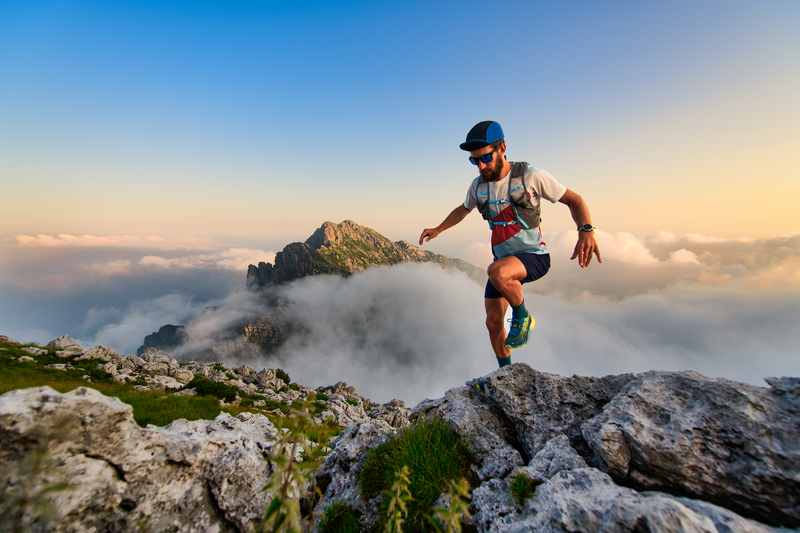
95% of researchers rate our articles as excellent or good
Learn more about the work of our research integrity team to safeguard the quality of each article we publish.
Find out more
ORIGINAL RESEARCH article
Front. Malar. , 28 March 2024
Sec. Pathogenesis
Volume 2 - 2024 | https://doi.org/10.3389/fmala.2024.1362644
This article is part of the Research Topic Women in Malaria Research View all 14 articles
The Plasmodium falciparum parasite infected more than 240 million people and killed around 600,000 patients last year alone. A key aspect of the pathophysiology of P. falciparum is the increased rigidity and adhesiveness of the membrane of infected red blood cells (iRBC). Optical tweezers (OT) have been proposed as a tool to evaluate and screen potential drugs because they can provide valuable information to determine a drug’s mechanism of action. The OT experimental design of this study was used to compare the plasma membrane stiffness of uninfected RBCs (uRBCs) and iRBCs, showing that the iRBCs were four times more rigid. The increased rigidity was more evident in those RBCs infected by the P. falciparum schizont stage. We also characterized the membrane deformability of iRBCs in vitro under the active concentration of common antimalarials on drug-resistant and non-drug-resistant P. falciparum strains. In addition, we also determined that the increased membrane rigidity of uRBCs during P. falciparum infection, known as the bystander effect, is partially reversed by antimalarial drugs. These findings suggest that single-cell mechanical measurements have potential uses in personalized medicine by characterizing the response to malaria treatment.
Malaria remains the most severe and widespread parasitic disease in the world. Over the centuries, it has spread to 91 countries, costing millions of lives. According to the WHO, in 2022, there were an estimated 249 million malaria cases worldwide. The estimated number of malaria deaths in 2022 stood at 608,000 (World malaria report, 2023). Despite this alarming scenario, malaria is a preventable and curable disease.
The capacity of red blood cells (RBCs) to deform is essential to pass through tiny capillaries and fulfill the role of oxygen exchange in organisms (Diez-Silva et al., 2010).
The asexual stages of P. falciparum generate significant pathophysiological effects after invading RBCs in the bloodstream, particularly the host cells’ mechanical properties. Previously, it has been reported that P. falciparum provokes modifications in the RBC plasma membrane by increasing the rigidity and cytoadherence. This last modification is thought to prevent the splenic clearance of the rigid RBCs (Maier et al., 2008; Batchelor et al., 2014; Nureye and Assefa, 2020). Therefore, there is an increased interest in studying the characterization of cell deformability to identify unknown biological features and mechanisms during malaria infection. Optical tweezers (OT) is one of the tools used to characterize significant increments in membrane stiffness in both uRBCs and P. falciparum iRBCs in vitro and in vivo. Additionally, this technology has allowed for the identification of the bystander effect, characterized by the presence of a more rigid uRBC after incubation with P. falciparum iRBCs (Paul et al., 2013). Experimental data suggest that ATP and cAMP could mediate this bystander effect (Paul et al., 2017).
The increased rigidity in the host plasma membrane appears to be dependent on the parasite species and pathobiology—for instance, increased membrane rigidity has also been observed in Plasmodium knowlesi infection, causing a similar capillary obstruction to that provoked by P. falciparum infection (Miller et al., 1971). The bystander effect was also identified in non-infected cells from patients infected with P. vivax, showing a similar outcome to that observed in P. falciparum cultures (Huang et al., 2013; Deng et al., 2015). Nevertheless, different studies have shown discrepancies between P. vivax and infected host cell deformability data. Initially, both Handayani et al. (2008) and Malleret et al. (2015) reported an increment in the deformation capacity of infected reticulocytes. Other studies identified an increment in the corner frequency of the circulating RBCs from human patients, meaning that host cells became more rigid during infection. However, they clarified that the study did not consider whether the analyzed RBCs were infected or not nor the maturity of the erythrocytes (Jayavanth et al., 2004; Zhang et al., 2016).
On the other hand, two different microfluidic devices used by Huang et al. and Deng et al. showed that P. falciparum iRBCs that were exposed to cytotoxic drugs often trigger a dynamic process of cytoskeleton reorganization, affecting the RBC mechanical properties (Huang et al., 2013; Deng et al., 2015). Few studies have evaluated the effect of drugs using OT technology to identify the structural changes or mechanisms of action against malaria parasites. Therefore, we here present the utilization of OT to study in vitro the deformability of P. falciparum iRBCs treated with the most common antimalarials. In addition, we also examine the bystander and the drug effects on uRBCs in vitro.
A commercial OT device measured the plasma membrane rigidity in uRBCs and synchronized iRBCs in the ring and schizont stages of P. falciparum. These measurements were based on the mean of the cell membrane spring constant (K), defined as the relation between the force applied to the cell membrane and the resulting cell elongation.
Our initial results showed that the stretching force applied to iRBCs increases proportionally to the parasite stage. Consequently, as parasites grow within RBCs, more force is needed to elongate them to the same degree as uRBCs or iRBCs in the early stages. The OT measurement showed that changes in cell elongation of uRBCs by forces as small as 15 pN could be detected. However, iRBCs in the ring and schizont stages required greater force magnitudes to reach similar deformation distances at 50 and 80 pN, respectively (Figure 1).
Figure 1 Optical images of (A) uRBCs at 0, 15.5 39.4 and 102.1pN of tensile forces, (B) ring stage at 0, 17, 51 and 65pN of tensile forces and (C) schizont stage at 0, 14.7, 54.2 and 81.3 pN of tensile forces by optical tweezers. Red arrows show the laser position in the optical trap at the elongation moment.
On the other hand, the average spring constant for uRBCs was 20.65pN/μm, while iRBCs in the ring and schizont stages were 39.46 and 82.33pN/μm, respectively (Figure 2). These results also showed a significant stage-dependent iRBC rigidification in the schizont stage (p =<0.0001) and in the ring stage (p = 0.0428) in comparison with uRBCs (Table 1). The values for uRBCs were in accordance with previous reports using OT (Dao et al., 2003; Yoon et al., 2008).
Figure 2 Spring constant (K) of iRBCs in each parasite developmental stage. Each point represents the spring constant (K) for one individual cell. One-way ANOVA. *p = 0.0428, ****p< 0.0001.
Table 1 Individual stiffness values of HB3 P. falciparum in different parasite stages (ring and schizont) normalized to uRBC cell control.
The difference in RBC stiffness was also examined following treatment with standard drugs. We chose to use mefloquine (MQ), chloroquine (CQ), artemisinin (ART), and pyrimethamine (PYR) in a concentration four times greater than their established EC50 for 4 h (Table 2). For this study, uRBCs from the same donor were evaluated with each antimalarial compound to determine whether the observed effects were specific to iRBCs. Interestingly, no antimalarial drug significantly altered the uRBCs’ rigidity (Supplementary Figure S1).
Table 2 Drug concentrations and stiffness values of schizont-stage HB3 P. falciparum normalized to DMSO control in each anti-malarial drug treatment.
It is worth noting that MQ, CQ, and ART increased the rigidity of the plasma membrane of iRBCs with schizonts. Still only MQ (p = 0.03) and ART (p< 0.0001) showed significant differences in the spring constant compared to untreated controls (Figure 3, Table 1). In contrast, PYR decreased stiffness by 7% (Figure 3, Table 1).
Figure 3 Purified trophozoites (iRBC) were incubated with various antimalarial compounds for 4 h. Each point represents the spring constant (K) for one trophozoite iRBC. A significant increase in K was observed with MQ and ART at 1 µM and 20 nM, respectively, compared to untreated DMSO control without drug (w/o drug). One-way ANOVA with multiple comparisons. ****p< 0.0001; *p = 0.0308.
We also tested the effect of CQ on synchronized iRBCs in the ring stage since CQ has the most antimalarial activity in this stage (Zhang et al., 1986). In this situation, membrane stiffness was also significantly augmented in comparison with the untreated control (p = 0.03, Figure 4).
Figure 4 (A) Purified trophozoites (iRBC) were incubated with CQ for 4 h. (B) Synchronized rings (iRBC) were incubated with CQ for 4 h. Each point represents the spring constant (K) for one iRBC. A significant increase in K was observed with CQ in the ring stage compared to untreated DMSO control without drug (w/o drug). T-test. *p = 0.0376.
Our initial data showed that PYR-resistant HB3 presented a 7% decrease in stiffness of the RBC plasma membrane. Previously, it was reported that the HB3 strain is susceptible to almost all antimalarial drugs except pyrimethamine (Nzila and Mwai, 2010). Therefore, we also assessed the rigidity of the cell membrane after treatment with PYR on the 3D7 strain, a P. falciparum strain reported to be susceptible to PYR. Remarkably, the membrane rigidity of RBCs in P. falciparum 3D7 samples treated with PYR was 26% higher than that of the untreated group (Figure 5).
Figure 5 Purified trophozoites (iRBC) from HB3 strain (A) and 3D7 strain (B) were incubated with PYR for 4 h. Each point represents the spring constant (K) for one iRBC. A significant increase in K was observed with PYR in the 3D7 strain compared to untreated DMSO control without drug (w/o drug). T-test. *p = 0.04.
Furthermore, we determined that changes in cell membrane rigidity depended on the strain’s sensitivity to drugs. In the case of the HB3 strain, with an experimental IC50 of 68.7 μM to PYR, no significant changes were detected in cell membrane rigidity, with a median deformability of 0.93. In contrast, the PYR-sensitive 3D7 strain (IC50 = 8 nM) showed a significantly increased stiffness after drug treatment with a median deformability of 1.26 and p = 0.04. Individual concentrations and stiffness values were normalized to DMSO control (Table 3).
Table 3 Stiffness value of the schizont stage of HB3 and 3D7 P. falciparum strains normalized to DMSO control in pyrimethamine treatment at 20 nM.
To assess the bystander effect, we first measured the stiffness of uRBCs from two independent in vitro cultures infected with HB3 and 3D7 strains, which were synchronized at the schizont stage. Then, we compared these measurements with a uRBC culture from the same donor but without the prior presence of parasites. We determined that the spring constant in uRBCs from HB3 and 3D7 cultures was 29% and 35% greater, respectively, than for those that had never been in contact with parasites (Figure 6, Table 4).
Figure 6 Bystander effect. (A) Spring constant (K) of uRBC from an in vitro culture infected with HB3 strain. (B) Spring constant (K) of uRBC from an in vitro culture infected with 3D7 strain. A uRBC culture from the same donor without previous parasite contact was used as control. The bars represent the mean ± SEM values. T-test. *p = 0.04.
Table 4 Individual stiffness value of uRBCs from two independent in vitro cultures infected with HB3 and 3D7 strains normalized to uRBC culture from the same donor without a previous parasite contact.
After that, we evaluated the effect of MQ, CQ, ART, and PYR in those uRBC cultures that were previously exposed to iRBCs in a standard in vitro culture. As a control, we measured the stiffness of uRBCs treated with the same drugs and cultured without P. falciparum parasites. The measurement by OT showed that the stiffness dropped by 18%, 28%, 25%, and 14% after being exposed to MQ, CQ, ART, and PYR, respectively, though only with statistical significance for CQ (p = 0.03) and ART (p = 0.0090) (Figure 7, Table 5).
Figure 7 Bystander effect in response to antimalarials. Spring constant (K) of uRBC from an in vitro culture infected with HB3 strain in response to treatment with antimalarials for 4 h. The bars represent mean ± SEM. One-way ANOVA with multiple comparisons. *p = 0.03; **p = 0.009.
Table 5 Individual stiffness value of uRBCs from in vitro culture infected with HB3 strain in response to antimalarial drugs.
The increment in P. falciparum iRBC membrane stiffness has been known for over 30 years (Paulitschke and Nash, 1993). In this study, we confirmed that plasma membrane rigidity increased fourfold in iRBCs, depending mainly on the parasite strain and drug sensitivity. Specifically, the stiffness caused by HB3 and 3D7 strains varied differentially after the selected antimalarials were applied to resistant and non-resistant strains.
Previously, Suresh et al. (2005) demonstrated that the stiffness of iRBCs increased 10-fold compared with uRBCs using OT. In our study, iRBCs exhibited a stiffness four times greater than that of uRBCs. This difference in magnitude between the two studies could have originated in the experimental approach, including the configuration of the OT, bead attachment solutions, and in vitro conditions. Nevertheless, both studies reached similar conclusions regarding the changes in RBC rigidity during different malaria parasite stages.
Our OT measurements suggest that the mechanical properties of the HB3 PYR-resistant strain were not affected upon treatment with PYR. However, the plasma membrane of iRBCs showed increased stiffness after using MQ, CQ, and ART on the same HB3 strain. The opposite effect was observed in the case of the PYR-sensitive 3D7 strain (Figure 5). It is worth noting that the antimalarials tested in this study are known to generate oxidative stress on parasites and host cells (Gunjan et al., 2016; Egwu et al., 2021; Wagner and Chitnis, 2023). Previous reports demonstrated that oxidative stress, such as lipid peroxidation, can modify the membrane structure and mechanical properties of living cells (Chng et al., 2021). Hence, we are tempted to point out that the increased rigidity of iRBCs is probably related to the induction of cell membrane lipid peroxidation. Nevertheless, testing other compounds that do not generate reactive oxygen species is necessary to discard this hypothesis.
We were also able to replicate the bystander effect observed in those uRBCs present in the same P. falciparum iRBC in vitro culture, showing that those uninfected cells became more rigid after being co-cultured with iRBCs. Notably, the tested antimalarial drugs partially reverted the bystander effect that previously increased the rigidity of uRBCs (Figure 7). This outcome could be part of a secondary unreported benefit of antimalarials on patients by avoiding the premature sequester of uRBCs in the spleen and the associated anemia. This bystander effect may represent a possible explanation for the origin of uRBC hemolysis, which exacerbates anemia during infection, as well as the susceptibility to clogging in arteries and capillaries (Dondorp et al., 1999). As the rigidity of uRBCs in this phenomenon is mediated primarily by ATP (Paul et al., 2019), it is plausible that a plasma membrane protein related to ATP could be responsible for the reversal of rigidity attributed to antimalarials. Although very interesting for further experimentation, it is out of the scope of the present work.
This study supports previous reports that identified a lower parasite invasion in patients with Dantu and beta-thalassemia phenotypes, which are known to manifest an irregular deformability of the RBC plasma membrane (Kariuki et al., 2020; Introini et al., 2022). Interestingly, a recent report revealed that the antimalarial compound M5717 significantly decreased iRBC rigidity and cytoadherence, provoking a parasite clearance delay in the blood torrent of mice and humans in preclinical and clinical trials. Therefore, we suggest that RBC rigidity may be used as an additional parameter to characterize and optimize drug activity screening before moving to in vivo models or preclinical trials (Parkyn Schneider et al., 2023). Furthermore, this type of information could also be used as a personalized medicine tool by characterizing associated changes in the rigidity of the cell membranes before administering a particular treatment (Di Carlo, 2012).
The malaria parasite strains HB3 (pyrimethamine-resistant) of P. falciparum (a generous gift of the Experimental Therapeutics Division of the Walter Reed Army Institute of Research in Silver Spring, MD) and 3D7 (pyrimethamine-sensitive) (ATCC) were cultivated following the method described by Haynes et al. (1976), with some modifications. In brief, we used O+ erythrocytes in a complete medium of RPMI 1640 supplemented with 25 mM HEPES, 0.2% sodium bicarbonate, and 10% human serum. All experiments obtained blood for RBC isolation from a single donor to avoid inter-donor variability. Before blood extraction, the volunteer was required to obtain medical certificates of good health free of medication regimes. The blood was drawn with citrate phosphate dextrose adenine (CPDA) as an anticoagulant at 10% v/v after extraction. The cultures were maintained at 37°C in a gas mixture of 5% CO2, 5% O2, and 90% N2 at 2% hematocrit and synchronized with alanine and a thermal cycling incubator as described in Almanza et al. (2011).
The configuration of the OT (MMI GmbH, Germany) was set on an inverted Olympus IX83 microscope with a ×100 oil immersion objective. The MBPS2 sensitive position detector and the MMI CellManipulator software were used to control the solid-state laser electronically with a wavelength of 1,070 nm at 8 W.
The optical tweezers must be calibrated each time before measuring the magnitude of the forces acting on the polystyrene particles. Optical stiffness, K, was determined by the oscillatory drag force method. For further measurements, forces were calculated for the optical tweezers using Hooke’s law:
where K is the optical stiffness of the trap, and x is the displacement from the trap’s center (Supplementary Figures S2, S3).
The K constant of each cell was calculated by data analysis through image processing using ImageJ software. The boundary of each cell was detected in each frame for the same group of images. The changes in cell length were used along with the restoring force and Hooke’s equation to calculate the K constant (Supplementary Figure S4).
RBC samples were prepared by taking previously processed RBCs. The RBCs were washed twice with PBS and re-suspended in PBS and ACD (citric acid, sodium citrate, and dextrose). Subsequently, polystyrene particles with a diameter of 3 μm, previously washed with PBS and left at 4°C for 1 h to obtain a better adherence of the particles to the RBC, were added. Then, polystyrene particles were used to deform the selected uRBC or iRBC. OT was used to manipulate the cell only when one bead was attached to the membrane’s edge, and the cell’s opposite side was attached to the glass plate, allowing stretching without shedding. The experiments were performed in the linear regime, as all deformation vs. force curves present this behavior. The cell also always recovered its initial shape after each stretching (Supplementary Figure S5).
Magnetic columns were used to enrich the population of late-stage parasites following the methodology of Coronado et al. (2013). The isolated parasites were washed twice with PBS and diluted in PBS and ACD. Polystyrene particles were added and left at 4°C for 1 h. Each sample was then visualized under the microscope, and OT stretched the cell.
In the case of the drug-treated group, the samples were incubated previously with concentrations of chloroquine (CQ) 1 μM, mefloquine (MQ) 1 μM, artemisinin (ART) 20 nM, and pyrimethamine at 20 nM for 4 h as described previously by Deng et al. (2015) (Supplementary Figure S6). The samples were incubated at 37°C. Posteriorly, polystyrene particles were added and incubated at 4°C for 1 h. The samples were then placed under the microscope, and the cell was stretched. We also evaluated the bystander effect with the drugs mentioned above using the same conditions and incubation time. For the bystander effect evaluation, uRBCs were in contact with parasites for 48 h prior to experimentation, and all assays were performed while the parasites in culture were in schizont stage.
Relative deformability, RD, expresses the deformability of the treated cells, with Dt normalized to the median deformability of cells in the DMSO control for a given experimental series, Dctrl, according to the following formula:
This procedure facilitates the assessment of the effects of treatment on deformability and is easily compared between experiments.
All experiments were independently repeated at least twice, and 15–20 cells were analyzed per sample. The dispersion graphs of all experiments are presented. Statistical analysis was calculated using Prism Gradpad v7 with a t-test when comparing one group to another. Multiple comparison analysis was performed with one-way ANOVA. Data were evaluated by analysis of variance, and significant differences between groups were determined using Bonferroni’s modification. The relationship between the force applied and the changes in RBC diameter was evaluated by linear regression to calculate the K spring constant. P< 0.05 was considered statistically significant. Significance was considered as follows: *p< 0.05; **p< 0.01; ***p< 0.005, ****p< 0.0001.
The original contributions presented in the study are included in the article/Supplementary Material. Further inquiries can be directed to the corresponding author.
The studies involving humans were approved by Bioethical committee of Universidad Católica Santa María La Antigua (USMA) in Panama with Number 2023-PO60. The studies were conducted in accordance with the local legislation and institutional requirements. The participants provided their written informed consent to participate in this study.
LC: Conceptualization, Data curation, Formal Analysis, Writing – review & editing, Writing – original draft, Visualization, Validation, Supervision, Software, Resources, Project administration, Methodology, Investigation, Funding acquisition. DD: Conceptualization, Formal Analysis, Investigation, Methodology, Validation, Visualization, Writing – original draft, Writing – review & editing. PP: Writing – review & editing, Writing – original draft, Methodology, Investigation, Formal Analysis. RC: Writing – review & editing, Writing – original draft, Supervision, Funding acquisition, Data curation, Conceptualization. LP: Writing – review & editing, Writing – original draft, Supervision, Investigation. CS: Funding acquisition, Methodology, Resources, Supervision, Writing – original draft, Writing – review & editing. ES-G: Conceptualization, Data curation, Formal Analysis, Methodology, Supervision, Validation, Visualization, Writing – original draft, Writing – review & editing.
The author(s) declare financial support was received for the research, authorship, and/or publication of this article. Secretaría Nacional de Ciencia, Tecnología e Innovación (SENACYT) grant FIED19-R1-007 (LC) and other partial funds from Panama’s National Research System (SNI) (LC, RC, and CS). Support also came from SENACYT reinsertion grants (LC and RC).
The authors declare that the research was conducted in the absence of any commercial or financial relationships that could be construed as a potential conflict of interest.
The author(s) declared that they were an editorial board member of Frontiers, at the time of submission. This had no impact on the peer review process and the final decision.
All claims expressed in this article are solely those of the authors and do not necessarily represent those of their affiliated organizations, or those of the publisher, the editors and the reviewers. Any product that may be evaluated in this article, or claim that may be made by its manufacturer, is not guaranteed or endorsed by the publisher.
The Supplementary Material for this article can be found online at: https://www.frontiersin.org/articles/10.3389/fmala.2024.1362644/full#supplementary-material
Almanza A., Herrera L., Tayler N., Coronado L., Spadafora C. (2011). Automated Synchronization of P. falciparum using a Temperature Cycling Incubator. Curr. Trends Biotechnol. Pharm. 5, 1130–1133.
Batchelor J. D., Malpede B. M., Omattage N. S., DeKoster G. T., Henzler-Wildman K. A., Tolia N. H. (2014). Red blood cell invasion by Plasmodium vivax: structural basis for DBP engagement of DARC. PloS Pathog. 10. doi: 10.1371/journal.ppat.1003869
Chng C.-P., Sadovsky Y., Hsia K. J., Huang C. (2021). Site-specific peroxidation modulates lipid bilayer mechanics. Extrem. Mech. Lett. 42, 101148. doi: 10.1016/j.eml.2020.101148
Coronado L. M., Tayler N. M., Correa R., Giovani R. M., Spadafora C. (2013). Separation of Plasmodium falciparum late stage-infected erythrocytes by magnetic means. J. Vis. Exp., e50342. doi: 10.3791/50342
Dao M., Lim C. T., Suresh S. (2003). Mechanics of the human red blood cell deformed by optical tweezers. J. Mech. Phys. Solids 51, 2259–2280. doi: 10.1016/j.jmps.2003.09.019
Deng X., Duffy S. P., Myrand-Lapierre M. E., Matthews K., Santoso A. T., Du Y. L., et al. (2015). Reduced deformability of parasitized red blood cells as a biomarker for anti-malarial drug efficacy. Malar. J. 14, 1–9. doi: 10.1186/s12936-015-0957-z
Di Carlo D. (2012). A mechanical biomarker of cell state in medicine. J. Lab. Autom. 17, 32–42. doi: 10.1177/2211068211431630
Diez-Silva M., Dao M., Han J., Lim C. T., Suresh S. (2010). Shape and biomechanical characteristics of human red blood cells in health and disease. MRS Bull. 35, 382–388. doi: 10.1557/mrs2010.571
Dondorp A. M., Angus B. J., Chotivanich K., Silamut K., Ruangveerayuth R., Hardeman M. R., et al. (1999). Red blood cell deformability as a predictor of anemia in severe falciparum malaria. Am. J. Trop. Med. Hyg. 60, 733–737. doi: 10.4269/ajtmh.1999.60.733
Egwu C. O., Tsamesidis I., Pério P., Augereau J.-M., Benoit-Vical F., Reybier K. (2021). Superoxide: A major role in the mechanism of action of essential antimalarial drugs. Free Radic. Biol. Med. 167, 271–275. doi: 10.1016/j.freeradbiomed.2021.03.001
Gunjan S., Singh S. K., Sharma T., Dwivedi H., Chauhan B. S., Imran Siddiqi M., et al. (2016). Mefloquine induces ROS mediated programmed cell death in malaria parasite: Plasmodium. Apoptosis 21, 955–964. doi: 10.1007/s10495-016-1265-y
Handayani S., Chiu D. T., Tjitra E., Kuo J. S., Lampah D., Kenangalem E., et al. (2008). High Deformability of Plasmodium vivax-Infected Red Blood Cells under Microfluidic Conditions. doi: 10.1086/596048
Haynes J. D., Diggs C. L., Hines F. A., Desjardins R. E. (1976). Culture of human malaria parasites Plasmodium falciparum. Nature 263, 767–769. doi: 10.1038/263767a0
Huang S., Undisz A., Diez-Silva M., Bow H., Dao M., Han J. (2013). Dynamic deformability of Plasmodium falciparum-infected erythrocytes exposed to artesunate in vitro. Integr. Biol. (Camb). 5, 414–422. doi: 10.1039/C2IB20161E
Introini V., Marin-Menendez A., Nettesheim G., Lin Y. C., Kariuki S. N., Smith A. L., et al. (2022). The erythrocyte membrane properties of beta thalassaemia heterozygotes and their consequences for Plasmodium falciparum invasion. Sci. Rep. 12. doi: 10.1038/S41598-022-12060-4
Jayavanth S., Jagadeesan K., Singh M. (2004). Influence of P. vivax malaria on erythrocyte aggregation and deformability. Clin. Hemorheol. Microcirc. 31, 257–266.
Kariuki S. N., Marin-Menendez A., Introini V., Ravenhill B. J., Lin Y. C., Macharia A., et al. (2020). Red blood cell tension protects against severe malaria in the Dantu blood group. Nature 585, 579–583. doi: 10.1038/s41586-020-2726-6
Maier A. G., Rug M., O’Neill M. T., Brown M., Chakravorty S., Szestak T., et al. (2008). Exported proteins required for virulence and rigidity of Plasmodium falciparum-infected human erythrocytes. Cell 134, 48–61. doi: 10.1016/j.cell.2008.04.051
Malleret B., Li A., Zhang R., Tan K. S. W., Suwanarusk R., Claser C., et al. (2015). Plasmodium vivax: restricted tropism and rapid remodeling of CD71-positive reticulocytes. Blood 125, 1314–1324. doi: 10.1182/blood-2014-08-596015
Miller L. H., Usami S., Chien S. (1971). Alteration in the rheologic properties of Plasmodium knowlesi–infected red cells. A possible mechanism for capillary obstruction. J. Clin. Invest. 50, 1451–1455. doi: 10.1172/JCI106629
Nureye D., Assefa S. (2020). Old and recent advances in life cycle, pathogenesis, diagnosis, prevention, and treatment of malaria including perspectives in Ethiopia. Sci. World J. 2020. doi: 10.1155/2020/1295381
Nzila A., Mwai L. (2010). In vitro selection of Plasmodium falciparum drug-resistant parasite lines. J. Antimicrob. Chemother. 65, 390. doi: 10.1093/jac/dkp449
Parkyn Schneider M., Looker O., Rebelo M., Khoury D. S., Dixon M. W. A., Oeuvray C., et al. (2023). The delayed bloodstream clearance of Plasmodium falciparum parasites after M5717 treatment is attributable to the inability to modify their red blood cell hosts. Front. Cell. Infect. Microbiol. 13. doi: 10.3389/FCIMB.2023.1211613/BIBTEX
Paul A., Padmapriya P., Natarajan V. (2017). Diagnosis of malarial infection using change in properties of optically trapped red blood cells. Biomed. J. 40, 101–105. doi: 10.1016/j.bj.2016.10.001
Paul A., Pallavi R., Tatu U. S., Natarajan V. (2013). The bystander effect in optically trapped red blood cells due to Plasmodium falciparum infection. Trans. R. Soc Trop. Med. Hyg. 107, 220–223. doi: 10.1093/trstmh/trt010
Paul A., Ramdani G., Tatu U., Langsley G., Natarajan V. (2019). Studying the rigidity of red blood cells induced by Plasmodium falciparum infection. Sci. Rep. 9. doi: 10.1038/S41598-019-42721-W
Paulitschke M., Nash G. B. (1993). Membrane rigidity of red blood cells parasitized by different strains of Plasmodium falciparum. J. Lab. Clin. Med. 122, 581–589.
Suresh S., Spatz J., Mills J. P., Micoulet A., Dao M., Lim C. T., et al. (2005). Connections between single-cell biomechanics and human disease states: gastrointestinal cancer and malaria. Acta Biomater. 1, 15–30. doi: 10.1016/j.actbio.2004.09.001
Wagner M. P., Chitnis C. E. (2023). Lipid peroxidation and its repair in malaria parasites. Trends Parasitol. 39, 200–211. doi: 10.1016/j.pt.2022.12.006
World malaria report (2023). Available online at: https://www.who.int/publications/i/item/9789240086173 (Accessed December 4, 2023).
Yoon Y. Z., Kotar J., Yoon G., Cicuta P. (2008). The nonlinear mechanical response of the red blood cell. Phys. Biol. 5. doi: 10.1088/1478-3975/5/3/036007
Zhang Y., Asante K. S. O., Jung A. (1986). Stage-dependent inhibition of chloroquine on Plasmodium falciparum in vitro. J. Parasitol. 72, 830–836. doi: 10.2307/3281830
Keywords: malaria, Plasmodium falciparum, optical tweezers, spring constant (K), rigidity, cell membrane, antimalarials
Citation: Dorta D, Padmore P, Correa R, Pineda L, Spadafora C, Sarmiento-Gómez E and Coronado LM (2024) Optical tweezers to measure the elasticity of red blood cells: a tool to study the erythrocyte response to antimalarials. Front. Malar. 2:1362644. doi: 10.3389/fmala.2024.1362644
Received: 28 December 2023; Accepted: 01 March 2024;
Published: 28 March 2024.
Edited by:
Alena Pance, University of Hertfordshire, United KingdomReviewed by:
Viola Introini, European Molecular Biology Laboratory, SpainCopyright © 2024 Dorta, Padmore, Correa, Pineda, Spadafora, Sarmiento-Gómez and Coronado. This is an open-access article distributed under the terms of the Creative Commons Attribution License (CC BY). The use, distribution or reproduction in other forums is permitted, provided the original author(s) and the copyright owner(s) are credited and that the original publication in this journal is cited, in accordance with accepted academic practice. No use, distribution or reproduction is permitted which does not comply with these terms.
*Correspondence: Lorena M. Coronado, bGNvcm9uYWRvQGluZGljYXNhdC5vcmcucGE=
Disclaimer: All claims expressed in this article are solely those of the authors and do not necessarily represent those of their affiliated organizations, or those of the publisher, the editors and the reviewers. Any product that may be evaluated in this article or claim that may be made by its manufacturer is not guaranteed or endorsed by the publisher.
Research integrity at Frontiers
Learn more about the work of our research integrity team to safeguard the quality of each article we publish.