- 1The Australian Institute of Marine Science, Crawley, WA, Australia
- 2Marine Science Institute, The University of Texas at Austin, Port Aransas, TX, USA
- 3Department of Marine Biology, Texas A&M University, Galveston, TX, USA
The largest animals in the oceans eat prey that are orders of magnitude smaller than themselves, implying strong selection for cost-effective foraging to meet their energy demands. Whale sharks (Rhincodon typus) may be especially challenged by warm seas that elevate their metabolism and contain sparse prey resources. Using a combination of biologging and satellite tagging, we show that whale sharks use four strategies to save energy and improve foraging efficiency: (1) fixed, low power swimming, (2) constant low speed swimming, (3) gliding, and (4) asymmetrical diving. These strategies increase foraging efficiency by 22–32% relative to swimming horizontally and resolve the energy-budget paradox of whale sharks. However, sharks in the open ocean must access food resources that reside in relatively cold waters (up to 20°C cooler than the surface) at depths of 250–500 m during the daytime, where long, slow gliding descents, continuous ram ventilation of the gills and filter-feeding could rapidly cool the circulating blood and body tissues. We suggest that whale sharks may overcome this problem through their large size and a specialized body plan that isolates highly vascularized red muscle on the dorsal surface, allowing heat to be retained near the center of the body within a massive core of white muscle. This could allow a warm-adapted species to maintain enhanced function of organs and sensory systems while exploiting food resources in deep, cool water.
Introduction
Through convergent evolution, the three largest species of living sharks and all balaenid whales feed by sieving large volumes of sea water through specialized cranial morphology to extract planktonic and small nektonic prey (Pivorunas, 1979; Taylor et al., 1983). The extreme mass of these sharks and whales and the contrasting small body mass of their food items require that they regularly consume enormous numbers of prey. This demand is especially high for endothermic cetaceans in polar seas that must allocate most of their ingested energy to maintain a core body temperature of 37°C that may be 25–38°C warmer than the surrounding sea water. Even though the enhanced productivity of temperate and polar seas relative to the tropics provides for the high energy demands of the great whales, there is evidence that their populations are limited by the availability of prey (Hunt, 2006). Metabolic rates of two ectothermic filter-feeding sharks, the basking shark (Cetorhinus maximus), and the megamouth shark (Megachasma pelagios), are likely reduced by the cold temperatures of their high-latitude or deep ocean habitats. Whale sharks (Rhincodon typus), however, reside in warm oceans, which elevates their metabolism relative to the other filter-feeding sharks. The challenge for whale sharks of meeting this higher energetic demand is amplified by the typically oligotrophic conditions of tropical and subtropical seas.
These large sharks and whales primarily use continuous ram filtration (Colman, 1997; Goldbogen et al., 2006; Simon et al., 2009) or lunge feeding (Goldbogen et al., 2008; Nakaya et al., 2008) to gather food, but both methods are energetically expensive because of the enormous increase in hydrodynamic drag when they open their mouth while swimming (Sims, 1999; Goldbogen et al., 2007). In addition to continuous ram filtration, whale sharks can also use suction feeding while remaining stationary when prey is aggregated into dense patches (Compagno, 1984, 1990; Clark and Nelson, 1997), although this technique is energetically inefficient when prey concentrations are low. This inefficiency has led to the suggestion that whale sharks are even more dependent on dense aggregations of prey than other filter-feeding sharks (Compagno, 1984). Since prey aggregations vary widely in space and time, searching widely to locate and then harvest patches of prey greatly escalates the energetic challenges for these animals and is expected to generate strong selective pressure for cost-efficient foraging. The daily vertical migrations of oceanic plankton from warmer surface waters by night to colder, deeper waters during the daytime impose an additional physiological challenge on warm-adapted whale sharks. We used a combination of biologging and satellite tagging studies to provide insights into the factors that drive behavior in whale sharks and show that a specialized body plan that conserves environmentally-derived heat may resolve the conflict between the need for energy-efficiency and access to food resources in cold, deep water.
Methods
Biologging
We studied two whale sharks (7 and 4 m total length) in the vicinity of Point Cloates (113° 59′ 7.21″ E, 21° 54′ 46.17″ S), Ningaloo Reef in Western Australia in April 2009 (see Wilson et al., 2006 for location map). We attached a buoyant digital video and data logger (see Supplementary Material for specifications, calibrations of sensors and video from the deployments) to each shark by a 1-m long steel tether at the end of which was a titanium dart that was embedded in the sub-dermal layer at the base of the dorsal fin. The tether contained a fusible link that dissolved after ca. 16 h in sea water, so that the instrument and tether floated to the surface and could be retrieved. The field of view of the video camera (recording at 30 Hz) included the top of the shark's head from the gill slits forward and the area above and in front of its head. The data logger included an accelerometer (reporting at 20 Hz) and a magnetometer (1 Hz) that recorded static and dynamic accelerations of the instrument and the strength of Earth's magnetic field on three orthogonal axes. Additional sensors recorded time, depth, and speed (at 1 Hz), and a GPS receiver recorded longitude and latitude when the instrument's antenna was above the water surface. Using these data, we reconstructed geo-referenced three-dimensional swimming paths for each shark (Davis et al., 1999) on the coastal shelf off the reef front in water depths of 30–100 m. We also used the accelerometers to determine the frequency and amplitude of the unit's lateral movements caused by the shark's propulsive strokes and validated this by the video record.
Biologging Analysis
Tail-beat amplitude and frequency were determined from the output of the X-axis accelerometer, which was aligned perpendicular to both the longitudinal axis of the instrument and the gravitational axis. Data from this accelerometer traced a sinusoidal path over time while the shark was actively swimming (verified by observing yaw of the head on the video recordings). Output from this accelerometer was converted to units of gravitational force (N) then to m s−2 using data from calibrations performed in the laboratory and corrected for the geomagnetic field at the deployment site. The dynamic component of the accelerometer signal created by the lateral movement of the instrument was obtained by passing a 15-s moving average filter through the data stream to remove the static (gravitational) component of acceleration. The magnitude of lateral movements of the instrument was calculated from the dynamic acceleration by applying a 15-s moving standard deviation filter. Histograms of these standard deviations revealed two separated peaks for each shark. Observations within the lower peak (smaller standard deviations) were interpreted as instrument wobble during gliding. Larger values of the 15-s moving standard deviation were interpreted as lateral swings of the instrument resulting from the shark's propulsive strokes. Tail-beat frequency (s−1) was calculated from the X-axis dynamic acceleration data stream as the inverse of the time between consecutive values of zero acceleration. Tail-beat amplitude (in m) was calculated as two times the second anti-derivative of a sine function representing dynamic acceleration, using the amplitude of dynamic acceleration and frequency. This estimate of tail-beat amplitude was considered to be proportional to the lateral distance traversed by the tail during each propulsive stroke, but because the attachment point differed for the two sharks, direct comparisons of amplitude values were not made. Glides were defined as cessation of propulsive stroking for a period of more than three tail beats, based on the modal tail-beat frequency for each shark. The thresholds for glides were 4.8 s for the 7-m shark and 10 s for the 4-m shark.
Surface waves created anomalous readings from the speed sensor while the sharks were at shallow depths. Therefore, analyses pertain to periods when the sharks were more than 2 m below the surface. In order to calculate changes in position while near the surface, swimming speed was estimated by interpolating values immediately before and immediately after surface intervals.
Satellite Tagging
We attached a SPLASH tag (Wildlife Computers, Redmond, Washington, USA) using a one-meter tether and fin clasp to the dorsal fin of a male whale shark (5 m total length) at Christmas Island (10°29′ S, 105°57′ E) during January 2008. The tag sampled depth (± 0.5 m), light and temperature (± 0.05°C) every 60 s for the duration of the 88 days of deployment giving 185 position estimates of Argos classes 1–3. Depths in the habitats occupied by the shark were estimated using the GEBCO gridded bathymetry data (http://www.gebco.net/data_and_products/gridded_bathymetry_data/) provided by Google Earth (http://earth.google.com/). Sequences longer than 1 day in which the shark was consistently above bottom depths of < 200 or > 200 m were designated as “shelf” and “open ocean,” respectively.
Results
Biologging
The two sharks exhibited strikingly similar locomotor habits despite a nearly two-fold difference in length and an estimated five-fold difference in body mass. The sharks spent part of the time (16–41%) within 5 m of the sea surface. The rest of the time they performed “dives” (descent from near the sea surface toward the bottom followed by immediate ascent) or swam horizontally at depth, usually close to the sea floor at depths of 30–70 m. The sharks tended to swim away from the reef during the morning and afternoon and toward the reef in the evening (Figure 1A).
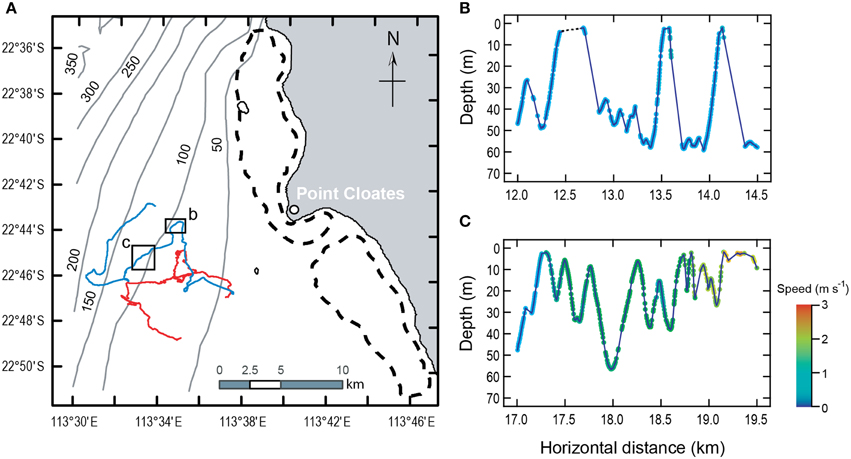
Figure 1. Movements of two whale sharks for 16–17 h at Ningaloo Reef, Western Australia. (A) Positions were dead-reckoned at 1 s intervals using speed, depth, and magnetometer information and corrected using known locations at deployment and recovery. Color of the swimming path distinguishes the whale sharks (red: 7-m long; blue: 4-m long). Arrows show direction of movement. Boxes in (A) delineate region of detail shown in (B,C). Broken lines identify Ningaloo Reef. Gray lines are bathymetric contours (numbers indicate depth in m). Both sharks swam away from the reef during the morning and afternoon and toward the reef in the evening, each covering roughly 40 km (including both vertical and horizontal movement). They spent 79.4 and 58.6% (larger and smaller shark, respectively) of the time performing “dives” (descent from near the sea surface toward the bottom followed by immediate ascent) or swimming horizontally, usually near the sea floor. The remainder of the time was within 5 m of the sea surface. Dives were characterized by slow, constant speeds, and gliding descents (B) although some isolated instances of gliding did occur on ascent. High speeds and powered descents (C) were rare. Each point in (B,C) represents depth and horizontal distance from start of deployment for a propulsive stroke. Symbol color indicates swimming speed. Gaps between points show periods of gliding.
Whale sharks took advantage of their negative buoyancy to incorporate periods of gliding into their descents (Figure 1B). The median duration of individual glides was 5–20 s (mean = 8.1–24.6 s), accounting for 10.6–17.6% of the time below the surface layer (depth > 5 m).
Dives were asymmetrical with respect to the characteristics of the descent and ascent phases (Figure 1B). Considering only those dives with a single, continuous descent followed by a single, continuous ascent to the starting depth (n = 14 for the large shark and 25 for the smaller shark), gliding represented 46–60% of the duration of descents. Swimming speeds during descent were 8–49% faster than during the subsequent ascent, but descent angles were significantly shallower than ascent angles, so that sharks traveled farther using negative buoyancy and hydrodynamic lift on descent than while actively swimming on ascent between the same depths.
During all activities away from the surface, speeds of the sharks while actively swimming (i.e., excluding gliding) were very low even for such large animals (mean = 0.58–0.81 m s−1 Figure 1C). Despite these essentially constant swimming speeds, the amplitude, and frequency of propulsive strokes varied widely (Figures 2A,B). Coefficients of variation (CV) for stroke amplitude ranged from 117 to 276% (n ≥ 6481 strokes per shark). Stroke frequency was much less variable (median = 0.35–0.65 Hz, CV = 33–41%). However, the combinations of stroke frequency and stroke amplitude used by each shark were limited to a narrow band (Figures 2C,D). Within this band of data there was a sharply defined axis of locomotor gaits, such that variation in amplitude accounted for 78–91% (R2) of the total variation in stroke frequency.
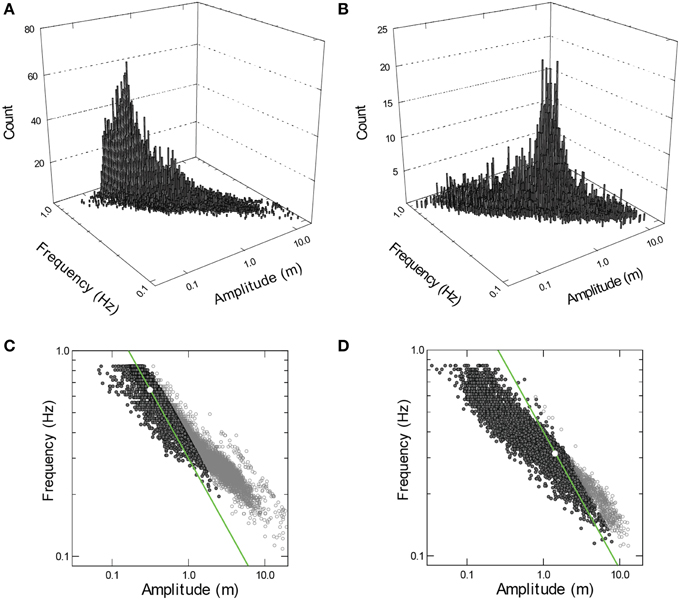
Figure 2. (A,B) Frequency histograms for combinations of propulsive stroke frequency and amplitude used by the larger (A,C; n = 11, 657 strokes) and smaller (B,D; n = 6481) whale shark. Data projected onto the horizontal planes (C,D) define a narrow band within which there is a sharply defined axis of locomotor gaits (see narrow ridges in A,B). Modal combination of frequency and amplitude is identified by a white circle. Power (P) at the caudal fin was calculated as: P = m · p · A2 · f2 · (1 - cos(f · p)), where, m is the mass of water affected by the caudal fin (assumed constant for each shark and removed from the calculation), A is amplitude (in m), and f is stroke frequency (in tail beats per second). Combinations of frequency and amplitude producing power equivalent to the power at modal frequency and amplitude are identified by a green line. Symbols outlined in black identify data P ≤ 2 times modal power.
Satellite Tagging
The deployment of the Splash tag produced tracks of the animal both while swimming over coastal shelves and in the open ocean (30 and 70% of record, respectively). In the open ocean, the whale shark used the surface layer (defined here as the upper 10 m of the water column) extensively during the day (06.00–18.00 h). Long intervals in the surface layer were punctuated by occasional descents of long duration to 300–500 m deep. At night (18.00–06.00 h), use of the upper 10 m was greatly reduced and descents generally reached depths of around 100 m (Figure 3).
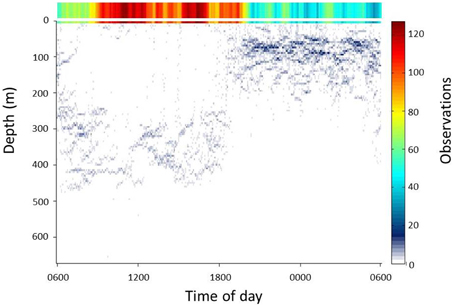
Figure 3. Summary of vertical movements of whale sharks over a 24 h cycle during times when the sharks resided in the open ocean (water depths > 200 m). Observations are color-coded relative to the total number of observations within 5 m depth intervals and each 5 min period of the 24 h cycle. The right axis shows the scale for the color coding. Observations within the top 5 m of the water column are shown enlarged at the top of the plot.
Discussion
Our biologging study showed that whale sharks took advantage of their negative buoyancy to incorporate periods of gliding into their descents, a pattern consistent with another study of this species (Gleiss et al., 2011b) and an energy-conservation tactic previously documented in marine mammals and birds (Williams et al., 2000; Davis et al., 2001; Gleiss et al., 2011a). Other tactics to conserve energy revealed by biologging included asymmetrical diving and constant low speed swimming, implying strong selective pressure for cost-efficient foraging in the species.
Slow swimming speed greatly reduces the rate of energy expenditure, but it also reduces the rate of energy gain during filter feeding or distance searched. Balancing this trade-off, theory predicts an optimal foraging speed for continuous horizontal swimming (Weihs, 1975). Assuming each shark's modal swimming speed was optimal for foraging, the narrow variability in the speed of the largest shark resulted in foraging efficiencies (energy gain divided by energy expended) ≥ 98% of the maximum value 90% of the time (Figures 4A,B).
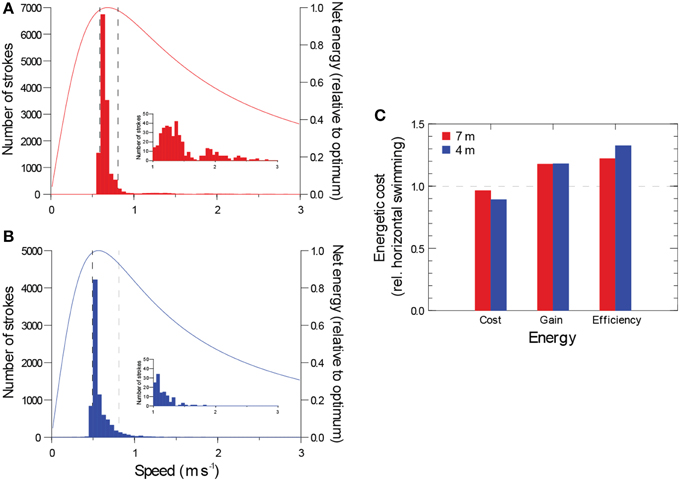
Figure 4. (A, B) Distribution of active swimming speeds for two whale sharks with the theoretical relationship between net energy while foraging for a hypothetical fish swimming constantly and horizontally (from Weihs, 1975). Insets show right tail of each distribution. Bars show number of strokes for a given swimming speed. Broken lines enclose central 90% of all strokes. (C) Estimated locomotor cost, gain in foraging volume, and net foraging efficiency achieved by diving relative to horizontal swimming (horizontal swimming = 1.0).
To gauge the energetic impact of gliding and diving, we estimated the energy savings and foraging gains they produced in comparison to a hypothetical fish swimming constantly and horizontally (Weihs, 1975). The extensive gliding and faster speeds during descents resulted in locomotor costs approximately 29% (larger shark) and 49% (smaller shark) of the cost of swimming horizontally for the same period at the slower modal horizontal swimming speed. Ascents, however, were 1.9 (larger shark) and 1.5 (smaller shark) times the cost of horizontal swimming because of the effects of gravity and speeds faster than horizontal swimming. Since descents were 34 and 49% longer in duration than ascents, the energy savings accrued during descent exceeded the penalty of the ascent, producing a small net savings in locomotor costs achieved by diving (3.6 and 10.9% for the larger and smaller shark, respectively). The difference between the two sharks was primarily due to the faster ascent speed of the larger shark relative to its horizontal speed. Diving produced a greater benefit for foraging rate (volume searched or filtered per unit of time). Faster ascent and descent speeds (vs. horizontal swimming) and the long distances traversed while gliding resulted in an 18% increase in foraging rate for both sharks. Combining the reductions in locomotor costs and the gains in foraging rate, foraging efficiency (gains divided by costs) were 22 and 32% greater for diving sharks than for horizontal swimming (Figure 4C).
Although our sample sizes were small, there is very good evidence that the results of our biologging study were typical of whale sharks. Gleiss et al. (2011b) deployed “Daily Diary” data loggers (Wilson et al., 2006) on nine sharks ranging in estimated size from 3.5 to 8 m total length at Ningaloo Reef between 2008 and 2009. These sharks displayed movement patterns identical to those recorded by our study, descending from the surface to the seabed on multiple occasions and then returning to the surface. Moreover, as was the case in our study, descents were characterized by gliding, “dives” tended to be asymmetric and the sharks swam very slowly. These consistent behaviors between studies and among sharks of varying lengths and body masses imply that the patterns we observed are likely to be typical of whale sharks.
The four tactics used by whale sharks—fixed, low power swimming; constant low speed swimming; gliding; and asymmetrical diving—may reduce their field metabolic rate and increase their rate of energy intake and foraging efficiency which could help to reconcile the energy-budget paradox (Parker and Boeseman, 1954). It is likely that the other larger filter-feeding sharks and whales use similar energetic tactics to address their common energetic challenge (Gleiss et al., 2011a). It might be expected that some of these tactics are employed by an even broader array of large animals that feed on small prey. It has been shown, however, that terrestrial carnivores ≥ 21.5 Kg are unable to meet their energetic demands by feeding on prey less than half their own mass (Carbone et al., 1999). The ability of the largest marine animals (far greater than a few tens of kilograms) to subsist on prey many orders of magnitude smaller than themselves rests both with their ability to capture large numbers of prey at one time and to elevate their foraging efficiency through the tactics identified here. These tactics are not available to large terrestrial organisms.
Satellite tagging revealed diurnal cycles in foraging by whale sharks in the open ocean. During the day, sharks remained in the surface layer with occasional descents of long duration to 300–500 m depths, but at night, use of the upper 10 m was greatly reduced and descents generally reached depths of around 100 m. These day/night patterns of vertical movement in the open ocean appear are typical of whale sharks and have been recorded by many other studies that have tagged and tracked this species both at Ningaloo and in the western Indian Ocean (e.g., Wilson et al., 2006; Brunnschweiler and Sims, 2011). Such patterns are consistent with diel cycles in the vertical distribution of zooplankton in the open ocean, which typically accumulates during the day at depths of 250–500 m to form the deep scattering layer (Hays, 2003). At night, plankton ascends to congregate at the thermocline at around 100 m (Hays, 2003).
Recent studies have shown that the descent to access food resources in the deep scattering layer during the day can expose whale sharks to temperatures more than 20°C lower than those at the surface and this has metabolic consequences for ectothermic whale sharks. The minimum temperatures on these deep dives are inversely related to the time spent basking in warmer surface waters following ascent (Thums et al., 2013), suggesting that whale sharks swim at the surface as a form of behavioral thermoregulation that allows them to warm up after losing heat while filter feeding at depths > 250 m, when the immense surface area of the gills is exposed to cold water (Thums et al., 2013). Similar patterns of behavioral thermoregulation have been recorded in other oceanic fishes, such as tunas (Thunnus obesus; Holland et al., 1992), swordfish (Takahashi et al., 2003), sunfish (Nakamura et al., 2015) leatherback turtles (Dermochelys coriacea; Hays et al., 2006; Houghton et al., 2008), and other sharks (Carey and Scharold, 1990; Klimley et al., 2002).
The energy-efficient foraging behavior of whale sharks, characterized by gliding, asymmetrical diving, and very slow swimming, may pose problems when whale sharks must move into colder water to feed. Long, slow gliding descents may cool the body because there is little muscle activity to produce heat to offset the exposure to colder water. Furthermore, the continuous ram ventilation of the gills and filter feeding at depth are likely to cool the blood and body tissues very rapidly. With their energy budget so tightly constrained by the high demand imposed by large body size and the low supply of small, scarce prey, whale sharks are unlikely to have the metabolic flexibility to increase muscle activity to produce heat that could offset this exposure to colder water, as is the case for endothermic fishes, such as tunas and lamnid sharks.
We suggest that a specialized body plan and large body mass helps whale sharks to manage the competing needs for cost-effective foraging and access to food in cold water. Their body consists of four layers (Figure 5A): (1) a massive core of poorly-vascularized white muscle surrounds the vertebral column and internal organs; (2) surrounding this core is a thin band of highly vascularized red muscle; (3) overlying the red muscle is a layer of connective tissue that can be more than 20 cm thick and; (4) the skin, which consists of a very thin but dense external layer of denticles or placoid scales. We suggest that this body plan effectively isolates the body core from the highly-vascularized red muscle used for routine activity so that heat can be retained near internal organs and the central nervous system. In effect, this body plan is the inverse of that of endothermic fishes, such as lamnid sharks (Figure 5B) and tunas where heat exchangers in the highly-vascularized body core retain metabolically-derived heat to enhance the function of organs and sensory systems while swimming in cold water (Carey et al., 1971; Dickson and Graham, 2004). Because whale sharks are relatively inactive they are unlikely to generate much metabolic heat. Instead, their body plan may allow them to retain heat obtained from the environment. Thermal inertia is likely to be a key part of this strategy and could explain why whale sharks reach such immense sizes, with the largest individual measured reliably weighing 34 metric tons and estimated to be 18 m in total length (Chen et al., 1997).
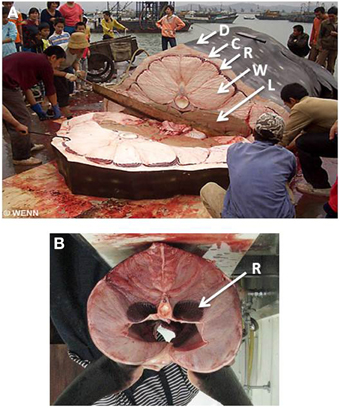
Figure 5. (A) Cross-section view of a whale shark landed at a fishing dock in southern China. Note a thin external layer of denticles or placoid scales (D), a layer of connective tissue (C) a thin band of red muscle (R), and a central core of white muscle surrounding the vertebral column (W). Lobes of the liver (L) are also visible at the top of the body cavity. (B) Cross-section view of a lamnid shark, the porbeagle (Lamna nasus) showing red muscle (R) next to body core. Note that the photograph has been rotated so that the dorsal surface of the body is uppermost. Photograph by Matthieu Deuté (Own work), via Wikimedia Commons.
Both other fishes and marine reptiles foraging in the open ocean also use “gigantothermy” as a strategy for thermoregulation. The ocean sunfish (Mola mola), the world's heaviest teleost, is diurnally active and makes frequent excursions to cool waters at depths of 100–200 m to feed on jelly plankton. These feeding bouts are interspersed by periods of re-warming at the surface and the large body mass of these animals has been shown to increase foraging time in at depth (Nakamura et al., 2015). Similarly, leatherback turtles, which also feed upon jelly plankton, are one of the largest living reptiles, growing to 2.2 m and weighing as much as 900 kg (Goff and Lien, 1988). Like whale sharks, leatherbacks use a combination of body mass and insulating layers to retain heat, although they also have specialized heat exchangers in the neck that allow them to regulate body temperature (Paladino et al., 1990). This combination of morphologies means that the species can avoid overheating in tropical climates and maintain body temperatures above ambient in cool conditions, with the result that the species has the widest distribution of any marine reptile, ranging from the tropics to the Arctic Circle (Paladino et al., 1990). In terrestrial systems, gigantothermy is also thought to be the means by which the sauropod dinosaurs, which were exceptionally large reptiles (Sander and Clauss, 2008), maintained body temperatures (Paladino et al., 1990).
Ultimately, the body plan and size of whale sharks may have been driven by the energetic constraints of feeding on prey that are small in size and have patchy distributions. This has led to a design for thermoregulation involving a specialized body plan that allows these sharks to accumulate and conserve environmentally-derived heat to access food in deep, cool water. This contrasts with other inhabitants of the open ocean such as lamnid sharks, tunas, and marine mammals that achieve the same goal by retaining metabolic heat either using heat exchangers or through an insulating layer on the outer surface of the body.
Conflict of Interest Statement
The authors declare that the research was conducted in the absence of any commercial or financial relationships that could be construed as a potential conflict of interest.
Acknowledgments
This study was made possible by funding from Apache Energy Ltd, the Nancy Lee and Perry R. Bass Endowment at The University of Texas Marine Science Institute, the Australian Government Department of Environment, Water, Heritage, and the Arts (DEWHA), Australian Institute of Marine Science, SeaWorld Research and Rescue Foundation and the Save our Seas Foundation. We thank Iain Field, Terry, and Rick Maxwell, and staff of the Western Australian Department of Parks and Wildlife (DPaW) for assistance with permitting, field work, and logistics, and Edward R. Farrell for help with data processing and analysis. William Hagey at Pisces Design fabricated the video and data recorders and the attachment system with a grant from the National Science Foundation to R. Davis. All research was done under permits issued by DEWHA and DPaW and ethics approvals from the University of Western Australia. We appreciate the comments of Andrew Esbaugh, Daniel Weihs, Terrie Williams and reviewers that greatly improved the manuscript.
Supplementary Material
The Supplementary Material for this article can be found online at: http://journal.frontiersin.org/article/10.3389/fmars.2015.00064
References
Brunnschweiler, J., and Sims, D. (2011). Diel oscillations in whale shark vertical movements associated with meso-and bathypelagic diving. Am. Fish. Soc. Symp. 76, 1–14.
Carbone, C., Mace, G. M., Roberts, S. C., and Macdonald, D. W. (1999). Energetic constraints on the diet of terrestrial carnivores. Nature 402, 286–288. doi: 10.1038/46266
Carey, E., and Scharold, J. (1990). Movements of blue sharks in course and depth. Mar. Biol. 106, 329–342. doi: 10.1007/BF01344309
Carey, F., Teal, J., Kanwisher, J., Lawson, K., and Beckett, J. (1971). Warm-bodied fish. Am. Zool. 11, 137–143. doi: 10.1093/icb/11.1.137
Chen, C. T., Liu, K. M., and Joung, S. J. (1997). Preliminary Report on Taiwan's Whale Shark Fishery. Taipei: TRAFFIC East Asia.
Clark, E., and Nelson, D. R. (1997). Young whale sharks, Rhincodon typus, feeding on a copepod bloom near La Paz, Mexico. Env. Biol. Fish. 50, 63–73. doi: 10.1023/A:1007312310127
Colman, J. (1997). A review of the biology and ecology of the whale shark. J. Fish Biol. 51, 1219–1234. doi: 10.1111/j.1095-8649.1997.tb01138.x
Compagno, L. (1984). FAO Species Catalogue, Vol. 4. Sharks of the World. An Annotated and Illustrated Catalogue of Shark Species Known to Date, Part 1, Hexanchiformes to Lamniformes. FAO Fisheries Synopsis (125) Vol. 4, Pt. 1. Rome: FAO.
Compagno, L. (1990). “Relationships of the megamouth shark, Megachasma pelagios (Lamniformes, Megachasmidae), with comments on its feeding habits,” in Elasmobranchs as Living Resources: Advances in the Biology, Ecology, Systematics and Status of the Fisheries, eds H. L. Pratt, S. H. Gruber, and T. Taniuchi (Seattle, WA: National Oceanographic and Atmospheric Administration), 97–109.
Davis, R. W., Fuiman, L. A., Williams, T. M., and Le Beouf, B. J. (2001). Three-dimensional movements and swimming activity of a northern elephant seal. Comp. Biochem. Physiol. A Mol. Integr. Physiol. 129, 759–770. doi: 10.1016/S1095-6433(01)00345-2
Davis, R. W., Fuiman, L. A., Williams, T. M., Collier, S. O., Hagey, W. P., Kanatous, S. B., et al. (1999). Hunting behavior of a marine mammal beneath the Antarctic fast-ice. Science 283, 993–996. doi: 10.1126/science.283.5404.993
Dickson, K. A., and Graham, J. B. (2004). Evolution and consequences of endothermy in fishes. Physiol. Biochem. Zool. 77, 998–1018. doi: 10.1086/423743
Gleiss, A. C., Jorgensen, S. J., Liebsch, N., Sala, J. E., Norman, B., Hays, G. C., et al. (2011a). Convergent evolution in locomotory patterns of flying and swimming animals. Nat. Commun. 2, 352. doi: 10.1038/ncomms1350
Gleiss, A., Norman, B., and Wilson, R. (2011b). Moved by that sinking feeling: variable diving geometry underlies movement strategies in whale sharks. Funct. Ecol. 25, 595–607. doi: 10.1111/j.1365-2435.2010.01801.x
Goff, G. P., and Lien, J. (1988). Atlantic leatherback turtles, Dermochelys coriacea, in cold water off Newfoundland and Labrador. Can. Field Nat. Ottawa 102, 1–5.
Goldbogen, J. A., Calambokidis, J., Croll, D. A., Harvey, J. T., Newton, K. M., Oleson, E. M., et al. (2008). Foraging behavior of humpback whales: kinematic and respiratory patterns suggest a high cost for a lunge. J. Exp. Biol. 211, 3712–3719. doi: 10.1242/jeb.023366
Goldbogen, J. A., Calambokidis, J., Shadwick, R. E., Oleson, E. M., McDonald, M. A., and Hildebrand, J. A. (2006). Kinematics of foraging dives and lunge-feeding in fin whales. J. Exp. Biol. 209, 1231–1244. doi: 10.1242/jeb.02135
Goldbogen, J., Pyenson, N., and Shadwick, R. (2007). Big gulps require high drag for fin whale lunge feeding. Mar. Ecol. Prog. Ser. 349, 289–301. doi: 10.3354/meps07066
Hays, G. (2003). A review of the adaptive significance and ecosystem consequences of zooplankton diel vertical migrations. Hydrobiologia 503, 163–170. doi: 10.1023/B:HYDR.0000008476.23617.b0
Hays, G. C., Hobson, V. J., Metcalfe, J. D., Righton, D., and Sims, D. W. (2006). Flexible foraging movements of leatherback turtles across the North Atlantic Ocean. Ecology 87, 2647–2656. doi: 10.1890/0012-9658(2006)87[2647:FFMOLT]2.0.CO;2
Holland, K., Brill, R., Chang, R., and Sibert, J. (1992). Physiological and behavioural thermoregulation in bigeye tuna (Thunnus obesus). Nature 354, 410–412. doi: 10.1038/358410a0
Houghton, J. D., Doyle, T. K., Davenport, J., Wilson, R. P., and Hays, G. C. (2008). The role of infrequent and extraordinary deep dives in leatherback turtles (Dermochelys coriacea). J. Exp. Biol. 211, 2566–2575. doi: 10.1242/jeb.020065
Hunt, G. Jr. (2006). “Evidence for bottom-up control of upper trophic level marine populations,” in Whales, Whaling, and Ocean Ecosystems, eds J. Estes, D. DeMaster, D. Doak, T. Williams and R. Jr. Brownell (Berkeley, CA: University of California Press), 50–64.
Klimley, A., Beavers, S., Curtis, T., and Jorgensen, S. (2002). Movements and swimming behavior of three species of sharks in La Jolla Canyon, California. Environ. Biol. Fish. 63, 117–135. doi: 10.1023/A:1014200301213
Nakamura, I., Goto, Y., and Sato, K. (2015). Ocean sunfish rewarm at the surface after deep excursions to forage for siphonophores. J. Anim. Ecol. 84, 590–603. doi: 10.1111/1365-2656.12346
Nakaya, K., Matsumoto, R., and Suda, K. (2008). Feeding strategy of the megamouth shark Megachasma pelagios (Lamniformes: Megachasmidae). J. Fish Biol. 73, 17–34. doi: 10.1111/j.1095-8649.2008.01880.x
Paladino, F. V., O'Connor, M. P., and Spotila, J. R. (1990). Metabolism of leatherback turtles, gigantothermy, and thermoregulation of dinosaurs. Nature 344, 858–860. doi: 10.1038/344858a0
Parker, H. W., and Boeseman, M. (1954). The basking shark, Cetorhinus maximus, in winter Proc. Zool. Soc. Lond. 1954, 185–194. doi: 10.1111/j.1096-3642.1954.tb01487.x
Sander, P. M., and Clauss, M. (2008). Sauropod gigantism. Science 322, 200–201. doi: 10.1126/science.1160904
Simon, M., Johnson, M., Tyack, P., and Madsen, P. (2009). Behaviour and kinematics of continuous ram filtration in bowhead whales (Balaena mysticetus). Proc. Biol. Sci. 276, 3819–3828. doi: 10.1098/rspb.2009.1135
Sims, D. (1999). Threshold foraging behaviour of basking sharks on zooplankton: life on an energetic knife-edge? Proc. Biol. Sci. 266, 1437–1443. doi: 10.1098/rspb.1999.0798
Takahashi, M., Okamura, H., Yokawa, K., and Okazaki, M. (2003). Swimming behaviour and migration of a swordfish recorded by an archival tag. Mar. Freshwat. Res. 54, 527–534. doi: 10.1071/MF01245
Taylor, L., Compagno, L., and Struhsaker, P. (1983). Megamouth—a new species, genus and family of lamnoid shark (Megachasma pelagios), Family Megachasmidae from the Hawaiian Islands. Proc. Calif. Acad. Sci. 43, 87–110.
Thums, M., Meekan, M. G., Stevens, J., Wilson, S., and Polovina, J. (2013). Evidence for behavioural thermoregulation by the world's largest fish. J. Roy. Soc. Interface 10:20120477. doi: 10.1098/rsif.2012.0477
Weihs, D. (1975). An optimal swimming speed of fish based on feeding efficiency. Isr. J. Technol. 13, 163–167.
Williams, T. M., Davis, R. W., Fuiman, L. A., Francis, J., Le, B. J., Horning, M., et al. (2000). Sink or swim: strategies for cost-efficient diving by marine mammals. Science 288, 133–136. doi: 10.1126/science.288.5463.133
Keywords: gigantothermy, whale shark, exothermy, diving, body plan, thermal inertia
Citation: Meekan MG, Fuiman LA, Davis R, Berger Y and Thums M (2015) Swimming strategy and body plan of the world's largest fish: implications for foraging efficiency and thermoregulation. Front. Mar. Sci. 2:64. doi: 10.3389/fmars.2015.00064
Received: 18 March 2015; Accepted: 21 August 2015;
Published: 15 September 2015.
Edited by:
Graeme Clive Hays, Deakin University, AustraliaReviewed by:
Gail Schofield, Deakin University, AustraliaNuno Queiroz, University of Porto, Portugal
Michael Brian Bennett, The University of Queensland, Australia
Copyright © 2015 Meekan, Fuiman, Davis, Berger and Thums. This is an open-access article distributed under the terms of the Creative Commons Attribution License (CC BY). The use, distribution or reproduction in other forums is permitted, provided the original author(s) or licensor are credited and that the original publication in this journal is cited, in accordance with accepted academic practice. No use, distribution or reproduction is permitted which does not comply with these terms.
*Correspondence: Mark G. Meekan, The Australian Institute of Marine Science, c/o UWA Oceans Institute (MO96), 35 Stirling Hwy, Crawley, WA 6009, Australia,bS5tZWVrYW5AYWltcy5nb3YuYXU=