- 1School of Anatomical Sciences, Faculty of Health Sciences, University of the Witwatersrand, Johannesburg, South Africa
- 2Department of Anthropology and Center for the Advanced Study of Human Paleobiology, The George Washington University, Washington, DC, United States
- 3Nash Family Department of Neuroscience, Center for Discovery and Innovation, Icahn School of Medicine at Mount Sinai, New York, NY, United States
- 4Friedman Brain Institute, Icahn School of Medicine at Mount Sinai, New York, NY, United States
- 5Centre for Zoo and Wild Animal Health, Copenhagen Zoo, Frederiksberg, Denmark
- 6Department of Zoology, King Saud University, Riyadh, Saudi Arabia
- 7Mammal Research Institute, Department of Zoology and Entomology, University of Pretoria, Pretoria, South Africa
- 8Faculté des Sciences, University of Kisangani, Kisangani, Democratic Republic of Congo
- 9Department of African Zoology, Royal Museum for Central Africa, Tervuren, Belgium
- 10Laboratory of Histology and Neuropathology, Université Libre de Bruxelles, Brussels, Belgium
In the current study we employed immunohistochemical techniques to investigate the localisation of three calcium-binding proteins (parvalbumin, calbindin, and calretinin) in 10 neuronal structures of the cerebellar cortex (stellate cells, basket cells, parallel fibres, climbing fibres, Purkinje cells, granule cells, Golgi type II cells, Lugaro neurons, unipolar brush neurons, and mossy fibres) in 143 species from across the mammalian radiation. Most often, parvalbumin was localised in the neural structures of the molecular and Purkinje cell layers but was absent in the granule cell layer. Calbindin was most often immunolocalised in the neural structures of the Purkinje cell layer and mossy fibres, whereas calretinin was most often immunolocalised in the climbing fibres of the molecular layer and all neural structures of the granule cell layer. Despite this general consistency, variations in the localisation of these three calcium-binding proteins were found in every lineage, and almost every species, the one exception being the western tree hyrax that showed the full suite of most often observed calcium-binding protein chemoarchitecture for the mammalian cerebellar cortex. These consistencies and variances in the calcium-binding protein chemoarchitecture of the cerebellar cortex of mammals may play significant roles in the species-specific learning and refining of motor, perceptual, and cognitive skills and capacities required to survive in the environments they inhabit.
1 Introduction
The mammalian cerebellum contains approximately 73% (± 10%) of the total number of neurons found in the mammalian brain (Herculano-Houzel et al., 2015). In African elephant brains, the cerebellum contains an extraordinary 97.5% of the total brain neurons (Herculano-Houzel et al., 2014), with the vast majority of these being the granule cells of the cerebellar cortex. The cerebellar cortex, in all vertebrates studied to date, has a trilaminate architecture, each cerebellar cortical layer being comprised of a distinct subset of neural structures (Kappers et al., 1960). Within the most superficial layer, the molecular layer, stellate, and basket cells, as well as parallel and climbing fibres are located. The Purkinje cell layer is comprised primarily of a monolayer of the large Purkinje cells. Within the deepest layer, the granule cell layer, contains granule cells, Golgi type II cells, Lugaro neurons, unipolar brush neurons, and mossy fibres (Hull and Regehr, 2022).
This complement of cerebellar cortical neurons has long been thought to be organised into a trisynaptic arc, forming repeated modules, that convert incoming mossy fibre inputs into unique discharge patterns of the granule cells, that in turn modify the output of Purkinje cells to regulate motor outputs – the Marr-Albus model (Marr, 1969; Albus, 1971; Ito, 1972). While this view has not been completely overturned, it is clear based on more recent findings, that the cerebellar cortex is far more complex than envisaged in this model, as the cerebellar cortex has a greater diversity of cell types (based on molecular analyses), with greater functionality, connectivity, regional specializations, modulatory mechanisms, and population dynamics (e.g., Hawkes, 2018; Hull and Regehr, 2022). While not explicitly stated, it is implicit that these older and more recent concepts of cerebellar cortical structure and physiology apply to the cerebellar cortex of all vertebrates.
Calcium dynamics are an essential part of intracellular signalling that controls several key compartmental functions in all neuronal types (e.g., Grienberger and Konnerth, 2012). The calcium-binding proteins, parvalbumin (PV), calbindin D-28k (CB, referred to as calbindin herein), and calretinin (CR) affect intraneuronal calcium transients (e.g., Schwaller et al., 2002). PV acts as a slow buffer, CB a faster buffer, and CR a modulator, of calcium dynamics (e.g., Billing-Marczak and Kuznicki, 1999; Schmidt et al., 2003; Barinka and Druga, 2010). The calcium-binding proteins have been reported to be expressed in specific neuron types and cellular structures in the cerebellar cortex of rodents (e.g., Rogers, 1989; Celio, 1990; Résibois and Rogers, 1992; Schwaller et al., 2002); however, in other species such as the short-beaked echidna (Ashwell et al., 2007), bottlenose dolphin (Kalininchenko and Pushchin, 2008), human (Satoh et al., 1991; Braak and Braak, 1993; Flace et al., 2014), African elephant (Maseko et al., 2013a), and tree pangolin (Imam et al., 2019), the expression pattern of these calcium-binding proteins is substantially different to that observed in the laboratory rodents.
This apparent phylogenetic variance in the distribution of the calcium-binding proteins prompted us to undertake a more comprehensive comparative study of cerebellar chemoarchitecture in mammals. In the current study, we analysed the immunolocalization of three calcium-binding proteins (PV, CB, and CR) for 10 neuronal structures of the cerebellar cortex (stellate cells, basket cells, parallel fibres, climbing fibres, Purkinje cells, granule cells, Golgi type II cells, Lugaro neurons, unipolar brush neurons, and mossy fibres) in 143 mammalian species, a phylogenetic diversity that encompasses much of the mammalian radiation.
2 Materials and methods
2.1 Specimens
The data reported here was derived from two sources: (a) previously published literature (6 species); and (b) tissue processed and analysed specifically for the current study (137 species). From the literature, we obtained information for the short-beaked echidna (Ashwell et al., 2007), the tree pangolin (Imam et al., 2019), the bottlenose dolphin (Kalininchenko and Pushchin, 2008), the Norway rat (Rogers, 1989; Celio, 1990; Résibois and Rogers, 1992), humans (Satoh et al., 1991; Braak and Braak, 1993; Flace et al., 2014), and the African elephant (Maseko et al., 2013a). Blocks of cerebellar cortex for each of the 137 specimens were prepared and examined specifically for the current study. All tissue blocks used in the current study were from adults of the species and were sampled from the vermal region of the cerebellum and included several folia. For the specimens curated in the Manger laboratory, the animals were treated and used according to the guidelines of the University of Witwatersrand Animal Ethics Committee (AESC No. 2012/53/01) that correspond to those of the NIH for care and use of animals in scientific experimentation. The brains were obtained after the animals were vv with sodium pentobarbital (i.p.) in line with management decisions independent of the current study (Bertelsen, 2018), or for use in other unrelated studies. For smaller mammals, once euthanised, the right atrium was opened, and the left ventricle cannulated to allow whole body perfusion with an initial rinse of 0.9% saline solution at a temperature of 4°C, followed by 4% paraformaldehyde in 0.1 M phosphate buffer (PB) at 4°C. For larger mammals, once euthanised the heads were removed and the cranial vasculature perfused with an initial rinse of 0.9% saline solution at a temperature of 4°C, followed by 4% paraformaldehyde in 0.1 M phosphate buffer (PB) at 4°C (e.g., Manger et al., 2009). The brains, which showed no signs of neuropathology, were removed from the skull and post-fixed in 4% paraformaldehyde in 0.1 M PB (24 h at 4°C), allowed to equilibrate in 30% sucrose in 0.1 M PB and then stored in an antifreeze solution at -20°C until use (Manger et al., 2009).
For the specimens curated in the Sherwood laboratory, the animals were treated and used according to the guidelines of the George Washington University Animal Ethics Committee (IACUC number A2002-023), that correspond to those of the NIH for care and use of animals in scientific experimentation. Brains were collected opportunistically from subjects following death unrelated to the current study from individuals that lived in zoos and research centres. Within 24 hours of each subject’s death, the brain was removed and immersed in 10% formalin. In most cases, the brain was transferred to 0.1 M phosphate buffered saline (PBS) with 0.1% sodium azide solution after 10-14 days and stored at 4° C until use in the current study.
2.2 Sectioning and immunohistochemical staining
The cerebellar blocks were allowed to equilibrate in 30% sucrose in 0.1 M PB, and subsequently frozen in crushed dry ice. The frozen blocks were mounted to an aluminium stage and a 1 in 4 series of sections of 50 µm thickness were cut using a sliding microtome. The four series were stained for Nissl, PV, CB, and CR (Table 1). Sections used for Nissl staining were mounted on 0.5% gelatine-coated glass slides and then cleared in a solution of 1:1 chloroform and 100% alcohol overnight, after which the sections were then stained with 1% cresyl violet.
The sections used for immunohistochemistry were initially treated for 30 min with an endogenous peroxidase inhibitor (49.2% methanol: 49.2% 0.1 M PB: 1.6% of 30% H2O2), followed by three 10 min rinses in 0.1 M PB. The sections were then pre-incubated at room temperature for 3 h in a blocking buffer solution containing 3% normal goat serum (NGS, BioWest, Nuaillé, France), 2% bovine serum albumin (BSA, Sigma) and 0.25% Triton X-100 (Merck) in 0.1 M PB. The sections were then placed in a primary antibody solution (blocking buffer with appropriately diluted primary antibody, Table 1) and incubated at 4°C for 48 h under gentle agitation. To identify parvalbumin, calbindin and calretinin containing structures we used anti-PV, anti- CB, and anti- CR antibodies (Table 1). For all immunostains, the incubation in the primary antibody solution was followed by three 10 min rinses in 0.1 M PB, after which the sections were incubated in a secondary anti-rabbit antibody solution for 2 h at room temperature. The secondary antibody solution contained a 1:1000 dilution of biotinylated anti-rabbit IgG (BA-1000, Vector Labs), in a solution containing 3% NGS and 2% BSA in 0.1 M PB. This was followed by three 10 min rinses in 0.1 M PB after which the sections were incubated in AB solution (Vector Labs) for 1 h. After three further 10 min rinses in 0.1 M PB, the sections were placed in a solution of 0.05% diaminobenzidine in 0.1 M PB for five minutes (2 ml/section), followed by the addition of 3 µl of 30% H2O2 to each 1 ml of solution in which each section was immersed. Chromatic precipitation of the sections was monitored visually under a low power stereomicroscope, this process being allowed to continue until the background staining of the sections was appropriate for analysis without obscuring any immunoreactive neuronal structures. The precipitation process was terminated by immersing the sections in 0.1 M PB and then rinsing them twice more in 0.1 M PB. To check for non-specific staining from the immunohistochemistry protocol, we omitted the primary antibody and the secondary antibody in selected sections, which produced no evident staining. The immunohistochemically stained sections were mounted on 0.5% gelatine-coated slides and left to dry overnight. The sections were subsequently dehydrated in graded series of alcohols, cleared in xylene, and cover slipped with DePeX.
2.3 Analysis
All sections were examined with a light microscope at low and high magnifications, and the immunoreactivity of the 10 structures in each species was recorded (Tables 2–4). Digital photomicrographs were captured using an Axiocam 208 colour camera mounted to a Zeiss Axioskop microscope (with Zeiss A-Plan 5X/012, Zeiss Plan-NeoFluar 10X/0.30, and Zeiss Plan-NeoFluar 40X/0.75 objectives). No pixelation adjustments, or manipulation of the captured images were undertaken, except for the adjustment of contrast, brightness, and levels using Adobe Photoshop.

Table 2 Presence (+), absence (-), or lack of data (dd – data deficient) of parvalbumin immunostaining in various neural structures of the cerebellar cortex in mammalian species.

Table 3 Presence (+), absence (-), or lack of data (dd – data deficient) of calbindin immunostaining in various neural structures of the cerebellar cortex in mammalian species.

Table 4 Presence (+), absence (-), or lack of data (dd – data deficient) of calretinin immunostaining in various neural structures of the cerebellar cortex in mammalian species.
2.4 Antibody characterization and specificity
The antibodies used and associated details are listed in Table 1.
2.4.1 Parvalbumin
To reveal neurons expressing PV, we used the PV28 anti-PV rabbit polyclonal antibody from Swant (PV28, Swant; RRID AB_10000343) at a dilution of 1:5000. The pattern of immunoreactivity in the cerebellar cortex was mostly similar across mammals, but with specific variances of interest.
2.4.2 Calbindin
To reveal neurons expressing CB, we used the CB38a anti-CB rabbit polyclonal antibody from Swant (CB38a, Swant; RRID AB_10000340) at a dilution of 1:5000. The pattern of immunoreactivity in the cerebellar cortex was mostly similar across mammals, but with specific variances of interest.
2.4.3 Calretinin
To reveal expressing CR, we used the 7699/3H anti-CR rabbit polyclonal antibody from Swant (7699/3H, Swant; RRID AB_10000321) at a dilution of 1:5000. The pattern of immunoreactivity in the cerebellar cortex was mostly similar across mammals, but with specific variances of interest.
3 Results
Here we report our observations, supplemented by previous reports in the literature (Tables 2–4), detailing the immunolocalization of three calcium-binding proteins (PV, CB, CR) within 10 specific structures of the cerebellar cortex from 143 different mammal species. While the localization of these calcium-binding proteins showed substantive consistency across species, significant variations in a range of cell types and neural structures were noted with these variations being both lineage-specific and species-specific.
3.1 Laminar architecture of the cerebellar cortex
In all species studied, Nissl staining revealed a trilaminate structure of the cerebellar cortex (Figure 1). The three layers observed include the superficial, neuron sparse, molecular layer, deep to which was the monolayer of Purkinje cells. Deep to the Purkinje cell layer, the cell dense granular layer was observed, comprised not only of a high density of small granule cells, but also lower densities of Golgi type II neurons, Lugaro neurons, and unipolar brush cells. No mammal species investigated exhibited any specific difference in the laminar organization of the cerebellar cortex. Variations observed in the thickness of the layers (Figure 1) in the present study may only be reliably related to the plane of section rather than specific variations in layer thicknesses in different lineages or species and were not measured. Nissl staining indicated that all the commonly reported neuronal elements that form the cerebellar cortex were present in all mammal species investigated.
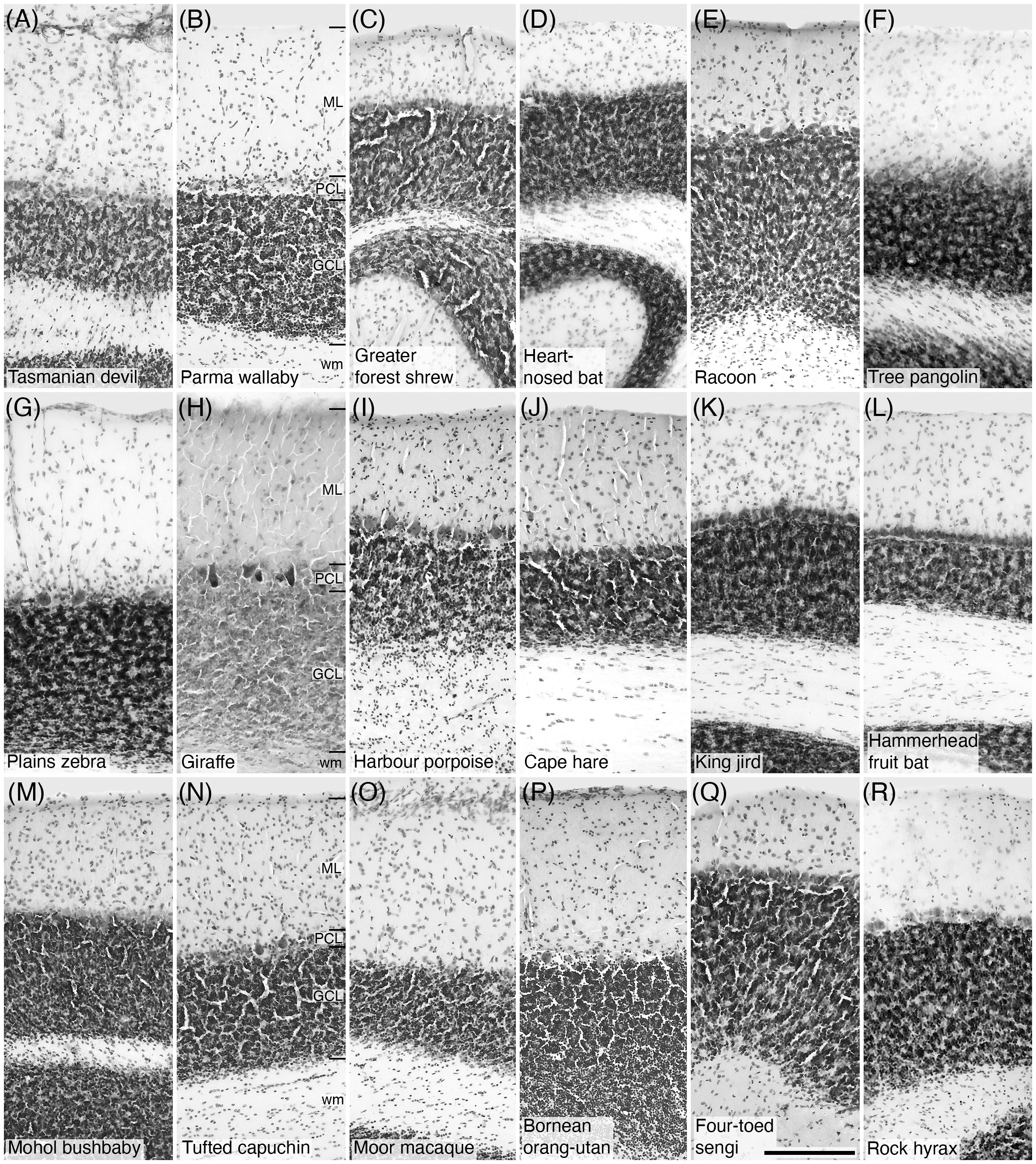
Figure 1 Photomicrographs of Nissl-stained sections showing the laminar pattern of the cerebellar cortex in eighteen of the species studied. (A) Tasmanian devil; (B) Parma wallaby; (C) Greater forest shrew; (D) Heart-nosed bat; (E) Raccoon; (F) Tree pangolin; (G) Plains zebra; (H) Giraffe; (I) Harbour porpoise; (J) Cape hare; (K) King jird; (L) Hammerhead fruit bat; (M) Mohol bushbaby; (N) Tufted capuchin; (O) Moor macaque; (P) Bornean orang-utan; (Q) Four-toed sengi; (R) Rock hyrax. In all species studied a trilaminate pattern was observed, the most superficial layer being the molecular layer (ML), the monolayer Purkinje cell layer (PCL) lying in the middle, and the granule cell layer (GCL) being the most internal layer lying just above the white matter (wm), these layers are demarcated in (B, H, N), but are apparent in all photomicrographs. Scale bar in (A) = 200 µm and applies to all. For species names, please refer to Tables 1–4.
3.2 Stellate cells
Stellate cells were observed in the upper part of the molecular layer of the cerebellar cortex in all species studied (Figure 1), and these cells exhibited similarly shaped soma, although the immunostains used in the current study did not consistently reveal the dendrites. In most of the species studied the stellate cells were parvalbumin-immunoreactive (PV+) (Figure 2; Table 2). An absence of parvalbumin-immunoreactivity (PV-) within stellate cells was observed in the three closely related Cetartiodactyla subfamilies Hippotraginae, Alcelaphinae, and Caprinae. PV- stellate cells were noted in the short-beaked echidna, the African buffalo, the minke whale, the Cape hare, and the two-toed sloth (Table 2). In the majority of examined species the stellate cells were immunonegative for calbindin (CB-), although calbindin-immunoreactive (CB+) stellate cells were noted in the African buffalo, human (Flace et al., 2014), and the African elephant (Maseko et al., 2013a) (Figure 3A; Table 3). While most species investigated revealed an absence of calretinin-immunoreactivity (CR-) in stellate cells (Table 4), calretinin-immunoreactive (CR+) stellate cells were noted in the cetaceans studied, and the short-beaked echidna (Ashwell et al., 2007), the Tasmanian devil, the European polecat, the Asian small-clawed otter, the raccoon, the Northern fur seal, the tree pangolin (Imam et al., 2019), the Arabian oryx, the Arabian ibex, the African buffalo, the dromedary camel, and the black-rumped agouti (Figures 3B-J, 4; Table 4).
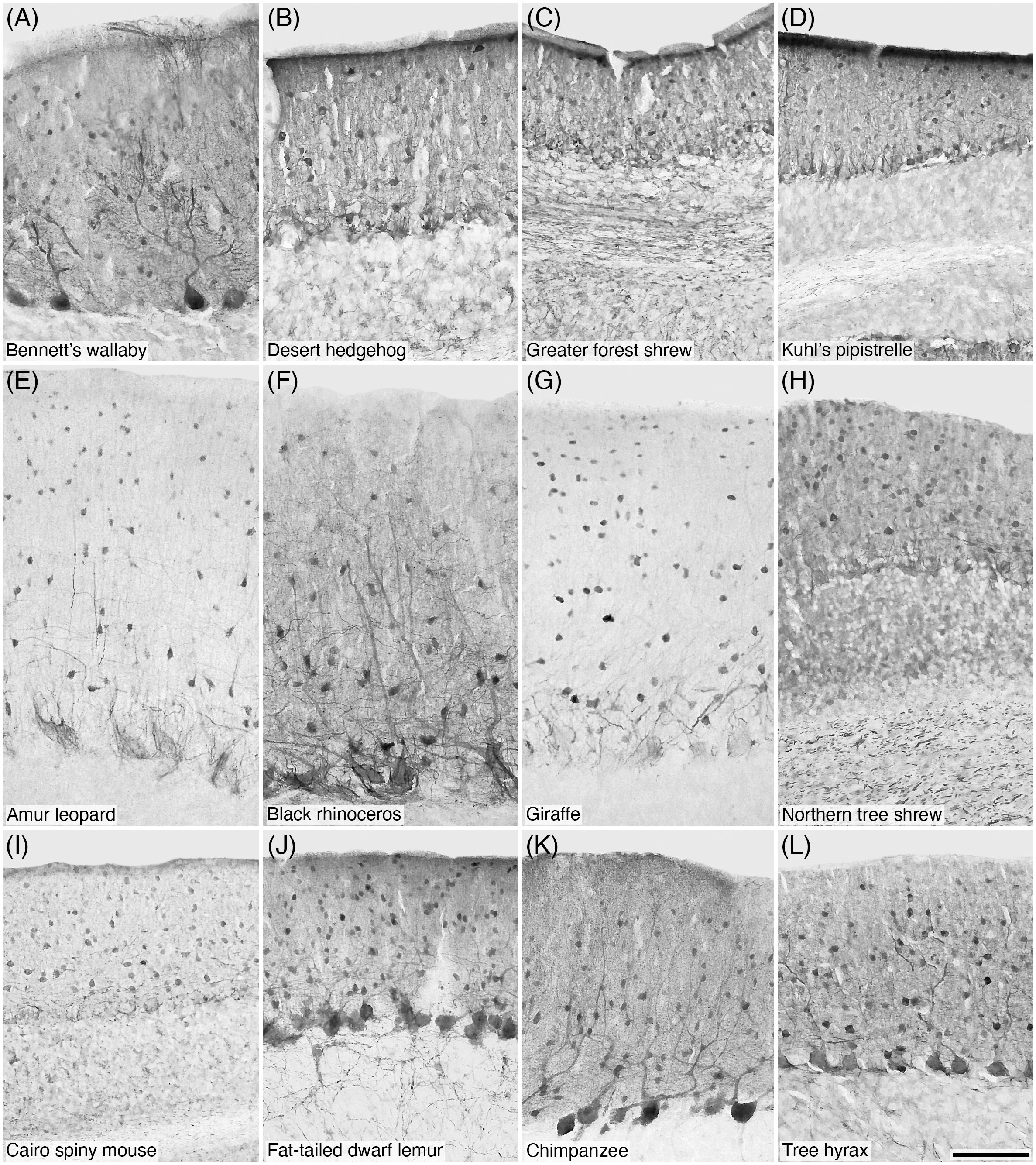
Figure 2 Photomicrographs of PV+ stellate and basket cells in the molecular layer of the cerebellar cortex of twelve of the species studied: (A) Bennett’s wallaby; (B) Desert hedgehog; (C) Greater forest shrew; (D) Kuhl’s pipistrelle; (E) Amur leopard; (F) Black rhinoceros; (G) Giraffe; (H) Northern tree shrew; (I) Cairo spiny mouse; (J) Fat-tailed dwarf lemur; (K) Chimpanzee; and (L) Tree hyrax. Scale bar in (L) = 100 µm and applies to all.
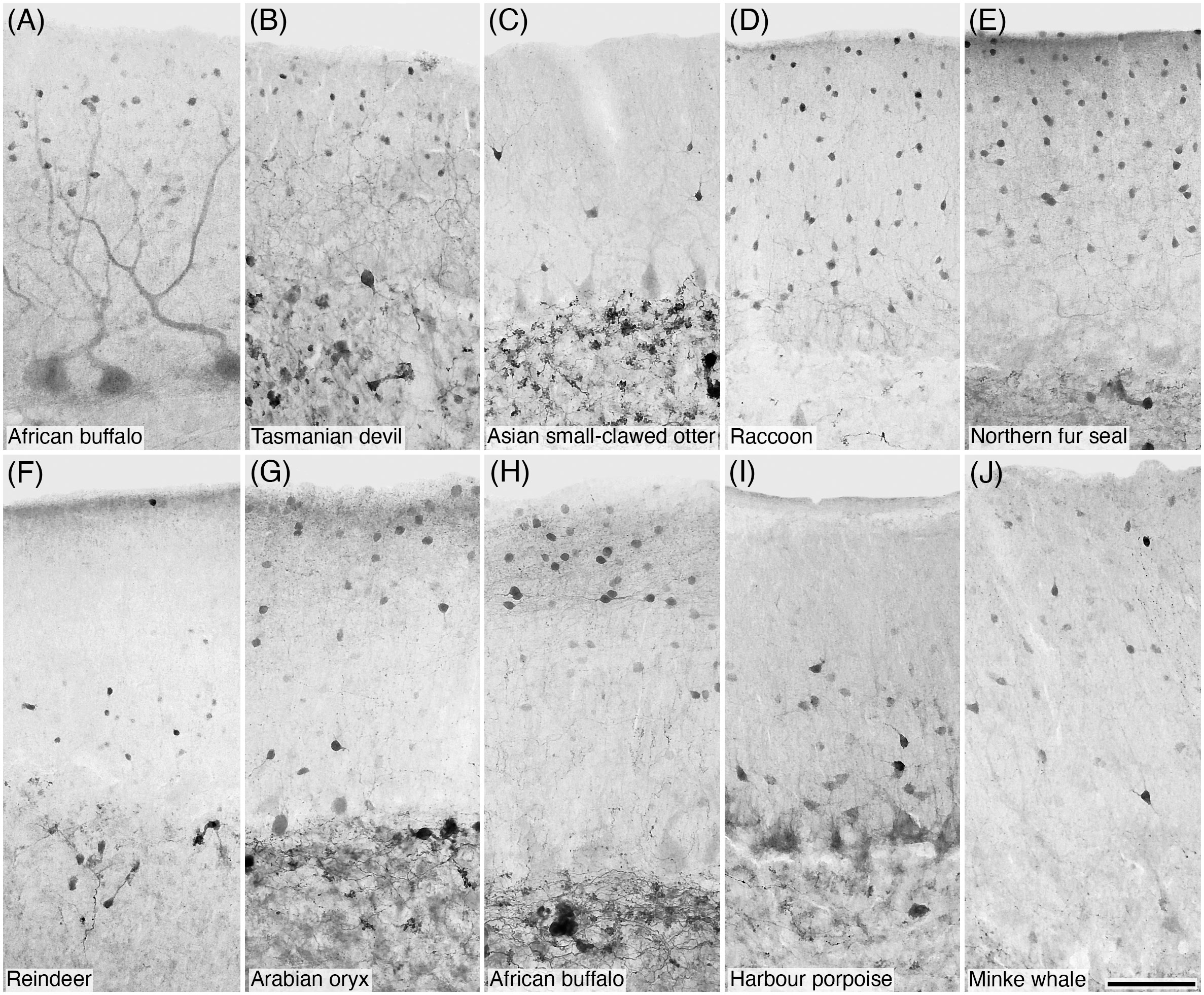
Figure 3 Photomicrographs of CB+ (A) and CR+ (B–J) stellate and/or basket cells in the molecular layer of the cerebellar cortex of ten of the species studied: (A) African buffalo; (B) Tasmanian devil; (C) Asian small-clawed otter; (D) Raccoon; (E) Northern fur seal; (F) Reindeer; (G) Arabian oryx; (H) African buffalo; (I) Harbour porpoise; and (J) Minke whale. Scale bar in (J) = 100 µm and applies to all.
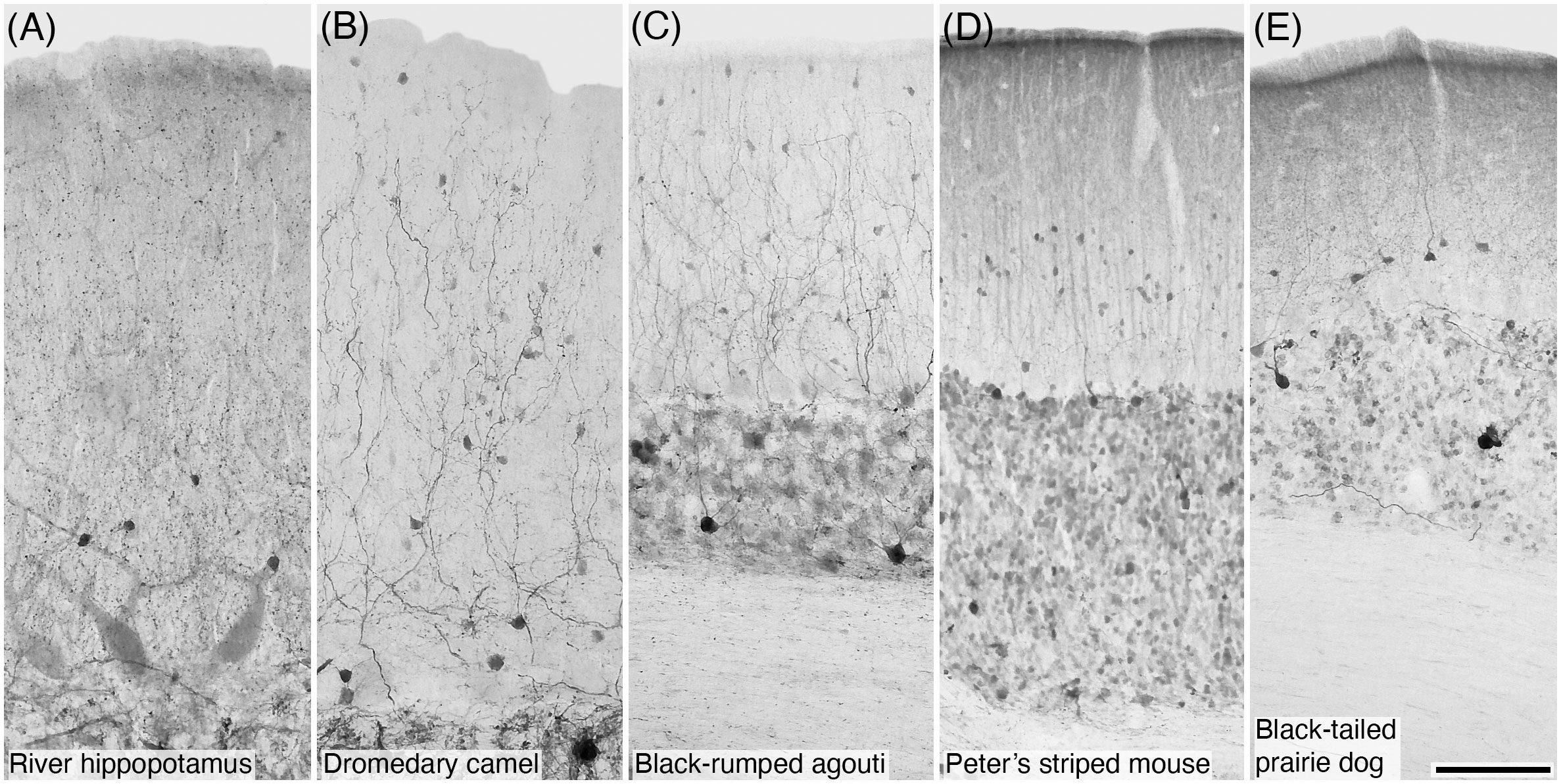
Figure 4 Photomicrographs of CR+ stellate and/or basket cells in the molecular layer of the cerebellar cortex of five of the species studied: (A) River hippopotamus; (B) Dromedary camel; (C) Black-rumped agouti; (D) Peter’s striped mouse; and (E) Black-tailed prairie dog. Scale bar in (E) = 100 µm and applies to all.
3.3 Basket cells
Basket cells were observed in the lower part of the molecular layer of the cerebellar cortex in all species studied (Figure 1), and these cells exhibited similarly shaped soma, although the immunostains used in the current study did not reveal the dendrites. In most of the species the basket cells were PV+ (Figure 3; Table 2). PV- basket cells were noted in the closely related Cetartiodactyla subfamilies Hippotraginae, Alcelaphinae, and Caprinae. PV- basket cells were also noted in the short-beaked echidna, the African buffalo, the minke whale, the Cape hare, and the two-toed sloth (Table 2). The majority of examined species revealed that the basket cells were CB-, although CB+ basket cells were reported for the human (Flace et al., 2014) and the African elephant (Maseko et al., 2013a; Table 3). In most of the species examined the basket cells were CR- (Table 4), although CR+ basket cells were noted for the closely related Mustelidae and Procyonidae families within the Carnivora, as well as the closely related family Hippopotamidae and infraorder Cetacea (Figures 3B–J, 4A; Table 4). CR+ basket cells were also noted in the large-eared slit-face bat, the Northern fur seal, the tree pangolin (Imam et al., 2019), the reindeer, the greater kudu, the domestic pig, the dromedary camel, the black-rumped agouti, Peter’s striped mouse, and the black-tailed prairie dog (Figures 3B–J, 4B–E; Table 4).
3.4 Parallel fibres
The axons of the granule cells ascend to form the distinct parallel fibres within the molecular layer of the cerebellar cortex. In the species studied, these parallel fibres were most clearly observed in the deeper portions of the molecular layer (Figure 5), although they were found throughout the molecular layer. Across species the majority exhibited parallel fibres that were PV+ (Table 2; Figures 5A–J). PV- parallel fibres were noted in the marsupials, Erinaceidae, microchiropterans, megachiropterans, Pilosa, Cetartiodactyla, Rodentia, and Primate lineages (Table 2; Rogers, 1989; Celio, 1990; Résibois and Rogers, 1992; Kalininchenko and Pushchin, 2008). PV- parallel fibres were also noted in the short-beaked echidna (Ashwell et al., 2007), the greater forest shrew, the greater Malayan chevrotain, and the Cape hare (Table 2). In all species investigated, apart from the human (Flace et al., 2014), the parallel fibres were CB- (Table 3). The majority of species revealed parallel fibres that were CR- (Table 3), but CR+ parallel fibres were noted in the Northern fur seal, African buffalo (Table 3; Figures 5K, L), and the Norway rat (Rogers, 1989; Celio, 1990; Résibois and Rogers, 1992).
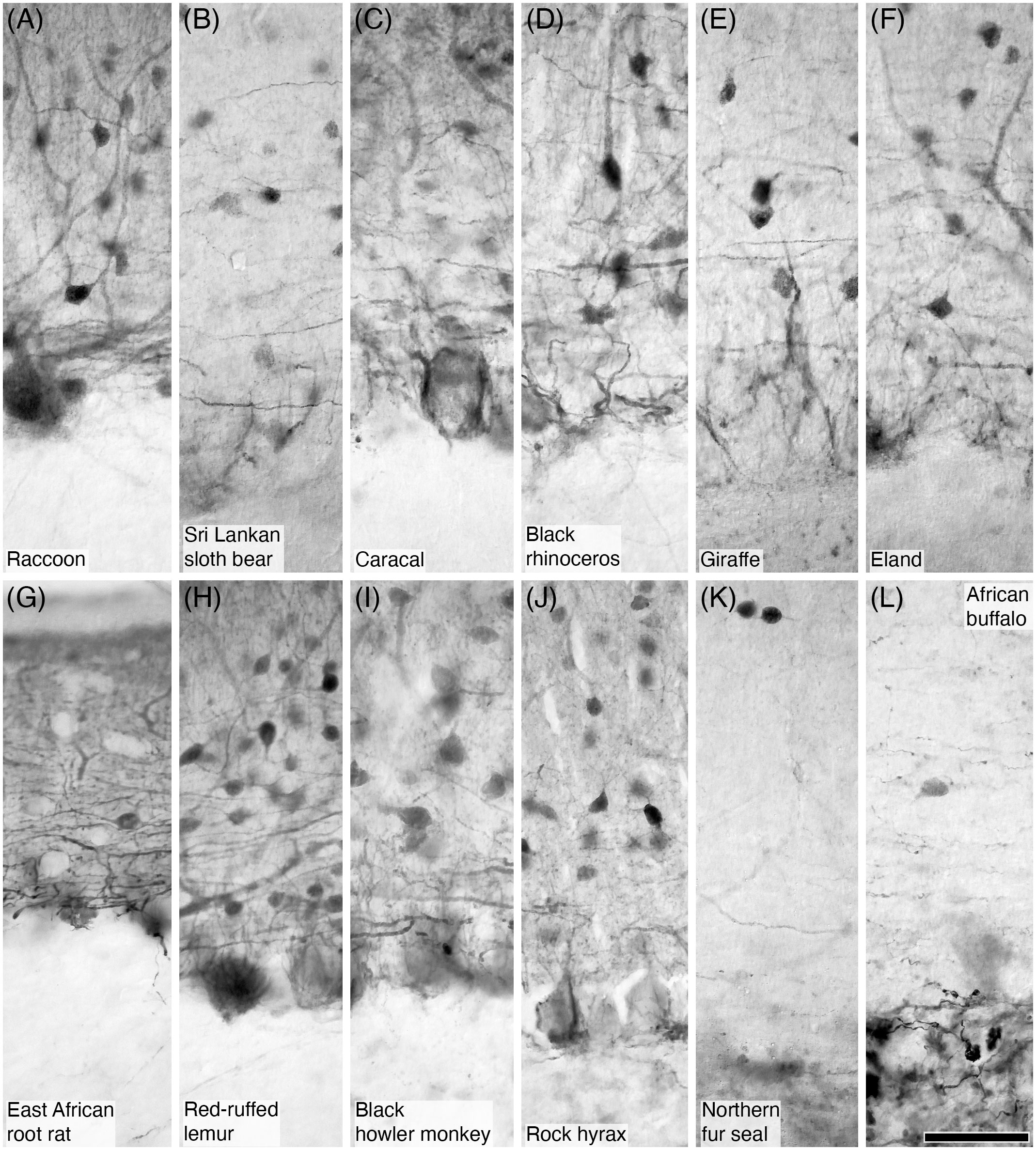
Figure 5 Photomicrographs of PV+ (A–J) and CR+ (K, L) parallel fibres in the molecular layer of the cerebellar cortex in twelve of the species studied. (A) Raccoon; (B) Sri Lankan sloth bear; (C) Caracal; (D) Black rhinoceros; (E) Giraffe; (F) Eland; (G) East African root rat; (H) Red-ruffed lemur; (I) Black howler monkey; (J) Rock hyrax; (K) Northern fur seal; and (L) African buffalo. In all species immunopositive parallel fibres were observed in the molecular layer, especially in the deeper aspects of this layer immediately superficial to the Purkinje cell layer. These parallel fibres exhibited the typical appearance of this neural structure. Scale bar in (L) = 50 µm and applies to all.
3.5 Climbing fibres
Climbing fibres were observed in all species, and these formed intricate branched patterns around the Purkinje cell bodies and dendrites in the molecular layer of the cerebellar cortex. These ascending axons presented with a ‘beaded’ appearance, with the beads presumably representing boutons at which chemical synapses were formed with the Purkinje cells and other neuronal targets (Figure 6). In all species the climbing fibres were PV- (Table 2), and most had climbing fibres that were CB-, apart from CB+ climbing fibres noted in the short-beaked echidna (Ashwell et al., 2007), tree pangolin (Imam et al., 2019), human (Flace et al., 2014), and the African elephant (Maseko et al., 2013a) (Table 3). In contrast, most species revealed CR+ climbing fibres (Figure 6), although CR- climbing fibres were noted for the Pilosa, Afrosoricida, and the Hominoidea lineages (Table 4). CR- climbing fibres were noted for the Arabian oryx, African buffalo, tree pangolin (Imam et al., 2019), several species of rodents and primates, as well as the rock hyrax (Table 4).
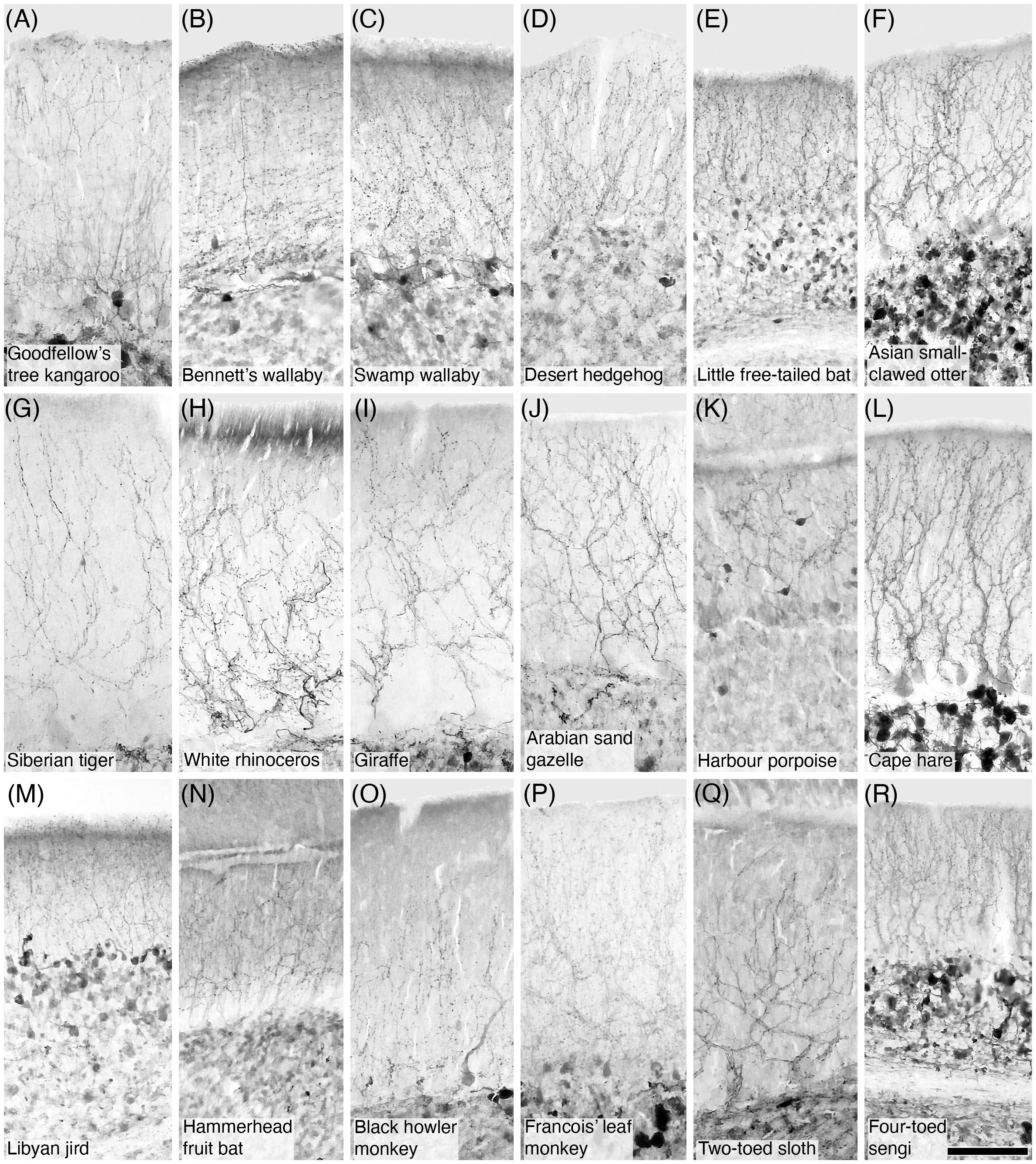
Figure 6 Photomicrographs of CR+ climbing fibres in the molecular layer of the cerebellar cortex in eighteen of the species studied. (A) Goodfellow’s tree kangaroo; (B) Bennett’s wallaby; (C) Swamp wallaby; (D) Desert hedgehog; (E) Little free-tailed bat; (F) Asian small-clawed otter; (G) Siberian tiger; (H) White rhinoceros; (I) Giraffe; (J) Arabian sand gazelle; (K) Harbour porpoise; (L) Cape hare; (M) Libyan jird; (N) Hammerhead fruit bat; (O) Black howler monkey; (P) Francois’ leaf monkey; (Q) Two-toed sloth; (R) Four-toed sengi. In all species studied calretinin-immunopositive climbing fibres were observed in the molecular layer. These climbing fibres exhibited the typical ‘beaded’ appearance of this neural structure Scale bar in (R) = 100 µm and applies to all.
3.6 Purkinje cells
Purkinje cells were observed in all species studied, forming the typical monolayer of these neurons between the inner border of the molecular layer and the outer border of the granular cell layer (Figures 1, 7, 8). In all species studied the Purkinje cells presented with relatively large somata from which dendrites emerged on the pial aspect to ramify extensively and invest into the molecular layer. Immunostaining revealed that in most species Purkinje cells were PV+ (Figure 7; Table 2); however, there were several species with PV- Purkinje cells. PV- Purkinje cells were noted in the Orders Erinaceidae and Soricidae, the family Ursidae within the Carnivora, and the subfamilies Hippotraginae, Alcelaphinae, and Caprinae within the Cetartiodactyla, as well as in in two rodents, the Cape porcupine and Wagner’s gerbil (Table 2). Across all species investigated, the Purkinje cells were CB+, one of the few instances of complete similarity noted in the current study (Figure 8; Table 3). In contrast, most species investigated revealed CR- Purkinje cells (Table 4); however, CR+ Purkinje cells were noted in the red slender loris, although the staining appeared limited to the soma (Figure 8; Table 4).
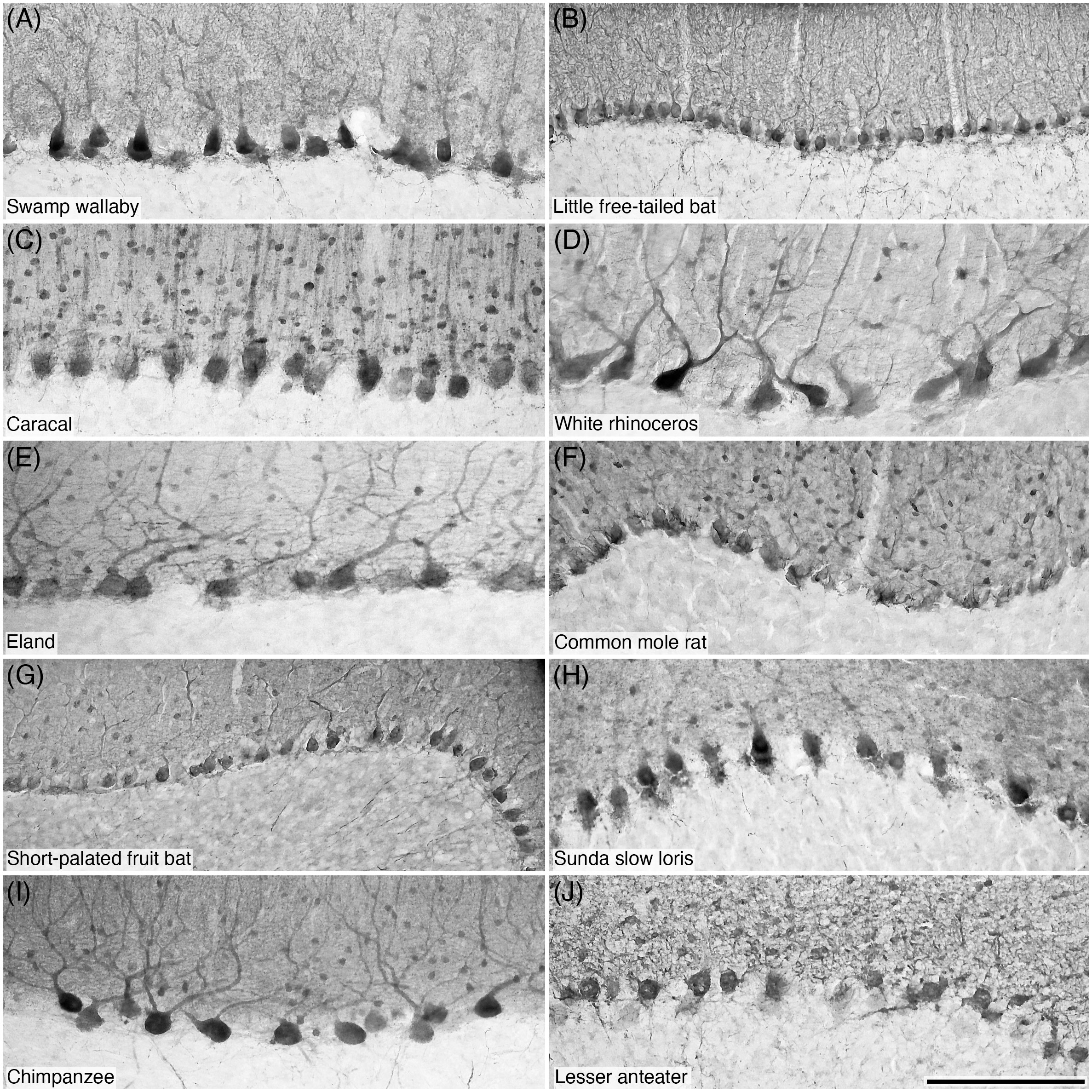
Figure 7 Photomicrographs of PV+ Purkinje cells in the Purkinje cell layer of the cerebellar cortex of ten of the species studied: (A) Swamp wallaby; (B) Little free-tailed bat; (C) Caracal; (D) White rhinoceros; (E) Eland; (F) Common mole rat; (G) Short-palated fruit bat; (H) Sunda slow loris; (I) Chimpanzee; and (J) Lesser anteater. Note that PV immunostaining reveals the structure typical of these neurons. Scale bar in (J) = 200 µm and applies to all.
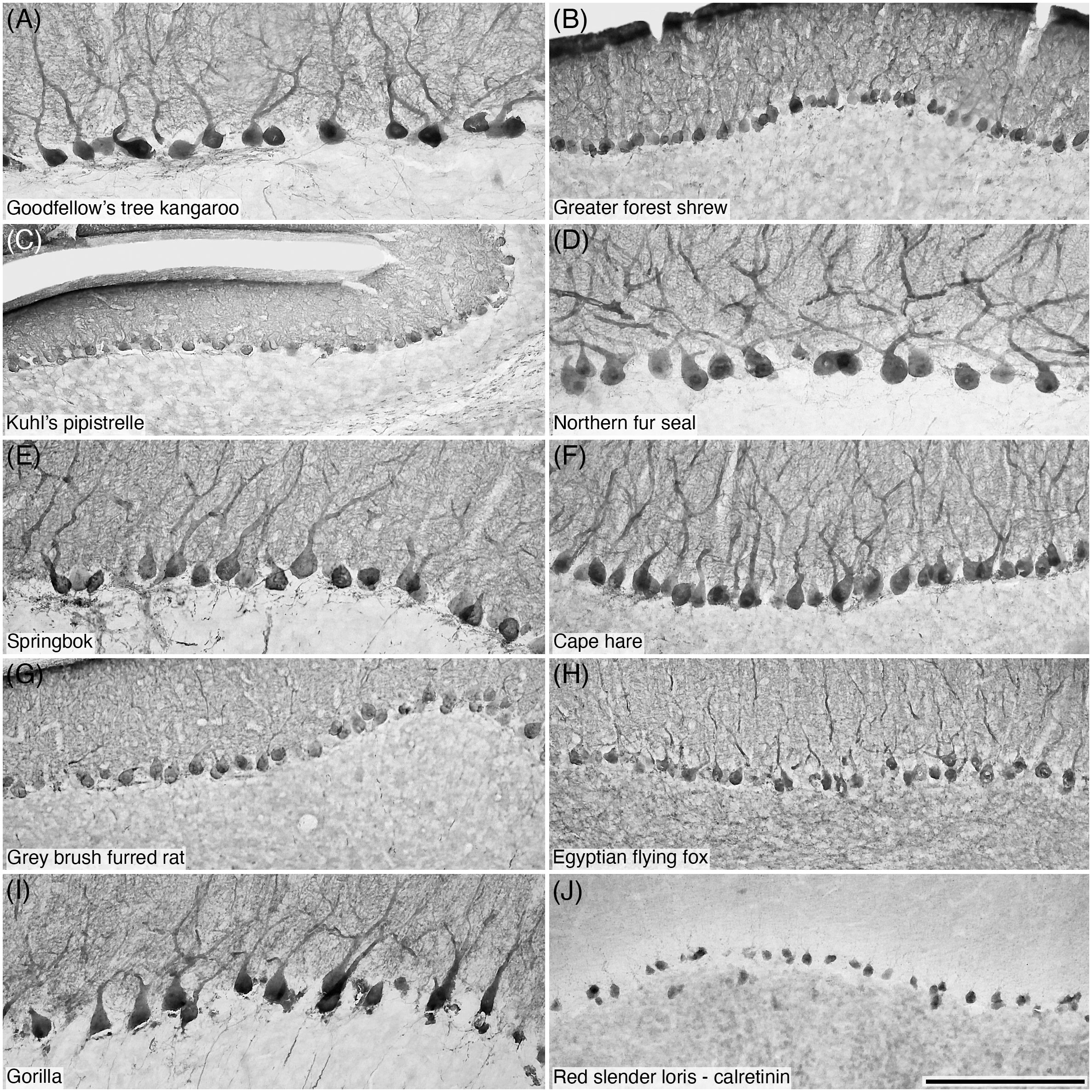
Figure 8 Photomicrographs of CB+ Purkinje cells (A–I) and CR+ Purkinje cells (J) in the Purkinje cell layer of the cerebellar cortex of ten of the species studied: (A) Goodfellow’s tree kangaroo; (B) Greater forest shrew; (C) Kuhl’s pipistrelle; (D) Northern fur seal; (E) Springbok; (F) Cape hare; (G) Grey brush furred rat; (H) Egyptian flying fox; (I) Gorilla; and (J) Red slender loris. Note that CB immunostaining reveals the structure typical of these neurons (A-I), while the sole species in which CR immunostaining was observed in Purkinje cells, the Red slender loris (J), only the soma exhibits immunopositivity. Scale bar in (J) = 200 µm and applies to all.
3.7 Granule cells
In all species a distinct granular cell layer formed the deepest layer of the cerebellar cortex, the neurons comprising this layer being primarily granule cells (Figure 1). The soma of the granule cells across species were generally quite small and spherical in shape (Figure 9). In the species studied, no evidence for PV+ granule cells was noted (Table 2); however, CB+ granule cells were noted in the human (Flace et al., 2014) (Table 3). In contrast, most species evinced granule cells that were CR+ (Figure 10; Table 4), although it should be noted that this CR+ staining was not particularly crisp in its appearance, with the granule cells as a whole exhibiting rather “blurred” specificity in the staining of individual neurons, perhaps due to the high density of granule cells. CR- granule cells were noted in the short-beaked echidna (Ashwell et al., 2007) and the African elephant (Maseko et al., 2013a) (Table 4).
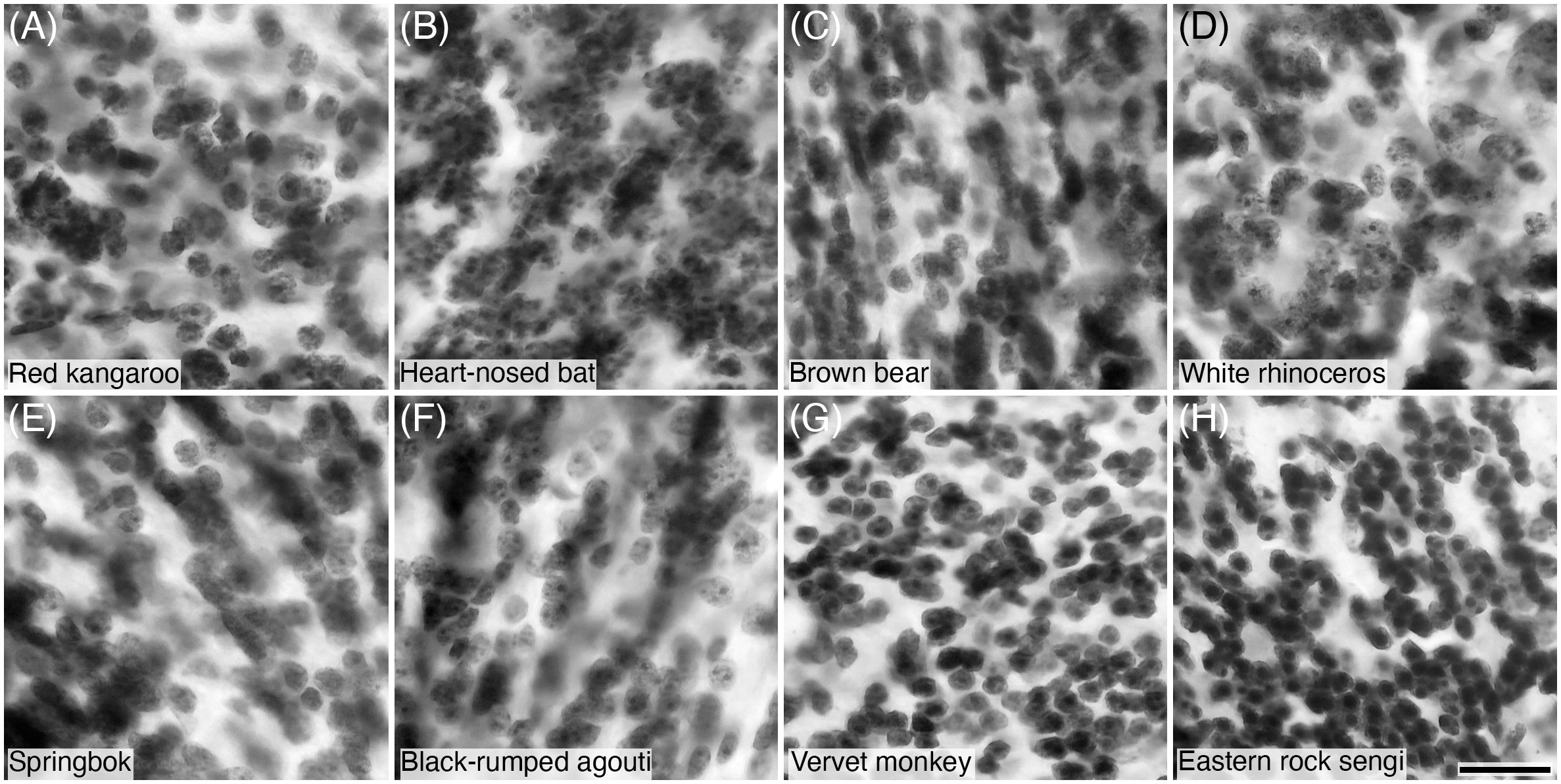
Figure 9 Photomicrographs of Nissl-stained granule cells in the granular cell layer of the cerebellar cortex of eight of the species studied: (A) Red kangaroo; (B) Heart-nosed bat; (C) Brown bear; (D) White rhinoceros; (E) Springbok; (F) Black-rumped agouti; (G) Vervet monkey; and (H) Eastern rock sengi. Note that all granule cells show a distinct spherical shaped soma. Scale bar in (H) = 20 µm and applies to all.
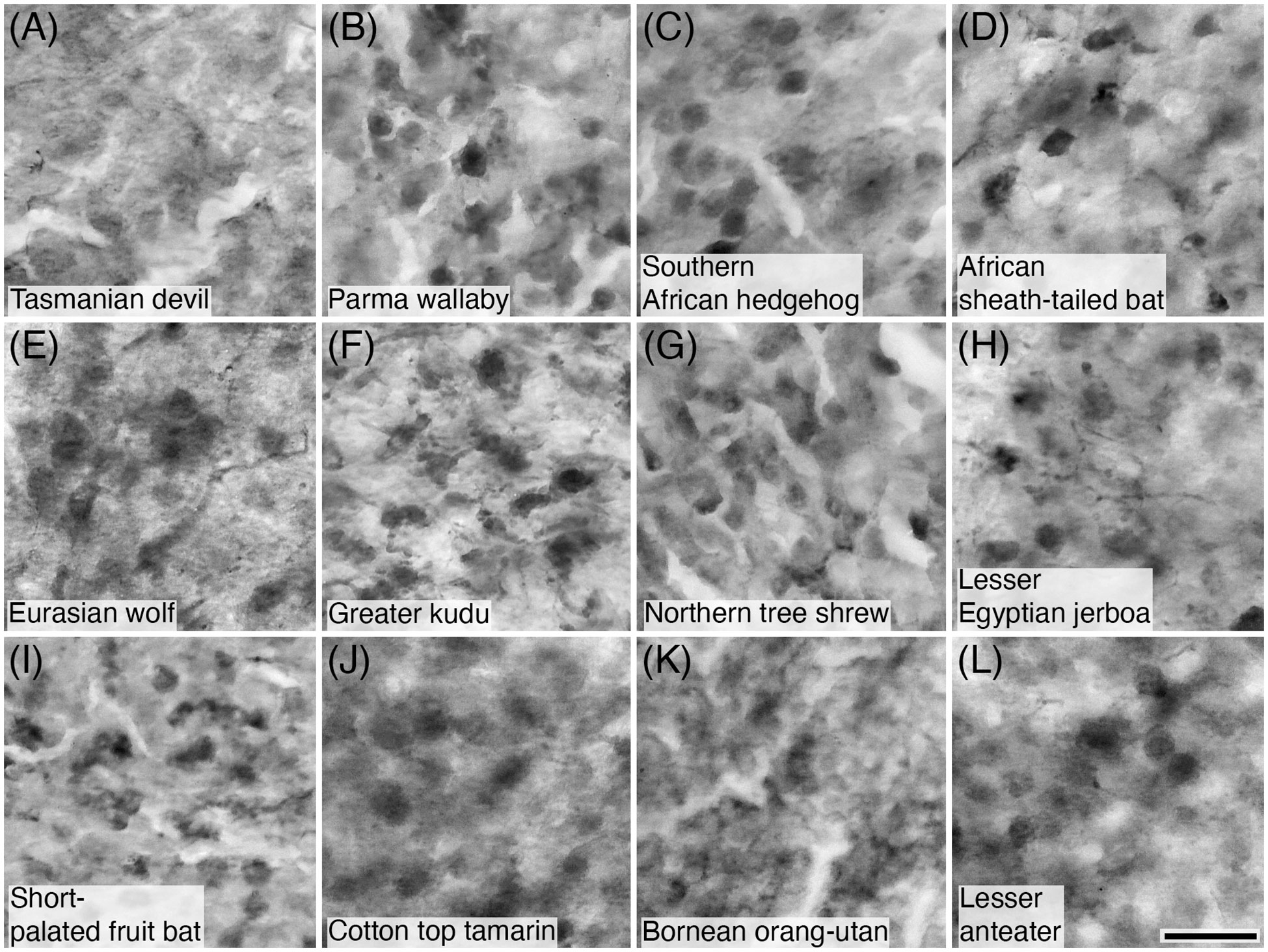
Figure 10 Photomicrographs of CR+ granule cells in the granular cell layer of the cerebellar cortex of twelve of the species studied: (A) Tasmanian devil; (B) Parma wallaby; (C) Southern African hedgehog; (D) African sheath-tailed bat; (E) Eurasian wolf; (F) Greater kudu; (G) Northern tree shrew; (H) Lesser Egyptian jerboa; (I) Short-palated fruit bat; (J) Lesser anteater; (K) Bornean orang-utan; and (L) Lesser anteater. Scale bar in (L) = 20 µm and applies to all.
3.8 Mossy fibres
Mossy fibres, exhibiting the numerous and characteristic varicosities and filipodia typical of these fibres (Palay and Chan-Palay, 1974), were observed in the granule cell layer of all species (Figures 11–13). Mossy fibres in most species studied were PV- (Table 2); however, PV+ mossy fibres were observed in the megachiropterans, in16 of the 24 rodent species studied and in the African buffalo, the Northern tree shrew, and the fat-tailed dwarf lemur (Table 2; Figure 11). CB+ mossy fibres were observed in most species (Table 3; Figure 12), while CB- mossy fibres were noted in the marsupials, Erinaceidae, and within the cetaceans and closely related Cetartiodactyls such as the river hippopotamus, domestic pig, and dromedary camel (Table 2). CR+ mossy fibres were observed in more than half of species studied (Table 4; Figure 13), although CR- mossy fibres were noted in the marsupials, microchiropterans, most carnivores (apart from the European polecat and the Asian small-clawed otter, Figure 13C), perissodactyls, the short-beaked echidna (Ashwell et al., 2007), the greater forest shrew, the tree pangolin (Imam et al., 2019), the giraffe, reindeer, axis deer, scimitar-horned oryx, Arabian oryx, Peter’s striped mouse, the agile monkey, the mandrill, and the African elephant (Maseko et al., 2013a) (Table 4).
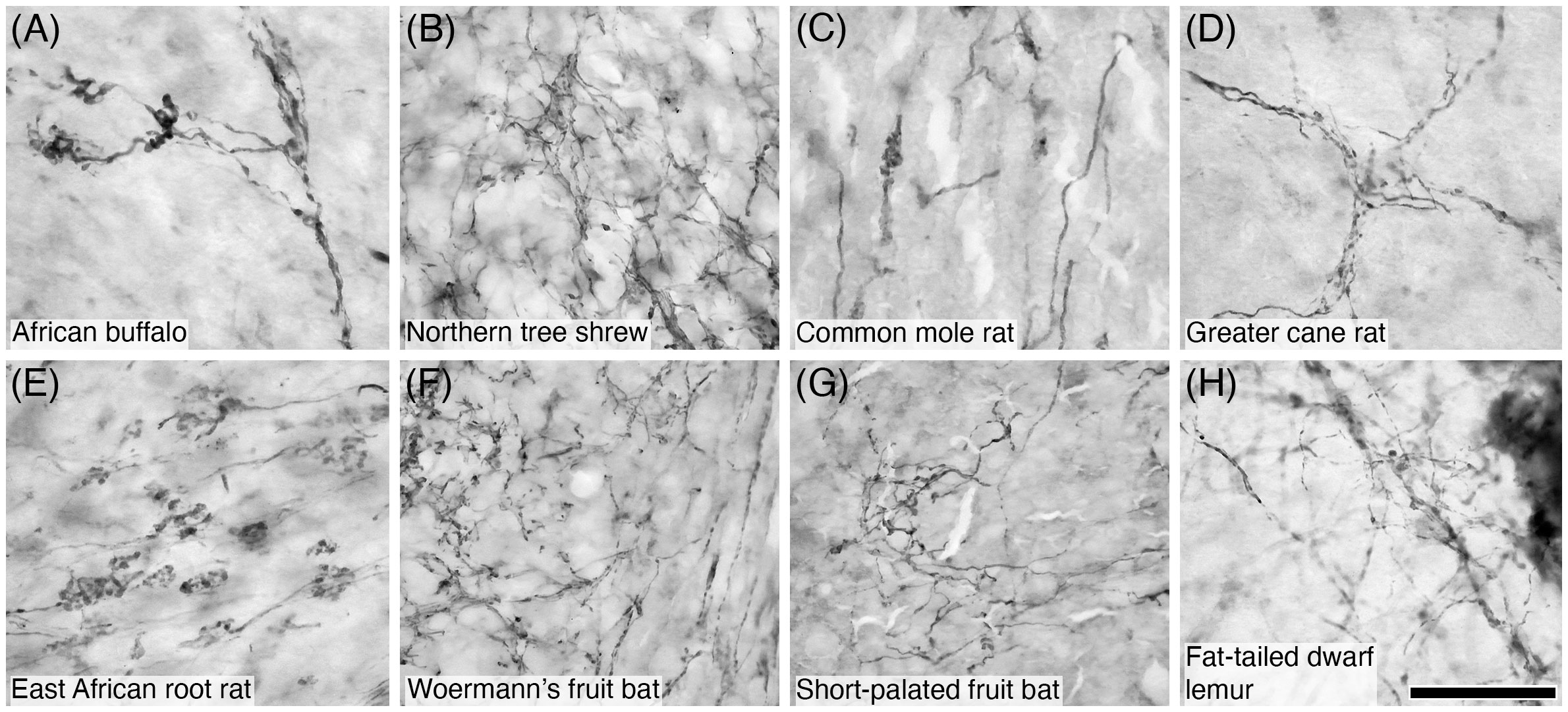
Figure 11 Photomicrographs of PV+ mossy fibres in the granular layer of the cerebellar cortex of eight of the species studied: (A) African buffalo; (B) Northern tree shrew; (C) Common mole rat; (D) Greater cane rat; (E) East African root rat; (F) Woermann’s fruit bat; (G) Short-palated fruit bat; (H) Fat-tailed dwarf lemur. Note the presence of the numerous varicosities and filipodia typically associated with mossy fibres. Scale bar in (H) = 50 µm and applies to all.
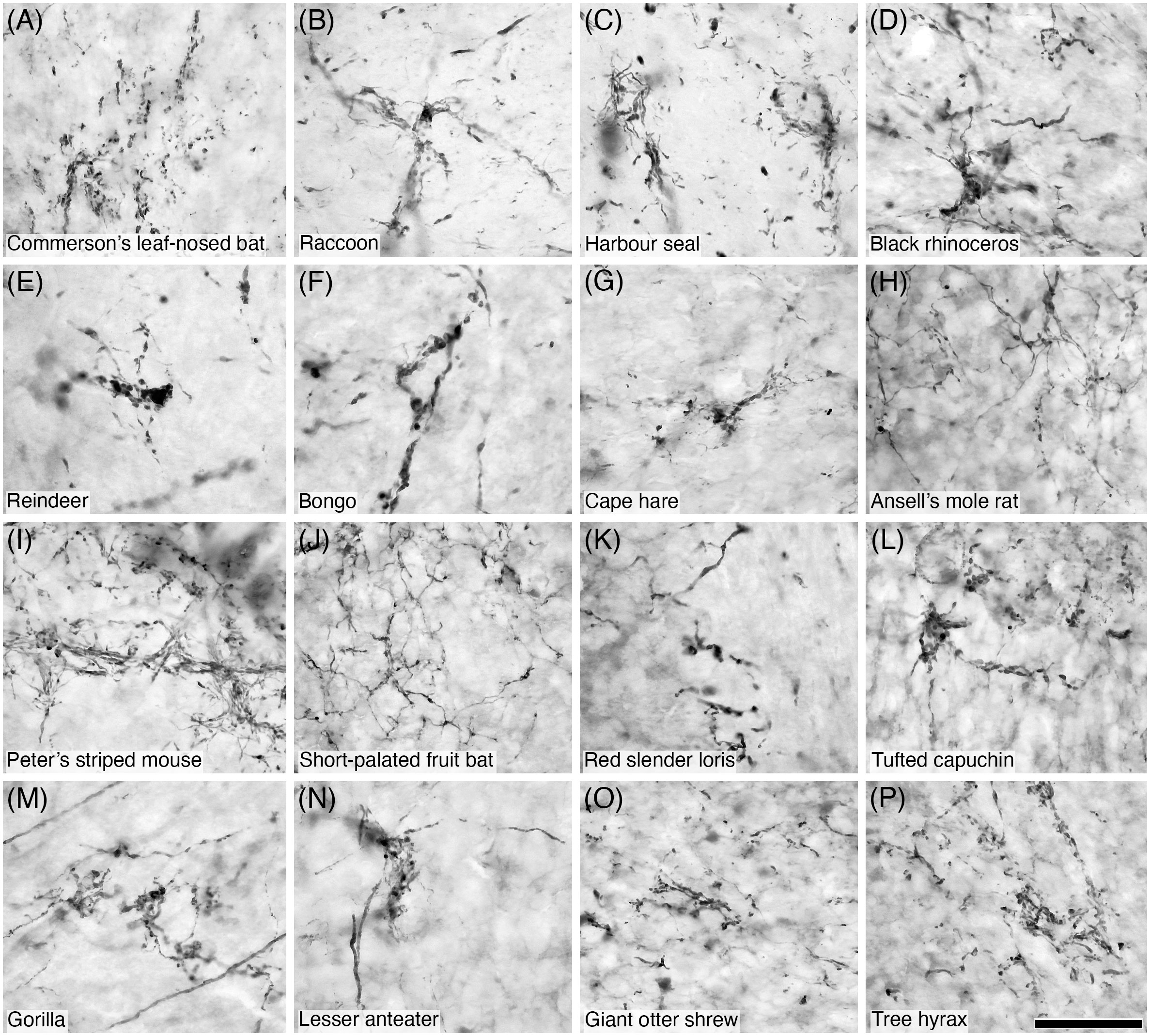
Figure 12 Photomicrographs of CB+ mossy fibres in the granular layer of the cerebellar cortex of sixteen of the species studied: (A) Commerson’s leaf-nosed bat; (B) Raccoon; (C) Harbour seal; (D) Black rhinoceros; (E) Reindeer; (F) Bongo; (G) Cape hare; (H) Ansell’s mole rat; (I) Peter’s striped mouse; (J) Short-palated fruit bat; (K) Red slender loris; (L) Tufted capuchin; (M) Gorilla; (N) Lesser anteater; (O) Giant otter shrew; (P) Tree hyrax. Note the presence of the numerous varicosities and filipodia typically associated with mossy fibres. Scale bar in (P) = 50 µm and applies to all.
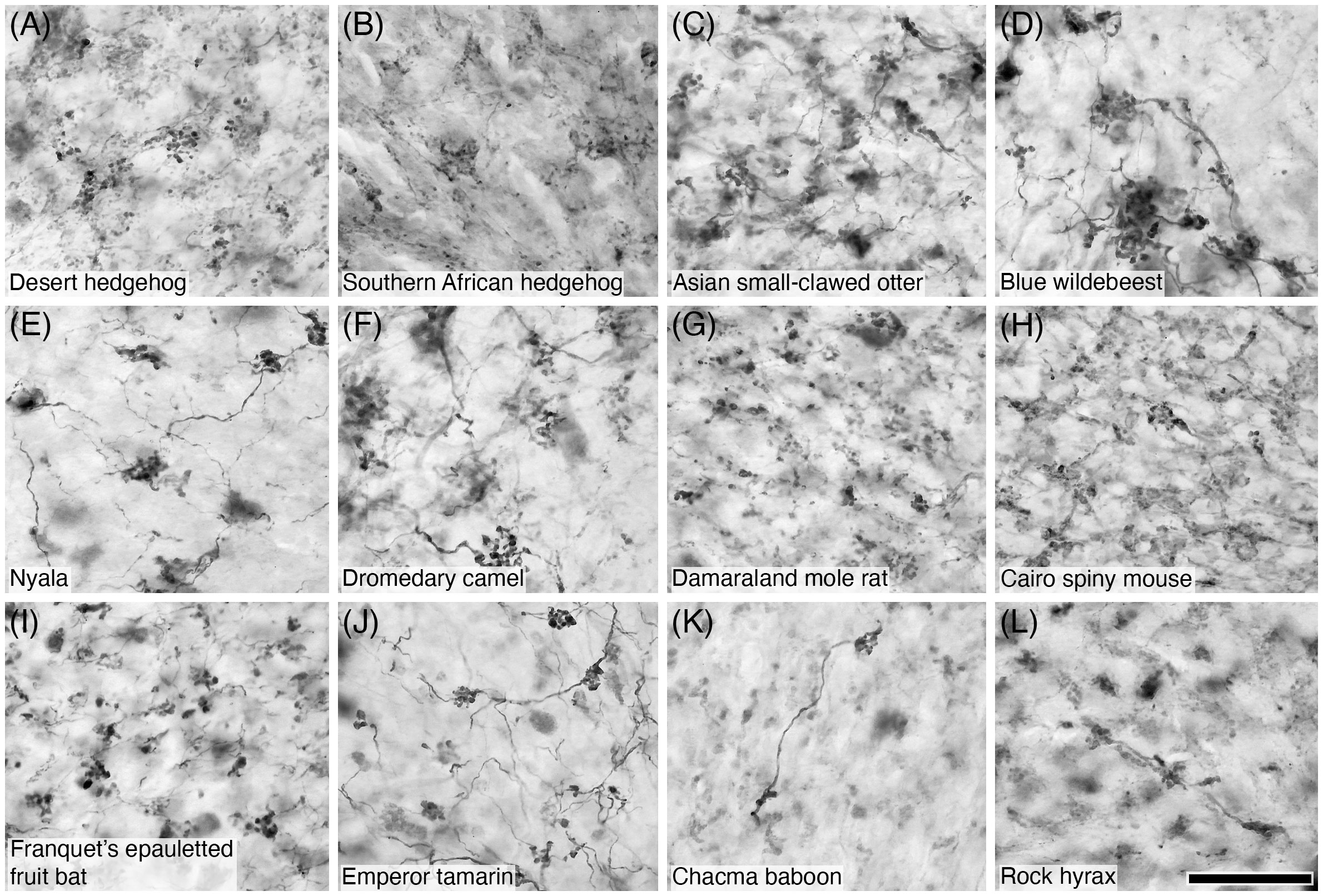
Figure 13 Photomicrographs of CR+ mossy fibres in the granular layer of the cerebellar cortex of sixteen of the species studied: (A) Desert hedgehog; (B) Southern African hedgehog; (C) Asian small-clawed otter; (D) Blue wildebeest; (E) Nyala; (F) Dromedary camel; (G) Damaraland mole rat; (H) Cairo spiny mouse; (I) Franquet’s epauletted fruit bat; (J) Emperor tamarin; (K) Chacma baboon; (L) Rock hyrax. Note the presence of the numerous varicosities and filipodia typically associated with mossy fibres. Scale bar in (L) = 50 µm and applies to all.
3.9 Golgi type-II cells
Golgi type-II cells were located in the granular layer of the cerebellar cortex in all species (Tables 2–4). The immunostaining used in the current study revealed the soma, primary dendrites, and secondary dendritic branches of these neurons (Figures 14–16). This appearance was similar across all species, although distinct differences in the soma and dendritic sizes are evident between species (Figures 14–16). Golgi type-II cells were most commonly PV- (Table 2); however, PV+ Golgi type-II cells were observed in the microchiropterans, the King and Libyan jirds, the related tamarin monkeys, pygmy marmoset, tufted capuchin, and squirrel monkey, bottlenose dolphin (Kalininchenko and Pushchin, 2008), Cape hare, and human (Satoh et al., 1991) (Figure 14; Table 2). Golgi type-II cells were CB- in most mammals studied (Table 3), although CB+ Golgi type-II cells were observed in the Scimitar-horned and Arabian oryxes (Figures 15B, C), Goodfellow’s tree kangaroo, the greater kudu, bottlenose dolphin (Kalininchenko and Pushchin, 2008), the Cape porcupine, lesser Egyptian jerboa, Wagner’s gerbil (Figures 15A, D, E, F), and the human (Flace et al., 2014) (Table 3). CR+ Golgi type-II cells were observed in most species studied (Table 3; Figure 15). CR- Golgi type-II cells were noted in the Cetartiodactyla (although they were present in the closely related harbour porpoise, minke whale and river hippopotamus (Figures 16H–J); although not in the bottlenose dolphin, Kalininchenko and Pushchin, 2008), the closely related bathyergid African mole rats and thyronomyid greater cane rat, the megachiropterans, the Strepsirrhini, Cebidae, and Cercopithecinae primate lineages, the Pilosa, and Macroscelidea, as well as the short-beaked echidna (Ashwell et al., 2007) and African elephant (Maseko et al., 2013a) (Table 4).
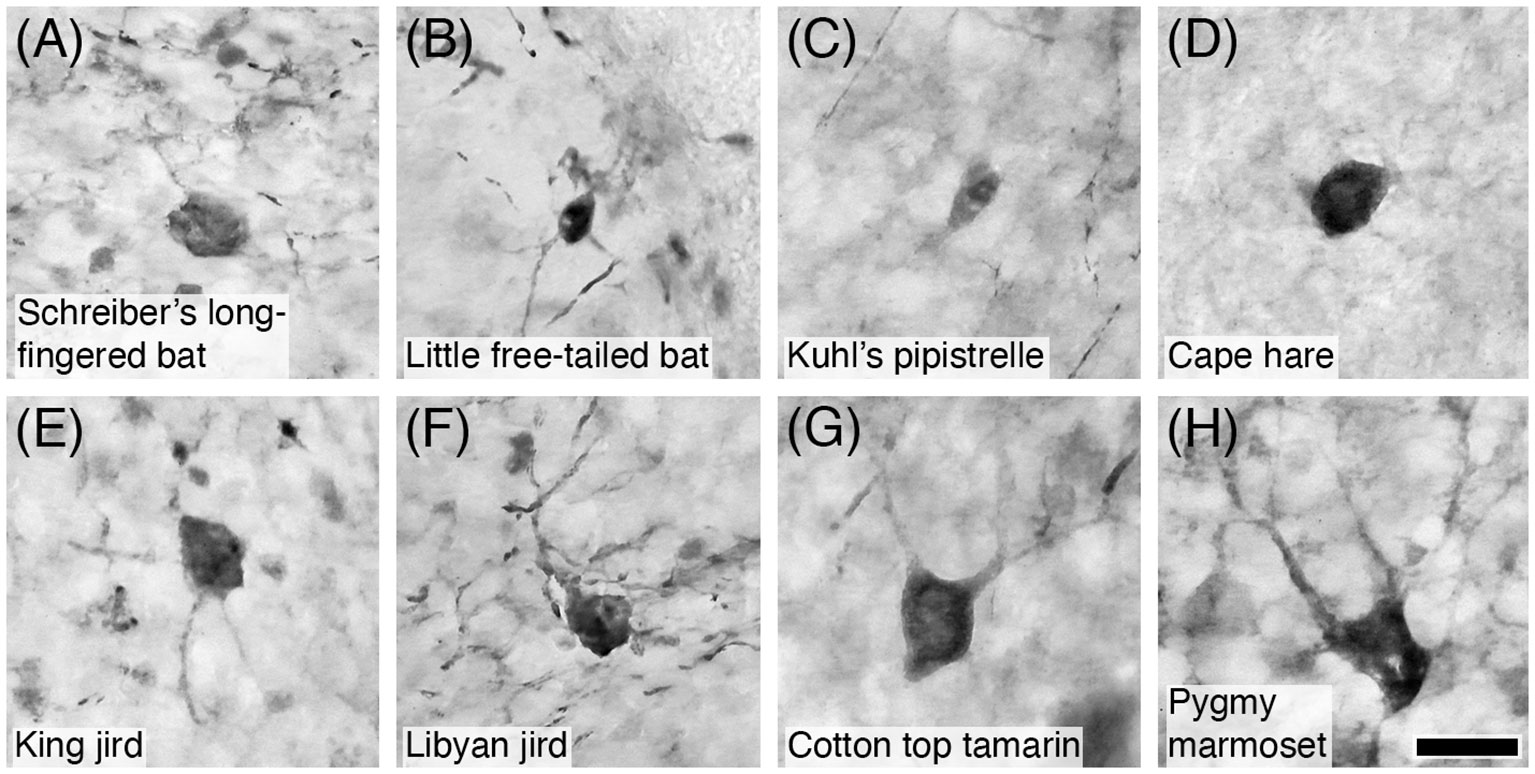
Figure 14 Photomicrographs of PV+ Golgi type-II neurons in the granular layer of the cerebellar cortex of eight of the species studied: (A) Schreiber’s long-fingered bat; (B) Little free-tailed bat; (C) Kuhl’s pipistrelle; (D) Cape hare; (E) King jird; (F) Libyan jird; (G) Cotton top tamarin; and (H) Pygmy marmoset. Note that PV immunostaining reveals the soma and proximal dendrites typical of these neurons. Scale bar in (H) = 20 µm and applies to all.
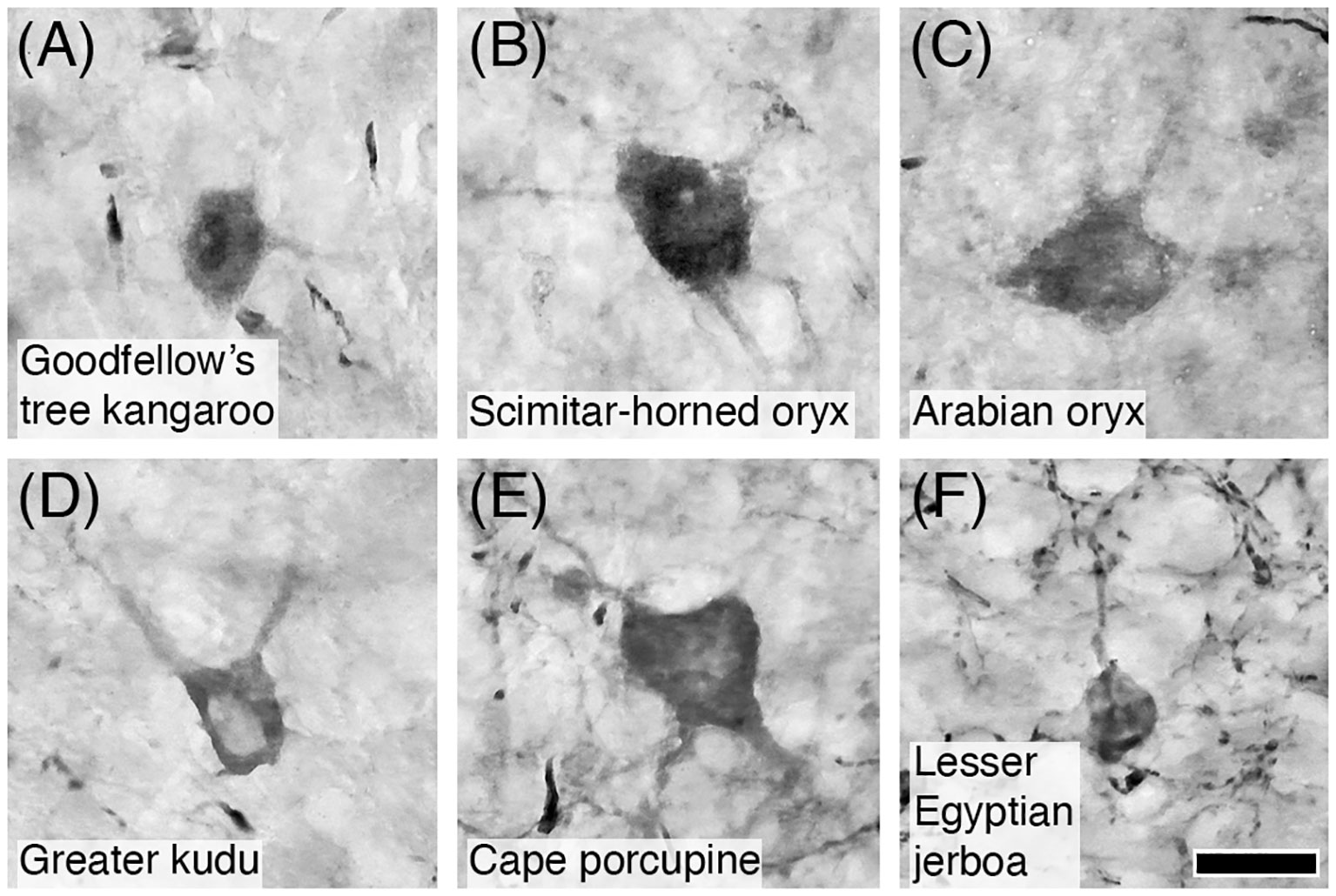
Figure 15 Photomicrographs of CB+ Golgi type-II neurons in the granular layer of the cerebellar cortex of six of the species studied: (A) Goodfellow’s tree kangaroo; (B) Scimitar-horned oryx; (C) Arabian oryx; (D) Greater kudu; (E) Cape porcupine; and (F) Lesser Egyptian jerboa. Note that CB immunostaining reveals the soma and proximal dendrites typical of these neurons. Scale bar in (F) = 20 µm and applies to all.
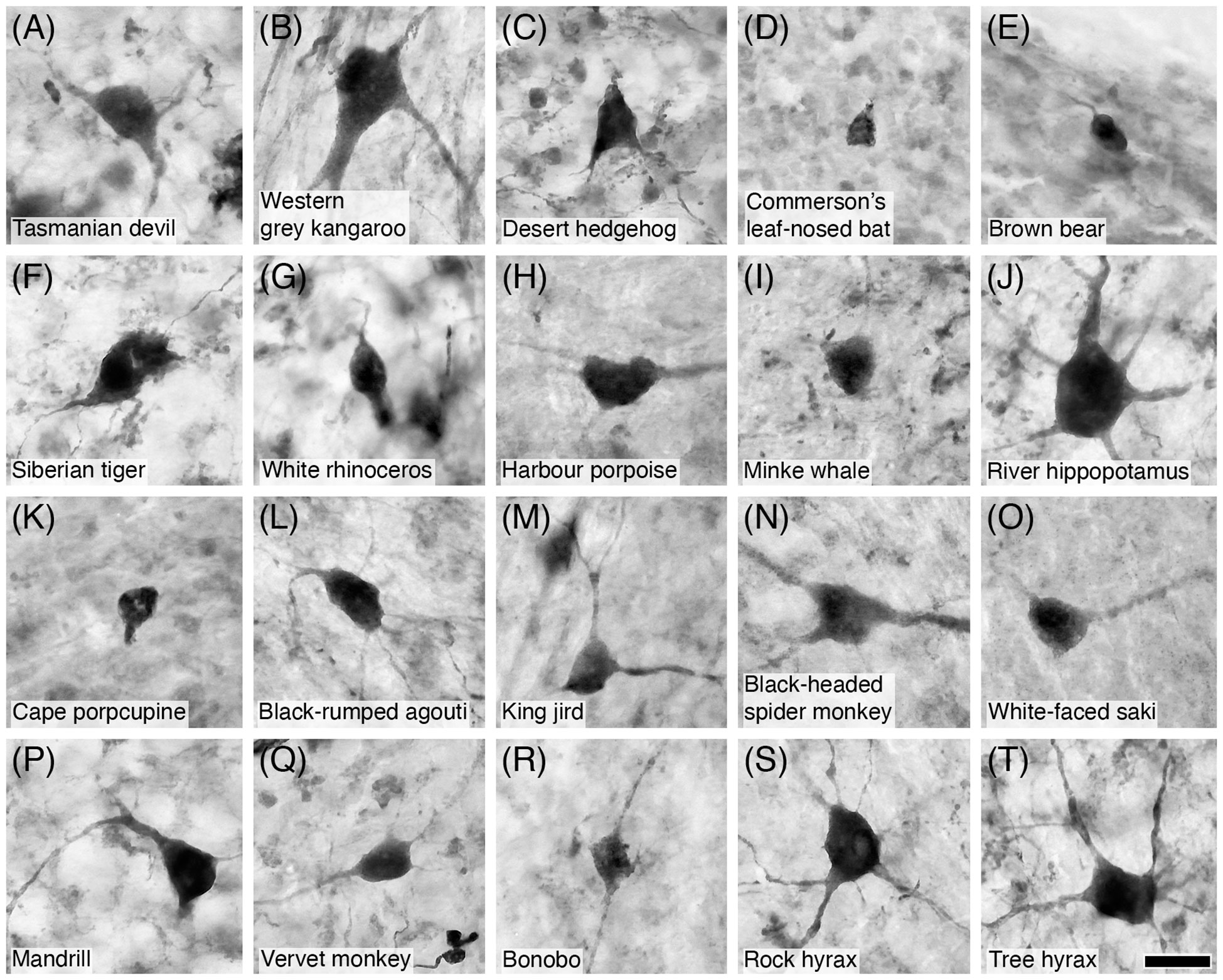
Figure 16 Photomicrographs of CR+ Golgi type-II neurons in the granular layer of the cerebellar cortex of twenty of the species studied: (A) Tasmanian devil; (B) Western grey kangaroo; (C) Desert hedgehog; (D) Commerson’s leaf-nosed bat; (E) Brown bear; (F) Siberian tiger; (G) White rhinoceros; (H) Harbour porpoise; (I) Minke whale; (J) River hippopotamus; (K) Cape porcupine; (L) Black-rumped agouti; (M) King jird; (N) Black-headed spider monkey; (O) White-faced saki; (P) Mandrill; (Q) Vervet monkey; (R) Bonobo; (S) Rock hyrax; and (T) Tree hyrax. Note that CR immunostaining reveals the soma, primary dendrites, and secondary dendritic branches, typical of these neurons. Scale bar in (T) = 20 µm and applies to all.
3.10 Lugaro neurons
Lugaro neurons were observed in the upper granule layer and Purkinje cell layer of the cerebellar cortex in all species studied. These neurons, that have a similar soma area as the Golgi type II neurons, differ in their soma shape, dendritic orientation, and number of primary dendrites (Figure 17). The two primary dendrites of the Lugaro neurons generally run either parallel to, or orthogonal to, the Purkinje cell layer (Figure 17). In the species studied, we did not observe any Lugaro neurons that were PV+ (Table 2). All species, apart from humans (Flace et al., 2014), exhibited Lugaro neurons that were CB- (Table 3). In contrast, all but two species exhibited CR+ Lugaro neurons (Table 3; Figure 17), these two species being the short-beaked echidna (Ashwell et al., 2007) and the bottlenose dolphin (Kalininchenko and Pushchin, 2008).
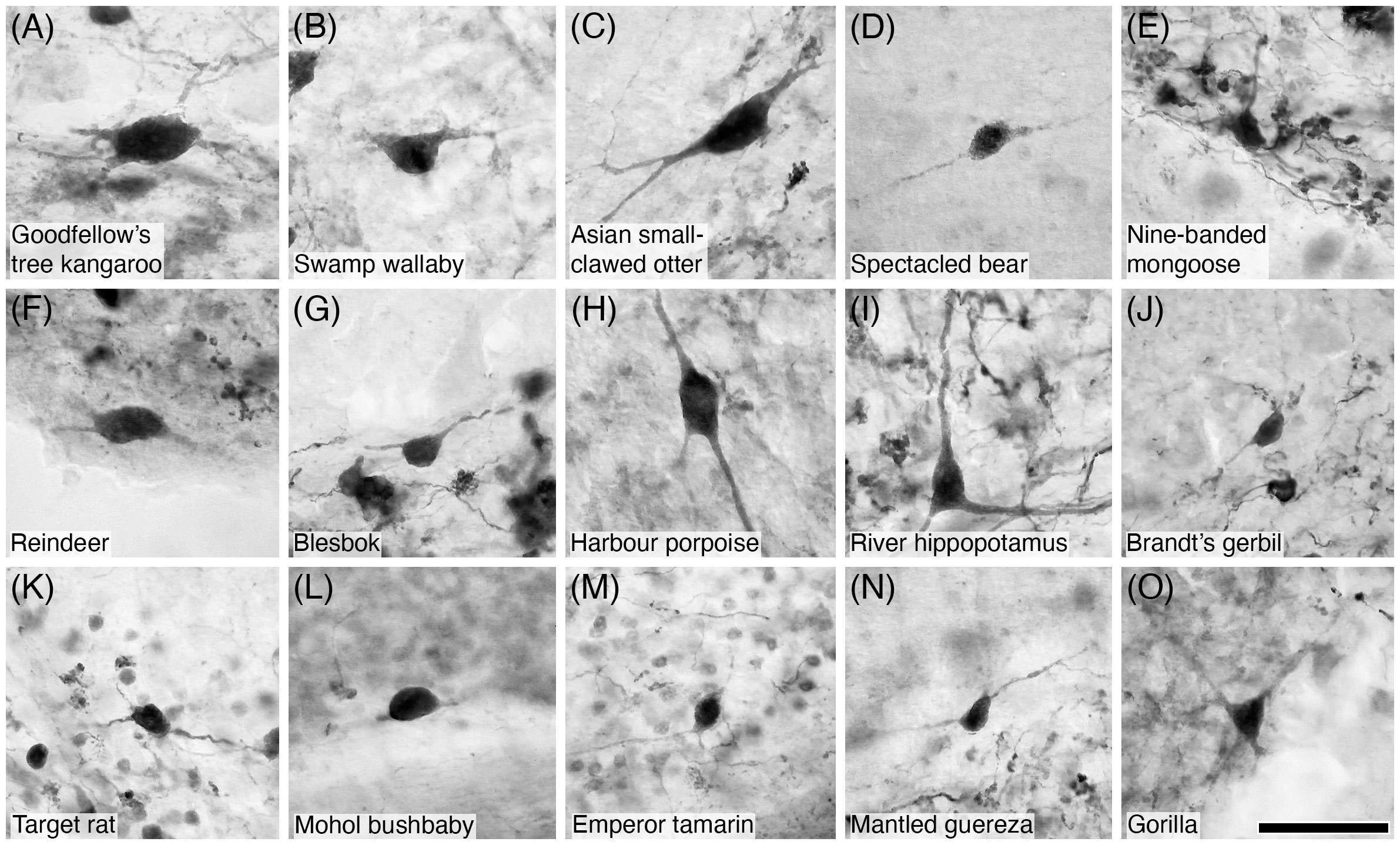
Figure 17 Photomicrographs of CR+ Lugaro neurons in the cerebellar cortex of fifteen of the species studied: (A) Goodfellow’s tree kangaroo; (B) Swamp wallaby; (C) Asian small-clawed otter; (D) Spectacled bear; (E) Nine-banded mongoose; (F) Reindeer; (G) Blesbok; (H) Harbour porpoise; (I) River hippopotamus; (J) Brandt’s gerbil; (K) Target rat; (L) Mohol bushbaby; (M) Emperor tamarin; (N) Mantled guereza; and (O) Gorilla. Note that CR immunostaining reveals the soma, primary dendrites, and sometimes the secondary dendritic branches, typical of Lugaro neurons. Scale bar in (O) = 50 µm and applies to all.
3.11 Unipolar brush neurons
Unipolar brush neurons were observed in the granular cell layer of all species. These neurons exhibited the typical round/oval shaped soma, with a single thick dendrite emerging to project a short distance and then terminate in a brush dendriole (Figure 18). Across the species investigated, unipolar brush cells were mostly PV- (Table 2); however, PV+ unipolar brush neurons were observed in the microchiropteran bats (Table 2; Figure 19). Interestingly, these PV+ microchiropteran unipolar brush neurons were not revealed in their entirety, with the soma and proximal part of the dendrite being stained, but not the brush dendriole characteristic of this neuronal type (Figure 19). In all species investigated the unipolar brush neurons were CB- (Table 3). CR+ unipolar brush neurons were observed in most species (Figure 18), but CR- unipolar brush neurons were noted in the Erinaceidae, Soricidae, microchiropteran bats, species of the Genus Oryx the short-beaked echidna (Ashwell et al., 2007), Goodfellow’s tree kangaroo, the pacarana, the Norway rat, and the African elephant (Maseko et al., 2013a) (Table 4).
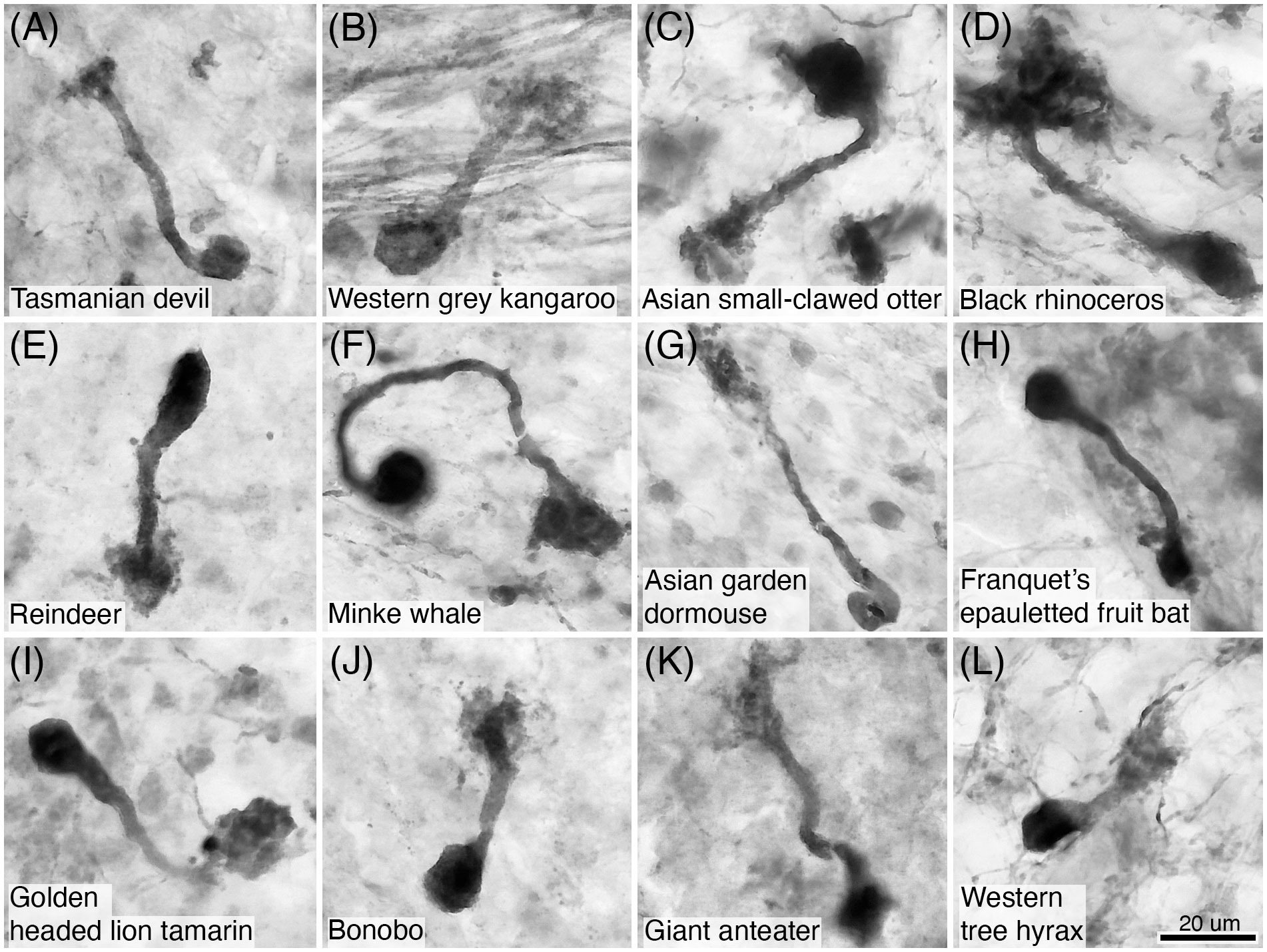
Figure 18 Photomicrographs of CR+ unipolar brush neurons in the granular layer of the cerebellar cortex of twelve of the species studied: (A) Tasmanian devil; (B) Western grey kangaroo; (C) Asian small-clawed otter; (D) Black rhinoceros; (E) Reindeer; (F) Minke whale; (G) Asian garden dormouse; (H) Franquet’s epauletted fruit bat; (I) Golden headed lion tamarin; (J) Bonobo; (K) Giant anteater; and (L) Western tree hyrax. Note that CR immunostaining reveals the soma, dendrite and the brush dendriole typical of these neurons. Scale bar in (L) = 20 µm and applies to all.
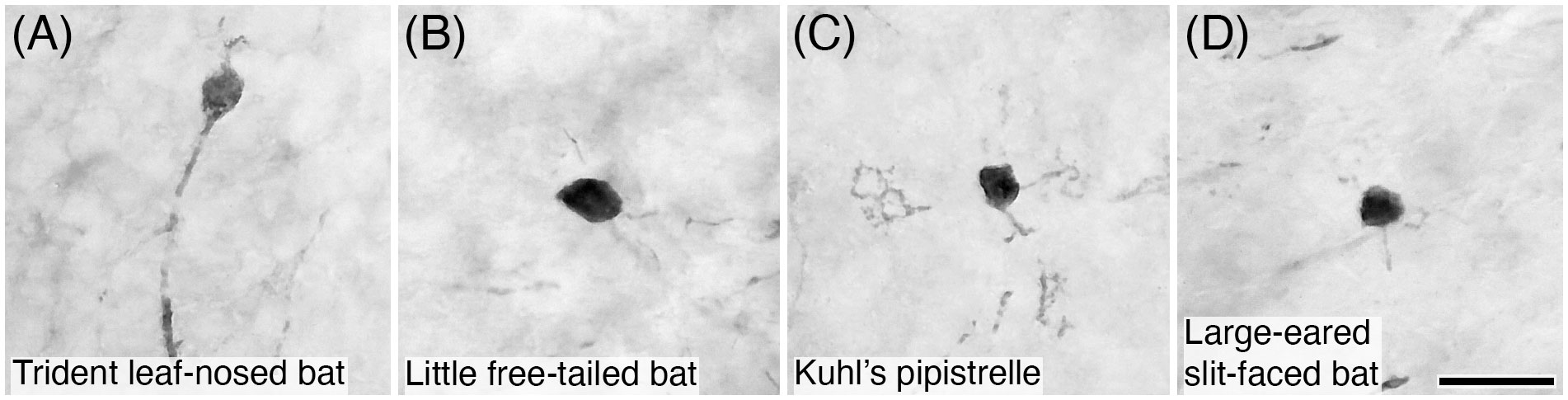
Figure 19 Photomicrographs of PV+ unipolar brush neurons in the granular layer of the cerebellar cortex of four species of microchiropteran bats: (A) Trident leaf-nosed bat; (B) Little free-tailed bat; (C) Kuhl’s pipistrelle; and (D) Large-eared slit-faced bat. Note that PV immunostaining reveals the soma and the dendrite, but not the brush dendriole typical of these neurons. Scale bar in (D) = 20 µm and applies to all.
4 Discussion
The current study provides over 4000 novel observations related to calcium-binding protein chemoarchitecture in 10 cell types/neural structures forming the circuitry of the cerebellar cortex of 143 mammal species. While there are substantial consistencies in the localization of the three different calcium-binding proteins investigated, there are also significant variations associated with lineages and individual species. The variations noted may be of importance to understanding the motor, perceptual, and cognitive neural processes of different species and how these relate to their life histories and the environments they inhabit.
4.1 Study limitations and potential confounding factors
While the large complement of species investigated herein covers much of the phylogenetic breadth of the mammalian radiation, 143 species accounts for only approximately 2% of known extant mammal species. Clearly it would be of significant value to include more species, as the coverage provided in the current study may miss important variations in the distribution of calcium-binding proteins in the cerebellar cortex. Furthermore, the specimens used in the current study were not all collected with the same methodology. Variations in post-mortem delay, as well as fixation and storage methods, need to be acknowledged. It would be advantageous to have all specimens collected with the same method, but to increase the diversity of species studied, this was not possible. We also acknowledge that the current work was primarily undertaken on a single adult individual of each the species, and thus individual variations have been generalised to the species, which can be problematic. Despite this, in 8 species (Bennett’s wallaby, desert hedgehog, African sheath-tailed bat, European polecat, giraffe, Ansell’s mole rat, Egyptian flying fox, and chimpanzee), two or more individuals were examined, and the results obtained were identical between individuals. Inter-species variability in the conformation of the calcium-binding proteins investigated may prevent antibodies binding to the targeted site on the protein, potentially leading to the recording of false negative results. In addition, the antibodies used for the calcium-binding proteins in the studies cited from the literature may have varied, thus leading to different results. While this is not the case for the tree pangolin (Imam et al., 2019), the African elephant (Maseko et al., 2013a), and the calbindin staining done in humans (Flace et al., 2014), this concern may apply to the parvalbumin antibody used in the short-beaked echidna (Ashwell et al., 2007) and bottlenose dolphin (Kalininchenko and Pushchin, 2008), all three antibodies used in the Norway rat (Rogers, 1989; Celio, 1990; Résibois and Rogers, 1992), and the parvalbumin and calretinin antibodies used in human (Satoh et al., 1991; Braak and Braak, 1993). It is also important to note that at any specific time, even though a neuron may be capable of producing specific calcium-binding proteins, they may not be doing so (e.g., Caballero and Tseng, 2016; Schwaller, 2020). Thus, as the time of death of the animals used in this study it may be the case that a certain neural structure, that can express a specific calcium-binding protein, may not be doing so, and thus lead to a false negative observation. Thus, while we generalise our results, we do so with caution, and temper our conclusions cognizant of these potential confounding factors.
4.2 Is there a common expression pattern of calcium-binding proteins in the mammalian cerebellar cortex?
Understanding whether there is a common pattern of expression of the calcium-binding proteins in the 10 cell types and neural structures of the mammalian cerebellar cortex examined is of importance for understanding cerebellar functions across species, especially in terms of translational studies from laboratory rodents to humans. While for each neural structure there is the most commonly recorded observation (Table 5), it is reasonable to conclude that while there is substantial consistency in the patterns of calcium-binding protein expression in the neural structures examined across species, there is also significant variation that prevents us from describing with certainty what might be termed a definitive “typical” pattern across mammals – for each calcium-binding protein there were variations noted in certain neural structures in certain lineages/species (Tables 2–4). As presented in Table 5, stellate cells, basket cells, and parallel fibres were most frequently found to be PV+, and CB/CR-; climbing fibres were most frequently found to be CR+ and PV/CB-; Purkinje cells were most frequently found to be PV/CB+ and CR-; mossy fibres were most frequently found to be CB/CR+, but PV-; and granule cells, Golgi-type II cells, Lugaro neurons and unipolar brush cells were most frequently found to be CR+ and PV/CB-.
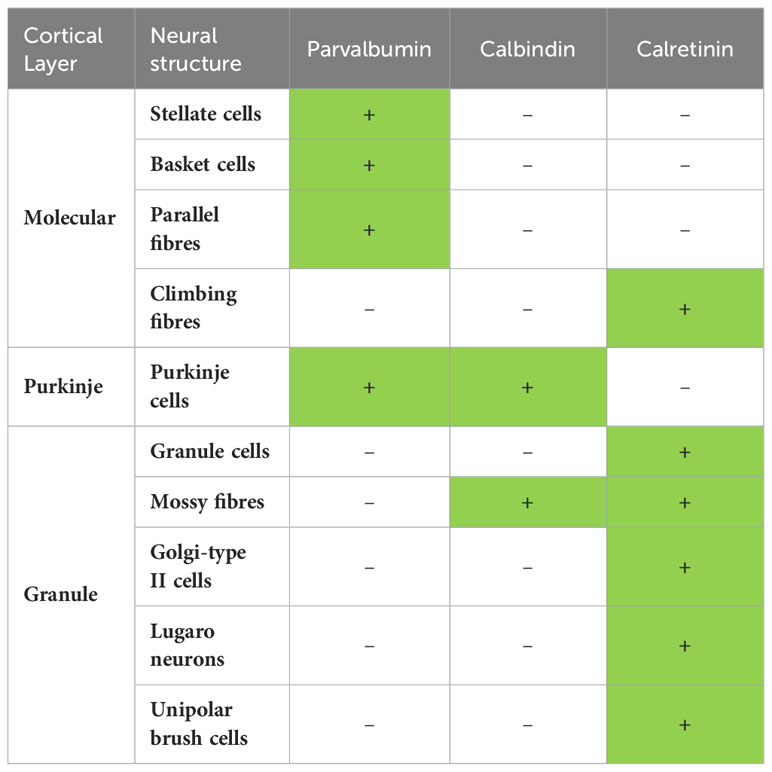
Table 5 Summary of the most frequent occurrence of immunopositivity (+, green fill) and immunonegativity (-, white fill) for parvalbumin, calbindin and calretinin in the 10 neuronal structures examined in the mammalian cerebellar cortices examined in this study.
This summary indicates that most often PV is found in the neural structures of the molecular and Purkinje cell layers, but is most often absent in the granule cell layer. CB is most often found in the neural structures of the Purkinje cell layer and mossy fibres, while CR is most often found in the climbing fibres of the molecular layer and all neural structures of the granule cell layer. But can this generalised pattern of calcium-binding proteins in the neural circuitry of the cerebellar cortex be considered “typically mammalian”? Based on the results of the current study, the answer is both yes and no, depending on the lineage/species and the structure being investigated (Tables 2–4). It is difficult to conclude with certainty that this chemoarchitectural pattern (Table 5) is “typically mammalian” given the variation observed. This generalised pattern of calcium-binding protein chemoarchitecture is likely better considered to be a “guideline”, or more specifically what occurs most often in the mammals investigated in the current study, of what one might expect when examining the mammalian cerebellar cortex for the localisation of the calcium-binding proteins, especially in previously unstudied species, but certainly cannot be considered definitive of mammals in toto.
4.3 Lineage-specific variations and lack of variations
In contrast to the generalisation outlined above, the shared variations that are found within lineages allow for more robust conclusions to be made, especially in lineages where more than one species was investigated; however, it must be borne in mind that these conclusions are based only on the species studied herein and may not apply to those species within a lineage that we have not studied. Here we outline a few, of the many, examples of these shared lineage-specific variations (see Tables 2–4 for a comprehensive record of these variations). PV+ Golgi type II cells and unipolar brush neurons in the microchiropterans, but not most other species (Table 2), distinguishes the chemoarchitecture of the microchiropteran cerebellar cortex from most other mammals in our dataset. PV+ mossy fibres of the megachiropterans, but PV- parallel fibres, serves to distinguish these species from most other species examined (Table 2). CB+ Golgi type II neurons in the genus Oryx (Table 3) distinguishes this genus from many others in the Cetartiodactyla and other mammals. While CB- mossy fibres in all the marsupials studied (Table 3) indicates this to be a feature of marsupials, but not most other mammals. CR+ basket cells in cetaceans, hippopotamus, domestic pig, and dromedary camel, distinguishes these closely related cetartiodactyls from others within this order as well as from most other mammalian species (Table 4). The CR- Golgi-type II cells of the bathyergid African mole rats distinguishes these species from other rodents and many other mammals (Table 4).
Of the lineages where more than one species was examined, the greatest amount of “lineage-typical” variation from the most commonly observed chemoarchitecture outlined above was observed in the microchiropterans (5 variations) and Erinaceidae (4 variations). Those lineages with the least number of shared variations include the Carnivora, Perissodactyla, Cetartiodactyla, Primates, and Hyracoidea, each having 1 variation, but not always the same variation. Thus, there are lineages that vary more, or less, from the most commonly observed calcium-binding protein chemoarchitecture; however, it must be emphasised that there are variations in every lineage examined – no lineage represents the most commonly observed chemoarchitecture of calcium-binding proteins.
4.4 Individual species variations and lack of variations
In addition to these potential lineage related variations, we noted individual species that either presented with many variations from the most commonly observed chemoarchitecture, or those that varied very little (Tables 2–4). While we identify species that possess several variations, we remind the reader of the limitations of the current study outlined above. The species that evinced the most variations compared to the most commonly observed chemoarchitecture was the short-beaked echidna that presented with 11 variations (Ashwell et al., 2007). A substantial number of variations were also noted in the greater forest shrew (5 variations), the northern fur seal (4), the tree pangolin (5) (Imam et al., 2019), the Arabian oryx (10), the African buffalo (9), the Norway rat (5) (Rogers, 1989; Celio, 1990; Résibois and Rogers, 1992), the human (8) (primarily related to the calbindin data reported by Flace et al., 2014), the two-toed sloth (5), and the African elephant (8) (Maseko et al., 2013a). Of these more variable species, it should be noted that the greater forest shrew, northern fur seal, tree pangolin, Arabian oryx, African buffalo, and African elephant all underwent very similar preservation, storage, and immunostaining procedures, indicating that the variations noted here for these species are likely to be true variations and not related to technical differences. Of specific interest for the field of translational research are the number of variations noted in the Norway rat (the commonly used laboratory rat) and the human. Of the 30 characters recorded for each species in this study, the Norway rat and the human only unequivocally shared 7 characters, with 10 characters being unequivocally different, and 13 characters being indeterminate due to lack of data in humans (Satoh et al., 1991; Braak and Braak, 1993; Flace et al., 2014). This indicates that the calcium-binding protein chemoarchitecture of the Norway rat and human cerebellar cortex are substantially different, making inferences regarding the potential functional correlates of the calcium-binding proteins in the neural circuitry of the human cerebellar cortex as derived from studies of the rat cerebellar cortex tenuous.
While some species did present with a great deal of variation, others were very similar to the most commonly observed chemoarchitecture. The only species to show the full suite of most commonly observed chemoarchitecture, in terms of the neural structures containing calcium-binding proteins, was the western tree hyrax (an Afrotherian). Several species of carnivore, the three species of perissodactyls studied, the nyala, bongo, East African root rat, Asian garden dormouse, black-tailed prairie dog, several species of primate including the non-human apes, the giant otter shrew, and the rock hyrax all exhibited only one variation to the most commonly observed “ chemoarchitecture, although it should be noted that the variable trait often differed between these species (Tables 2–4). The perhaps unexpected conclusion that can be drawn from this comparison is that, in terms of the chemoarchitecture of the calcium-binding proteins in the mammalian cerebellar cortex, the most species evincing the most commonly observed chemoarchitecture of the calcium-binding proteins in the cerebellar cortex was the western tree hyrax (Dendrohyrax dorsalis), the reason for this being unclear.
4.5 Potential functional implications of chemoarchitectural variations of calcium-binding proteins in the mammalian cerebellar cortex
As outlined earlier, calcium dynamics are an essential part of intracellular compartmental functioning in all neuronal types (e.g., Grienberger and Konnerth, 2012). The calcium-binding proteins affect intraneuronal calcium transients (e.g., Schwaller et al., 2002), with parvalbumin acting as a slow buffer, calbindin a faster buffer, and calretinin a modulator (e.g., Billing-Marczak and Kuznicki, 1999; Schmidt et al., 2003; Barinka and Druga, 2010). It is thought that these proteins are critical for synaptic plasticity and thus learning (Schwaller et al., 2002). Thus, the presence or absence of specific calcium-binding proteins in the various cell types and neural structures that form the cerebellar circuit, can affect how neural information is processed and the resultant neural or behavioural outcomes. It is known that the cerebellar cortex has a greater diversity of cell types (based on molecular analyses), with greater functionality, connectivity, regional specializations, modulatory mechanisms, and population dynamics (e.g., Witter and de Zeeuw, 2015; Hawkes, 2018; Hull and Regehr, 2022), than envisaged in the Marr-Albus model (Marr, 1969; Albus, 1971; Ito, 1972). The current study adds to this complexity of the cerebellar cortex, especially when comparing across mammalian species – it is very difficult to envisage that circuit activity as determined in the commonly studied Norway rat will be definitively applicable to humans, or any other mammal. Precisely how the variability in the chemoarchitecture of the calcium-binding proteins will specifically affect the processing of neural information in the cerebellar cortex of different mammalian species and how this will affect the neural and behavioural outcomes requires detailed investigations that are beyond the scope of the current study.
The cerebellum is known to be involved in a variety of motor (e.g., Manto et al., 2012), perceptual (e.g., Baumann et al., 2015), and cognitive (e.g., Schmahmann, 1996; Molinari et al., 2008; D’Angelo and Casali, 2012) functions. The variety of calcium-binding protein based chemoarchitectures outlined in the current study will likely play a role in altering, or perhaps fine-tuning, these varied functions across mammalian species as necessary for vital functions related to each individual species’ life history; however, how variations in the expression patterns of these proteins may specifically affect these vital functions is unclear.
One interesting example is the African elephant, which is known to have the largest absolute and relative cerebellum size of all vertebrates (Maseko et al., 2012), containing 257 x109 neurons (Herculano-Houzel et al., 2014; three times more than the entire human brain, Herculano-Houzel, 2009), an each of these neurons appears to be more structurally complex than the homologous neurons in other mammals (Maseko et al., 2013a; Jacobs et al., 2014). The elephant cerebellum is associated with an enlarged inferior olivary nuclear complex, a key structure providing climbing fibre input to the cerebellar cortex (de Zeeuw et al., 1998; Maseko et al., 2013b). In the present study it is shown that the calcium-binding protein based chemoarchitecture of the African elephant cerebellar cortex shows 8 variances compared that most commonly observed. The elephant must use this circuitry to control one of the most complex musculature appendages found in mammals – the trunk, which can undergo almost unlimited degrees of freedom of movement. The variations in the chemoarchitecture of the elephant cerebellar cortex likely play a significant role in the control of the movement of this specialised appendage, as well as in learning to use, and in refining the use of, this appendage, but at present it is unclear precisely what role this varied calcium-binding chemoarchitecture may play.
As a perhaps more speculative example, the cerebellum appears to play a role in the perception of the passage of time, especially in perceptual and motor timing tasks in the millisecond to second range (e.g., Mathew et al., 1998; Muller and Nobre, 2014; Mioni et al., 2020). Given the role calcium-binding proteins play on calcium dynamics within cellular compartments (Schwaller et al., 2002), and the variations seen across mammalian species reported herein, it would be reasonable to conclude that the manner in which different mammalian species perceive the passage of time, in this sub-second range, is likely to differ, in both lineages and individual species. The microchiropterans studied herein all rely on echolocation for the acquisition of food, and this lineage shows significant variations in chemoarchitecture compared to other mammals. Using echolocation during flight to capture fast moving prey, is heavily reliant on millisecond range neural processing to coordinate acoustic signal processing and vocal motor control (e.g., Moss and Sinha, 2003). Thus, it is possible that the variations observed in the expression of calcium-binding proteins in the cerebellar cortex of the microchiropterans play a significant role in allowing them to accomplish prey capture during flight – perhaps, in some currently unknown way, the perception of the passage of time is slowed for the microchiropterans during prey localisation and capture, allowing them to respond to evasive actions of the prey with greater precision and making them more successful hunters. Perhaps with further investigations into the role of the calcium-binding proteins, and their effect on calcium dynamics in specific cellular components of the cerebellar cortical neural circuitry, specifically in a comparative setting, a deeper understanding of the role of the cerebellum in control of the trunk or prey capture using echolocation may be reached. Such an understanding may, more broadly, lead to greater insights into the neural and perceptual worlds of mammals and how they successfully control, learn, and refine a range of life-sustaining activities in the environments they inhabit.
Data availability statement
The original contributions presented in the study are includedin the article/supplementary material. Further inquiries can bedirected to the corresponding author.
Ethics statement
The animals used in this study were treated according to the guidelines of the University of Witwatersrand (Clearance number 2013/05/02B) and the George Washington University (IACUC number A2002-023) Animal Ethics Committees, which correspond to those of the NIH for care and use of animals in scientific experimentation. The study was conducted in accordance with the local legislation and institutional requirements.
Author contributions
AB: Conceptualization, Formal analysis, Investigation, Methodology, Project administration, Visualization, Writing – original draft. CS: Methodology, Resources, Validation, Writing – review & editing, Funding acquisition. PH: Methodology, Resources, Validation, Writing – review & editing. MB: Resources, Writing – review & editing. AA: Resources, Writing – review & editing, Funding acquisition. OM: Resources, Writing – review & editing. NB: Resources, Writing – review & editing. CK-K: Resources, Writing – review & editing. EG: Resources, Writing – review & editing. PM: Conceptualization, Data curation, Formal analysis, Funding acquisition, Investigation, Methodology, Project administration, Resources, Supervision, Validation, Visualization, Writing – original draft.
Funding
The author(s) declare financial support was received for the research, authorship, and/or publication of this article. This work was supported by funding from the South African National Research Foundation (PM), the National Science Foundation (EF-2021785, DRL-2219759) (CS), Researcher Supporting Project (RSPD2023R602) King Saud University (AA).
Conflict of interest
The authors declare that the research was conducted in the absence of any commercial or financial relationships that could be construed as a potential conflict of interest.
The author(s) declared that they were an editorial board member of Frontiers, at the time of submission. This had no impact on the peer review process and the final decision.
Publisher’s note
All claims expressed in this article are solely those of the authors and do not necessarily represent those of their affiliated organizations, or those of the publisher, the editors and the reviewers. Any product that may be evaluated in this article, or claim that may be made by its manufacturer, is not guaranteed or endorsed by the publisher.
References
Albus J. S. (1971). A theory of cerebellar function. Math. Biosci. 10, 25–61. doi: 10.1016/0025-5564(71)90051-4
Ashwell K. W. S., Paxinos G., Watson C. R. R. (2007). Cyto- and chemoarchitecture of the cerebellum of the short-beaked echidna (Tachyglossus aculeatus). Brain Behav. Evol. 70, 71–89. doi: 10.1159/000102970
Barinka F., Druga R. (2010). Calretinin expression in the mammalian neocortex: a review. Physiol. Res. 59, 665–677. doi: 10.33549/physiolres.931930
Baumann O., Borra R. J., Bower J. M., Cullen K. E., Habas C., Ivry R. B., et al. (2015). Consensus paper: The role of the cerebellum in perceptual processes. Cerebellum 14, 197–220. doi: 10.1007/s12311-014-0627-7
Bertelsen M. F. (2018). “Issues surrounding surplus animals in zoos,” in Fowler’s zoo and wild animal medicine, current therapy, vol. 9 . Eds. Miller R. E., Lamberski N., Calle P. (Elsevier, London), 134–137.
Billing-Marczak K., Kuznicki J. (1999). Calretinin – sensory or buffer – function still unclear. Pol. J. Pharmacol. 51, 173–178.
Braak E., Braak H. (1993). The new monodendritic neuronal type within the adult human cerebellar granule cell layer shows calretinin-immunoreactivity. Neurosci. Lett. 154, 199–202. doi: 10.1016/0304-3940(93)90206-z
Caballero A., Tseng K. Y. (2016). GABAergic function as a limiting factor for prefrontal maturation during adolescence. Trends Neurosci. 39, 441–448. doi: 10.1016/j.tins.2016.04.010
Celio M. R. (1990). Calbindin D-28k and parvalbumin in the rat nervous system. Neuroscience 35, 375–475. doi: 10.1016/0306-4522(90)90091-h
D’Angelo E., Casali S. (2012). Seeking a unified framework for cerebellar function and dysfunction: from circuit operations to cognition. Front. Neural Circuits 6, 116. doi: 10.3389/fncir.2012.00116
de Zeeuw C. I., Simpson J. I., Hoogenraad C. C., Galjart N., Koekkoek S. K. E., Ruigrok T. J. H. (1998). Microcircuitry and function of the inferior olive. Trends Neurosci. 21, 391–400. doi: 10.1016/s0166-2236(98)01310-1
Flace P., Lorusso L., Laiso G., Rizzi A., Cagiano R., Nico B., et al. (2014). Calbindin-D28K immunoreactivity in the human cerebellar cortex. Anat. Rec. 297, 1306–1315. doi: 10.1002/ar.22921
Grienberger C., Konnerth A. (2012). Imaging calcium in neurons. Neuron 73, 862–885. doi: 10.1016/j.neuron.2012.02.011
Hawkes R. (2018). The Ferdinando Rossi memorial lecture: Zones and stripes – pattern formation in the cerebellum. Cerebellum 17, 12–16. doi: 10.1007/s12311-017-0887-0
Herculano-Houzel S. (2009). The human brain in numbers: a linearly scaled-up primate brain. Front. Hum. Neurosci. 3. doi: 10.3389/neuro.09.031.2009
Herculano-Houzel S., Avelino-de-Souza K., Neves K., Porfirio J., Messeder D., Feijó L. M., et al. (2014). The elephant brain in numbers. Front. Neuroanat. 8. doi: 10.3389/fnana.2014.00046
Herculano-Houzel S., Catania K., Manger P. R., Kaas J. H. (2015). Mammalian brains are made of these: A dataset of the numbers and densities of neuronal and nonneuronal cells in the brain of Glires, Primates, Scandentia, Eulipotyphlans, Afrotherians and Artiodactylsm and their relationship with body mass. Brain Behav. Evol. 86, 145–163. doi: 10.1159/000437413
Hull C., Regehr W. G. (2022). The cerebellar cortex. Annu. Rev. Neurosci. 45, 151–175. doi: 10.1146/annurev-neuro-091421-125115
Imam A., Bhagwandin A., Ajao M. S., Spocter M. A., Manger P. R. (2019). The brain of the tree pangolin (Manis tricuspis). VI. The brainstem and cerebellum. J. Comp. Neurol. 527, 2440–2473. doi: 10.1002/cne.24721
Ito M. (1972). Neural design of the cerebellar motor control system. Brain Res. 40, 81–84. doi: 10.1016/0006-8993(72)90110-2
Jacobs B., Johnson N. L., Wahl D., Schall M., Maseko B. C., Lewandowski A., et al. (2014). Comparative neuronal morphology of the cerebellar cortex in afrotherians, carnivores, cetartiodactyls, and primates. Front. Neuroanat. 8. doi: 10.3389/fnana.2014.00024
Kalininchenko S. G., Pushchin I. I. (2008). Calcium-binding proteins in the cerebellar cortex of the bottlenose dolphin and harbour porpoise. J. Chem. Neuroanat. 35, 364–370. doi: 10.1016/j.jchemneu.2008.03.003
Kappers C. U. A., Huber G. C., Crosby E. C. (1960). The comparative anatomy of the nervous system of vertebrates, including man (New York, NY: Hafner Publishing Company).
Manger P. R., Pillay P., Maseko B. C., Bhagwandin A., Gravett N., Moon D. J., et al. (2009). Acquisition of brains from the African elephant (Loxodonta africana): Perfusion-fixation and dissection. J. Neurosci. Meth. 179, 16–21. doi: 10.1016/j/jneumeth.2009.01.001
Manto M., Bower J. M., Conforto A. B., Delgado-Garcia J. M., da Guarda S. N. F., Gerwig M., et al. (2012). Consensus paper: Roles of the cerebellum in motor control – The diversity of ideas on cerebellar involvement in movement. Cerebellum 11, 457–487. doi: 10.1007/s12311-011-0331-9
Marr D. (1969). A theory of cerebellar cortex. J. Physiol. 202, 437–470. doi: 10.1113/jphysiol.1969.sp008820
Maseko B. C., Jacobs B., Spocter M. A., Sherwood C. C., Hof P. R., Manger P. R. (2013a). Qualitative and quantitative aspects of the microanatomy of the African elephant cerebellar cortex. Brain Behav. Evol. 81, 40–55. doi: 10.1159/000345565
Maseko B. C., Patzke N., Fuxe K., Manger P. R. (2013b). Architectural organization of the African elephant diencephalon and brainstem. Brain Behav. Evol. 82, 83–128. doi: 10.1159/000352004
Maseko B. C., Spocter M. A., Haagensen M., Manger P. R. (2012). Elephants have relatively the largest cerebellum size of mammals. Anat. Rec. 295, 661–672. doi: 10.1002/ar.22425
Mathew R. J., Wilson W. H., Turkington T. G., Coleman R. E. (1998). Cerebellar activity and disturbed time sense after THC. Brain Res. 797, 183–189. doi: 10.1016/s0006-8993(98)00375-8
Mioni G., Grondin S., Bardi L., Stablum F. (2020). Understanding time perception through non-invasive brain stimulation techniques: A review of studies. Behav. Brain Res. 377, 112232. doi: 10.1016/j.bbr.2019.112232
Molinari M., Chiricozzi F., Clausi S., Tedesco A., de Lisa M., Leggio M. (2008). Cerebellum and detection of sequences, from perception to cognition. Cerebellum 7, 611–615. doi: 10.1007/s12311-008-0060-x
Moss C. F., Sinha S. R. (2003). Neurobiology of echolocation in bats. Curr. Opin. Neurobiol. 13, 751–758. doi: 10.1016/j.conb.2003.10.016
Muller T., Nobre A. C. (2014). Perceiving the passage of time: neural possibilities. Ann. N. Y. Acad. Sci. 1326, 60–71. doi: 10.1111/nyas.12545
Résibois A., Rogers J. H. (1992). Calretinin in rat brain: an immunohisto-chemical study. Neuroscience 46, 101–134. doi: 10.1016/0306-4522(92)90012-q
Rogers J. H. (1989). Immunoreactivity for calretinin and other calcium-binding proteins in cerebellum. Neuroscience 31, 711–721. doi: 10.1016/0306-4522(89)90435-1
Satoh J., Tabira T., Sano M., Nakayama H., Tateishi J. (1991). Parvalbumin-immunoreactive neurons in the human central nervous system are decreased in Alzheimer’s disease. Acta Neuropathol. 81, 388–395. doi: 10.1007/BF00293459
Schmahmann J. D. (1996). The cerebrocerebellar system: anatomic substrates of the cerebellar contribution to cognition and emotion. Hum. Brain Mapp. 4, 174–198. doi: 10.1002/(SICI)1097-0193(1996)4:3<174::AID-HBM3>3.0.CO;2-0
Schmidt H., Stiefel K. M., Racay P., Schwaller B., Eilers J. (2003). Mutational analysis of dendritic Ca2+ kinetics in rodent Purkinje cells: role of parvalbumin and calbindin D28k. J. Physiol. 551, 13–32. doi: 10.1113/jphysiol.2002.035824
Schwaller B. (2020). Cytosolic Ca2+ buffers are inherently Ca2+ signal modulators. Cold Spring Harb. Perspect. Biol. 12, a035543. doi: 10.1101/cshperspect.a035543
Schwaller B., Durussel I., Jermann D., Herrmann B., Cox J. A. (1997). Comparison of the Ca2+-binding properties of human recombinant calretinin-22k and calretinin. J. Biol. Chem. 272, 29633–29671. doi: 10.1074/jbc.272.47.29663
Schwaller B., Meyer M., Schiffmann S. (2002). ‘New’ functions for ‘old’ proteins: The role of the calcium-binding proteins calbindin D-28k, calretinin and parvalbumin, in cerebellar physiology. Studies with knockout mice. Cerebellum 1, 241–258. doi: 10.1080/147342202320883551
Keywords: calcium-binding proteins (CBPs), cerebellar evolution, brain evolution, cerebellar function, RRID - AB_10000343, RRID - AB_10000340, RRID - AB_10000321
Citation: Bhagwandin A, Sherwood CC, Hof PR, Bertlesen MF, Alagaili AN, Mohammed OB, Bennett NC, Kaswera-Kyamakya C, Gilissen E and Manger PR (2024) Parvalbumin, calbindin, and calretinin immunostaining of 10 neural structures within the cerebellar cortex of 143 mammal species. Front. Mamm. Sci. 3:1355791. doi: 10.3389/fmamm.2024.1355791
Received: 14 December 2023; Accepted: 19 February 2024;
Published: 08 March 2024.
Edited by:
Ruth Benavides-Piccione, Spanish National Research Council (CSIC), SpainReviewed by:
Matthew T. K. Kirkcaldie, University of Tasmania, AustraliaJavier DeFelipe, Universidad Politécnica de Madrid, Spain
Copyright © 2024 Bhagwandin, Sherwood, Hof, Bertlesen, Alagaili, Mohammed, Bennett, Kaswera-Kyamakya, Gilissen and Manger. This is an open-access article distributed under the terms of the Creative Commons Attribution License (CC BY). The use, distribution or reproduction in other forums is permitted, provided the original author(s) and the copyright owner(s) are credited and that the original publication in this journal is cited, in accordance with accepted academic practice. No use, distribution or reproduction is permitted which does not comply with these terms.
*Correspondence: Paul R. Manger, UGF1bC5NYW5nZXJAd2l0cy5hYy56YQ==