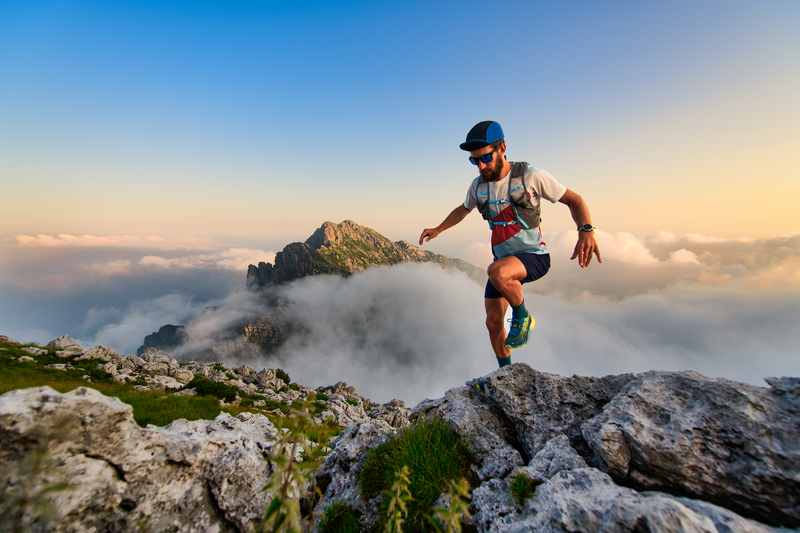
94% of researchers rate our articles as excellent or good
Learn more about the work of our research integrity team to safeguard the quality of each article we publish.
Find out more
ORIGINAL RESEARCH article
Front. Mar. Sci. , 29 September 2014
Sec. Global Change and the Future Ocean
Volume 1 - 2014 | https://doi.org/10.3389/fmars.2014.00045
The metabolic carbon requirements and excretion rates of three major zooplankton groups in the Southern Ocean were studied in February 2009. The research was conducted in the framework of the ATOS research project as part of the Spanish contribution to the International Polar Year. The objective was to ascertain the possible consequences of the predicted zooplankton shift from krill to salps in the Southern Ocean for the cycling of biogenic carbon and the concentration and stoichiometry of dissolved inorganic nutrients. The carbon respiratory demands and NH4-N and PO4-P excretion rates of <5 mm size copepods, krill and salps were estimated by incubation experiments. The carbon-specific metabolic rates and N:P metabolic quotients of salps were higher than those of krill (furcilia spp. and adults) and copepods, and as expected there was a significant negative relation between average individual zooplankton biomass and their metabolic rates, each metabolic process showing a particular response that lead to different metabolic N:P ratios. The predicted change from krill to salps in the Southern Ocean would encompass not only the substitution of a pivotal group for Antarctic food webs (krill) by one with an indifferent trophic role (salps). In a zooplankton community dominated by salps the respiratory carbon demand by zooplankton will significantly increase, and therefore the proportion of primary production that should be allocated to compensate for the global respiratory C-losses of zooplankton. At the same time, the higher production by salps of larger, faster sinking fecal pellets will increase the sequestration rate of biogenic carbon. Similarly, the higher N and P excretion rates of zooplankton and the changes in the N:P stoichiometry of the metabolic products will modify the concentration and proportion of N and P in the nutrient pool, inducing quantitative and qualitative changes on primary producers that will translate to the whole Southern Ocean ecosystem.
The consequences of human-induced global perturbations in polar areas are predicted to include significant changes in structure and function of marine ecosystems (Smetacek and Nicol, 2005; Duarte, 2008; Wassmann et al., 2008), and although their specific nature is difficult to foretell, it is most likely that smooth environmental changes could result in non-linear and probably irreversible ecosystem shifts (Duarte et al., 2012).
Zooplankton play a fundamental role in the transfer and cycling of biogenic carbon in marine systems, controlling not only the fraction of primary production available to upper consumers, but the magnitude and fate of vertical carbon flux (either recycled or sequestered). Zooplankton can also modify the chemical environment of phytoplankton by increasing the “per cell” quota of nutrients (i.e., reduction of cell concentration by grazing), and change the N:P ratio of dissolved nutrients by excreting N and P at different rates (Sterner, 1986, 1990). In this sense, the inverse relation between the N:P quotient of the metabolic products of Arctic zooplankton and temperature has been suggested as one of the tipping elements that could induce non-linear changes in the Arctic marine ecosystems by global warming (Alcaraz et al., 2013).
In the Southern Ocean the major mesozooplankton groups are copepods, krill, and salps. Krill constitute an essential node, directly transferring matter and energy from micro auto- and heterotrophs to upper consumers including birds, fish, seals and whales (Atkinson et al., 2004; Smetacek and Nicol, 2005), and recently being also the target of commercial fisheries (Omori, 1978; Constable et al., 2000; Atkinson et al., 2004). From the biogeochemical point of view, krill contributes decisively to the vertical flux of biogenic carbon (Pakhomov et al., 2002; Pakhomov, 2004; Tanimura et al., 2008; Ruiz-Halpern et al., 2011) and is an important source of recycled dissolved organic carbon and iron (Tovar-Sanchez et al., 2007).
Although the role of salps in the Antarctic food webs and biogeochemical cycles is less known, as a source of food for upper trophic levels seem to be of minor importance (but see Dubischar et al., 2012). However, their contribution to the vertical flux of biogenic carbon is higher than that of krill (Pakhomov et al., 2002; Pakhomov, 2004; Tanimura et al., 2008), with higher ingestion rates and the egestion of larger, faster sinking fecal pellets (Pakhomov et al., 2006; Ducklow et al., 2012).
Regarding the smaller size fractions of mesozooplankton (Copepods and furcilia) their role in Antarctic food webs is complex. Although their food include micro auto- and heterotrophs, copepods and furcilia show a clear preference for heterotrophic preys (Wickham and Berninger, 2007) and their contribution to the vertical flux is lower than that of salps or krill, as a large proportion of their fecal material is degraded while sinking (Dagg et al., 2003). However, their specific rates of carbon demand and nutrient cycling can be higher than those of krill and salps (Ikeda and Mitchell, 1982; Alcaraz et al., 1998).
During the last decades the Southern Ocean appears to be experiencing crucial structural and functional changes (Constable et al., 2014) that affect particularly the two main planktonic grazers, krill, and salps. All analyzed data on their relative abundance suggest, aside from a strong inter-annual variability, a sustained decreasing trend of krill (from 38 to 75% per decade, Atkinson et al., 2004) and their substitution by salps (Smetacek and Nicol, 2005; Murphy et al., 2007). In the zone west of the Antarctic Peninsula, for the decade 1993–2004 aside from the alternation of “salp years” (1994, 1997, 1999) with positive anomalies of krill biomass (1996, 1998), a constant decreasing tendency to negative biomass anomalies for krill, in opposition to positive anomalies for salps, has been also recorded (Ross et al., 2008). The reasons of this community shifts are not clear, but the changes of krill distribution appear to be related to chlorophyll concentration (Atkinson et al., 2004; Montes-Hugo et al., 2009) and their inter-annual variability to the changes in the extent of winter sea ice (Atkinson et al., 2004; Murphy et al., 2007). The decimation of baleen whales could also explain the present zooplankton shift by changes in the recycling characteristics of iron and nutrients in surface waters (Smetacek, 2008) that would have affected the structure and function of primary producers and of the whole Antarctic food web.
In order to ascertain the consequences of zooplankton shifts for the biogeochemical cycles in the Southern Ocean, we have analyzed the effects of community structure on the metabolic demand of biogenic carbon and on the stoichiometry of the recycled inorganic nutrients. The main objectives were (1) To determine how the predicted shift will affect the global respiratory carbon loss by zooplankton, the proportion of primary production required to compensate for it, and the carbon vertical flux, and (2) The changes in the contribution of zooplankton excretion to the N and P required by phytoplankton, and the N:P proportion of the excreted products. These are basic questions to answer in a future scenario where krill-salps fluctuations will be more frequent and salps are predicted to substitute krill.
The study was made in the framework of the ATOS research project in January-February 2009 on board the R/V “Hespérides” during the ATOS Antarctic cruise (ATOS-II), as part of the Spanish contribution to the International Polar Year. In a network of stations located in the vicinity of the Antarctic Peninsula (Figure 1), the abundance, community composition, and individual biomass of zooplankton was analyzed on samples obtained with a double WP-2 net hauled vertically between 200 m depth (or less in shallower stations) and surface. The volume of water filtered was measured with a back-stop General Oceanics Flow-Meter® placed at the mouth of the net at a distance from the holding ring equivalent to 1/3 of its diameter. The samples corresponding to the two nets were mixed and homogenized in a container, concentrated and fixed in 4% formalin in seawater (final concentration) for abundance, taxonomic and biomass studies.
Figure 1. Map of the area sampled during the ATOS-Antarctic cruise with the position of the studied stations.
Crustacean zooplankton abundance and biomass as carbon (“in situ” and in the experimental bottles) was estimated according to the biovolume (BV)—zooplankton carbon (Czoo) factor (Alcaraz et al., 2003). The number of organisms and biovolume (BV) determinations were made with the free-user program for image analysis ZooImage® (http://www.sciviews.org/zooimage) on scanned images of preserved organisms made with an EPSOM 4990 Photo scanner at 2400 dpi. Organisms were previously stained in a 0.05% eosin-Y aquatic solution for 24 h. The BV-Czoo factor conversion used was that given by Alcaraz et al. (2003, 2010, 2014) for Arctic zooplankton: 1 mm3 BV = 0.08 mg Czoo. In the case of S. thompsoni blastozoids, the individual C contents (CS) was calculated by two methods: according to the relationships between atrial-oral length and CS given by Huntley et al. (1989), and by the relation between the nucleus volume (NV) and CS (Alcaraz et al., 2003). The correlation coefficient between the CS obtained by the two methods was r = 0.99. Krill biomass was measured with a Simrad® EK60 multifrequency echosounder, and the data taken from Ruiz-Halpern et al. (2011), where more details can be obtained.
The taxonomic composition of zooplankton was analyzed automatically on the scanned samples using appropriate shape identification algorithms and specific training sets (Fernandes et al., 2009; Saiz et al., 2013) for 10 main taxons or categories of Antarctic zooplankton chosen after the study of selected samples: Two groups of adult copepods, Calanoids and Oithona; nauplius; adult and juvenile euphausiids (furcilia); polychaets, chaetognaths, salps, foraminifers and a group of unidentified organisms. The percentage error of automatic classification as compared to manual classification under stereomicroscope in paired samples ranged from 0 (chaetognaths) to less than 6% for nauplii and copepods.
Chlorophyll a (Chl a) concentration was determined in the studied stations by filtering 50 mL samples onto 25-mm diameter GF/F filters from the depths where primary production was measured. Chlorophyll extracted by acetone was measured by fluorescence according to Parsons et al. (1984), and Chl a transformed into phytoplankton carbon units using a C:Chl a ratio of 100 (mg–mg) following Hewes et al. (1990) for relatively poor Antarctic waters.
In situ primary production was measured by the 14C technique (Steemann-Nielsen, 1952) as described in Morán et al. (2001). Water sampled at 3 depths including the surface (1 m), the subsurface (5 m) and the deep chlorophyll maximum (DCM) was transferred into transparent (light) and dark 150 ml polycarbonate bottles, and inoculated with 100 μCi activity of a 14C working solution. Inoculated bottles were suspended at the corresponding depths from a drifting buoy and incubated in situ for 4 h at the same time of the day (from 12.00 to 4.00 p.m.), always including noon. At the end of the incubation period duplicated 5 ml aliquots were transferred into 20 ml scintillation vials for the determination of total labeled organic carbon production (TPP). The remaining volume was filtered through 0.22 μm mesh membrane filters (cellulose membrane filters) of 25 mm diameter to determine particulate primary production (PPP > 0.22 μm). Samples were acidified with 100 μl of 10% HCl and shaken for 12 h to remove inorganic 14C. Then, 10 ml of scintillation cocktail (Packard Ultima Gold XR) were added to TPP vials and the disintegrations per minute were counted after 24 h with a scintillation counter (EG&G/Wallace).
As we had no data on irradiance we integrated the solar curve along the daylight hours corresponding to the latitude and date of the study, considered as proportional to the theoretical irradiance without cloud covering. We calculated also the maximum theoretical irradiance, equivalent to the integral of the maximum (noon) irradiance along the duration of the day. The proportion of the maximum total theoretical irradiance that corresponded to theoretical irradiance, multiplied by the duration of the day gave us the factor f to transform hourly primary production rates into daily rates,
where f is the factor to multiply hourly primary production rates to obtain daily rates, t and t' the hour of sunrise and sunset during the study, TI the solar curve equivalent to the theoretical irradiance, and MTI the maximum theoretical irradiance, equivalent to the irradiance (height of the solar curve) at noon.
Metabolism (respiration and excretion of ammonia and phosphate) was estimated by incubation experiments on copepods, krill juveniles (unidentified furcilia) and adults (Euphausia superba and E. crystallorophias), and salps (blastozoids of Salpa thompsoni), the most significant groups of Antarctic zooplankton. Experimental copepods, furcilia and salp blastozoids were obtained by vertical WP-2 net tows made at a speed of 10 m min−1 from 100 m depth to surface, conducted with the same net as for the study of the zooplankton community structure but fitted with a 6-L plastic bag as cod end to avoid damaging the organisms. Adult krill were caught with short (<3 min) horizontal or oblique trawls using an IKMT net provided with a 20 L rigid PVC cod end and a Scanmar® HC4-D net sounder to control the depth of the trawl. When a krill swarm was located with the Simrad® EK60 echosounder, the ship re-traced the course and the haul was made across the previously observed depth and position of the krill swarm.
WP-2 samples were immediately transferred into thermally isolated 10 L containers filled with “in situ” water and transported to the laboratory. Salps and furcilia were separated by gently screening the sample using a 5 mm plastic grid submerged in a 2 L jar containing 0.2 μm-filtered seawater at “in situ” temperature, and individually sorted and transferred with a plastic spoon into separated 2 L Pyrex® bottles containing 0.2 μm-filtered seawater. The <5 mm size-fraction copepods were repeatedly cleaned, screened and concentrated using a 200 μm netting submerged in filtered seawater in order to discard phyto- and microzooplankton. Adult krill were gently transferred from the IKMT cod-end into 50 L on-deck containers provided with circulating surface water, individually sorted with a hand net provided with a 200 mL plastic bucket as cod-end and kept on separate 10 L thermally isolated containers at in situ temperature.
Incubation experiments for simultaneous estimation of respiration and excretion rates (Table 1) were made in Pyrex® bottles from 250 mL to 5 L volume, depending on the biomass of experimental organisms. The bottles were closed by silicone stoppers holding the O2 probes and a syringe needle to compensate for pressure changes as described in Alcaraz et al. (1998, 2010, 2013, 2014) and sketched in Almeda et al. (2011). Experimental organisms (either an aliquot of the <5 mm copepods, or from 2 to 4 individuals in the case of larger organisms) were transferred in less than 1 h after capture to experimental bottles filled with in situ seawater obtained with 12 L Niskin bottles from 20 to 40 m depth, depending of the depth of the maximum chlorophyll, filtered by gravity through 0.2 μm Acro-Pack® filters and O2-saturated. Control bottles contained only filtered seawater. Once confirmed that there were only intact organisms in the experimental bottles (i.e., all the organisms showing normal swimming behavior), experimental and control bottles were stopped without trapping air bubbles and incubated for 12–24 h in thermostatic baths at the 0–200 m depth “in situ” integrated average temperature ±0.1°C and dim light.
Table 1. Taxonomic composition (ind m−3) and biomass (μmol C m−3, bold italics) for the main zooplankton groups in the studied stations (see Methods).
Zooplankton respiration was estimated as the decreasing rate of dissolved oxygen concentration during the incubation. The analyses were made with an OXY-10 Pre-Sens® oxygen sensor (optodes, Alcaraz et al., 2010) that allowed semi-continuous (every 5 min.) measurements of O2 concentration using 6–8 O2 probes for experimental bottles, and 2–4 for control ones. Respiration rates were estimated as the difference between the slopes of the linear regression equations describing the changes in O2 concentration during the incubations in experimental and control bottles (Alcaraz et al., 2010, 2013). Oxygen consumption was transformed into respiratory C losses using a respiratory quotient (RQ, the molar ratio of CO2 produced to O2 consumed) of 0.97 (Omori and Ikeda, 1984).
Excretion rates were estimated in the same incubation experiments as for respiration. Ammonia and phosphate excretion rates were calculated as the difference in the final concentrations in experimental and control bottles. At the end of the incubation water samples were siphoned from the bottles using silicone tubes ending in broad plastic tips enclosed with 100 μm-mesh in order to avoid extracting zooplankton organisms with the water sample. Ammonia was analyzed by the fluorimetric method described by Kéruel and Aminot (1997), and phosphate according to Grasshoff et al. (1999). At the end of the incubations, experimental zooplankton was transferred to vials and fixed in 4% formalin (final concentration) for further measurement of experimental biomass as zooplankton carbon.
Metabolic rates were normalized to per unit of zooplankton carbon biomass (C- specific metabolic CR, NE, and PE) by dividing daily gross respiration and excretion rates (μmol C, μmol N and μmol P day−1) by the corresponding experimental biomass in μmol C. Specific metabolic data from other authors when expressed in different units have been re-calculated using the wet mass, dry mass, and organic C transform factors given in Harris et al. (2000). The taxonomic composition and individual biomass of experimental organisms were analyzed as described above. The metabolic CR:NE, CR:PE, and NE:PE quotients were calculated as the ratios between the specific corresponding metabolic rates in each individual experiment and expressed in atoms.
The daily global average respiratory carbon losses and N and P supplied by zooplankton were calculated by the addition of the average respiratory losses and ammonia and phosphate excreted by the different zooplankton groups. These were calculated as the product of the average in situ C biomass of each group by their corresponding C-specific metabolic rates,
where CL, NS, and PS are the daily respiratory C loss and N and P excreted by the group, CR NE and PE the corresponding C-specific metabolic rates, and CZOO the average in situ biomass of the corresponding zooplankton group as carbon.
The total theoretical daily carbon ingested by zooplankton and vertical carbon flux in μmol C m−3 day−1 were calculated respectively by the addition of the carbon ingested and egested by the different groups. The daily carbon respiratory losses of each group were considered as equivalent to the carbon assimilated. Therefore, the carbon daily ingested and egested can be estimated from the carbon respiratory losses and the assimilation efficiencies of the different groups (0.7 and 0.52 for krill and salps respectively, Pond et al., 1995; Pakhomov et al., 2006) as follows,
where CI is the global carbon ingestion; CLG are the respiratory C losses for the different groups, and AEG are the corresponding assimilation efficiencies.
The theoretical carbon egested was considered as equivalent to the non-assimilated C and equivalent to the vertical carbon flux as fecal pellets. It was calculated by the addition of the daily fecal pellets production (carbon egested) by salps and krill. The carbon egested by copepods and other small zooplankters was not included in the estimations of carbon export as their fecal pellets are mainly recycled in surface waters and therefore their contribution to the vertical C transfer is negligible, and their carbon egestion in the present conditions and for the predicted salps-krill shift would not change,
where CEX is the global carbon egested as fecal pellets, and CLG and AEG as described above.
The fraction of total and particulate primary production (TPP and PPP) daily ingested by zooplankton to compensate for their C metabolic losses and vertically exported has been expressed as a percentage,
To estimate the consequences of the zooplankton shift for the carbon and nutrient flux we have assumed a change in the proportion of krill and salps biomass from the present situation (a krill-based zooplankton community) to that of a “salp year” (average salps/krill ratio = 10, Huntley et al., 1989; Loeb et al., 1997, 2010; Alcaraz et al., 1998). In terms of biomass the substitution falls in the known range of a “salps year,” between 2900 and 6200 μmol C m−3 (Alcaraz et al., 1998; Tanimura et al., 2008). Average biomass and numbers and the corresponding standard deviations (krill excepted) for the different zooplankton groups were calculated globally for the whole stations sampled. The relationships between individual biomass and C-specific metabolic rates or metabolic quotients have been estimated by linear regression on log-transformed data. All the statistical analysis have been made using JMP® 7.0 software.
The most abundant and frequent zooplankton group in the study area were copepods. Calanoids, Oithona sp., and nauplii contributed to 97.7% of zooplankton as numbers. Foraminifera were scarce but present in most of the stations, followed by chaethognaths and polychaets. Furcilia and salps (Salpa thompsoni) were observed only in Stations 8 and 26 respectively (Table 1). Copepods occurred in all the stations, and reached concentrations up to 430 individuals m−3 (Station 8, Table 1). We had no data on krill numbers as we estimated krill biomass by acoustic methods, and the nets used to capture experimental animals (WP-2 and IKMT) are not adequate to sample krill.
In terms of biomass (as μmol C m−3) krill dominated the zooplankton community (91.9%), followed by copepods and furcilia sp. (7.2 and 0.35% of total biomass respectively, Table 1). The remaining groups had variable importance, salps contributing to less than 0.2% of total zooplankton C. The average zooplankton biomass (>200 μm-size), including krill, accounted for 5109 μmol C m−3, or 12.26 g C m−2 (0–200 m depth).
The best fit of the time changes of O2 concentration in control and experimental bottles was the negative linear regression, the average determination coefficient being r2 = 0.83. As no short-term decreases in the rate of O2 consumption were observed indicating a linear trend in the respiration rates, we assumed a similar linear response for ammonia and phosphate excretion. Average respiratory losses (CR) of copepods and furcilia sp. were similar, 0.0348 and 0.0330 d−1 respectively. The respiration rates of salps (S. thompsoni) were higher by a factor of 2.5 than for the crustacean zooplankton groups (0.0841 d−1), while the lowest CR corresponded to adult krill (Euphausia superba, 0.0102 d−1). Carbon-specific ammonia (NE) and phosphate (PE) excretion rates were also lower for crustaceans than for salps (Table 2). The lowest excretion rates corresponded also to Adult E. superba (NE = 0.0004, std. 0.0002 μmol NH4-N μmol C−1ZOO d−1, and PE = 0.0003, std. 0.0003 μmol PO4-P μmol C−1ZOO d−1). In the case of salps NE = 0.0073, std. 0.0006 μmol NH4-N μmol C−1ZOO d−1, and PE = 0.0017, std. 0.0004 μmol PO4-P μmol C−1ZOO d−1.
Table 2. Average values and standard deviation (in italics between brackets) of biomass (μmol C m−3), individual biomass range (μm C ind−1), C- specific metabolic rates (CR, carbon respiration, d−1; NE, ammonia excretion, μmol NH4-N μmol C−1 d−1; PE, μmol PO4-P μmol C−1 d−1), and C:N, C:P and N:P metabolic ratios by atoms for the studied zooplankton groups.
The atomic CR:NE quotients for the different groups ranged from 11.5 (salps, E. thompsoni) to 28.4 (furcilia sp.), in both cases higher by a factor from 2 to 5 than the expected Redfield ratio, and also higher than the average values from previously recorded data (Table 2), while the CR:NE atomic metabolic ratios for krill were similar to the average literature values (Table 2). CR:PE quotients ranged from 43.2 to 103.4 (furcilia sp. and copepods respectively) and fell within the values given in the scarce previous data (Table 2). Regarding the NE:PE atomic quotients, again the values were lower than the expected Redfield ratios. The lowest values corresponded to krill, with an average NE:PE value for the whole group of 2.4, followed by salps, NE:PE = 4.6. The highest N:P quotient, 8.1, corresponded to copepods (Table 2).
The individual biomass of the experimental groups (Table 2 and Figure 2) spanned six orders of magnitude, from copepods (0.16–3.46 μmol C ind−1) to adult E. superba (6833.1–59,676 μmol C ind−1), with intermediate values for developmental stages of krill (furcilia sp., 2.56–6.49 μmol C ind−1), E. cristallorophias (176.53–356.66 μmol C ind−1) an salps, S. thompsoni (156.0–193.0 μmol C ind−1). There was a significant, negative relationship between the specific metabolic rates and individual biomass when the whole range of individual biomass data was considered (Figure 2). The relationships between individual biomass and respiration, ammonia and phosphate excretion rates as described by the exponents of the equations (salps excluded) were CR = −0.199, NE = −0.238 and PE = −0.177 (Table 3A). When considering individually each group, the exponents were still negative, but were only significant for groups with large data sets and/or a broad span in individual biomass, like copepods and krill (data not shown). As expected by their high average specific metabolic rates, salps occupy an outsider position in the graph (Figure 2). Regarding the effects of individual biomass on metabolic stoichiometry, CR:NE was not related to individual biomass, while CR:PE and NE:PE metabolic quotients were inversely and significantly related to individual biomass (Figure 3 and Table 3B).
Figure 2. Relationships between individual biomass of experimental zooplankton (μmol C ind−1) and C-specific metabolic rates. Black dots: Respiration (d−1); open squares: μmol NH4-N μmol C−1zoo day−1; Black triangles: μmol PO4-P μmol C−1zoo day−1. The values for salps are indicated by larger symbols and enclosed in a shaded circle. The corresponding equations are indicated in Table 3A.
Table 3. Equations relating individual zooplankton biomass (Czoo) with (A): C-specific metabolic respiration (CR), and ammonia and phosphate excretion rates, NE and PE respectively, and (B): with CR:NE, CR:PE and NE:PE metabolic quotients (atoms) corresponding to Figures 2, 3.
Figure 3. Relationships between individual biomass of experimental zooplankton (μmol C ind−1) and metabolic quotients in atoms. Black dots: CR:NE; Open squares: CR:PE; black triangles: NE: PE. The corresponding equations are indicated in Table 3B.
The average chlorophyll concentration was 0.985 μg L−1 ± 0.237 SE, equivalent to 8211.6 μmol C m−3 ± 1978.3 SE (Ruiz-Halpern et al., 2011). The depth-integrated (0–50 m) total primary production (TPP) ranged from 24.1 mg C m−3 h−1 to 363.3 μg C m−3 h−1, and particulate primary production (PPP) from 13.3 to 207.5 μmol C m−3 h−1 at St. 16 and 2 respectively (data not shown). The average TPP and PPP (according to Equation 1) were 1624.7 and 758.9 μmol C m−3 day−1. We had no data on the assimilation rate of N and P by phytoplankton, therefore we assumed it to agree with a 106:16:1 C:N:P atomic proportion (Redfield et al., 1963), the N and P theoretically required by phytoplankton for TPP thus being 245.1 μmol N m−3 day−1, and 15.5 μmol P m−3 day−1.
The carbon theoretically ingested by zooplankton to compensate for their daily average C respiratory losses, once corrected for the assimilation efficiency, averaged 110.9 μmol C m−3 day−1(Table 4), about 1.3% of the phytoplankton biomass (as carbon), and about 6.8 and 14.6% of the daily total primary production (TPP) and particulate primary production (PPP) respectively (Table 5). The N and P excreted as ammonia and phosphate for the zooplankton community, 4.88 and 2.41 μmol N and P m−3 day−1 respectively (Table 4), were equivalent to 2 and 14.7% of the N and P required by phytoplankton for total primary production (TPP, Table 5). Regarding the vertical flux, the carbon exported accounted for 33.3 μmol C m−3 day−1, 2 and 4.4% of TPP and PPP respectively (Table 5).
Table 4. Zooplankton biomass (μmol C m−3), average metabolic carbon requirements (theoretical carbon ingestion, CI), ammonia (NEX) and phosphate (PEX) excreted (μmol m−3 day−1) for Present and Salp-Krill shift conditions.
Table 5. Allocation and fate of biogenic C, N, and P during our study (Present) and in the case of the predicted Salp-Krill shift.
In the case of the predicted substitution of krill by salps, and assuming primary production rates equivalent to those found during our study, the carbon requirements by zooplankton would average 772.8 μmol C m−3 day−1 (Table 4), equivalent to about 10% of the phytoplankton standing stock, and about 47 and 100% of TPP and PPP respectively (Table 5). The ammonia and phosphate excreted will be 35.82 and 7.96 μmol N and P m−3 day−1 respectively (Table 4), or 14.6 and 52% of the N and P required by phytoplankton for TPP (Table 5). The new vertical carbon flux would increase by a factor of ten, equivalent to around 23 and 49% of TPP and PPP respectively (Table 5). The average N:P atomic quotient of the excreted products by the whole zooplankton community would increase from 2.0 in the present conditions to 4.5 for the predicted shift (Table 6).
Table 6. Average CR:NE, CR:PE and NE:PE metabolic quotients (atoms) for the whole zooplankton community.
The zooplankton community during the ATOS-II cruise was dominated by krill, accounting for more than 90% of total zooplankton biomass. The situation therefore corresponded to a non-“salp year” (in the sense of Huntley et al., 1989 and Alcaraz et al., 1998), in which salps (usually E. thompsoni and Ihlea rakovitzai) are the dominant group at least in terms of biomass (Alcaraz et al., 1998; Le Fèvre et al., 1998; Perissinotto and Pakhomov, 1998), displace krill as the main grazer, and can reach more than 90% of total zooplankton biomass (Loeb et al., 1997, 2010; Alcaraz et al., 1998; Atkinson et al., 2004).
The average zooplankton biomass ranged between the 2500 μmol C m−3 found by Ward et al. (1995) and the more than 12,500 μmol C m3 given by Pauly et al. (2000) and by Tanimura et al. (2008) in summer 2002–2003. Part of the differences in the zooplankton community with previous data should be attributed to the strong inter-annual variability coupled with the patchy nature of distribution and abundance that characterize zooplankton in general, and especially Southern Ocean krill and salps (Nishikawa et al., 1995; Loeb et al., 1997; Atkinson et al., 2004; Smetacek and Nicol, 2005). The zooplankton abundance and biomass during summer, strongly dependent from the ice conditions, the position of the circumpolar current, and primary production (Atkinson et al., 2004; Ward et al., 2004; Ross et al., 2008; Montes-Hugo et al., 2009) show strong spatial and temporal changes, the alternative dominance of salps and krill being of different duration but occurring at 3–4 years interval since 1993 (Ross et al., 2008). The progressive tendency to the reduction of krill abundance (Atkinson et al., 2004; Murphy et al., 2007), and differences in the methods of biomass estimation (i.e., nets or echosounders) can also explain the between years differences observed in total zooplankton biomass. Especially in the case of krill, biomass estimations made with different sampling gears are hardly comparable. While echosounders seem to be quite reliable (Ruiz-Halpern et al., 2011), nets clearly induce avoidance reactions in krill that lead to large underestimations (Sameoto et al., 2000).
The metabolic rates of zooplankton during our study fell within the range of previous works. The average values for copepods were similar to those given by Ikeda and Mitchell (1982) and Alcaraz et al. (1998), especially regarding ammonia and phosphate excretion (NE and PE). In the case of adult krill (E. superba and E. crystallorophias) our average metabolic rates were similar to those found by Hirche (1983), Auerswald et al. (2009), and Ikeda and Mitchell (1982), but lower than data from Meyer et al. (2009, 2010), Atkinson et al. (2002), Ruiz-Halpern et al. (2011), and Lehette et al. (2012). The metabolic rates of larval krill (furcilia) were very similar to those given by Frazer et al. (2002) and Meyer and Oettl (2005). Regarding salps, the estimated metabolic rates were higher by a factor of two than those given by Ikeda and Bruce (1986), Iguchi and Ikeda (2004), and Alcaraz et al. (1998), but lower than the data given by Ikeda and Mitchell (1982).
About eight decades ago Marshall et al. (1935) observed a decrease of zooplankton respiration during long incubation experiments in filtered seawater. The decrease is attributable to the combination of multiple factors derived from the experimental conditions like starvation, capture and manipulation stress, food quality and composition, animal crowding and container volume, etc. (Mayzaud, 1973; Ikeda, 1976, 1977; Checkley et al., 1992; Harris et al., 2000). Therefore, the short-time rate of decrease of metabolic rates along successive measurements, when these are made as close as possible to the starting of the incubation, should allow to estimate metabolic rates at time = 0, considered to be equivalent to the “in situ” rates. Recently Ruiz-Halpern et al. (2011) and Lehette et al. (2012) obtained by this method biomass- specific ammonia excretion rates for Antarctic krill significantly higher than previous ones. But although the “in situ” rates given by both authors are similar, the rate of decrease given by the exponential model of Ruiz-Halpern et al. (2011) is about twice than that estimated by the potential equation of Lehette et al. (2012). The eventual underestimation of our metabolic rates (12–24 h incubation in filtered seawater) as compared with those of Ruiz-Halpern et al. (2011) would have been 32 and 54%, and from 17 to 40% as compared to Lehette et al. (2012).
In our case the decrease in oxygen concentration in experimental and control flasks displayed a linear trend indicative of a constant respiration rate, a response generally observed in crustaceans for substrate (O2) concentrations above 70% (Alcaraz, 1974). As the factors responsible for the decrease of metabolic rates affect similarly all the metabolic processes (Mayzaud, 1973; Ikeda, 1976, 1977; Checkley et al., 1992; Harris et al., 2000), we assumed a similar linear trend for excretion rates as for respiration, and therefore the data on ammonia and phosphate excretion were not corrected. Another reason for not correcting the metabolic rates of krill for the possible effects of starvation (or any other laboratory conditions that could modify metabolic rates) was the lack of previous data of their effects on the metabolism of salps, therefore precluding the comparison of the metabolic rates of both zooplankton groups.
Ikeda and Mitchell (1982) and more recently Phillips et al. (2009) reported metabolic rates of S. thompsoni as high as those observed here, significantly higher than other groups of similar average individual C content. Large differences in the mass-specific metabolic rates of different zooplankton groups, aside from the effects of individual biomass could be due to the use of inadequate body mass conversion factors when normalizing the units in which the metabolism is expressed (Ikeda and Mitchell, 1982). Schneider (1990) comparing literature data on metabolic rates for crustacean and gelatinous zooplankton found biomass-specific ammonia excretion rates in crustaceans to be about one order of magnitude higher than for gelatinous zooplankton when expressed as per dry mass. However, when using organic C or N as biomass units, the metabolic rates of both groups were equivalent or higher for gelatinous organisms (Schneider, 1990). Differences in the degree of gut fullness (the C gut contents can make from 10 to 60% of their body C, Pakhomov et al., 2006) when salps C-contents is indirectly estimated by inadequate factors relating body carbon with salps dimensions are a complementary source of variability.
The negative relation between individual biomass and C-specific metabolic rates when all the zooplankton groups were included was significant and consistent with the non-similarity theory (Heusner, 1982; Riisgård, 1998). However, salps fell out of range, being clearly outliers. The different relationships between individual biomass (C contents) and C-specific respiration, ammonia and phosphate excretion rates as described by the exponents of the power equations were quite similar to those given by Ikeda and Mitchell (1982) and Ikeda (1985), as were the coefficients of the equations.
In general, the metabolic quotients were similar to previously reported values for most groups except for copepods (Ikeda and Mitchell, 1982). Average CE:NE ratios were higher and CE:PE and NE:PE ratios lower than Redfield's ones by a factor of at least two, as previously reported in previous studies (see Table 2). The deviation of the metabolic ratios from the theoretical Redfield's seems to be general for high latitude zooplankton (Hirche, 1983). Higher than Redfield et al. (1963) CE:NE ratios are indicative of the use of carbohydrates and/or lipids as metabolic substrate, of herbivorous feeding (Conover and Corner, 1968; Mayzaud, 1973), or of underestimating ammonia excretion (Ruiz-Halpern et al., 2011; Lehette et al., 2012). In the case of furcilia, aside from the above mentioned reasons the high CE:NE values would be consequence of low NE rates (Meyer and Oettl, 2005) due to metabolic N retention, characteristic of fast growing larval crustaceans (Elser et al., 1996). Contrarily, the CE:NE quotient lower than 12 of salps could be due to relatively high NE rates, indicating the use of N-rich metabolic substrate (Mayzaud and Conover, 1988) or to the differences in the slopes and intercepts of C-scaled specific respiration and excretion rates, similar to those of jellyfish, as discussed by Pitt et al. (2013). The differences in the relationships between individual biomass and specific respiration and ammonia and phosphate excretion rates explain the relation observed between individual body C and CE:NE, CE:PE and NE:PE metabolic quotients. It is particularly important in the case of the NE:PE ratio, as the variance induced by individual biomass to the quotient can be up to 10% (Ikeda, 1985), that would be added to the effect taxonomic differences. The consequences of changes in the proportion of excreted ammonia and phosphate by zooplankton would be the modification of the N:P stoichiometry of nutrients available for phytoplankton (Elser et al., 1996; Sterner, 1986, 1990).
The phytoplankton C concentration corresponded to what Hewes et al. (1990) qualifies of relatively low Chl a concentration waters. Total primary production (TPP) was in the range of previous estimations for the same area in late summer (from 1163 μmol C m−3 day−1, Figueiras et al., 2001, to 2500 μmol C m−3 day−1, Morán et al., 2001), but higher than the values observed by Basterretxea and Aristegui (1999) during late spring (558–930 μmol C m−3). These differences, aside from the intrinsic inter annual variability, could be due to changes in the depth range considered in the estimates of primary production. Likely by the same logic the ratio particulate primary production/total primary production (PPP/TPP) was lower than the values given by Morán et al. (2001) corresponding to offshore waters of the same area.
During our cruise zooplankton required a very low percentage of both the phytoplankton standing stock and the particulate carbon produced by phytoplankton (PPP). Similar low impacts on phytoplankton standing stock and primary production in the Southern Ocean by krill and salps grazing have been reported by Tanimura et al. (2008) with grazing impacts ranging from 0.1 to 1%, exceptionally up to 6% of phytoplankton C. During our study most of the phytoplankton C was required for crustaceans (copepods plus adult krill and furcilia), which needed 98% of the carbon necessary to balance the global respiratory C losses of total zooplankton, while salps required less than 1%. However, there was a radical difference when considering the C requirements of the zooplankton groups during our study as compared to the 1994 “salp year,” when crustaceans required only 14% of the carbon allocable to total zooplankton respiratory losses and the remaining 86% corresponded to salps (Alcaraz et al., 1998; Perissinotto and Pakhomov, 1998).
The average supply of ammonia by zooplankton to the N required by phytoplankton for TPP was lower than previous data for a similar area and season of the year (Alcaraz et al., 1998). By groups, krill contributed to more than 70% of the total ammonia excreted, while salps provided only 1.2%. This contrasts with the conditions found during 1994 (a “salp year”), when zooplankton excretion provided up to 7.3% of the N and P required by phytoplankton (Alcaraz et al., 1998), salps alone accounting for 96% of the nutrients excreted. During our study, the total phosphate excreted provided almost 10% of the phytoplankton requirements, again with krill as the main contributors and salps providing less than 0.5% of the P required for TPP. Both the N and P supplied could be roughly 43% higher if theoretical “in situ” metabolic rates had been estimated according to the methods of Ruiz-Halpern et al. (2011) and Lehette et al. (2012), but as discussed above the linear trend in O2 consumption suggested similarly constant excretion rates, and therefore the theoretical “in situ” rates were not calculated.
The fraction of PPP required to compensate for the respiratory losses of zooplankton, aside from being an estimator of the relative importance of classical, herbivorous food webs in marine ecosystems (Calbet et al., 1996) is also related to the trophic efficiency of the system (Alcaraz, 1988; Alcaraz et al., 1994) and equivalent to the reciprocal of the quotient Production/Respiration (P/R), considered as a descriptor of the ecosystem's entropy when the respiration of the whole ecosystems is taken into consideration (Odum, 1956; Margalef, 1974).
Assuming the zooplankton shift from krill to salps will lead to a zooplankton community composition equivalent to that of a typical salp year (average salps/krill = 10, Huntley et al., 1989; Loeb et al., 1997, 2010; Alcaraz et al., 1998) the proportion of PPP necessary to compensate for the C respiratory losses of total zooplankton will increase by a factor of 9. At the same time the importance of the so-called regenerative plankton loop will proportionally decrease (Parsons and Lalli, 1988; Miller et al., 1991).
In a future salps-dominated Southern Ocean around half of total primary production (TPP) and roughly 100% of particulate primary production (PPP) will be necessary to compensate for the respiratory zooplankton losses, and near 50% of it will be packed into large, fast sinking fecal pellets (Pakhomov et al., 2006), thus intensifying the rate of vertical carbon flux to deep waters and increasing the turnover time of biogenic carbon (i.e., from short-lived to long-lived and sequestered, Fortier et al., 1994; Le Fèvre et al., 1998).
Quantitative and qualitative changes in the nutrient environment for phytoplankton due to the climate shift in the Southern Ocean (Montes-Hugo et al., 2009), the lack of metabolic N:P homeostasis (Alcaraz et al., 2013) and the differential regeneration rates of N and P in relation to the food web structure (Elser et al., 1988, 1996) are, besides grazing, prime factors of change in the competitive relations for phytoplankton communities. Although the most direct effects of the predicted shifts in Southern Ocean zooplankton will derive from the different quality as food of krill and salps, the higher specific carbon demand due to the new zooplankton community structure, coupled with higher vertical carbon flux via salps fecal pellets, will strongly affect the characteristics of carbon cycling. At the same time, the increased rate of nutrient re-supply by zooplankton will increase. Coupled with the new NE:PE metabolic quotient, the N:P quotient of the nutrient pool will rise by a factor of two, a change that could contribute to modify the structure and function of primary producers (Sterner, 1986, 1990) accelerating the changes already observed in the community structure of phytoplankton by Montes-Hugo et al. (2009).
As a conclusion, a major change in the relative proportion of krill and salps in the Southern Ocean can induce significant variations in the marine food webs. Aside from the direct trophic effects, other major changes will take place via the increase of the metabolic carbon requirements of zooplankton and its vertical export, the higher overall contribution of zooplankton excretion to the nutrient requirements by phytoplankton, and the increasing N:P ratios of the recycled nutrients. Moreover, the zooplankton shift will result in a decrease of the P/R quotient (Odum, 1956; Margalef, 1974), indicator of a potential increase of the trophic efficiency of the system, while paradoxically the regeneration processes in surface waters will decrease.
The authors declare that the research was conducted in the absence of any commercial or financial relationships that could be construed as a potential conflict of interest.
The authors wish to express their gratitude to the crew of the R/V Hespérides, the technicians of the UTM and two unknown reviewers whose comments contributed significantly to clarify and improve the paper. This work was supported by the Spanish funded projects ATOS (POL 2006-0550/CTM) to Carlos M. Duarte, PERFIL (CTM 2006-12344-C01) to Miquel Alcaraz, and the UE funded project ATP (www.eu-atp.org) contract # 226248 to P. Wassmann.
Alcaraz, M. (1974). Respiración en crustáceos: influencia de la concentración de oxígeno en el medio. Inv. Pesq. 38, 397–411.
Alcaraz, M. (1988). Summer zooplankton metabolism and its relation to primary production in the western Mediterranean. Oceanol. Acta 9, 185–191.
Alcaraz, M., Almeda, R., Calbet, A., Saiz, E., Duarte, C. M., Lasternas, S., et al. (2010). The role of arctic zooplankton in biogeochemical cycles: respiration and excretion of ammonia and phosphate during summer. Polar Biol. 33, 1719–1731. doi: 10.1007/s00300-010-0789-9
Alcaraz, M., Almeda, R., Saiz, E., Calbet, A., Duarte, C. M., Agustí, S., et al. (2013). Effects of temperature on the metabolic stoichiometry of Arctic zooplankton. Biogeosciences 10, 689–697. doi: 10.5194/bg-10-689-2013
Alcaraz, M., Felipe, J., Grote, U., Arashkevich, E., and Nikishina, A. (2014). Life in a warming ocean: thermal thresholds and metabolic balance of Arctic zooplankton. J. Plankton Res. 36, 3–10. doi: 10.1093/plankt/fbt111
Alcaraz, M., Saiz, E., Calbet, A., Fernandez, J. A., Trepat, I., and Broglio, E. (2003). Estimating zooplankton biomass through image analysis. Mar. Biol. 143, 307–315. doi: 10.1007/s00227-003-1094-8
Alcaraz, M., Saiz, E., and Estrada, M. (1994). Excretion of ammonia by zooplankton and its potential contriobution to nitrogen requirements for primary production in the Catalan Sea (NW Mediterranean). Mar. Biol. 119, 69–76. doi: 10.1007/BF00350108
Alcaraz, M., Saiz, E., Fernandez, J. A., Trepat, I., Figueiras, F., Calbet, A., et al. (1998). Antarctic zooplankton metabolism: carbon requirements and ammonium excretion of salps and crustacean zooplankton in the vicinity of the Bransfield strait during January 1994. J. Mar. Syst. 17, 347–259. doi: 10.1016/S0924-7963(98)00048-7
Almeda, R., Alcaraz, M., Calbet, A., and Saiz, E. (2011). Metabolic rates and carbon budget of early developmental stages of the marine cyclopoid copepod Oithona davisae. Limnol. Oceanogr. 56, 403–414. doi: 10.4319/lo.2011.56.1.0403
Atkinson, A., Meyer, B., Stübing, D., Hagen, W., Schmidt, K., and Bathmann, U. (2002). Feeding and energy budgets of Antarctic krill Euphausia superba at the onset of winter- II. Juveniles and adults. Limnol. Oceanogr. 47, 953–966. doi: 10.4319/lo.2002.47.4.0953
Atkinson, A., Siegel, V., Pakhomov, E., and Rothery, P. (2004). Long-term decline in krill stock and increase in salps within the Southern Ocean. Nature 432, 1000–1003. doi: 10.1038/nature02996
Pubmed Abstract | Pubmed Full Text | CrossRef Full Text | Google Scholar
Auerswald, L., Pape, C., Stübing, D., Lopata, A., and Meyer, B. (2009). Effect of short-term starvation of adult Antarctic krill, Euphausia superba, at the onset of summer. J. Exp. Mar. Biol. Ecol. 381, 47–56. doi: 10.1016/j.jembe.2009.09.011
Basterretxea, G., Aristegui, J. (1999). Phytoplankton biomass and production during late spring (1991) and summer, and (1993) in the Bransfield Strait. Polar Biol. 21, 11–22. doi: 10.1007/s003000050328
Calbet, A., Alcaraz, M., Saiz, E., Estrada, M., and Trepat, I. (1996). Planktonic herbivorous food webs in the Catalan Sea (NW Mediterranean): temporal variability and comparison of indices of phytoplankton-zooplankton coupling based in state variables and rate processes. J. Planklton Res. 18, 2329–2347. doi: 10.1093/plankt/18.12.2329
Checkley, D. M., Dagg, M. J., and Uye, S. (1992). Feeding, excretion and egg production by individual and populations of the marine copepods Acartia spp. and Centropages furcatus. J. Plankton Res. 14, 71–96. doi: 10.1093/plankt/14.1.71
Conover, R. J., and Corner, E. D. S. (1968). Respiration and nitrogen excretion by some marine zooplankton in relation to their life cycles. J. Mar. Biol. Assoc. U.K. 48, 49–75. doi: 10.1017/S0025315400032410
Constable, A. J., de la Mare, W., Agnew, D. J., Everson, I., and Miller, D. (2000). Managing fisheries to conserve the Antarctic marine ecosystem: practical implementation of the convention on the conservation of Antarctic Marine Resources (CCAMLR). ICES J. Mar. Sci. 57, 778–791. doi: 10.1006/jmsc.2000.0725
Pubmed Abstract | Pubmed Full Text | CrossRef Full Text | Google Scholar
Constable, A. J., Melbourne-Thomas, J., Corney, S. P., Arrigo, K. R., Barbraud, C., Barnes, D., et al. (2014). Climate change and Southern Ocean ecosystems I: how changes in physical habitats directly affect marine biota. Global Change Biol. 20, 3004–3025. doi: 10.1111/gcb.12623
Pubmed Abstract | Pubmed Full Text | CrossRef Full Text | Google Scholar
Dagg, M. J., Urban-Rich, J., and Peterson, J. O. (2003). The potential contribution of fecal pellets from large copepods to the flux of biogenic silica and particulate organic carbon in the Antarctic Polar Front region near 170° W. Deep Sea Res. II 50, 675–691. doi: 10.1016/S0967-0645(02)00590-8
Duarte, C. M., Agustí, S., Wasmann, P., Arrieta, J. M., Alcaraz, M., Coello, A., et al. (2012). Tipping elements in the Arctic marine ecosystem. Ambio 41, 44–55. doi: 10.1007/s13280-011-0224-7
Pubmed Abstract | Pubmed Full Text | CrossRef Full Text | Google Scholar
Dubischar, C. D., Pakhomov, E. A., von Harbou, L., Hunt, B. P. V., and Bathmann, U. V. (2012). Salps in the Lazarev Sea, Southern Ocean: II. Biochemical composition and potential prey value. Mar. Biol. 159, 15–24. doi: 10.1007/s00227-011-1785-5
Ducklow, H., Clarke, A., Dickhut, R., Doney, S. C., Geisz, H., Huang, K., et al. (2012). “The marine system of the western Antarctic Peninsula,” in Antarctic Ecosystems: An Extreme Environment in a Changing World, eds A. D. Rogers, N. M. Johnston, E. J. Murphy and A. Clarke (Oxford: Blackwell Publishing Ltd.), 121–159. doi: 10.1002/9781444347241.ch5
Elser, J. J., Dobberfuhl, D. R., MacKay, N. A., and Schampel, J. H. (1996). Organism size, life history, and N:P stoichiometry. BioScience 46, 674–684. doi: 10.2307/1312897
Pubmed Abstract | Pubmed Full Text | CrossRef Full Text | Google Scholar
Elser, J. J., Elser, M. M., MacKay, N. A., and Carpenter, S. R. (1988). Zooplankton-mediated transitions between N and P limited algal growth. Limnol. Oceanogr. 33, 1–14. doi: 10.4319/lo.1988.33.1.0001
Fernandes, J. A., Irigoien, X., Boyra, G., Lozano, J. A., and Albaina, A. (2009). Optimizing the number of classes in automated zooplankton classification. J. Plankton Res. 31, 19–29. doi: 10.1093/plankt/fbn098
Figueiras, F. G., Pérez, F. F., Pazos, Y., and Rios, A. F. (2001). Dissolved and particulate primary production and bacterial production in offshore Antarctic waters during austral summer: coupled or uncoupled? Mar. Ecol. Prog. Ser. 222, 25–39. doi: 10.3354/meps222025
Fortier, L. Le Fèvre, J., and Legendre, L. (1994). Export of biogenic carbon to fish and to the deep ocean: the role of large planktonic microphages. J. Plankton Res. 16, 809–839. doi: 10.1093/plankt/16.7.809
Frazer, T. K., Quetin, L. B., and Ross, R. M. (2002). Energetic demands of larval krill, Euphausia superba, in winter. J. Exp. Mar. Biol. Ecol. 277, 157–171. doi: 10.1016/S0022-0981(02)00328-3
Grasshoff, K., Kremling, K., and Ehrhardt, M. (1999). Methods of Seawater Analysis. Weinheim: Wiley-VCH.
Harris, R. P., Wiebe, P. H., Lenz, J., Skjoldal, H. R., and Huntley, M. (2000). Zooplankton Methodology Manual. London: Academic Press.
Heusner, A. A. (1982). Energy metabolism and body size. I. Is the 0.75 mass exponent of Kleiber's equation a statistical artifact? Respir Physiol. 48, 1–12. doi: 10.1016/0034-5687(82)90046-9
Pubmed Abstract | Pubmed Full Text | CrossRef Full Text | Google Scholar
Hewes, C. D., Sakshaug, E., Reid, F. M. H., and Holm-Hansen, O. (1990). Microbial autotrophic and heterotrophic eucaryotes in Antarctic waters: relationships between biomass and chlorophyll, adenosine triphosphate and particulate organic carbon. Mar. Ecol. Prog. Ser. 63, 27–35. doi: 10.3354/meps063027
Hirche, H. J. (1983). Excretion and respiration of the Antarctic krill Euphausia superba. Polar Biol. 1, 205–209. doi: 10.1007/BF00443189
Huntley, M. E., Sykes, P. F., and Martin, V. (1989). Biometry and trophodynamics of Salpa thompsoni Foxton (Tunicata: Thaliacea) near the Antarctic Peninsula in austral summer, 1983-1984. Polar Biol. 10, 59–70. doi: 10.1007/BF00238291
Iguchi, N., and Ikeda, T. (2004). Metabolism and elemental composition of aggregate and solitary forms of Salpa thompsoni (Tunicata: Thaliacea) in waters off the Antarctic Peninsula during austral summer 1999. J. Plankton Res. 26, 1025–1037. doi: 10.1093/plankt/fbh093
Ikeda, T. (1976). The effect of laboratory conditions on the extrapolation of experimental measurements to the ecology of marine zooplankton. I. Effect of feeding conditions on the respiration rate. Bull. Plankton Soc. Jpn. 23, 1–10.
Ikeda, T. (1977). The effect of laboratory conditions on the extrapolation of experimental measurements to the ecology of marine zooplankton. IV. Changes in respiration and excretion rates of boreal zooplankton species maintained under fed and starved conditions. Mar. Biol. 41, 241–252. doi: 10.1007/BF00394910
Ikeda, T. (1985). Metabolic rates of epipelagic marine zooplankton as a function of body mass and temperature. Mar. Biol. 85, 1–12. doi: 10.1007/BF00396409
Ikeda, T., and Bruce, B. (1986). Metabolic activity and elemental composition of krill and other zooplankton from Prydz Bay, Antarctica, during early summer (November-December). Mar. Biol. 92, 545–555. doi: 10.1007/BF00392514
Ikeda, T., and Mitchell, A. W. (1982). Oxygen uptake, ammonia excretion and phosphate excretion by krill and other Antarctic zooplankton in relation to their body size and chemical composition. Mar. Biol. 71, 283–289. doi: 10.1007/BF00397045
Kéruel, R., and Aminot, A. (1997). Fluorometric determination of ammonia in sea and estuarine waters by direct segmented flow analysis. Mar. Chem. 57, 265–275. doi: 10.1016/S0304-4203(97)00040-6
Le Fèvre, J., Legendre, L., and Rivkin, R. B. (1998). Fluxes of biogenic carbon in the Southern Ocean: roles of large microphagous zooplankton. J. Mar. Syst. 17, 325–345. doi: 10.1016/S0924-7963(98)00047-5
Lehette, P., Tovar-Sánchez, A., Duarte, C. M., and Hernández-Leon, S. (2012). Krill excretrion and its effects on primary production. Mar. Ecol. Prog. Ser. 459, 29–38. doi: 10.3354/meps09746
Loeb, V., Hofmann, E. E., Klinck, J. M., and Holm-Hansen, O. (2010). Hydrographic control of the marine ecosystem in the South Shetland-Elephant island and Bransfield strait region. Deep-Sea Res. II 57, 519–542. doi: 10.1016/j.dsr2.2009.10.004
Loeb, V., Siegel, V., Holm-Hansen, O., Hewitt, R., Fraser, W., Trivelpiece, W., et al. (1997). Effects of sea-ice extent and krill or salp dominance on the Antarctic food web. Nature 387, 897–900. doi: 10.1038/43174
Marshall, S. M., Nicholls, A. G., and Orr, A. P. (1935). On the biology of Calanus finmarchicus VI. Oxygen consumption in relation to environmental conditions. J. Mar. Biol. Ass. U.K. 20, 341–346. doi: 10.1017/S0025315400009991
Mayzaud, P. (1973). Respiration and nitrogen excretion of zooplankton. II. Studies on the metabolic characteristics of starved animals. Mar. Biol. 21, 19–28. doi: 10.1007/BF00351188
Mayzaud, P., and Conover, R. J. (1988). O:N atomic ratio as a tool to describe zooplankton metabolism. Mar. Ecol. Prog. Ser. 45, 289–302. doi: 10.3354/meps045289
Meyer, B., Auerswald, L., Siegel, V., Spahic, S., Pape, C., Fach, B. A., et al. (2010). Seasonal variation in body composition, metabolic activity, feeding, and growth of adult krill Euphausia superba in the Lazarev Sea. Mar. Ecol. Prog. Ser. 398, 1–18. doi: 10.3354/meps08371
Meyer, B., Fuentes, V., Guerra, C., Schmidt, K., And others (2009). Physiology, growth and development of larval krill Euphausia superba in autumn and Winter in the Lazarev Sea, Antarctica. Limnol. Oceanogr. 54, 1595–1614. doi: 10.4319/lo.2009.54.5.1595
Meyer, B., and Oettl, B. (2005). Effects of short-term starvation on composition and metabolism of larval Antarctic krill Euphausia superba. Mar. Ecol. Prog. Ser. 292, 263–270. doi: 10.3354/meps292263
Miller, C. B., Frost, B. W., Booth, B., Wheeler, P. A., Landry, M. R., and Welschmeyer, N. (1991). Ecological processes in the subarctic Pacific: iron limitation cannot be the whole story. Oceanography, 4, 71–78. doi: 10.5670/oceanog.1991.05
Montes-Hugo, M., Doney, S. C., Ducklow, H., Fraser, W., Martinson, D., Stammerjoh, S. E., et al. (2009). Recent changes in phytoplankton communities associated with rapid regional climate change along the Western Antarctic Peninsula. Science 323, 1470–1473. doi: 10.1126/science.1164533
Pubmed Abstract | Pubmed Full Text | CrossRef Full Text | Google Scholar
Morán, X. A., Gasol, J. M., Pedrós-Alió, C., and Estrada, M. (2001). Dissolved and particulate primary production and bacterial production in offshore Antarctic waters during austral summer: coupled or uncoupled? Mar. Ecol. Prog. Ser. 222, 25–39. doi: 10.3354/meps222025
Murphy, E. J., Watkins, J. L., Trathan, P. N., Reid, K., Meredith, M. P., Thorpe, S. E., et al. (2007). Spatial and temporal operation of the Scotia Sea ecosystem: a review of large-scale links in a centered food web. Phyl. Trans. R. Soc. B 362, 1213–1148.
Nishikawa, J., Naganobu, M., and Ichii, T. (1995). Distribution of salps near the South Shetland Islands during austral summer, 1990-1991 with special reference to krill distribution. Polar Biol. 15, 31–39. doi: 10.1007/BF00236121
Odum, H. T. (1956). Primary production in flowing waters. Limnol. Oceanogr. 1, 102-117. doi: 10.4319/lo.1956.1.2.0102
Omori, M. (1978). Zooplankton fisheries of the world: a review. Mar. Biol. 48, 199–205. doi: 10.1007/BF00397145
Pubmed Abstract | Pubmed Full Text | CrossRef Full Text | Google Scholar
Pakhomov, E. A. (2004). Salp/krill interactions in the eastern Atlantic sector of the Southern Ocean. Deep Sea Res. II 51, 2645–2660. doi: 10.1016/j.dsr2.2001.03.001
Pakhomov, E. A., Dubischar, C. D., Strass, V., Brichta, M., and Bathmann, U. V. (2006). The tunicate Salpa thompsoni ecology in the Southern Ocean. I. Distribution, biomass, demography and feeding ecophysiology. Mar. Biol. 149, 609–623. doi: 10.1007/s00227-005-0225-9
Pakhomov, E. A., Froneman, P. W., and Perissinotto, R. (2002). Salp/krill interactions in the Southern Ocean: spatial segregation and implications for the carbon flux. Deep Sea Res. II 49, 1881–1907. doi: 10.1016/S0967-0645(02)00017-6
Parsons, T. R., and Lalli, C. M. (1988). Comparative oceanic ecology of the plankton communities of the subarctic Atlantic and Pacific Oceans. Mar. Biol. A. Rev. 26, 317–359.
Parsons, T. R., Maita, Y., and Lalli, C. M. (1984). A Manual of Chemical and Biological Methods for Seawater Analysis. Cambridge: Pergamonn Press.
Pauly, T., Nicol, S., Higginbottom, I., Hosie, G., and Kitchener, J. (2000). Distribution and abundance of Antarctic krill (Euphausia superba) off East Antarctica (80-150° E) during the Austral summer of 1995-1996. Deep Sea Res. II 47, 2465–2488. doi: 10.1016/S0967-0645(00)00032-1
Perissinotto, R., and Pakhomov, E. A. (1998). The trophic role of the tunicate Salpa thompsoni in the Antarctic marine ecosystem. J. Mar. Syst. 17, 361–374. doi: 10.1016/S0924-7963(98)00049-9
Phillips, B., Kremer, P., and Madin, L. P. (2009). Defecation by Salpa thompsoni and its contribution to vertical flux in the Southern Ocean. Mar. Biol. 156, 455–467. doi: 10.1007/s00227-008-1099-4
Pitt, K. A., Duarte, C. M., Lucas, C. H., Sutherland, K. R., Condon, R. H., Mianzan, H., et al. (2013). Jellyfish body plans provide allometric advantages beyond low carbon content. PLoS ONE 8:e72683. doi: 10.1371/journal.pone.0072683
Pubmed Abstract | Pubmed Full Text | CrossRef Full Text | Google Scholar
Pond, D. W., Priddle, J., Sargent, J. R., and Watkins, J. L. (1995). Laboratory studies of assimilation and egestion of algal lipid by Antarctic krill- methods and initial results. J. Exp. Mar. Biol. Ecol. 187, 253–268. doi: 10.1016/0022-0981(94)00187-I
Redfield, A. C., Ketchum, B. H., and Richards, F. A. (1963). “The influence of organisms in the composition of seawater,” in The Sea, ed M. N. Hill (New York, NY: Interscience), 26–76.
Riisgård, H. U. (1998). No foundation of a “3/4 power scaling law” for respiration in biology. Ecol. Lett. 1, 71–73. doi: 10.1046/j.1461-0248.1998.00020.x
Ross, R., Quetin, L. B., Martinson, D. G., Iannuzzi, R. A., Stammerjohn, S. E., and Smith, R. C. (2008). Palmer LTR: Patterns of distribution of five dominant zooplankton species in the epipelagic zone west of the Antarctic Peninsula, 1993-2004. Deep Sea Res. II 55, 2086–2105. doi: 10.1016/j.dsr2.2008.04.037
Ruiz-Halpern, S., Duarte, C. M., Tovar-Sanchez, A., Pastor, M., Hortskotte, B., Lasternas, S., et al. (2011). Antarctic krill as a source of dissolved organic carbon to the Antarctic ecosystem. Limnol. Oceanogr, 56, 521–528. doi: 10.4319/lo.2011.56.2.0521
Saiz, E., Calbet, A., Isari, S., Antó, M., Velasco, E. M., Almeda, R., et al. (2013). Zooplankton distribution and feeding in the Arctic Ocean during a Phaeocystis pouchetii Bloom. Deep Sea Res I 72, 17–33. doi: 10.1016/j.dsr.2012.10.003
Sameoto, D., Wiebe, P., Runge, L., Postel, L., Dunn, J., Miller, C., et al. (2000). “Collecting zooplankton,” in Zooplankton Methodology Manual, ed R. P. Harris, P. H. Wiebe, J. Lenz, H. R. Skjoldal, and M. Huntley (London: Academic Press), 55–81. doi: 10.1016/B978-012327645-2/50004-9
Schneider, G. (1990). A comparison of carbon based ammonia excretion rates between gelatinous and non-gelatinous zooplankton: implications and consequences. Mar. Biol. 106, 219–225. doi: 10.1007/BF01314803
Smetacek, V. (2008). “Are declining Antarctic krill stocks a result of a global warming or the decimation of whales?” in Impacts of Global Warming on Polar Ecosystems, ed C. Duarte (Bilbao: Fundación BBVA), 45–81.
Smetacek, V., and Nicol, S. (2005). Polar ocean ecosystems in a changing world. Nature 437, 362–368. doi: 10.1038/nature04161
Pubmed Abstract | Pubmed Full Text | CrossRef Full Text | Google Scholar
Steemann-Nielsen, E. J. (1952). The use of radioactive carbon (l4C) for measuring organic production in the sea. Cons. Perm. Int. Explor. Mer. 18, 117–140. doi: 10.1093/icesjms/18.2.117
Sterner, R. W. (1986). Herbivores' direct and indirect effect on algal populations. Science 231, 605–607. doi: 10.1126/science.231.4738.605
Pubmed Abstract | Pubmed Full Text | CrossRef Full Text | Google Scholar
Sterner, R. W. (1990). The ratio of nitrogen to phosphorus resupplied by herbivores: Zooplankton and the algal arena. Am. Nat. 136, 209–229. doi: 10.1086/285092
Tanimura, A., Kawaguchi, S., Oka, N., Nishkawa, J., Toczko, S., Takahashi, K. T., et al. (2008). Abundance and grazing impacts of krill, salps and copepods along the 140° E meridian in the Southern Ocean during summer. Antarct. Sci. 20, 365–379. doi: 10.1017/S0954102008000928
Tovar-Sanchez, A. C., Duarte, C. M., Hernández-León, S., and Sañudo Wilhemy, S. A. (2007). Krill as a central node for iron cycling in the Southern Ocean. Geophys. Res. Lett. 34, L11601, doi: 10.1029/2006GL029096.
Ward, P., Atkinson, A., Murray, A. W. A., Wood, A. G., Williams, R., and Poulet, S. A. (1995). The summer zooplankton community at South Georgia - biomass, vertical migration and grazing. Polar Biol. 15, 195–208. doi: 10.1007/BF00239059
Ward, P., Grant, S., Brandon, M., Siegel, V., Sushin, V., Loeb, V., et al. (2004). Mesozooplankton community structure in the Scotia Sea during the CCAMLR 2000 survey: January-February 2000. Deep Sea Res. II 51, 1351–1367. doi: 10.1016/j.dsr2.2004.06.016
Wassmann, P., Carroll, J., and Bellerby, R. G. J. (2008). Carbon flux and ecosystem feedback in the northern Barents Sea in an era of climate change. Deep-Sea Res. PT II, 55, 2143–2153. doi: 10.1016/j.dsr2.2008.05.025
Keywords: Southern Ocean, zooplankton, community shifts, metabolism, carbon cycling, C:N:P stoichiometry
Citation: Alcaraz M, Almeda R, Duarte CM, Horstkotte B, Lasternas S and Agustí S (2014) Changes in the C, N, and P cycles by the predicted salps-krill shift in the southern ocean. Front. Mar. Sci. 1:45. doi: 10.3389/fmars.2014.00045
Received: 10 June 2014; Accepted: 09 September 2014;
Published online: 29 September 2014.
Edited by:
Paul F. J. Wassmann, University of Tromsø - Norway's Arctic University, NorwayReviewed by:
Joanna Carey, Marine Biological Laboratory, USACopyright © 2014 Alcaraz, Almeda, Duarte, Horstkotte, Lasternas and Agustí. This is an open-access article distributed under the terms of the Creative Commons Attribution License (CC BY). The use, distribution or reproduction in other forums is permitted, provided the original author(s) or licensor are credited and that the original publication in this journal is cited, in accordance with accepted academic practice. No use, distribution or reproduction is permitted which does not comply with these terms.
*Correspondence: Miquel Alcaraz, Institut de Ciènces del Mar, CSIC, P. Marítim de la Barceloneta 37-49, 08003 Barcelona, Catalonia, Spain e-mail:bWlxdWVsQGljbS5jc2ljLmVz
Disclaimer: All claims expressed in this article are solely those of the authors and do not necessarily represent those of their affiliated organizations, or those of the publisher, the editors and the reviewers. Any product that may be evaluated in this article or claim that may be made by its manufacturer is not guaranteed or endorsed by the publisher.
Research integrity at Frontiers
Learn more about the work of our research integrity team to safeguard the quality of each article we publish.