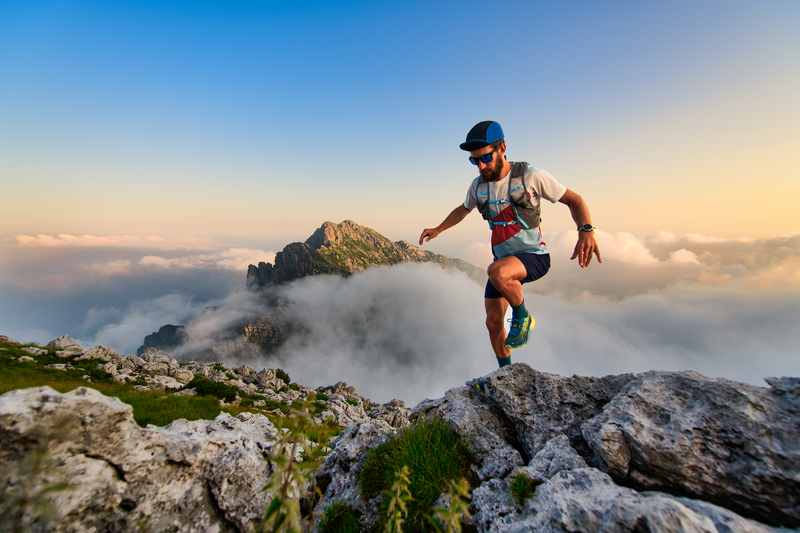
95% of researchers rate our articles as excellent or good
Learn more about the work of our research integrity team to safeguard the quality of each article we publish.
Find out more
REVIEW article
Front. Mamm. Sci. , 16 February 2024
Sec. Nervous System and Cognate Behaviors
Volume 3 - 2024 | https://doi.org/10.3389/fmamm.2024.1309665
This article is part of the Research Topic Editors' Showcase: Nervous System and Cognate Behaviors View all 9 articles
The claustrum, a structure having extensive connectivity with the rest of the brain and being involved in many high-cognitive processes, is still one of the least understood parts of the mammalian nervous system. Accelerated advancement of genetic tools for rodents in the last decade have resulted in many breakthroughs about its interaction with cortical and subcortical regions, while human/primate studies have been invaluable in revealing its effects on conscious behaviour. However, these findings did not elucidate conclusively the principles of its internal dynamics, which would clarify its function within the brain network. The first step in this direction is to know the characteristics of major types of neurons in the claustrum. In this review, we are looking at the data allowing a comparison between the main neuronal types of the claustrum in primates and rodents, with the aim of showing the extent of known commonalities and differences, and highlighting the research gap between the two orders. The results indicate that in both there is a ratio excitatory/inhibitory neurons higher than in the cortex, but with a lower baseline activity of the excitatory neurons due to the higher inhibition. The local excitation in the claustrum is provided by collaterals of neurons projecting to the cortex. Secondary neuronal markers such as Calcium binding proteins and somatostatin tend to be expressed differently in the claustrum of primates than in that of rodents, specifically in more classes of neurons and across a larger area. The spatial distribution of neuropeptide Y might be a conserved motif across the two orders. The work in rodents has an undisputable advance in the study of electrical properties for each class of claustrum neurons. However, for a deep understanding of the claustrum function in the human brain, primate studies remain indispensable.
The claustrum is a sheet-like group of neurons located underneath the lateral temporal and caudal orbitofrontal cerebral cortex. Major landmarks for its position are the insular cortex and the piriform cortex laterally, while the striatum and the amygdala are in its medial vicinity (Figure 1). In big mammals the white matter encases the claustrum completely, with the external capsule separating it from the striatum, and the extreme capsule from the insular cortex. In smaller mammals, particularly rodents, the extreme capsule is less developed, and the claustrum appears immediately adjacent to the cortex [Figure 1 (Mathur et al., 2009; Smith et al., 2019)]. Regarding its dimensions, when measuring the changes accompanying the increase in brain size across species, the claustrum expands faster than the allocortex (from 2.7% the volume of the allocortex in mice to 18% in humans), but slower than the neocortex [0.45% (humans) – 6.5% (mice)]. Consequently, the ratio of its volume to the total cortical volume is relatively steady, around 1% [0.44% (humans) – 2% (mice)] (Kowiański et al., 1999).
Figure 1 Differences in claustrum complex across species. (A) The claustrum complex in humans, at the level of anterior commissure. Laterally, the insular cortex borders entirely the insular component of the complex, with the extreme capsule in-between. The external capsule separates the claustrum from the putamen. The amygdalar complex lies medio-ventrally. The ventral claustrum is located just beneath the piriform cortex (not shown). (B) The claustrum complex in marmosets at the level of anterior commissure. Like in humans, the insular component is sandwiched between the insular cortex and the putamen, with the extreme and external capsules in-between, respectively. The endopiriform nucleus lies between the insular cortex and lateral amygdala. The endopiriform nucleus also extends into piriform cortex at the widest extent of the claustrum (not shown). (C) The claustrum complex in rats. It straddles the border of insular and piriform cortices, with the insular component located within the insular cortex and separated from the striatum by the external capsule, while the endopiriform nucleus extends into piriform cortex and is bordered medially by the amygdaloid complex. In mice it has a similar structure. For an elaborate discussion about the borders and components of the endopiriform nucleus in humans, non-human primates and rodents, see Smith et al., 2019. Colours indicate the insular claustrum (magenta), the endopiriform nucleus (cyan), the insular cortex (violet), amygdaloid complex (gold) and the anterior commissure (grey). ac, anterior commissure; Amy, amygdaloid complex; Cd, caudate nucleus; ec, external capsule; ex, extreme capsule; Ins, insular cortex; Pir, piriform cortex; Pu, putamen; STR, striatum. Based on Smith et al., 2019. See also Figure 1 in Madden et al., 2022.
The claustrum remains one of the least understood parts of the mammalian nervous system. In the last 15 years, it has become increasingly popular among researchers due to its widespread bidirectional connectivity with the cortex and mostly unidirectional inputs from major subcortical structures, which opened successful avenues for studying its involvement in the functioning of various brain networks [for recent reviews, see (Jackson et al., 2020; Wong et al., 2021; Madden et al., 2022)]. As a result, spectacular findings have been published about its anatomy and physiology. Particularly, the primate and the rodent claustrum have been in the research spotlight. This happened despite the major discoveries of the sensory maps in the claustrum, of connectivity patterns and of their physiological responses being revealed 40 years ago in cats (Olson and Graybiel, 1980; LeVay and Sherk, 1981a, b; Sherk and LeVay, 1981). With the advent of novel technologies allowing the manipulation of select classes of cells via genetic engineering, rodents, and particularly mice, have become a preferred animal model to study in detail the internal circuitry of the claustrum, together with its connectivity and interaction with the rest of the brain. Nevertheless, studies in humans continued to show the involvement of the claustrum in multiple high cognitive functions and brain disorders [for detailed reviews, see (Smythies et al., 2014; Patru and Reser, 2015; Atilgan et al., 2022)], but only at large scale, lacking mechanistic descriptions and satisfactory explanations of its function.
Consequently, although important theoretical concepts have been proposed to accommodate the recent experimental breakthroughs (Vidyasagar and Levichkina, 2019; Wong et al., 2021; Madden et al., 2022), currently we do not have principles of the claustrum function formulated and demonstrated in detail, in a similar manner as the canonical microcircuit for the neocortex, which explains the electrophysiological properties of the neocortex in terms of neuronal composition, and of local and global connectivity (Douglas et al., 1989; Douglas and Martin, 2004). The apparent lack of laminar organisation along the axis perpendicular to the cortical surface, the absence of extreme capsule in small mammals (Figure 1) and the common developmental origin with the insular cortex (Wullimann, 2017) suggest that the claustrum should be integrated in the circuitry of the nearby cortex as an additional cortical layer. However, this view cannot hold for multiple reasons. First, the developmental trajectory of the claustrum complex is significantly different than that of the cortex (Watson and Puelles, 2017; Hoerder-Suabedissen et al., 2022). Second, the intrinsic connectivity of the claustrum has patterns that cannot be found in any cortical layer: the connections are segregated along the dorso-ventral axis, there is an abundance of long-range internal projections along the rostro-caudal axis (Behan and Haberly, 1999; Smith and Alloway, 2010; Watson et al., 2017) and the vast majority of dendrites are confined to the claustrum, while avoiding nearby structures (Watakabe et al., 2014). Third, the distribution across the claustrum of the numerous connections with the cortex is different than the distribution of cortico-cortical connections of any area: in the claustrum they are segregated in a loose topographic arrangement, according to the functional subnetworks the areas are associated with: visual, auditory, somatosensory, motor, resting state etc [humans: (Pathak and Fernandez-Miranda, 2014), nonhuman primates: (Pearson et al., 1982; Minciacchi et al., 1991; Wada and Tsuchimochi, 1997; Reser et al., 2014); rodents: (Sadowski et al., 1997; Atlan et al., 2017)]. And fourth, although there are abundant connections between the claustrum and the nearby insular cortex (Augustine, 1985; Narikiyo et al., 2020), the laminar distributions within the insular cortex of the projections to/from claustrum do not differ from the laminar distributions within other cortical areas of projections to/from claustrum (Wang et al., 2023). All these lead to the conclusion that the claustrum and the cortex are separate brain regions.
Thus, we should strive to create a theory for the claustrum function that accommodates these biological realities. The first bottom-up steps consist in knowing the major classes of neurons present in the claustrum, their spatial distributions, together with their electrical properties, that would further help assess the patterns of anatomical and functional connectivity motifs. This type of knowledge has accumulated for species which could be studied with modern techniques - mice and rats. However, the caveat of this situation is addressing the involvement of the claustrum in a large spectrum of high cognitive functions, for which the more suited animal models are non-human primates, particularly the popular marmosets and macaques. Although published data has increased for them as well, there is a significant research gap between the two orders.
In the current article we are comparing the anatomy and physiology of major classes of neurons in the claustra of primates and rodents, highlighting the extent of this research gap. We will discuss the resemblances and differences between the two orders. In each chapter, we are first looking at the anatomical properties of the classes of claustrum neurons studied in detail in at least two species of each order, then at their physiological properties, where available. We understand by claustrum the region which includes the insular claustrum and the Endopiriform nucleus. We will specify whenever a property refers to one of these two large subdivisions.
The results indicate that the claustrum, despite its continuous appearance, has a heterogeneity of neurons in its composition, each with its particular spatial distribution.
The Golgi impregnation stain involves the introduction of silver nitrate to nervous tissue hardened with potassium dichromate. When absorbed by neurons, it colors their somas and their neurites in black, revealing fine morphological structures like dendritic spines in excitatory neurons (Golgi, 1873).
In human claustrum, Golgi staining revealed a heterogeneous population of neurons with poly-morphous perikarya and spiny dendrites, making about 93% of the claustrum neurons (Braak and Braak, 1982; Spahn and Braak, 1985). A numerous population of spiny neurons also exists in squirrel monkeys and rhesus monkeys, with the shape varying according to the position within the claustrum (Brand, 1981).
Biocytin/neurobiotin are conjugates of biotin (vitamin H), with a high affinity for avidin. When injected inside the neurons after electrophysiological recordings, they can afterwards be detected with avidin-peroxidase or avidin conjugated with fluorescent compounds, revealing fine morphological structures like in Golgi stains (Horikawa and Armstrong, 1988; Lapper and Bolam, 1991).
Biocytin/neurobiotin showed that the dominant type of neurons in the mouse claustrum are those with spiny dendrites, which project to the cortex (Kim et al., 2016; White and Mathur, 2018).
VGLUT is a synaptic vesicular protein used to transport glutamate, a major excitatory neurotransmitter in the mammalian central nervous system. It has three isoforms (VGLUT 1, 2 and 3 respectively); VGLUT 1 and 2 are commonly expressed in glutamatergic synapses, while VGLUT 3 is known to colocalize with other neurotransmitters (Takamori, 2006).
Detection of VGLUT mRNA has been used to investigate the distribution of excitatory neurons in the claustrum of primates and rodents. In the human brain, VGLUT3 is primarily expressed in the claustrum (Hawrylycz et al., 2015). VGLUT1 mRNA was detected in the claustrum of Japanese and rhesus macaques, along with low levels of VGLUT2 (Watakabe et al., 2014).
As for rodents, in the claustrum of the rat have been detected both VGLUT1 (Geisler et al., 2007) and VGLUT 2 cells (Hur and Zaborszky, 2005), but VGLUT3 was negligible (Herzog et al., 2004). In mice, one study found that VGLUT1 mRNA is expressed in 88–90% of claustral neurons (Takahashi et al., 2023) while another found it in 83% of DAPI-positive claustrum cells (which include both neurons and glia) (Atlan et al., 2018).
The excitatory neurons in the monkey claustrum have a baseline activity in-vivo of about 2-4 Hz (Shima et al., 1996; Remedios et al., 2014). This is relatively quiescent compared to the ~10-12 Hz in the cortex (Miller et al., 1996; Luck et al., 1997), or in the thalamus (Royal et al., 2006). The claustrum excitatory neurons respond to their characteristic stimuli with sharp increases of firing rates, followed by sharp decreases of activity (Remedios et al., 2010, 2014).
The baseline activity of excitatory neurons in rodents is also low. Electrophysiology recordings in-vivo in mice showed spontaneous firing rates up to 4 Hz, with the value strongly dependent on the state of the animal (Narikiyo et al., 2020). They reached maximum values during resting states and slow-wave sleep, and significantly lower values during active wakefulness. Interestingly, another study showed that during specific tasks which involve attention to sensory input and motor response, the baseline activity of claustro-cortical neurons is higher, on the order of 10 Hz, and increases one second before the delivery of the stimulus (Chevee et al., 2022).
Details about the action of claustrum neurons projecting on the cortex are available in rodents only. In mice, there is direct physiological evidence that they are excitatory. In one study, injections of an AAV retrograde virus in the frontal cortex led to the expression of Cre recombinase in claustro-cortical neurons. Then, these neurons were induced to express Channelrhodopsin across the entire cell surface with injection of another AAV virus in the claustrum. When their terminals in the frontal cortex were optically stimulated, the direct effect on the target neurons was excitatory (Jackson et al., 2018). Another study used a line of mice expressing Cre recombinase in VGLUT2 positive neurons, and the claustrum was injected with an AAV virus for Channelrhodopsin expression. Stimulation of ChR2-positive/VGLUT2-positive CLA axon terminals in the cortex evoked monosynaptic excitatory postsynaptic potentials (Fodoulian et al., 2020). Other two studies used transgenic mouse lines with Cre recombinase in subpopulations of claustro-cortical neurons to express channelrhodopsin in these neurons. Again, the optical stimulation of their terminals led to depolarisations of their target neurons in the cortex (Narikiyo et al., 2020; McBride et al., 2023).
So far, there is no direct evidence for excitatory neurons in the claustrum that project only within the claustrum. It is the neurons which project to the cortex that are also responsible for intrinsic excitation, through collaterals within the claustrum (Brand, 1981; Kim et al., 2016). This is in contrast with the cortex of primates and rodents, where there are excitatory neurons which project locally, within the cortical area that contains their soma, such as the spiny stellate or the star pyramidal cells in layer 4 (Anderson et al., 1993; Lübke et al., 2000).
The excitatory neurons in the claustrum of rodents can be divided in two large classes, slow-adapting and fast adapting, according to their intrinsic electrical properties. More refined analyses revealed up to six classes (Table 1).
In rats, in-vivo intracellular recordings of 31 claustrum cells showed two types of excitatory neurons: slow-adapting and fast adapting (Shibuya and Yamamoto, 1998). Each type was represented by approximately the same proportion of neurons. The first type responded to injections of step currents with a nearly constant firing rate of action potentials (slow-adaptation), while the firing rate of the second responding to the same stimuli decreased strongly in time (fast adaptation).
Two in-vitro studies in mice used samples of around 100 cells each that projected to the anterior cingulate cortex (ACC) (Chia et al., 2017; White and Mathur, 2018). The cells were found by injections of retrograde fluorescent tracers in the ACC. The responses to current injections revealed the same categories of slow and fast adapting neurons like in rats, each type having similar proportion of neurons. However, Chia et al. (2017) further divided the strongly adapting cells into three categories, based on the waveform of the first action potential in each train. Another recent study was made on a larger sample of neurons, out of which 174 were excitatory (Graf et al., 2020). The mice had also been injected with fluorescent retro beads in various parts of the cortex, thalamus, habenula and hippocampus. Some of the labelled, along with unlabeled claustrum cells were tested with electrophysiological techniques in-vitro. Specifically, for each neuron the authors measured 38 intrinsic electric properties from its response to injections of step currents. All the labelled cells from injections had intrinsic electrical properties of excitatory cells. Hierarchical clustering analysis further revealed 6 classes of excitatory neurons. The first four classes contained slow- and fast-adapting cells projecting to cortex like in the previous studies, but the total group of fast-adapting cells (labelled and unlabeled) was about twice as big as the slow-adapting cells (labelled and unlabeled). The slow adapting cells occupied one class, while the fast-adapting cells covered three classes, similar to Chia et al. (2017). The fifth class included almost exclusively subcortical-projecting cells (12 out of 13) which adapted the amplitude of the action potentials instead of the firing frequency. The last category was occupied by a single cell, unlabeled from injection of tracer, displaying a neurochemical marker for inhibitory neuros (vasoactive intestinal peptide - VIP), but having electrical properties of an excitatory neuron.
In human claustrum, Golgi staining revealed four populations of aspiny, inhibitory neurons, making about 7% of the claustrum neurons (Braak and Braak, 1982; Spahn and Braak, 1985). In squirrel monkeys and rhesus monkeys there are at least two populations of aspiny neurons with distinct morphologies (Brand, 1981).
Biocytin/neurobiotin revealed in the claustrum of the mouse neurons with aspiny dendrites, which project locally, and are inhibitory (Kim et al., 2016; White and Mathur, 2018).
GABA (γ-Aminobutyric acid) is a major inhibitory neurotransmitter in the mammalian central nervous system. The presence and quantification of GABAergic neurons can be determined through the detection in the somas of mRNA coding for glutamic acid decarboxylase (GAD) 65 or 67. The enzymes encoded by these genes are responsible for catalysing the production of GABA.
In macaque monkeys, GAD67 stains show a low fraction of the claustrum neurons (qualitative results only). These neurons are constrained within the boundaries of the claustrum complex, being virtually non-existent in the white matter surrounding the claustrum (extreme and external capsules) (Miyashita et al., 2005).
In rats, GAD65 and GAD67 exist in about 20% of claustrum neurons each, in many of them being colocalized (Feldblum et al., 1993). However, these estimates appear coarse compared to measures in other brain structures from the same article. The cells positive for GAD65 show homogeneous staining and are uniformly distributed throughout the claustrum, while 17.5% of the GAD67 positive cells have higher labelling intensities than the remaining labelled cells. Also, a subset of cells contains significant amounts of GAD67 mRNA, but not GAD65.
In mice, 9–11% of claustral neurons have GAD67 mRNA, being uniformly distributed across the claustral subregions (Takahashi et al., 2023). There was no co-localization between the signals for VGluT1 and GAD67 mRNAs (over 3400 VGLUT1-positive and 340 GAD67-positive cells analyzed). Another study found that 4% of DAPI-positive cells (which include both neurons and glia) were GAD65-positive. There was also a small amount of cells with co-localization between VGLUT1 and GAD65 (Atlan et al., 2018).
There are no studies to show the firing patterns of inhibitory interneurons in vivo. The few data about their electrical responses come from studies in slices, in mice (Table 1).
When electrically stimulated, the claustrum neurons that have smooth dendrites inhibit the other claustrum cells onto which they make monosynaptical projections (Kim et al., 2016). A later study recorded the response of 35 aspiny interneurons to injections of step currents and found two types of cells, covering three categories (White and Mathur, 2018). The first type, included in two categories, contained two thirds of the neurons, and consistently showed similar spike accommodations to positive current steps, and relatively low maximum firing rates, below 100 Hz. The electrical properties that differentiated the two categories were the resting membrane potentials and the amount of spontaneous firing rates. The second type of neurons, in the last category, responded with fast-spiking (maximum firing rate > 150 Hz), and minimal spike accommodation.
Moreover, Graf et al. (2020) found in a larger sample 152 claustrum cells which showed responses to step current injections typical to inhibitory interneurons (Graf et al., 2020). 63 electrical properties were used to classify the neurons. Hierarchical clustering analysis revealed the same two large types of neurons from White and Mathur (2018): low firing rates and high accommodation, and high firing rates and low accommodation. However, the neurons in White and Mathur (2018) that had low firing rates and a low resting membrane potential did not resemble any neuron in Graf et al. (2020) nor in Kim et al. (2016).
Calcium binding proteins like parvalbumin (PV), calbindin (CB) and calretinin (CR) mainly act as passive intracellular calcium ion buffers in order to regulate the resulting action potential (Gattoni and Bernocchi, 2019). They are usually expressed in both the somas and the neurites of select classes of neurons and do not co-localize.
In the human claustrum, Hinova-Palova et al. (2014) found seven classes of PV neurons, based on the existence of spiny or aspiny dendrites, and on size and shape of the soma (Hinova-Palova et al., 2014). Three of these classes contain multipolar-to-pyramidal in shape, with spiny dendrites, likely being excitatory neurons. Four of them are oval-to-fusiform in shape, with smooth aspiny dendrites, likely being inhibitory. The density of all PV-positive somas and neurites is large around the border between the insular claustrum and the Endopiriform nucleus, and relatively low at the dorsal and ventral extremities of the claustrum. Other study found five different morphologies of the PV-positive somas in human claustrum: fusiform, round, triangular, polygonal and pear shaped (Pirone et al., 2014). The same study found identical morphologies of PV-positive neurons in the chimpanzee claustrum (Pirone et al., 2014).
In monkeys, PV-positive neurons are more uniform in shape. Macaques show a single class of large cells, some of which resemble cortical pyramidal neurons, others inhibitory interneurons (Reynhout and Baizer, 1999). PV-positive neurons have also been reported in squirrel monkeys (Baizer et al., 2020) and in marmosets (Pham et al., 2019), the latter finding variable densities along the insular claustrum and the Endopiriform nucleus.
In rodents, rats and mice show a clear compartmentalization of PV expression in the claustrum. In rats, the neuropil of the insular claustrum contains high amounts of PV, while PV is virtually absent in the neuropil of the Endopiriform nucleus (Celio, 1990; Druga et al., 1993).
In mice, co-localization of GAD67 and PV showed that PV-positive neurons are approximately 20% of claustral GABAergic neurons, thus representing roughly 2% of the entire neuronal population in the claustrum (Takahashi et al., 2023). In the ventral part of the insular claustrum Real et al. (2003) and Dávila et al. (2005) found a disproportionately larger density of PV-positive neurites, compared to the rest of the claustrum, and they named this region core (Real et al., 2003; Dávila et al., 2005). Since then, the results have been replicated in other studies (Marriott et al., 2020; Ham and Augustine, 2022; Shelton et al., 2022; Grimstvedt et al., 2023; Takahashi et al., 2023). Recently, Takahashi et al. (2023) showed after 3D reconstruction of 6 individual PV-positive neurons that the PV-positive neurons with the soma in the core extend their neurites mostly within the core, while the neurites of PV neurons with the somas outside the core tend to avoid the core. Surprisingly, there are no substantial differences in the spread of PV-positive neurites of the claustrum neurons between the core and outside the core. The authors interpret the surplus of PV signal present in the core as coming from external PV-positive axons projecting on claustrum neurons or, alternatively, as a higher PV expression in the neurites within the core (Takahashi et al., 2023).
Electrophysiological studies in slices found direct evidence that the PV-positive neurons in the mouse insular claustrum are inhibitory (Kim et al., 2016). White and Mathur (2018) found in a sample of 18 PV-positive claustrum neurons that 10 of them were fast-spiking with minimal adaptation, while 8 had a relatively low firing rate, with adaptation and a depolarized resting membrane potential (White and Mathur, 2018). In a sample of 19 PV-positive neurons, Graf et al. (2020) found that 16 were fast-spiking, while 3 were slow-spiking and had a hyperpolarized resting membrane potential (Table 1).
In humans, CB immunostaining revealed across the claustrum two types of neurons: i. lightly stained, small multipolar (maximal diameter 10–15 µm), with hardly visible processes, intermingled with ii. a few larger, multipolar cells, having more darkly stained somas (maximal diameter 20 –30 µm), that emitted three or four beaded dendrites. Compared to other brain regions, the claustrum contained virtually no CB-positive neuropil (Prensa et al., 2003).
In macaques, Reynhout and Baizer (1999) found that the most numerous CB-positive neurons are the small, multipolar cells, like in humans, but with intense staining and circular somas. The second, less numerous class resembles the second type of human CB-positive cells, and the macaque PV-positive neurons in their large size and multipolarity. There is also a third, rare category of large, bipolar cells (Reynhout and Baizer, 1999).
In the marmoset, the distribution of CB-positive neurons is heterogenous, with a high density of CB-positive neurons reported in the caudal sections of the Endopiriform nucleus (Pham et al., 2019). In the squirrel monkey, CB immunoreactivity was only apparent in the somas and extreme proximal processes of positive neurons (Baizer et al., 2020).
In rodents, CB-positive neurons have aspiny dendrites and similar shapes with the ones in primates [rats (Druga et al., 1993); mice (Real et al., 2003)]. The staining pattern and neurite distribution of CB largely complement those of PV, being more numerous in the Endopiriform nucleus, indicating a separation of these neuronal populations [rats (Celio, 1990; Druga et al., 1993); mice (Real et al., 2003; Grimstvedt et al., 2023)].
In humans, CR-positive neurons are scattered across the claustrum. They are mostly bipolar with round or elongated soma (up to 20 µm diameter), with a few larger cells having a triangular soma (Prensa et al., 2003; Pirone et al., 2014). In macaques, they are bipolar cells with oval somas (Reynhout and Baizer, 1999), and in squirrel monkeys they have round, oval, or elongated somas (Baizer et al., 2020).
Intriguingly, rodents have about the same morphology, but more variable spatial distribution of CR-positive cells of the claustrum. In both rats (Druga et al., 2015) and mice (Real et al., 2003), CR neurons have smooth, aspiny dendrites. They are sparse in the core region, but in the rest of the insular claustrum the somas and neurites are dense. In rats, some dendrites extend to adjacent brain structures (Druga et al., 2015). In the Endopiriform nucleus, in both species CR neurons are relatively sparse, but in rats the lateral part contains medium-sized, multipolar neurons, with intense CR expression.
SOM, a regulatory peptide found in inhibitory interneurons, acts as a neurotransmitter or neuromodulator (Liguz-Lecznar et al., 2016). In both humans and crab-eating macaques, SOM-positive neurons with round or fusiform soma and faintly-labelled processes were found evenly scattered across the claustrum (Pirone et al., 2014).
As for rodents, in rats, they do not project to the cortex, have aspiny dendrites and are oval, round or triangular, of medium size, and are scattered across the claustrum (Kowiański et al., 2001). Some of them colocalize with CB (Kowiański et al., 2009). In mice, they represent about 30% of GABAergic neurons in the claustrum, and 3% of all claustrum neurons (Takahashi et al., 2023). The SOM signal from the neurites is stronger outside the core than in the core (Marriott et al., 2020). However, similarly to PV-positive neurons, their somas are evenly distributed across the insular claustrum, with the neurons in the core extending the neurites inside the core, and the neurons outside the core avoiding the core (Takahashi et al., 2023). The electrical properties of 28 SOM-positive neurons showed they have relatively low amounts of adaptation and respond with regular action potential firing when stimulated with step currents (Graf et al., 2020) (Table 1).
NPY is a highly abundant peptide commonly found in catecholamine-expressing neurons in both the central and peripheral nervous systems, and can elicit numerous physiological responses by activating specific pre- and postsynaptic receptors (Michel, 1991). NPY-positive neurons were observed evenly distributed in the human, macaque and chimpanzee claustrum; these darkly-stained neurons had round, fusiform or triangular soma as well as uniformly-stained processes (Pirone et al., 2014). NPY-positive beaded fibers were also found localized in the neuropil (Pirone et al., 2014). In squirrel monkey, NPY-immunoreactive fibers and cell bodies have been found in a moderate number, scattered across the claustrum (Smith et al., 1985).
In rats, NPY-positive neurons do not project to the cortex, have small- or medium-sized somas with oval, triangular or fusiform shapes, and their somas and neurites are scattered across the claustrum (Kowiański et al., 2001). They colocalize, in various proportions, with the calcium-binding proteins PV, CB and CR (Kowiański et al., 2009). In mice, they displayed a similar pattern of uniform expression when tested in the insular claustrum (Marriott et al., 2020).
We have reviewed the major neuronal components of the claustrum in humans, non-human primates and rodents in order to show common building blocks, together with the extent of their differences. We will discuss the results, point to outstanding questions, and suggest experimental approaches that could fill research gaps.
In the claustrum of both orders, the excitatory neurons are dominant, being around 90% of the neuronal population. All of them project to other brain structures and also provide intrinsic excitation to the claustrum. In vivo, they have a baseline activity relatively low compared to the cortex or the thalamus.
One difference is the intense presence of VGLUT3-positive neurons in the human claustrum and their sparse existence in the rodent claustrum. The question arises whether this is a new component in the human/non-human primate claustrum and to what extent it modifies its function compared to rodents, given that it colocalizes with other neurotransmitters.
The more advanced data in rodents shows that projection neurons have a landmark propensity to neural adaptation of firing rates or of amplitudes of their action potentials. Based on the level of adaptation, they are of at least two types. Intriguingly, neurons that project to a region in the cortex are a mixture of types [e.g., ACC-projecting neurons in Chia et al. (2017) and White and Mathur (2018)]. The adaptability may be a sign of claustrum projection neurons acting like high-pass filters for the signals received from the brain and like generators of sparse codes that are sent back to the brain (Benda, 2021). It would be interesting to know whether the primates show the same pattern. A combination of electrophysiological recordings with optotagging (Narikiyo et al., 2020; Chevee et al., 2022) could provide answers in this direction.
Both primates and rodents have about 10% inhibitory neurons in the claustrum. This is significantly different than the ~ 20% in the cortex (Wonders and Anderson, 2006). However, despite the lower proportion in the claustrum, they provide a higher amount of intrinsic inhibition, keeping the overall activity in the claustrum low (Remedios et al., 2010; Kim et al., 2016).
Some claustrum neurons that project to the cortex but have typical neurochemical markers of interneurons have been detected in mice (Graf et al., 2020), while in rats a few CR-positive interneurons extend their dendrites in the surrounding brain structures (Druga et al., 2015). This is in contrast with the claustrum interneurons in primates which are contained within the boundaries of the claustrum (Miyashita et al., 2005). This might be a consequence of the extensive isolation of the claustrum from the surrounding structures by the external and extreme capsules in primates, and might have led to changes in the motifs of claustrum circuitry.
In the claustrum of mice, the interneurons respond with either low firing rates and high spike accommodation, or high firing rates and low spike accommodation. Future theoretical studies should reveal the roles of these two types of reaction in the functioning of the claustrum. Also, it is not clear whether each region of the claustrum that connects preferentially with a cortical subnetwork (Atlan et al., 2017; Marriott et al., 2020) contains interneurons with single electrical properties, or each type of interneuron is evenly distributed across the claustrum. Optotagging combined with high-density Neuropixels recordings (Jun et al., 2017) might provide a response in both orders.
The expression of PV provides a significant difference between the claustrum of primates and rodents. This is embodied by the existence in primates of PV-positive neurons across the entire claustrum, which are both excitatory and inhibitory, with multiple sizes and shapes, compared with the anatomically-uniform and inhibitory population of PV-positive neurons in rodents, restricted to insular claustrum. Long-distance, PV-positive projection neurons also exist in other parts of the primate brain, such as in callosal axons connecting the two hemispheres [absent in rodents (Rockland, 2017)], or the optic radiation connecting LGN to V1 [marmosets (Ma et al., 2023); however, also present in rats (Lüth et al., 1993)]. We hypothesize that the PV-positive projection neurons in the claustrum of primates might connect with brain regions that in rodents are under-developed [e.g. supragranular layers of the cortex (Dehay et al., 2015)] or possibly inexistent [e.g. prefrontal cortex (Laubach et al., 2018)]. This can be verified with precise injections of new generations of recombinant adeno-associated viruses (rAAV) in the claustrum, which can selectively induce the expression of eGFP in PV-positive neurons (Tkatch et al., 2022).
In mice, the electrical properties of the PV-positive interneurons vary across the population (Kim et al., 2016; White and Mathur, 2018; Graf et al., 2020). It would be interesting to explore whether the PV-positive interneurons in primates, being more heterogeneous anatomically, have a greater variety of electrical properties.
PV is also a landmark for spatial organization of the insular claustrum in rodents. The core, a plexus of intense PV-positive neurites located in the ventral part of the insular claustrum, precisely colocalizes with neurons projecting to frontal-midline cortical regions (Atlan et al., 2017; Marriott et al., 2020; Shelton et al., 2022). The modularity of this arrangement is further highlighted by the propensity of interneurons to extend their neurites in the same region where their soma is, either inside or outside of the core (Takahashi et al., 2023). A qualitative description of a PV-plexus exists for human claustrum in a similar place as the rodent core (Hinova-Palova et al., 2014), but whether a core in primates with similar properties as the one in rodents exists, remains to be determined. This would not be impossible, given the existence of a PV core encompassing well-defined types of projection neurons in other brain structures of primates such as the thalamus (Jones, 2001).
In primates, the expression of CB is weak in the neurites of soma-positive neurons in the claustrum, in contrast with rodents. The overall distribution is similar in the two orders, with a tendency in rodents to complement the distribution of PV neurons. The situation is similar for CR, being relatively homogeneous in primates, but complementing PV in rodents, this time in the insular claustrum. In the Endopiriform nucleus there are additional clusters of neurons that are present in rats compared to mice, raising the question whether CR neurons add new motifs of connectivity in claustra of more complex brains.
SOM neurons are scattered across the claustrum of both primates and rodents. Their electrical properties in mice tend to be more uniform than those of PV-positive neurons. Also, detailed geometrical and imaging analysis in mice showed that their distribution of neurites complements that of PV-positive neurons, but the somas of both types of neurons are more evenly scattered than the neurites. An advanced geometrical analysis of the arrangement of the somas might make light in this issue.
Finally, NPY-positive neurons colocalize with other markers and are evenly distributed in the claustrum of both orders. This could indicate a global role in controlling the functioning of the claustrum.
Although appearing continuous in unstained sections and, in rodents, being hardly distinguishable from the adjacent cortex, the claustrum is a heterogeneous structure, with major variations across orders in the distributions of common neuronal components. Its quiet activity in spite of the wide connectivity with the brain fits with the metaphor of an orchestra conductor for its function (Crick and Koch, 2005) or the description as a coordinator of cortical activity (Narikiyo et al., 2020). How exactly the claustrum network echoes the multitude of signals received from the brain and what the complete range of its outputs over the cortex is should continue to be addressed with micro- and meso-scale analysis of the connectivity motifs, as well as meso- and macro-scale analysis of physiological characteristics of the interactions at global view. Rodents will continue to be at the frontiers of the first approach, while primates will provide indispensable insights for the latter.
MC: Writing – original draft, Writing – review & editing. RG: Funding acquisition, Project administration, Supervision, Writing – original draft, Writing – review & editing.
The author(s) declare financial support was received for the research, authorship, and/or publication of this article. This study was supported by the Discovery Early Career Researcher Award DE190100157 from the Australian Research Council, to RG. The funder had no role in study design, data collection and analysis, decision to publish, or preparation of the manuscript.
The authors declare that the research was conducted in the absence of any commercial or financial relationships that could be construed as a potential conflict of interest.
All claims expressed in this article are solely those of the authors and do not necessarily represent those of their affiliated organizations, or those of the publisher, the editors and the reviewers. Any product that may be evaluated in this article, or claim that may be made by its manufacturer, is not guaranteed or endorsed by the publisher.
Anderson J. C., Martin K. A. C., Whitteridge D. (1993). Form, function, and intracortical projections of neurons in the striate cortex of the monkey macacus nemestrinus. Cereb. Cortex 3, 412–420. doi: 10.1093/cercor/3.5.412
Atilgan H., Doody M., Oliver D. K., McGrath T. M., Shelton A. M., Echeverria-Altuna I., et al. (2022). Human lesions and animal studies link the claustrum to perception, salience, sleep and pain. Brain: J. Neurol. 145, 1610–1623. doi: 10.1093/brain/awac114
Atlan G., Terem A., Peretz-Rivlin N., Groysman M., Citri A. (2017). Mapping synaptic cortico-claustral connectivity in the mouse. J. Comp. Neurol. 525, 1381–1402. doi: 10.1002/cne.23997
Atlan G., Terem A., Peretz-Rivlin N., Sehrawat K., Gonzales B. J., Pozner G., et al. (2018). The claustrum supports resilience to distraction. Curr. Biol. 28, 2752–62.e7. doi: 10.1016/j.cub.2018.06.068
Augustine J. R. (1985). The insular lobe in primates including humans. Neurol. Res. 7, 2–10. doi: 10.1080/01616412.1985.11739692
Baizer J. S., Webster C. J., Baker J. F. (2020). The claustrum in the squirrel monkey. Anatom. Rec. 303, 1439–1454. doi: 10.1002/ar.24253
Behan M., Haberly L. B. (1999). Intrinsic and efferent connections of the endopiriform nucleus in rat. J. Comp. Neurol. 408, 532–548. doi: 10.1002/(SICI)1096-9861(19990614)408:4<532::AID-CNE7>3.0.CO;2-S
Braak H., Braak E. (1982). Neuronal types in the claustrum of man. Anat. Embryol. 163, 447–460. doi: 10.1007/BF00305558
Brand S. (1981). A serial section Golgi analysis of the primate claustrum. Anat. Embryol. 162, 475–488. doi: 10.1007/BF00301872
Celio M. R. (1990). Calbindin D-28k and parvalbumin in the rat nervous system. Neuroscience 35, 375–475. doi: 10.1016/0306-4522(90)90091-H
Chevee M., Finkel E. A., Kim S. J., O’Connor D. H., Brown S. P. (2022). Neural activity in the mouse claustrum in a cross-modal sensory selection task. Neuron 110, 486–501 e7. doi: 10.1016/j.neuron.2021.11.013
Chia Z., Silberberg G., Augustine G. J. (2017). Functional properties, topological organization and sexual dimorphism of claustrum neurons projecting to anterior cingulate cortex. Claustrum 2, 1357412. doi: 10.1080/20023294.2017.1357412
Crick F. C., Koch C. (2005). What is the function of the claustrum? Philosophical transactions of the Royal Society of London. Series B. Biol. Sci. 360, 1271–1279. doi: 10.1098/rstb.2005.1661
Dávila J. C., Real M. Á., Olmos L., Legaz I., Medina L., Guirado S. (2005). Embryonic and postnatal development of GABA, calbindin, calretinin, and parvalbumin in the mouse claustral complex. J. Comp. Neurol. 481, 42–57. doi: 10.1002/cne.20347
Dehay C., Kennedy H., Kosik Kenneth S. (2015). The outer subventricular zone and primate-specific cortical complexification. Neuron 85, 683–694. doi: 10.1016/j.neuron.2014.12.060
Douglas R. J., Martin K. A. (2004). Neuronal circuits of the neocortex. Annu. Rev. Neurosci. 27, 419–451. doi: 10.1146/annurev.neuro.27.070203.144152
Douglas R. J., Martin K. A. C., Whitteridge D. (1989). A canonical microcircuit for neocortex. Neural Comput. 1, 480–488. doi: 10.1162/neco.1989.1.4.480
Druga R., Chen S., Bentivoglio M. (1993). Parvalbumin and calbindin in the rat claustrum: An immunocytochemical study combined with retrograde tracing from frontoparietal cortex. J. Chem. Neuroanat. 6, 399–406. doi: 10.1016/0891-0618(93)90014-U
Druga R., Salaj M., Barinka F., Edelstein L., Kubová H. (2015). Calretinin immunoreactivity in the claustrum of the rat. Front. Neuroanat. 8. doi: 10.3389/fnana.2014.00160
Feldblum S., Erlander M. G., Tobin A. J. (1993). Different distributions of GAD65 and GAD67 mRNAS suggest that the two glutamate decarboxylases play distinctive functional roles. J. Neurosci. Res. 34, 689–706. doi: 10.1002/jnr.490340612
Fodoulian L., Gschwend O., Huber C., Mutel S., Salazar R. F., Leone R., et al. (2020). The claustrum-medial prefrontal cortex network controls attentional set-shifting. bioRxiv, 339259. doi: 10.1101/2020.10.14.339259
Gattoni G., Bernocchi G. (2019). Calcium-binding proteins in the nervous system during hibernation: neuroprotective strategies in hypometabolic conditions? Int. J. Mol. Sci. doi: 10.3390/ijms20092364
Geisler S., Derst C., Veh R. W., Zahm D. S. (2007). Glutamatergic afferents of the ventral tegmental area in the rat. J. Neurosci. 27, 5730. doi: 10.1523/JNEUROSCI.0012-07.2007
Golgi C. (1873). Sulla struttura della sostanza grigia dell cervello’, Gazz. Med. Lombarda 33, 224–246.
Graf M., Nair A., Wong K. L. L., Tang Y., Augustine G. J. (2020). Identification of mouse claustral neuron types based on their intrinsic electrical properties. eneuro 7, ENEURO.0216–20.2020. doi: 10.1523/ENEURO.0216-20.2020
Grimstvedt J. S., Shelton A. M., Hoerder-Suabedissen A., Oliver D. K., Berndtsson C. H., Blankvoort S., et al. (2023). A multifaceted architectural framework of the mouse claustrum complex. J. Comp. Neurol. doi: 10.1002/cne.25539
Ham G. X., Augustine G. J. (2022). Topologically organized networks in the claustrum reflect functional modularization. Front. Neuroanat. 16, 901807. doi: 10.3389/fnana.2022.901807
Hawrylycz M., Miller J. A., Menon V., Feng D., Dolbeare T., Guillozet-Bongaarts A. L., et al. (2015). Canonical genetic signatures of the adult human brain. Nat. Neurosci. 18, 1832–1844. doi: 10.1038/nn.4171
Herzog E., Gilchrist J., Gras C., Muzerelle A., Ravassard P., Giros B., et al. (2004). Localization of VGLUT3, the vesicular glutamate transporter type 3, in the rat brain. Neuroscience 123, 983–1002. doi: 10.1016/j.neuroscience.2003.10.039
Hinova-Palova D. V., Edelstein L., Landzhov B. V., Braak E., Malinova L. G., Minkov M., et al. (2014). Parvalbumin-immunoreactive neurons in the human claustrum. Brain Struct. Funct. 219, 1813–1830. doi: 10.1007/s00429-013-0603-x
Hoerder-Suabedissen A., Ocana-Santero G., Draper T. H., Scott S. A., Kimani J. G., Shelton A. M., et al. (2022). Temporal origin of mouse claustrum and development of its cortical projections. Cereb. Cortex, bhac318. doi: 10.1101/2022.05.20.492804
Horikawa K., Armstrong W. E. (1988). A versatile means of intracellular labeling: injection of biocytin and its detection with avidin conjugates. J. Neurosci. Methods 25, 1–11. doi: 10.1016/0165-0270(88)90114-8
Hur E. E., Zaborszky L. (2005). Vglut2 afferents to the medial prefrontal and primary somatosensory cortices: A combined retrograde tracing in situ hybridization. J. Comp. Neurol. 483, 351–373. doi: 10.1002/cne.20444
Jackson J., Karnani M. M., Zemelman B. V., Burdakov D., Lee A. K. (2018). Inhibitory control of prefrontal cortex by the claustrum. Neuron 99 (5), 1029–39.e4. doi: 10.1016/j.neuron.2018.07.031
Jackson J., Smith J. B., Lee A. K. (2020). The anatomy and physiology of claustrum-cortex interactions. Annu. Rev. Neurosci. 43. doi: 10.1146/annurev-neuro-092519-101637
Jones E. G. (2001). The thalamic matrix and thalamocortical synchrony. Trends Neurosci. 24, 595–601. doi: 10.1016/S0166-2236(00)01922-6
Jun J. J., Steinmetz N. A., Siegle J. H., Denman D. J., Bauza M., Barbarits B., et al. (2017). Fully integrated silicon probes for high-density recording of neural activity. Nature 551, 232–236. doi: 10.1038/nature24636
Kim J., Matney C. J., Roth R. H., Brown S. P. (2016). Synaptic organization of the neuronal circuits of the claustrum. J. Neurosci. 36, 773. doi: 10.1523/JNEUROSCI.3643-15.2016
Kowiański P., Dziewiątkowski J., Kowiańska J., Moryś J. (1999). Comparative anatomy of the claustrum in selected species: a morphometric analysis. Brain Behav. Evol. 53, 44–54. doi: 10.1159/000006581
Kowiański P., Dziewiątkowski J., Moryś J. M., Majak K., Wójcik S., Edelstein L. R., et al. (2009). Colocalization of neuropeptides with calcium-binding proteins in the claustral interneurons during postnatal development of the rat. Brain Res. Bull. 80, 100–106. doi: 10.1016/j.brainresbull.2009.06.020
Kowiański P., Timmermans J.-P., Moryś J. (2001). Differentiation in the immunocytochemical features of intrinsic and cortically projecting neurons in the rat claustrum — combined immunocytochemical and axonal transport study. Brain Res. 905, 63–71. doi: 10.1016/S0006-8993(01)02408-8
Lapper S. R., Bolam J. P. (1991). The anterograde and retrograde transport of neurobiotin in the central nervous system of the rat: comparison with biocytin. J. Neurosci. Methods 39, 163–174. doi: 10.1016/0165-0270(91)90082-B
Laubach M., Amarante L. M., Swanson K., White S. R. (2018). What, if anything, is rodent prefrontal cortex? eneuro 5, ENEURO.0315–18.2018. doi: 10.1523/ENEURO.0315-18.2018
LeVay S., Sherk H. (1981a). The visual claustrum of the cat. I. Structure and connections. J. Neurosci. 1, 956. doi: 10.1523/JNEUROSCI.01-09-00956.1981
LeVay S., Sherk H. (1981b). The visual claustrum of the cat. II. The visual field map. J. Neurosci. 1, 981. doi: 10.1523/JNEUROSCI.01-09-00981.1981
Liguz-Lecznar M., Urban-Ciecko J., Kossut M. (2016). Somatostatin and somatostatin-containing neurons in shaping neuronal activity and plasticity. Front. Neural circuits 10. doi: 10.3389/fncir.2016.00048
Lübke J., Egger V., Sakmann B., Feldmeyer D. (2000). Columnar organization of dendrites and axons of single and synaptically coupled excitatory spiny neurons in layer 4 of the rat barrel cortex. J. Neurosci. 20, 5300. doi: 10.1523/JNEUROSCI.20-14-05300.2000
Luck S. J., Chelazzi L., Hillyard S. A., Desimone R. (1997). Neural mechanisms of spatial selective attention in areas V1, V2, and V4 of macaque visual cortex. J. Neurophysiol. 77, 24–42. doi: 10.1152/jn.1997.77.1.24
Lüth H. J., Winkelmann E., Celio M. R. (1993). Light- and electron microscopic localization of parvalbumin, calbindin D-28k and calretinin in the dorsal lateral geniculate nucleus of the rat. J. fur Hirnforschung 34, 47–56.
Ma G., Worthy K. H., Liu C., Rosa M. G. P., Atapour N. (2023). Parvalbumin as a neurochemical marker of the primate optic radiation. iScience 26, 106608. doi: 10.1016/j.isci.2023.106608
Madden M. B., Stewart B. W., White M. G., Krimmel S. R., Qadir H., Barrett F. S., et al. (2022). A role for the claustrum in cognitive control. Trends Cogn. Sci. 26, 1133–1152. doi: 10.1016/j.tics.2022.09.006
Marriott B. A., Do A. D., Zahacy R., Jackson J. (2020). Topographic gradients define the projection patterns of the claustrum core and shell in mice. J. Comp. Neurol 529 (7), 1607–1627. doi: 10.1101/2020.09.11.293381
Mathur B. N., Caprioli R. M., Deutch A. Y. (2009). Proteomic analysis illuminates a novel structural definition of the claustrum and insula. Cereb. Cortex 19, 2372–2379. doi: 10.1093/cercor/bhn253
McBride E. G., Gandhi S. R., Kuyat J. R., Ollerenshaw D. R., Arkhipov A., et al. (2023). Influence of claustrum on cortex varies by area, layer, and cell type. Neuron 111 (2), 275–290.e5. doi: 10.1016/j.neuron.2022.10.026
Michel M. C. (1991). Receptors for neuropeptide Y: multiple subtypes and multiple second messengers. Trends Pharmacol. Sci. 12, 389–394. doi: 10.1016/0165-6147(91)90610-5
Miller E. K., Erickson C. A., Desimone R. (1996). Neural mechanisms of visual working memory in prefrontal cortex of the macaque. J. Neurosci. 16, 5154. doi: 10.1523/JNEUROSCI.16-16-05154.1996
Minciacchi D., Granato A., Barbaresi P. (1991). Organization of claustro-cortical projections to the primary somatosensory area of primates. Brain Res. 553, 309–312. doi: 10.1016/0006-8993(91)90840-R
Miyashita T., Nishimura-Akiyoshi S., Itohara S., Rockland K. S. (2005). Strong expression of NETRIN-G2 in the monkey claustrum. Neuroscience 136, 487–496. doi: 10.1016/j.neuroscience.2005.08.025
Narikiyo K., Mizuguchi R., Ajima A., Shiozaki M., Hamanaka H., Johansen J. P., et al. (2020). The claustrum coordinates cortical slow-wave activity. Nat. Neurosci. 23, 741–753. doi: 10.1038/s41593-020-0625-7
Olson C. R., Graybiel A. M. (1980). Sensory maps in the claustrum of the cat. Nature 288, 479. doi: 10.1038/288479a0
Pathak S., Fernandez-Miranda J. C. (2014). “Chapter 7 - Structural and Functional Connectivity of the Claustrum in the Human Brain,” in The Claustrum. Eds. Smythies J. R., Edelstein L. R., Ramachandran V. S. (Academic Press, San Diego), 209–224.
Patru M. C., Reser D. H. (2015). A new perspective on delusional states - Evidence for claustrum involvement. Front. Psychiatry 6, 1–14. doi: 10.3389/fpsyt.2015.00158
Pearson R. C. A., Brodal P., Gatter K. C., Powell T. P. S. (1982). The organization of the connections between the cortex and the claustrum in the monkey. Brain Res. 234, 435–441. doi: 10.1016/0006-8993(82)90883-6
Pham X., Wright D. K., Atapour N., Chan J. M.-H., Watkins K. J., Worthy K. H., et al. (2019). Internal subdivisions of the marmoset claustrum complex: identification by myeloarchitectural features and high field strength imaging. Front. Neuroanat. 13, 96. doi: 10.3389/fnana.2019.00096
Pirone A., Castagna M., Granato A., Peruffo A., Quilici F., Cavicchioli L., et al. (2014). Expression of calcium-binding proteins and selected neuropeptides in the human, chimpanzee, and crab-eating macaque claustrum. Front. Syst. Neurosci. 8, 99. doi: 10.3389/fnsys.2014.00099
Prensa L., Richard S., Parent A. (2003). Chemical anatomy of the human ventral striatum and adjacent basal forebrain structures. J. Comp. Neurol. 460, 345–367. doi: 10.1002/cne.10627
Real M. Á., Dávila J. C., Guirado S. (2003). Expression of calcium-binding proteins in the mouse claustrum. J. Chem. Neuroanat. 25, 151–160. doi: 10.1016/S0891-0618(02)00104-7
Remedios R., Logothetis N. K., Kayser C. (2010). Unimodal responses prevail within the multisensory claustrum. J. Neurosci. 30, 12902. doi: 10.1523/JNEUROSCI.2937-10.2010
Remedios R., Logothetis N., Kayser C. (2014). A role of the claustrum in auditory scene analysis by reflecting sensory change. Front. Syst. Neurosci. 8. doi: 10.3389/fnsys.2014.00044
Reser D. H., Richardson K. E., Montibeller M. O., Zhao S., Chan J. M. H., Soares J. G., et al. (2014). Claustrum projections to prefrontal cortex in the capuchin monkey (Cebus apella). Front. Syst. Neurosci. 8, 123. doi: 10.3389/fnsys.2014.00123
Reynhout K., Baizer J. S. (1999). Immunoreactivity for calcium-binding proteins in the claustrum of the monkey. Anat. Embryol. 199, 75–83. doi: 10.1007/s004290050211
Rockland K. (2017). “White Matter Tracts Visualized by Parvalbumin in Nonhuman Primates,” in Primates. Eds. Mark B., Maurice P.
Royal D. W., Sáry G., Schall J. D., Casagrande V. A. (2006). Correlates of motor planning and postsaccadic fixation in the macaque monkey lateral geniculate nucleus. Exp. Brain Res. 168, 62–75. doi: 10.1007/s00221-005-0093-z
Sadowski M., Moryś J., Jakubowska-Sadowska K., Narkiewicz O. (1997). Rat’s claustrum shows two main cortico-related zones. Brain Res. 756, 147–152. doi: 10.1016/S0006-8993(97)00135-2
Shelton A. M., Oliver D. K., Grimstvedt J. S., Lazarte I. P., Kapoor I., Swann J. A., et al. (2022). Single neurons and networks in the claustrum integrate input from widespread cortical sources. bioRxiv, 490864. doi: 10.1101/2022.05.06.490864
Sherk H., LeVay S. (1981). The visual claustrum of the cat. III. Receptive field properties. J. Neurosci. 1, 993. doi: 10.1523/JNEUROSCI.01-09-00993.1981
Shibuya H., Yamamoto T. (1998). Electrophysiological and morphological features of rat claustral neurons: An intracellular staining study. Neuroscience 85, 1037–1049. doi: 10.1016/S0306-4522(97)00609-X
Shima K., Hoshi E., Tanji J. (1996). Neuronal activity in the claustrum of the monkey during performance of multiple movements. J. Neurophysiol. 76, 2115–2119. doi: 10.1152/jn.1996.76.3.2115
Smith J. B., Alloway K. D. (2010). Functional specificity of claustrum connections in the rat: interhemispheric communication between specific parts of motor cortex. J. Neurosci. 30, 16832. doi: 10.1523/JNEUROSCI.4438-10.2010
Smith J. B., Alloway K. D., Hof P. R., Orman R., Reser D. H., Watakabe A., et al. (2019). The relationship between the claustrum and endopiriform nucleus: A perspective towards consensus on cross-species homology. J. Comp. Neurol. 527, 476–499. doi: 10.1002/cne.24537
Smith Y., Parent A., Kerkérian L., Pelletier G. (1985). Distribution of neuropeptide Y immunoreactivity in the basal forebrain and upper brainstem of the squirrel monkey (saimiri sciureus). J. Comp. Neurol. 236, 71–89. doi: 10.1002/cne.902360107
Smythies J., Edelstein L., Ramachandran V. (2014). The Claustrum: Structural, Functional, and Clinical Neuroscience (Cambridge (US): Academic Press). doi: 10.1016/C2012-0-02175-8
Spahn B., Braak H. (1985). Percentage of projection neurons and various types of interneurons in the human claustrum. Acta Anat (Basel) 122, 245–248. doi: 10.1159/000146023
Takahashi M., Kobayashi T., Mizuma H., Yamauchi K., Okamoto S., Okamoto K., et al. (2023). Preferential arborization of dendrites and axons of parvalbumin- and somatostatin-positive GABAergic neurons within subregions of the mouse claustrum. Neurosci. Res. 190, 92–106. doi: 10.1016/j.neures.2022.11.008
Takamori S. (2006). VGLUTs: ‘Exciting’ times for glutamatergic research? Neurosci. Res. 55, 343–351. doi: 10.1016/j.neures.2006.04.016
Tkatch T., Rysevaite-Kyguoliene K., Sabeckis I., Sabeckiene D., Pauza D. H., Baranauskas G. (2022). An efficient rAAV vector for protein expression in cortical parvalbumin expressing interneurons. Sci. Rep. 12, 17851. doi: 10.1038/s41598-022-21867-0
Vidyasagar T. R., Levichkina E. (2019). An integrated neuronal model of claustral function in timing the synchrony between cortical areas. Front. Neural circuits 13. doi: 10.3389/fncir.2019.00003
Wada J. A., Tsuchimochi H. (1997). Role of the claustrum in convulsive evolution of visual afferent and partial nonconvulsive seizure in primates. Epilepsia 38, 897–906. doi: 10.1111/j.1528-1157.1997.tb01255.x
Wang Q., Wang Y., Kuo H.-C., Xie P., Kuang X., Hirokawa K.E., et al. (2023). Regional and cell-type-specific afferent and efferent projections of the mouse claustrum. Cell Rep. 42, 112118. doi: 10.1016/j.celrep.2023.112118
Watakabe A., Ohsawa S., Ichinohe N., Rockland K. S., Yamamori T. (2014). Characterization of claustral neurons by comparative gene expression profiling and dye-injection analyses. Front. Syst. Neurosci. 8. doi: 10.3389/fnsys.2014.00098
Watson C., Puelles L. (2017). Developmental gene expression in the mouse clarifies the organization of the claustrum and related endopiriform nuclei. J. Comp. Neurol. 525, 1499–1508. doi: 10.1002/cne.24034
Watson G. D. R., Smith J. B., Alloway K. D. (2017). Interhemispheric connections between the infralimbic and entorhinal cortices: The endopiriform nucleus has limbic connections that parallel the sensory and motor connections of the claustrum. J. Comp. Neurol. 525, 1363–1380. doi: 10.1002/cne.23981
White M. G., Mathur B. N. (2018). Claustrum circuit components for top–down input processing and cortical broadcast. Brain Struct. Funct. 223, 3945–3958. doi: 10.1007/s00429-018-1731-0
Wonders C. P., Anderson S. A. (2006). The origin and specification of cortical interneurons. Nat. Rev. Neurosci. 7, 687–696. doi: 10.1038/nrn1954
Wong K. L. L., Nair A., Augustine G. J. (2021). Changing the cortical conductor’s tempo: neuromodulation of the claustrum. Front. Neural circuits 15, 658228. doi: 10.3389/fncir.2021.658228
Keywords: claustrum, human, primates, rodents, excitatory, inhibitory, calcium binding proteins, peptides
Citation: Chong MHY and Gămănuţ R (2024) Anatomical and physiological characteristics of claustrum neurons in primates and rodents. Front. Mamm. Sci. 3:1309665. doi: 10.3389/fmamm.2024.1309665
Received: 08 October 2023; Accepted: 30 January 2024;
Published: 16 February 2024.
Edited by:
Ruth Benavides-Piccione, Spanish National Research Council (CSIC), SpainReviewed by:
Alvaro Duque, Yale University, United StatesCopyright © 2024 Chong and Gămănuţ. This is an open-access article distributed under the terms of the Creative Commons Attribution License (CC BY). The use, distribution or reproduction in other forums is permitted, provided the original author(s) and the copyright owner(s) are credited and that the original publication in this journal is cited, in accordance with accepted academic practice. No use, distribution or reproduction is permitted which does not comply with these terms.
*Correspondence: Răzvan Gămănuţ, cmF6dmFuLmdhbWFudXRAbW9uYXNoLmVkdQ==
Disclaimer: All claims expressed in this article are solely those of the authors and do not necessarily represent those of their affiliated organizations, or those of the publisher, the editors and the reviewers. Any product that may be evaluated in this article or claim that may be made by its manufacturer is not guaranteed or endorsed by the publisher.
Research integrity at Frontiers
Learn more about the work of our research integrity team to safeguard the quality of each article we publish.