophs in the subtropical northeast Atlantic. Aquat. Microb. Ecol. 65, 43–53. doi: 10.3354/ame01534
Benavides, M., Agawin, N., Arístegui, J., Peene, J., and Stal, L. (2013a). Dissolved organic nitrogen and carbon release by a marine unicellular diazotrophic cyanobacterium. Aquat. Microb. Ecol. 69, 69–80. doi: 10.3354/ame01621
Benavides, M., Bronk, D. A., Agawin, N. S. R., Pérez-Hernández, M. D., Hernández-Guerra, A., and Arístegui, J. (2013b). Longitudinal variability of size-fractionated N2 fixation and DON release rates along 24.5°N in the subtropical North Atlantic. J. Geophys. Res. Ocean 118, 3406–3415. doi: 10.1002/jgrc.20253
Berman, T. (1999). Algal growth on organic compounds as nitrogen sources. J. Plankton Res. 21, 1423–1437. doi: 10.1093/plankt/21.8.1423
Berman-Frank, I., Bidle, K. D., Haramaty, L., and Falkowski, P. G. (2004). The demise of the marine cyanobacterium, Trichodesmium spp., via an autocatalyzed cell death pathway. Limnol. Oceanogr. 49, 997–1005. doi: 10.4319/lo.2004.49.4.0997
Bonnet, S., Biegala, I. C., Dutrieux, P., Slemons, L. O., and Capone, D. G. (2009). Nitrogen fixation in the western equatorial Pacific: rates, diazotrophic cyanobacterial size class distribution, and biogeochemical significance. Global Biogeochem. Cycles 23, 1–13. doi: 10.1029/2008GB003439
Bronk, D., and Glibert, P. (1991). A 15N tracer method for the measurement of dissolved organic nitrogen release by phytoplankton. Mar. Ecol. Prog. Ser. 77, 171–182. doi: 10.3354/meps077171
Bronk, D. A., See, J. H., Bradley, P., and Killberg, L. (2007). DON as a source of bioavailable nitrogen for phytoplankton. Biogeosciences 4, 283–296. doi: 10.5194/bg-4-283-2007
Capone, D. G., Burns, J. A., Montoya, J. P., Subramaniam, A., Mahaffey, C., Gunderson, T., et al. (2005). Nitrogen fixation by Trichodesmium spp.: An important source of new nitrogen to the tropical and subtropical North Atlantic Ocean. Global Biogeochem. Cycles 19:Gb2024. doi: 10.1029/2004GB002331
Capone, D. G., Ferrier, M. D., and Carpenter, E. J. (1994). Amino acid cycling in colonies of the planktonic marine cyanobacterium Trichodesmium thiebautii. Appl. Environ. Microbiol. 60, 3989–3995.
Capone, D. G., Zehr, J. P., Paerl, H. W., Bergman, B., and Carpenter, E. J. (1997). Trichodesmium, a globally significant marine cyanobacterium. Science 276, 1221–1229. doi: 10.1126/science.276.5316.1221
Carpenter, E. J., Bergman, B., Dawson, R., Siddiqui, P. J. A., Söderbäck, E., and Capone, D. G. (1992). Glutamine synthetase and nitrogen cycling in colonies of the marine diazotrophic cyanobacteria Trichodesmium spp. Appl. Environ. Microbiol. 58, 3122–3129.
Chen, Y.-B., Zehr, J. P., and Mellon, M. (1996). Growth and nitrogen fixation of the diazotrophic filamentous nonheterocystous cyanobacterium Trichodesmium sp. IMS 101 in defined media: evidence for a circadian rhythm. J. Phycol. 32, 916–923. doi: 10.1111/j.0022-3646.1996.00916.x
Dekaezemacker, J., and Bonnet, S. (2011). Sensitivity of N2 fixation to combined nitrogen forms (NOand NH) in two strains of the marine diazotroph Crocosphaera watsonii (Cyanobacteria). Mar. Ecol. Prog. Ser. 438, 33–46. doi: 10.3354/meps09297
Deutsch, C., Sarmiento, J. L., Sigman, D. M., Gruber, N., and Dunne, J. P. (2007). Spatial coupling of nitrogen inputs and losses in the ocean. Nature 445, 163–167. doi: 10.1038/nature05392
Devassy, V. P., Bhattathiri, P. M. A., and Qasim, S. Z. (1979). Succession of organisms following Trichodesmium phenomenon. Indian J. Mar. Sci. 8, 89–93.
Dron, A., Rabouille, S., Claquin, P., Le Roy, B., Talec, A., and Sciandra, A. (2012). Light-dark (12:12) cycle of carbon and nitrogen metabolism in Crocosphaera watsonii WH8501: relation to the cell cycle. Environ. Microbiol. 14, 967–981. doi: 10.1111/j.1462-2920.2011.02675.x
Dudek, N., Brzezinski, M. A., and Wheeler, P. A. (1986). Recovery of ammonium nitrogen by solvent extraction for the determination of relative 15N abundance in regeneration experiments. Mar. Chem. 18, 59–69. doi: 10.1016/0304-4203(86)90076-9
Falkowski, P. G. (1983). “Enzymology of Nitrogen Assimilation,” in Nitrogen in the Marine Environment, eds Carpenter, E. J., Capone, D. G. (New york, NY: Elsevier Academic Press), 839–868.
Fay, P. (1992). Oxygen relations of nitrogen fixation in cyanobacteria. Microbiol. Rev. 56, 340–373.
Feng, X., Bandyopadhyay, A., Berla, B., Page, L., Wu, B., Pakrasi, H. B., et al. (2010). Mixotrophic and photoheterotrophic metabolism in Cyanothece sp. ATCC 51142 under continuous light. Microbiology 156, 2566–2574. doi: 10.1099/mic.0.038232-0
Flynn, K. J., and Gallon, J. R. (1990). Changes in intracellular and extracellular α-amino acids in Gloeothece during N2-fixation and following addition of ammonium. Arch. Microbiol. 153, 574–579. doi: 10.1007/BF00245267
Foster, R. A., Sztejrenszus, S., and Kuypers, M. M. (2013). Measuring carbon and N2 fixation in field populations of colonial and free-living unicellular cyanobacteria using nanometer-scale secondary ion mass spectrometry 1. J. Phycol. 49, 502–516. doi: 10.1111/jpy.12057
Fuhrman, J. A. (1999). Marine viruses and their biogeochemical and ecological effects. Nature 399, 541–548. doi: 10.1038/21119
Fukuda, R., Ogawa, H., Nagata, T., and Koike, I. (1998). Direct determination of carbon and nitrogen contents of natural bacterial assemblages in marine environments. Appl. Envir. Microbiol. 64, 3352–3358.
Gallon, J. R. (1992). Reconciling the Incompatible: N2 Fixation and O2. New Phytol. 122, 571–609. doi: 10.1111/j.1469-8137.1992.tb00087.x
Glibert, P. M., and Bronk, D. A. (1994). Release of dissolved organic nitrogen by marine diazotrophic cyanobacteria Trichodesmium spp. Appl. Environ. Microbiol. 60, 3996–4000.
Großkopf, T., Mohr, W., Baustian, T., Schunck, H., Gill, D., Kuypers, M. M. M., et al. (2012). Doubling of marine dinitrogen-fixation rates based on direct measurements. Nature 488, 361–364. doi: 10.1038/nature11338
Guber, N. (2008). “The marine nitrogen cycle: overview and challenges,” in Nitrogen in the Marine Environment, eds Capone, D. G., Bronk, D. A., Mulholland, M. R., Carpenter, E. J. (San Diego, CA: Elsevier), 1–50.
Hewson, I., Govil, S., Capone, D., Carpenter, E., and Fuhrman, J. (2004). Evidence of Trichodesmium viral lysis and potential significance for biogeochemical cycling in the oligotrophic ocean. Aquat. Microb. Ecol. 36, 1–8. doi: 10.3354/ame036001
Holl, C. M., Joseph, P., and Montoya, J. P. (2005). Interactions between nitrate uptake and nitrogen fixation in continuous cultures of the marine diazotroph Trichodesmium (Cyanobacteria). J. Phycol. 41, 1178–1183. doi: 10.1111/j.1529-8817.2005.00146.x
Holl, C. M., and Montoya, J. P. (2008). Diazotrophic growth of the marine cyanobacterium Trichodesmium IMS101 in continuous culture: effects of growth rate on N2-fixation rate, biomass, and C:N:P stoichiometry. J. Phycol. 44, 929–937. doi: 10.1111/j.1529-8817.2008.00534.x
Holmes, R. M., Aminot, A., Kérouel, R., Hooker, B. A., and Peterson, B. J. (1999). A simple and precise method for measuring ammonium in marine and freshwater ecosystems. Can. J. Fish. Aquat. Sci. 56, 1801–1808. doi: 10.1139/f99-128
Holmes, R. M., McClelland, J. W., Sigman, D. M., Fry, B., and Peterson, B. J. (1998). Measuring NH in marine, estuarine and fresh waters: an adaptation of the ammonia diffusion method for samples with low ammonium concentrations. Mar. Chem. 60, 235–243. doi: 10.1016/S0304-4203(97)00099-6
Kana, T. M., Darkangelo, C., Hunt, M. D., Oldham, J. B., Bennett, G. E., and Cornwell, J. C. (1994). Membrane inlet mass spectrometer for rapid high-precision determination of N2, O2, and Ar in environmental water samples. Anal. Chem. 66, 4166–4170. doi: 10.1021/ac00095a009
Karl, D., Letelier, R., Tupas, L., Dore, J., Christian, J., and Hebel, D. (1997). The role of nitrogen fixation in biogeochemical cycling in the subtropical North Pacific Ocean. Nature 388, 533–538. doi: 10.1038/41474
Klawonn, I., Bonaglia, S., Brüchert, V., and Ploug, H. (2015). Aerobic and anaerobic nitrogen transformation processes in N2-fixing cyanobacterial aggregates. ISME J. 9, 1456–1466. doi: 10.1038/ismej.2014.232
Kleiner, D. (1985). Bacterial ammonium transport. FEMS Microbiol. Rev. 1, 87–100. doi: 10.1111/j.1574-6968.1985.tb01185.x
Knapp, A., Dekaezemacker, J., Bonnet, S., Sohm, J., and Capone, D. (2012). Sensitivity of Trichodesmium erythraeum and Crocosphaera watsonii abundance and N2 fixation rates to varying NO and PO concentrations in batch cultures. Aquat. Microb. Ecol. 66, 223–236. doi: 10.3354/ame01577
Konno, U., Tsunogai, U., Komatsu, D. D., Daita, S., Nakagawa, F., Tsuda, A., et al. (2010). Determination of total N2 fixation rates in the ocean taking into account both the particulate and filtrate fractions. Biogeosciences 7, 2369–2377. doi: 10.5194/bg-7-2369-2010
LaRoche, J., and Breitbarth, E. (2005). Importance of the diazotrophs as a source of new nitrogen in the ocean. J. Sea Res. 53, 67–91. doi: 10.1016/j.seares.2004.05.005
Lee Chen, Y., Tuo, S., and Chen, H. (2011). Co-occurrence and transfer of fixed nitrogen from Trichodesmium spp. to diatoms in the low-latitude Kuroshio Current in the NW Pacific. Mar. Ecol. Prog. Ser. 421, 25–38. doi: 10.3354/meps08908
Lenes, J. M., Darrow, B. P., Cattrall, C., Heil, C. A., Callahan, M., Vargo, G. A., et al. (2001). Iron fertilization and the Trichodesmium response on the West Florida shelf. Limnol. Oceanogr. 46, 1261–1277. doi: 10.4319/lo.2001.46.6.1261
Lenes, J. M., and Heil, C. A. (2010). A historical analysis of the potential nutrient supply from the N2 fixing marine cyanobacterium Trichodesmium spp. to Karenia brevis blooms in the eastern Gulf of Mexico. J. Plankton Res. 32, 1421–1431. doi: 10.1093/plankt/fbq061
Luo, Y.-W., Doney, S. C., Anderson, L. A., Benavides, M., Berman-Frank, I., Bode, A., et al. (2012). Database of diazotrophs in global ocean: abundance, biomass and nitrogen fixation rates. Earth Syst. Sci. Data 4, 47–73. doi: 10.5194/essd-4-47-2012
Masuda, T., Furuya, K., Kodama, T., Takeda, S., and Harrison, P. J. (2013). Ammonium uptake and dinitrogen fixation by the unicellular nanocyanobacterium Crocosphaera watsonii in nitrogen-limited continuous cultures. Limnol. Oceanogr. 58, 2029–2036. doi: 10.4319/lo.2013.58.6.2029
McCarthy, J. J., and Bronk, D. A. (2008). “Analytical methods for nitrogen chemical characterization and flux rates,” in Nitrogen in the Marine Environment, eds Capone, D. G., Bronk, D. A., Mulholland, M. R., Carpenter, E. J. (New York, NY: Elsevier Academic Press), 1219–1276.
McClelland, J. W., Holl, C. M., and Montoya, J. P. (2003). Relating low d15N values of zooplankton to N2-fixation in the tropical North Atlantic: insights provided by stable isotope ratios of amino acids. Deep. Sea Res. Part I Oceanogr. Res. Pap. 50, 849–861. doi: 10.1016/S0967-0637(03)00073-6
Meador, T. B., Aluwihare, L. I., and Mahaffey, C. (2007). Isotopic heterogeneity and cycling of organic nitrogen in the oligotrophic ocean. Limnol. Oceanogr. 52, 934–947. doi: 10.4319/lo.2007.52.3.0934
Mohr, W., Grosskopf, T., Wallace, D. W. R., and Laroche, J. (2010). Methodological underestimation of oceanic nitrogen fixation rates. PLoS ONE 5:e12583. doi: 10.1371/journal.pone.0012583
Mohr, W., Vagner, T., Kuypers, M. M. M., Ackermann, M., and LaRoche, J. (2013). Resolution of conflicting signals at the single-cell level in the regulation of cyanobacterial photosynthesis and nitrogen fixation. PLoS ONE 8:e66060. doi: 10.1371/journal.pone.0066060
Moisander, P. H., Beinart, R. A., Hewson, I., White, A. E., Johnson, K. S., Carlson, C. A., et al. (2010). Unicellular cyanobacterial distributions broaden the oceanic N2 fixation domain. Science 327, 1512–1514. doi: 10.1126/science.1185468
Mompeán, C., Bode, A., Benítez-Barrios, V. M., Domínguez-Yanes, J. F., Escánez, J., and Fraile-Nuez, E. (2013). Spatial patterns of plankton biomass and stable isotopes reflect the influence of the nitrogen-fixer Trichodesmium along the subtropical North Atlantic. J. Plankton Res. 35, 513–525. doi: 10.1093/plankt/fbt011
Montoya, J. P., Carpenter, E. J., and Capone, D. G. (2002). Nitrogen fixation and nitrogen isotope abundances in zooplankton of the oligotrophic North Atlantic. Limnol. Oceanogr. 47, 1617–1628. doi: 10.4319/lo.2002.47.6.1617
Montoya, J. P., Holl, C. M., Zehr, J. P., Hansen, A., Villareal, T. A., and Capone, D. G. (2004). High rates of N2 fixation by unicellular diazotrophs in the oligotrophic Pacific Ocean. Nature 430, 1027–1032. doi: 10.1038/nature02824
Montoya, J. P., Voss, M., Kahler, P., and Capone, D. G. (1996). A simple, high-precision, high-sensitivity tracer assay for N2 fixation. Appl. Environ. Microbiol. 62, 986–993.
Moutin, T., Karl, D. M., Duhamel, S., Rimmelin, P., Raimbault, P., Van Mooy, B. A. S., et al. (2008). Phosphate availability and the ultimate control of new nitrogen input by nitrogen fixation in the tropical Pacific Ocean. Biogeosciences 5, 95–109. doi: 10.5194/bg-5-95-2008
Mulholland, M., Bronk, D., and Capone, D. (2004). Dinitrogen fixation and release of ammonium and dissolved organic nitrogen by Trichodesmium IMS101. Aquat. Microb. Ecol. 37, 85–94. doi: 10.3354/ame037085
Mulholland, M., and Capone, D. (1999). Nitrogen fixation, uptake and metabolism in natural and cultured populations of Trichodesmium spp. Mar. Ecol. Prog. Ser. 188, 33–49. doi: 10.3354/meps188033
Mulholland, M., and Capone, D. (2001). Stoichiometry of nitrogen and carbon utilization in cultured populations of Trichodesmium IMS101: implications for growth. Limnol. Oceanogr. 46, 436–443. doi: 10.4319/lo.2001.46.2.0436
Mulholland, M. R. (2007). The fate of nitrogen fixed by diazotrophs in the ocean. Biogeosciences 4, 37–51. doi: 10.5194/bg-4-37-2007
Mulholland, M. R., and Bernhardt, P. W. (2005). The effect of growth rate, phosphorus concentration, and temperature on N2 fixation, carbon fixation, and nitrogen release in continuous cultures of Trichodesmium IMS101. Limnol. Oceanogr. 50, 839–849. doi: 10.4319/lo.2005.50.3.0839
Mulholland, M. R., Bernhardt, P. W., Ozmon, I., Procise, L. A., Garrett, M., O'Neil, J. M., et al. (2014). Contribution of diazotrophy to nitrogen inputs supporting Karenia brevis blooms in the Gulf of Mexico. Harmful Algae 38, 20–29. doi: 10.1016/j.hal.2014.04.004
Mulholland, M. R., and Capone, D. G. (2000). The nitrogen physiology of the marine N2-fixing cyanobacteria Trichodesmium spp. Trends Plant Sci. 5, 148–153. doi: 10.1016/S1360-1385(00)01576-4
Mulholland, M. R., Ohki, K., and Capone, D. G. (1999). Nitrogen Utilization and Metabolism Relative To Patterns of N2 Fixation in Cultures of Trichodesmium NIBB1067. J. Phycol. 35, 977–988. doi: 10.1046/j.1529-8817.1999.3550977.x
O'Neil, J., Metzler, P., and Glibert, P. (1996). Ingestion of 15N2-labelled Trichodesmium spp. and ammonium regeneration by the harpacticoid copepod Macrosetella gracilis. Mar. Biol. 125, 89–96.
O'Neil, J., and Roman, M. (1992). “Grazers and associated organisms of trichodesmium,” in Marine Pelagic Cyanobacteria: Trichodesmium and Other Diazotrophs NATO ASI Series, eds Carpenter, E. J., Capone, D. G., Rueter, J. G. (New York, NY: Springer), 61–73.
Orcutt, K. M., Lipschultz, F., Gundersen, K., Arimoto, R., Michaels, A. F., Knap, A. H., et al. (2001). A seasonal study of the significance of N2 fixation by Trichodesmium spp. at the Bermuda Atlantic Time-series Study (BATS) site. Deep Sea Res. Part II Top. Stud. Oceanogr. 48, 1583–1608. doi: 10.1016/S0967-0645(00)00157-0
Paerl, H. W., and Priscu, J. C. (1998). Microbial phototrophic, heterotrophic, and diazotrophic activities associated with aggregates in the permanent ice cover of Lake Bonney, Antarctica. Microb. Ecol. 36, 221–230. doi: 10.1007/s002489900109
Ploug, H., Musat, N., Adam, B., Moraru, C. L., Lavik, G., Vagner, T., et al. (2010). Carbon and nitrogen fluxes associated with the cyanobacterium Aphanizomenon sp. in the Baltic Sea. ISME J. 4, 1215–1223. doi: 10.1038/ismej.2010.53
Prufert-Bebout, L., Paerl, H. W., and Lassen, C. (1993). Growth, nitrogen fixation, and spectral attenuation in cultivated Trichodesmium species. Appl. Environ. Microbiol. 59, 1367–1375.
Pujo-Pay, M., and Raimbault, P. (1994). improvement of the wet-oxidation procedure for simultaneous determination of particulate organic nitrogen and phosphorus collected on filters. Mar. Ecol. Prog. Ser. 105, 203–207. doi: 10.3354/meps105203
Raimbault, P., and Garcia, N. (2008). Evidence for efficient regenerated production and dinitrogen fixation in nitrogen-deficient waters of the South Pacific Ocean: impact on new and export production. Biogeosciences 5, 323–338. doi: 10.5194/bg-5-323-2008
Raimbault, P., Slawyk, G., Boudjellal, B., Coatanoan, C., Conan, P., Coste, B., et al. (1999). Carbon and nitrogen uptake and export in the equatorial Pacific at 150°W: evidence of an efficient regenerated production cycle. J. Geophys. Res. 104, 3341. doi: 10.1029/1998JC900004
Reddy, K. J., Haskell, J. B., Sherman, D. M., and Sherman, L. A. (1993). Unicellular, aerobic nitrogen-fixing cyanobacteria of the genus Cyanothece. J. Bacteriol. 175, 1284–1292.
Romans, K. M., Carpenter, E. J., and Bergman, B. (1994). Buoyancy regulation in the colonial diazotrophic cyanobacterium Trichodesmium tenue: ultrastructure and storage of carbohydrate, polyphosphate, and nitrogen. J. Phycol. 30, 935–942. doi: 10.1111/j.0022-3646.1994.00935.x
Sherman, L., Meunier, P., and Colón-López, M. (1998). Diurnal rhythms in metabolism: a day in the life of a unicellular, diazotrophic cyanobacterium. Photosyn. Res. 58, 25–42. doi: 10.1023/A:1006137605802
Sigman, D. M., Casciotti, K. L., Andreani, M., Barford, C., Galanter, M., and Böhlke, J. K. (2001). A bacterial method for the nitrogen isotopic analysis of nitrate in seawater and freshwater. Anal. Chem. 73, 4145–4153. doi: 10.1021/ac010088e
Slawyk, G., and Raimbault, P. (1995). Simple procedure for simultaneous recovery of dissolved inorganic and organic nitrogen in 15N-tracer experiments and improving the isotopic mass balance. Mar. Ecol. Prog. Ser. 124, 289–299. doi: 10.3354/meps124289
Sohm, J. A., Edwards, B. R., Wilson, B. G., and Webb, E. A. (2011). Constitutive extracellular eolysaccharide (EPS) production by specific isolates of Crocosphaera watsonii. Front. Microbiol. 2:229. doi: 10.3389/fmicb.2011.00229
Thompson, A. W., and Zehr, J. P. (2013). Cellular interactions: lessons from the nitrogen-fixing cyanobacteria. J. Phycol. 49, 1024–1035. doi: 10.1111/jpy.12117
Tsunogai, U., Kido, T., Hirota, A., Ohkubo, S. B., Komatsu, D. D., and Nakagawa, F. (2008). Sensitive determinations of stable nitrogen isotopic composition of organic nitrogen through chemical conversion into N2O. Rapid Commun. Mass Spectrom. 22, 345–354. doi: 10.1002/rcm.3368
Van Dommelen, A., Keijers, V., Vanderleyden, J., and de Zamaroczy, M. (1998). (Methyl)ammonium transport in the nitrogen-fixing bacterium Azospirillum brasilense. J. Bacteriol. 180, 2652–2659.
Villareal, T. A., and Carpenter, E. J. (1990). Diel buoyancy regulation in the marine diazotrophic cyanobacterium Trichodesmium thiebautii. Limnol. Oceanogr. 35, 1832–1837. doi: 10.4319/lo.1990.35.8.1832
Wannicke, N., Koch, B., and Voss, M. (2009). Release of fixed N2 and C as dissolved compounds by Trichodesmium erythreum and Nodularia spumigena under the influence of high light and high nutrient (P). Aquat. Microb. Ecol. 57, 175–189. doi: 10.3354/ame01343
Warembourg, F. R. (1993). “Nitrogen isotope techniques,” in Nitrogen Isotope Techniques, eds Knowles, R., Paul, E. A., Melillo, J., Blackburn, H. (Amsterdam: Elsevier), 127–156.
Waterbury, J. B., and Willey, J. M. (1988). “Isolation and growth of marine planktonic cyanobacteria,” in Methods in Enzymology, eds Packer, L., Glazer, A. N. (New York, NY: Elsevier Academic Press), 100–105.
Webb, E. A., Ehrenreich, I. M., Brown, S. L., Valois, F. W., and Waterbury, J. B. (2009). Phenotypic and genotypic characterization of multiple strains of the diazotrophic cyanobacterium, Crocosphaera watsonii, isolated from the open ocean. Environ. Microbiol. 11, 338–348. doi: 10.1111/j.1462-2920.2008.01771.x
White, A. E., Foster, R. A., Benitez-Nelson, C. R., Masqué, P., Verdeny, E., Popp, B. N., et al. (2012). Nitrogen fixation in the Gulf of California and the Eastern Tropical North Pacific. Prog. Oceanogr. 109, 1–17. doi: 10.1016/j.pocean.2012.09.002
Wilson, S. T., Böttjer, D., Church, M. J., and Karl, D. M. (2012). Comparative assessment of nitrogen fixation methodologies, conducted in the oligotrophic North Pacific Ocean. Appl. Environ. Microbiol. 78, 6516–6523. doi: 10.1128/AEM.01146-12
Zehr, J. P., Jenkins, B. D., Short, S. M., and Steward, G. F. (2003). Nitrogenase gene diversity and microbial community structure: a cross-system comparison. Environ. Microbiol. 5, 539–554. doi: 10.1046/j.1462-2920.2003.00451.x
Zehr, J. P., Mellon, M. T., and Zani, S. (1998). New nitrogen-fixing microorganisms detected in oligotrophic oceans by amplification of nitrogenase (nif H) genes. Appl. Environ. Microbiol. 64, 5067.
Keywords: N2 fixation, excretion, dissolved organic nitrogen, Trichodesmium, Cyanothece, Crocosphaera
Citation: Berthelot H, Bonnet S, Camps M, Grosso O and Moutin T (2015) Assessment of the dinitrogen released as ammonium and dissolved organic nitrogen by unicellular and filamentous marine diazotrophic cyanobacteria grown in culture. Front. Mar. Sci. 2:80. doi: 10.3389/fmars.2015.00080
Received: 05 June 2015; Accepted: 23 September 2015;
Published: 23 October 2015.
Edited by:
Javier Arístegui, University of Las Palmas de Gran Canaria, SpainReviewed by:
Dirk De Beer, Max Planck Institute for Marine Microbiology, GermanyTravis Blake Meador, University of Bremen, Germany
Nicola Wannicke, Leibniz-Institut für Ostseeforschung Warnemünde, Germany
Deborah Ann Bronk, Virginia Institute of Marine Science, USA
Copyright © 2015 Berthelot, Bonnet, Camps, Grosso and Moutin. This is an open-access article distributed under the terms of the Creative Commons Attribution License (CC BY). The use, distribution or reproduction in other forums is permitted, provided the original author(s) or licensor are credited and that the original publication in this journal is cited, in accordance with accepted academic practice. No use, distribution or reproduction is permitted which does not comply with these terms.
*Correspondence: Hugo Berthelot, Aix Marseille Université, Centre National de la Recherche Scientifique/INSU, Université de Toulon, IRD, Mediterranean Institute of Oceanography, UM 110, Campus de Luminy, Bâtiment Oceanomed, 13288 Marseille, France, hugo.berthelot@mio.osupytheas.fr