- Department of Physiology and Cellular Biophysics Columbia University Irving Medical Center, New York, NY, United States
With more than 3,000 species and an almost ubiquitous presence, the economic importance of mosquitoes cannot be overemphasized. Anopheles mosquitoes are vectors for infectious diseases such as malaria – an endemic disease in tropical and sub-tropical regions of the world that infects more than 200 million people worldwide and causes over 400,000 deaths annually, with most casualties being infants or inhabitants of sub-Saharan Africa. The Aedes aegytpi and Culex quinquefasciatus species of mosquitoes are also vectors for arboviruses such as chikungunya virus, dengue virus, western equine encephalitis virus, and Zika virus. Consequently, insecticides are frequently used to stem the population of mosquitoes. Nevertheless, mosquito insecticide resistance has emerged as a major problem that has contributed to numerous failed eradication campaigns for the aforementioned diseases. In this mini-review, we expound on how fruit flies (Drosophila melanogaster) could be a complementary model system for studying mosquito insecticide resistance, with the ultimate goal of confirming any promising leads in mosquitoes.
Introduction
Malaria is a blood-borne disease caused by various plasmodium species that live within erythrocytes. However, while malaria can, on rare occasions, be transmitted via blood transfusions or congenital transmission, it is predominantly transmitted through the bite of a female mosquito of the Anopheles genus. Consequently, vector control strategies have long been a major component of attempts to limit the spread of infectious diseases transmitted by mosquitoes, such as malaria. This proved to be somewhat successful between 2000 and 2015, as the number of people infected with malaria steadily dropped, due at least in part to the use of insecticide-treated bed nets. However, over the past few years, mosquito insecticide resistance has become increasingly evident. Here, we briefly explore the major insecticide resistance mechanisms found in mosquitoes and discuss how we think studies in fruit flies can improve our understanding of mosquito insecticide resistance and contribute to the identification of new targets for insecticides. Thus, the rationale for this mini-review is not to provide a thorough understanding of the subject of mosquito insecticide resistance, but rather to provide an overview of the research themes in the field to Drosophila melanogaster (Dm) biologists, and briefly draw attention to how Dm genetics and cell biology can be co-opted to address questions relevant to mosquito insecticide resistance. For more thorough discussions of the subject of mosquito insecticide resistance, we refer readers to a number of reviews that have been published in recent years on this subject (Dusfour et al., 2019; Susanna and Pratiwi, 2021; Suh et al., 2023).
Types of insecticide resistance
Insecticide resistance usually develops gradually, as a result of several mechanisms that culminate in reducing the effectiveness of the insecticide. Nevertheless, there are four main insecticide resistance mechanisms referred to as target-site resistance, metabolic resistance, cuticular resistance and behavioral resistance; and many mosquito species can develop resistance through multiple mechanisms. We begin by briefly reviewing each type of insecticide resistance mechanism.
Target-site resistance
Target-site resistance develops when a mutation (usually a point mutation) develops in a specific insecticide target in a few flies that renders them refractory to the insecticide. This may arise when the mutation renders the molecular target incapable of binding to the insecticide to inhibit its activity, or the molecular target’s ability to engage and activate or inhibit downstream signaling cascades is impaired. This leads to a selective advantage for this initial small population of mosquitoes, eventually culminating in the whole population becoming largely resistant to the insecticide. Target-site resistance has been observed for insecticides that target genes encoding for the voltage-gated sodium channel (VGSC), acetylcholinesterase (AChE) and the γ-aminobutyric acid (GABA) receptor, Rdl (Resistance to dieldrin) (Hemingway et al., 2004).
Two classes of insecticides – pyrethroids and dichlorodiphenyltrichloroethane (DDT) – inhibit VGSCs. Pyrethroids modify the gating kinetics of VGSCs by inhibiting both activation and inactivation of the channel. Several single or multiple amino acid substitutions in the VGSC, such as the I1011M/V, L1014C/F/S/W and V1016G/I substitutions, block or at least reduce the affinity of the insecticide for the channel (Martinez-Torres et al., 1998; Brengues et al., 2003; Enayati et al., 2003; Chang et al., 2009).
AChE, the enzyme that catalyzes the hydrolysis of acetylcholine (ACh) in the nervous system, is the target site for organophosphate and carbamate-based insecticides [reviewed in (Fournier and Mutero, 1994)]. Organophosphate and carbamates have similar structures to ACh, as a result, they act as competitive inhibitors at the AChE active site. Biochemical assays in many mosquito species have revealed that the gene encoding AChE is a mutational hotspot. Relatively common single amino acid substitutions in many mosquito species that render the enzyme resistant to insecticides are the G119S, F290V and F331W substitutions (Weill et al., 2002; Weill et al., 2003; Alout et al., 2007a; Alout et al., 2007b; Djogbénou et al., 2008).
Cyclodiene insecticides, such as dieldrin, and phenyl pyrazones, such as fipronil, target the GABA receptor, Rdl, a gated chloride ion channel composed of five subunits [reviewed in (Kim and Hibbs, 2021)]. Dieldrin resistance has been linked to an A296S/G substitution found in many Anopheles species (Du et al., 2005; Wondji et al., 2011). Resistance to fipronil has also been reported in mosquitoes; however, the molecular lesion(s) responsible for it remain unclear (Liu et al., 2004).
Metabolic resistance
Metabolic resistance is the most common form of insecticide resistance and arises as a result of increased biodegradation of insecticides due to the activation of stress response pathways. It typically involves upregulation of glutathione-S-transferases (GSTs), cytochrome P450 monooxygenases (P450 enzymes), and esterases – a heterogeneous group of enzymes that typically includes carboxylesterase and cholinesterases (Salinas and Wong, 1999; Enayati et al., 2005; Liu, 2015). GSTs catalyze the conjugation of electrophilic compounds with reduced glutathione (GSH), which renders the resulting product less toxic and more hydrophilic, and thus more easily excreted than the hitherto unconjugated product (Habig et al., 1974). Some GSTs are also capable of catalyzing a dehydrochlorination reaction using GSH as a cofactor; and this appears to be the predominant mechanism by which resistance to DDT occurs (Clark and Shamaan, 1984). Nevertheless, in many other instances where elevated GST activity has been linked to insecticide resistance, the actual GST enzyme that confers resistance to the insecticide is unknown. This is partly due to the fact that the repertoire of GSTs in insects has rapidly expanded (many mosquito species have about 30 GST genes) as a result of gene duplications in the insect-specific delta and epsilon GSTs; and multiple delta- or epsilon-type GSTs can be overexpressed in a DDT-resistant strain such as Anopheles gambiae (Ortelli et al., 2003).
Similarly, genes encoding for esterases and P450 enzymes in insects can sometimes exceed 50 and 100, respectively, as amplification and duplication events have greatly increased their number. This has led to challenges in elucidating which P450 genes are required for insecticide resistance, as multiple P450 genes are typically induced as a result of exposing mosquitoes to insecticides. Further, the three major classes of enzymes linked to metabolic resistance are likely to act in concert, as the co-elevation of P450 enzymes and esterases in response to insecticide treatment has been reported (Vulule et al., 1999). In line with this, the mosquito P450 enzyme, CYP6Z8, metabolizes common pyrethroid metabolites produced by carboxylesterases such as 3-phenoxybenzoic alcohol and 3-phenoxybenzaldehyde, to 3-phenoxybenzoic acid and other more soluble derivatives (Chandor-Proust et al., 2013).
Cuticular resistance
Cuticular resistance arises from a thickening or alteration of the cuticle that reduces its permeability to the insecticide. Many cuticle proteins belonging to different protein families are expressed in various mosquito species (Zhou D. et al., 2017; Zhou Y. et al., 2017). In this regard, it has been shown that transcripts of the cuticle proteins, CPR63 and CPR47, are induced in pyrethroid-resistant strains of Culex pipiens pallens species than in non-resistant strains (Sun et al., 2017). Further studies showed that CPR63 might contribute to pyrethroid resistance by thickening the cuticle and thus, possibly, increasing the tolerance of mosquitoes to deltamethrin (Xu et al., 2022). Other cuticle proteins expressed at higher levels in pyrethroid-resistant relative to susceptible mosquito strains are CPR124, CPR127, CPR129 and CPR131 (Nkya et al., 2014; Vannini et al., 2014; Yahouédo et al., 2017; Huang et al., 2018). Lastly, CPLCG3, CPLCG4 and CPLCG5 are essential for cuticular resistance as they are involved in a putative cuticle thickening process (Vannini et al., 2014; Huang et al., 2018; Yahouédo et al., 2017).
Behavioral resistance
Perhaps the most intractable of all the insecticide resistance mechanisms is behavioral resistance, which, as the name suggests, refers to behavioral changes evoked by insects as a means to avoid further exposure to the insecticide. Forms of behavioral resistance include a reduction in the extent of mosquito entry into homes, an elevated rate of exit from the area sprayed with the insecticide, and a change in feeding times. In Tanzania, an increased proportion of Anopheles gambiae and Anopheles fenestus mosquitoes have been reported to feed outdoors as a result of the use of insecticide-resistant bed nets (Russell et al., 2011). Additionally, the introduction of indoor vector control in Bioko Island, Equatorial Guinea, led to an increase in the proportion of Anopheles gambiae mosquitoes that sought human hosts outdoors (Reddy et al., 2011).
Using fruit flies to gain a better understanding of target-site resistance
While the ideal model system for studying mosquito insecticide resistance and vector control are mosquitoes themselves, several reports lend credence to the proposition that Dm are also useful in studying the effects of mutations linked to insecticide resistance in mosquitoes. The well-developed genetic toolkit in Dm, make them an ideal insect model to first identify a broad class of genes that regulate a process with implications for developing insecticides to mosquitoes. Subsequently, more focused studies in mosquitoes examining multiple genes in the same class of genes, may identify the specific genes that regulate the process in mosquitoes. With regard to target-site resistance, both synonymous and nonsynonymous mutations in VGSCs have been associated with target-site resistance; but, in general, the significance of synonymous mutations are unclear. Previously assumed to be benign, growing evidence indicates that synonymous mutations can influence phenotypes by regulating gene expression and/or protein stability via an alteration in mRNA stability, a disruption of splicing activity, a change in the efficiency of miRNA binding, a perturbation of translational activity, and an interference with the function of long non-coding RNAs. Studies in Dm can test the ability of synonymous mutations in VGSCs to impact these cellular processes.
A gene that encodes for a VGSC in Dm is paralytic (para). This gene can encode for about 60 isoforms as a result of alternative splicing (Lin et al., 2009; Lin et al., 2012). As there are varied periods and patterns of expression of some of these isoforms, it would be interesting to determine how knock-in mutations in fruit flies that mimic the relevant synonymous mutations in mosquitoes regulate expression of these isoforms. Further, a Dm strain in which Para is tagged with GFP endogenously exists (Ravenscroft et al., 2020). The relevant knock-in mutations can be created in this strain to ascertain how Para localization and/or stability is affected. Lastly, the GAL4/UAS system can be exploited to overexpress constructs carrying the appropriate mutations (Fischer et al., 1988; Brand and Perrimon, 1993). Similar analyses can be performed for GABAR and AChE.
Fruit flies can also be used to gain a better understanding of how allelic drive can be used to reverse insecticide resistance. In a particularly noteworthy study, CRISPR/Cas9 gene editing of para was used to generate a series of common VGSC mutations found in mosquitoes and tested for susceptibility to various insecticides (Kaduskar et al., 2022). The study revealed that it was possible to replace the resistant allele with a native (susceptible) allele in population cages. Thus, this proof-of-principle study highlights how fruit flies can be employed to elucidate the potential of performing a targeted reversion of an insecticide-resistant strain to a wild-type susceptible state.
Using fruit flies to gain a better understanding of metabolic resistance
Forced expression of the mosquito P450 enzymes, CYP6P9a and CYP6P9b, in Dm conferred tolerance to both permethrin and deltamethrin (Riveron et al., 2013). Nevertheless, some discrepancies have been observed between data obtained from overexpressing detoxification genes in Dm and Anopheles gambiae mosquitoes. In such instances, the natural tendency is to dismiss the observations in Dm. However, the interpretation of such results is complicated by the fact that the outcome of a GAL4/UAS overexpression experiment is largely dependent on the extent and domain of overexpression. Even in fruit flies, where the GAL4/UAS toolkit is well-established, contradictory results can be obtained when the same gene is overexpressed in a specific Dm tissue using different tissue-specific GAL4 lines. Therefore, it appears the yardstick for success when overexpressing detoxification genes should be whether a protective effect against insecticides can be observed at all, as a result of using many GAL4 lines – both tissue-specific and ubiquitous. In this regard, the Gene-Switch system in Dm may be particularly valuable as it allows a dose-dependent expression of a transgene in response to varying concentrations of the RU486 (mifepristone) drug (Roman et al., 2001; McGuire et al., 2004; Nicholson et al., 2008; Ke and Hsu, 2019). While some studies in which a mosquito cDNA was overexpressed in Dm to test the effect of an insecticide have yielded promising results (Pavlidi et al., 2012; Riveron et al., 2013), it is possible that mosquito cDNAs may code for proteins that are incapable of interacting optimally with other endogenous regulatory factors. Hence, when overexpression of a mosquito cDNA fails to rescue Dm from insecticide-induced toxicity, it may be necessary to also test the effect of overexpressing the closest fruit fly ortholog of the mosquito gene. Ultimately, however, any promising results in fruit flies will have to be confirmed in mosquitoes.
Several peptidases, regulators of lipid and carbohydrate metabolism, sodium-calcium exchangers, and signaling molecules are induced alongside GSTs, P450 genes, and esterases in insecticide-resistant strains (Vontas et al., 2005; Liu et al., 2007). A relatively less explored aspect of metabolic insecticide resistance are the signaling pathways that regulate expression of what we refer to as insecticide resistance effector molecules (i.e., GSTs, P450 genes, and esterases). Identifying upstream regulators of these effector molecules is crucial, as it will furnish knowledge about the possibility of dismantling metabolic resistance mechanisms. A breakdown of the metabolic resistance mechanism should, in principle, enable an erstwhile resistant insecticide to be effective again. In one informative study, it was shown that GPCR signaling upregulates the expression of some P450 genes, raising the possibility that inhibitors of this specific GPCR signaling cascade may suppress insecticide resistance (Li et al., 2014). The readily available transgenic RNAi strains and sophisticated genetic tools in Dm make it particularly suited for exploring the regulatory relationships between the multiple genes induced in insecticide-resistant strains.
When mitochondrial function is impaired, it results in the activation of a mitochondrial stress signaling cascade that culminates in the induction of genes required for conferring tolerance to mitochondrial distress. As an example, we recently showed that RNAi-mediated knockdown of isocitrate dehydrogenase 2 (IDH2) leads to an upregulation of genes involved in Fe-S cluster biogenesis, redox and protein homeostasis, GSTs, P450 enzymes, esterases, regulators of lipid and carbohydrate metabolism, transporters, and signaling molecules, among other genes (Murari et al., 2022). This highlights the remarkable similarity in gene expression profile resulting from activating mitochondrial stress signaling or insecticide resistance. Further, it is widely accepted that a combination of piperonyl butoxide (PBO) and pyrethroids is more effective at reducing mosquito populations than using pyrethroids alone (Gleave et al., 2021). As PBO inhibits P450 enzymes, this observation raises the possibility that knocking down genes induced as a result of insecticide resistance may enhance the insecticidal effect of an ineffective insecticide. This is a phenomenon referred to as synergism. Given the similarity between the gene expression profile associated with insecticide resistance and mitochondrial stress signaling, we hypothesize that disrupting some components of the mitochondrial stress signaling cascade identified as a result of IDH2 disruption could synergize with low doses of an insecticide to cause lethality.
Furthermore, we have found that disruption of IDH2 in Dm flight muscles culminates in the induction of a ferroptosis-like form of cell death that may provide opportunities for addressing the issue of insecticide resistance. Ferroptosis is a non-apoptotic form of cell death that is triggered by iron-dependent lipid peroxidation (Stockwell et al., 2017); however, it has not been explored extensively in Dm or mosquitoes. As emerging evidence indicates that there are likely to be Dm-specific aspects of ferroptosis, if similar observations are found in mosquitoes, it may provide an additional mechanism for combating insecticide-resistant mosquitoes.
Using fruit flies to gain a better understanding of cuticular resistance
For the most part, the molecular basis of cuticular resistance is still being unraveled; but some candidate genes have emerged that may regulate expression of cuticle proteins. As a case in point, the laccase gene, which encodes for a diphenol oxidase, is induced in fenvalerate-resistant strains of Culex pipiens pallens (Pan et al., 2009). This gene can be expressed in fruit flies exposed to fenvalerate to examine whether it regulates the expression of cuticle proteins or confers tolerance to the insecticide. Further, fruit flies can be exposed to various insecticides and a time series RNA-Seq experiment performed to assess the order of induction of genes involved in cuticle biogenesis. Subsequently, RNAi and overexpression analyses can be used to decipher the regulatory relationships between the genes and the degree to which they impact insecticide resistance.
Using fruit flies to gain a better understanding of behavioral resistance
The challenge of using fruit flies to study behavioral insecticide resistance lies in the fact that a paradigm for studying it has to be established first, as there is some debate about whether behavioral insecticide resistance mechanisms should be classified as such, or simply regarded as avoidance mechanisms (Zalucki and Furlong, 2017). Nevertheless, one area in which research in fruit flies could prove informative for dissecting the mechanism of behavioral resistance to insecticides, is by examining whether the insecticide in question can trigger an aversive response in the olfactory system. It has been shown that pyrethrum extracts from flower heads of Tanacetum cinerariifolium, which have long been used as insect repellants, evoke olfactory responses to cause aversion in both Dm and Drosophila suzukii (Liu et al., 2021). Further studies revealed that pyrethrin, the major component of pyrethrum, activates at least three distinct odorant receptors – Or7a, Or42b and Or59b – to elicit the insecticide repellent response. A similar set of experiments in Aedes aegypti mosquitoes revealed the importance of AaOr31 in conferring pyrethrum repellence in mosquitoes (Liu et al., 2021). While the result of this olfactory system-induced aversive response is beneficial, conceivably, a comparable phenomenon could occur as a result of insecticide spraying to trigger an aversive response which culminates in behavioral resistance.
The extensive arsenal of genetic tools for probing the Dm olfactory system make it an ideal system to uncover a possible role of the olfactory system in behavioral resistance. The Dm olfactory system senses airborne molecules via the activation of receptors located on olfactory receptor neurons (ORNs). Each ORN expresses G-protein-coupled receptors which elicit a unique odorant response profile for that neuron. Each olfactory receptor functions together with an obligate co-receptor dubbed Orco (olfactory receptor co-receptor). As a start, fruit flies can be exposed to an insecticide and the phenomenon of behavioral resistance examined in orco mutants. If the behavioral insecticide resistance response is abrogated in orco mutants, it will mean further studies dissecting the effect of eliminating individual olfactory receptors may uncover which ones are required for behavioral insecticide resistance.
Using fruit flies to identify new targets of insecticides
Established in 2005 through a $50 million grant from the Bill and Melinda Gates Foundation to the Liverpool School of Tropical Medicine, the Innovative Vector Control Consortium (IVCC) has been spearheading efforts to identify new and improved insecticides. In this respect, more than 4.5 million compounds have been evaluated for potential use as insecticides, which resulted in the identification of six classes of new active ingredients that have implications for malaria vector control (see https://www.ivcc.com/research-development/insecticide-discovery-and-development/). Other screening efforts have involved the use of malaria vector species (Lees et al., 2019; Lees et al., 2020). Nevertheless, fruit flies (Dm) can be a complementary model system for identifying new molecular targets for insecticides. As previously alluded to with respect to metabolic resistance, studies in fruit flies can identify how the multiple genes induced in response to insecticides are regulated; and how this can be exploited to suppress insecticide resistance. Moreover, there are many other pharmaceutical products that may be useful insecticides but have not yet been studied extensively in fruit flies. These include pymetrozine and flonicamid, which are modulators of chordotonal organs; semicarbazones, some classes of diamides and several other modulators of nerve and muscle function; and growth and development targets such as ecdysone receptor agonists and inhibitors of respiration. Studies in fruit flies are likely to provide useful information about their roles, but with the ultimate aim of confirming any promising results in mosquitoes, and working with the IVCC to further exploit any promising findings to prevent disease transmission.
Many respiratory inhibitors proposed as insecticides exert their effect largely by altering reactive oxygen species (ROS) production or other mitochondrial processes that do not involve a disintegration of the oxidative phosphorylation (OXPHOS) complexes. Examples include several inhibitors of complex I (CI), such as fenazaquin, pyridaben, fenpyroximate, pyrimidifen, tolfenpyrad and tebufenpyrad. Nonetheless, the broad specificity of these CI inhibitors has limited their use. As a case in point, tebufenpyrad and pyridaben alter mitochondrial dynamics in rat dopaminergic neuronal cultures (Charli et al., 2016). Their broad specificity has also meant that concentrations used in the field, during clinical trials, had to be chosen judiciously. As sub-lethal levels of mitochondrial ROS can activate compensatory stress responses (Owusu-Ansah et al., 2008; Owusu-Ansah and Banerjee, 2009; Owusu-Ansah et al., 2013; Murari et al., 2021; Murari et al., 2022), this may explain, at least in part, why a clinical trial in Tanzania exploring the effectiveness of fenpyroximate and abamectin-treated durable wall liners as a control mechanism for malaria was not successful (Mpangala et al., 2021). We postulate that a more favorable outcome could be achieved with respiratory chain inhibitors that block aspects of the assembly of the OXPHOS system. We have been studying the mechanism of mitochondrial CI assembly in Dm and found that severe RNAi-mediated disruption of multiple CI subunits is lethal (Garcia et al., 2017). We hypothesize that a similar phenomenon may occur in other dipterans such as mosquitoes that could be exploited to develop new insecticides.
Mitochondrial CI is the most elaborate component of the OXPHOS system (Fiedorczuk et al., 2016; Agip et al., 2018; Rhooms et al., 2019). Mammalian CI has 45 subunits organized into two domains of the complex, oriented almost perpendicularly to each other, and referred to as the matrix and membrane domains (Figure 1) (Agip et al., 2019). There are three distinct functional modules of CI dubbed the N, Q, and P modules. The N module contains the flavin mononucleotide (FMN) cofactor and accepts electrons from NADH in the mitochondrial matrix. The Q module is situated between the N module and the membrane domain and transfers electrons to ubiquinone. The proton-pumping P module is essentially the membrane domain; and can be further sub-divided into a proximal PP and distal PD module [reviewed in (Vartak et al., 2014; Formosa et al., 2018; Rhooms et al., 2019)]. A total of 14 core subunits contain all the catalytic centers of CI and are conserved from the ancestral enzyme in bacteria to the eukaryotic enzyme. Seven core subunits (NDUFS1, NDUFS2, NDUFS3, NDUFS7, NDUFS8, NDUFV1 and NDUFV2) are encoded by the nucleus, translated in the cytoplasm, and imported into the mitochondrion; while the other core subunits are encoded and translated within the mitochondrion (ND1, ND2, ND3, ND4, ND4L, ND5 and ND6). The 31 remaining subunits are referred to as accessory or supernumerary subunits, as they are not directly involved in performing the bioenergetics functions of CI. During CI assembly, specific subcomplexes consisting of a few CI subunits form largely independently of each other and merge in a stereotypic fashion en route to forming the mature complex (Stroud et al., 2016; Garcia et al., 2017; Formosa et al., 2018). CI assembly factors (CIAFs) are proteins that are usually found in association with specific subcomplexes and assist with the assembly process; but they are subsequently released when assembly is complete. The N, Q and P modules are synthesized from specific subcomplexes or assembly intermediates that can be tracked by immunoblotting or complexome profiling techniques (Guerrero-Castillo et al., 2017; Formosa et al., 2020; Murari et al., 2020; Szczepanowska et al., 2020).
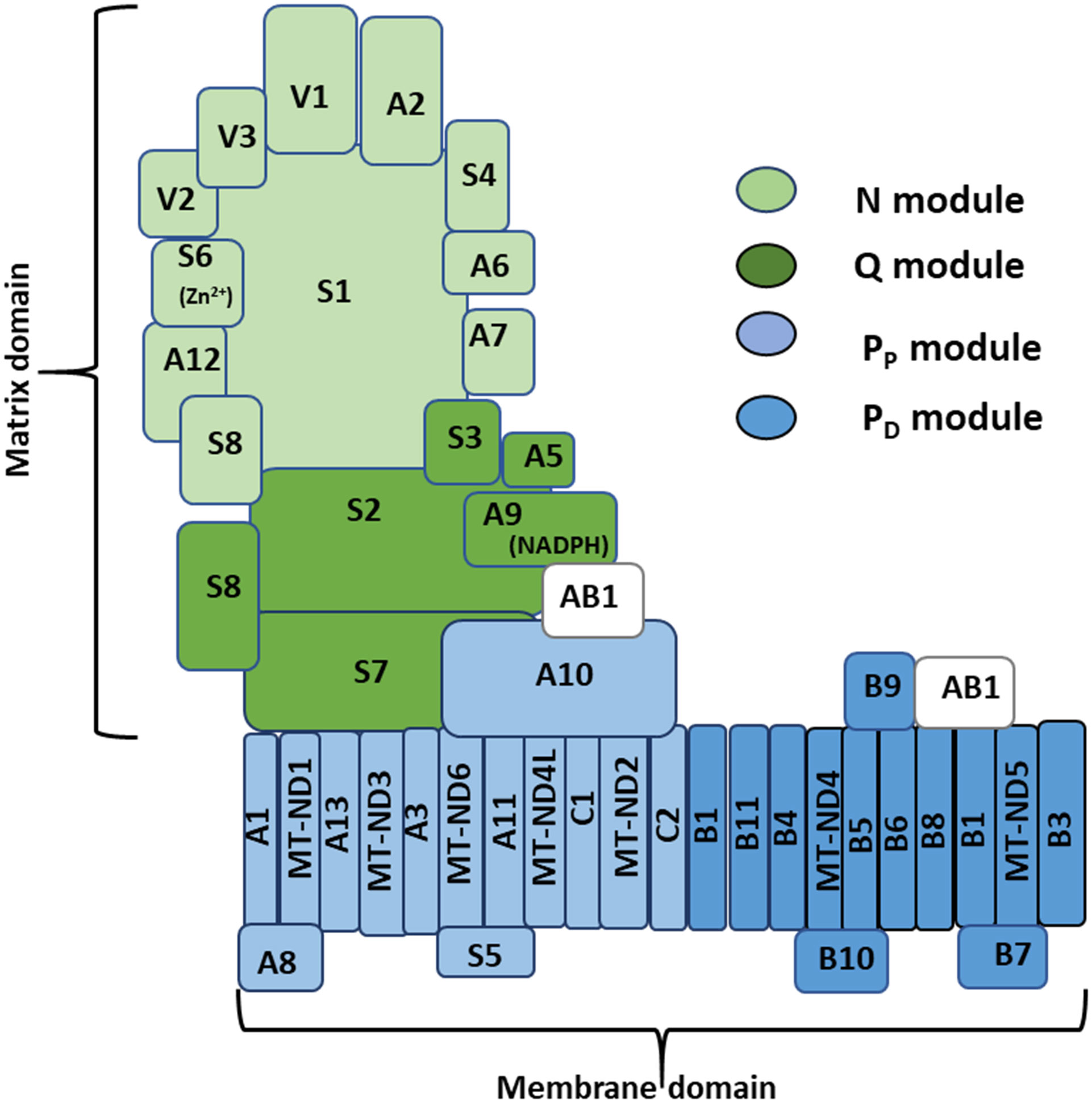
Figure 1 Architecture of mitochondrial complex I. A diagram of mitochondrial CI – based on mammalian Cryo-EM studies – depicting the hydrophilic matrix and hydrophobic membrane domains oriented almost perpendicularly to each other; and the relative positions of subunits localized to the N, Q, PP and PD modules. There are 45 subunits in mammalian CI, 44 of which are unique subunits, and NDUFAB1 (AB1), which appears twice in the complex. Fourteen of the 45 subunits are referred to as core subunits, as they are conserved in all organisms in which CI is present (see text for further details). NDUFS6 (S6) and NDUFA9 (A9) contain Zn2+ and NADPH as co-factors, respectively. Additional co-factors omitted for clarity include a flavin mononucleotide molecule and several Fe-S clusters found in association with some subunits in the matrix domain, and a number of phospholipid molecules intertwined with several subunits in the membrane domain.
Most of the mammalian accessory subunits are conserved in Dm (Garcia et al., 2017), but the extent to which each accessory subunit contributes to CI assembly differs between organisms. For instance, while RNAi-mediated disruption of NDUFA12 potently impairs CI assembly in Dm flight muscles and can cause lethality, a CRISPR-mediated knockout of NDUFA12 in human cells has minimal effects on CI assembly (Stroud et al., 2016; Garcia et al., 2017). We anticipate that such differences in roles of CI subunits in CI assembly can be exploited to make new arthropod- or diptera-specific CI assembly inhibitors, which can ultimately result in the development of novel insecticides.
Conclusion and future perspectives
There is an ever-present need to identify novel mosquito insecticides that are more effective and selective, and entail new mechanisms of action. This may require homology modeling for identifying novel chemistries and insect-specific modes of regulation of already established targets, as was performed for the housefly VGSC (O’Reilly et al., 2006). Alternatively, it may be necessary to screen for novel sites in genes that are well established insecticide targets, as was performed for the isooxazoline insecticide, A1443, a ligand-gated chloride channel antagonist (García-Reynaga et al., 2013). It is also crucial to uncover entirely novel biochemical targets, as was the case with specific classes of diamides that were found to modulate insect ryanodine receptor function (Sattelle et al., 2008). Molecular targets that regulate developmental pathways in insects, such as cell death, autophagy, molting, and basic organelle function could also be exploited. In fact, the importance of autophagy in the fat body, and ecdysone signaling in regulating molting and other aspects of mosquito physiology, has been described extensively, highlighting their potential utilization as targets of insecticides (Bryant and Raikhel, 2011; Childs et al., 2016; Shaw and Catteruccia, 2019; Werling et al., 2019; Brown et al., 2020; Ekoka et al., 2021; Maharaj et al., 2022). While, undoubtedly, studies in fruit flies can contribute to our understanding of all these putative aspects of mosquito insecticide development, we propose that developing insecticides that disrupt organelle function to cause cell death, especially those that inhibit mitochondrial CI assembly, via a mechanism distinct from how CI assembly is regulated in humans, holds great promise for identifying new and insect-specific targets of insecticides. However, we note that at best, Dm should only be regarded as a complementary model system for studying mosquito insecticide resistance as any promising leads would have to be confirmed in mosquitoes.
Author contributions
EO-A wrote the review and got feedback from KFBH and DV. All authors contributed to the generation of the figure. All authors approve the submission.
Funding
Funding for this review was provided by a Schaefer Research Scholars award and NIH grants AR077312 (R21), GM124717 (R35) and GM147902-01A1 (R01), and start-up funds from the Department of Physiology and Cellular Biophysics of the Columbia University Irving Medical Center to EO-A. KFBH and DV were supported by the aforementioned funds listed to EO-A.
Acknowledgments
We thank members of the EO-A lab for general discussions.
Conflict of interest
The authors declare that the research was conducted in the absence of any commercial or financial relationships that could be construed as a potential conflict of interest.
Publisher’s note
All claims expressed in this article are solely those of the authors and do not necessarily represent those of their affiliated organizations, or those of the publisher, the editors and the reviewers. Any product that may be evaluated in this article, or claim that may be made by its manufacturer, is not guaranteed or endorsed by the publisher.
References
Agip A. A., Blaza J. N., Bridges H. R., Viscomi C., Rawson S., Muench S. P., et al. (2018). Cryo-EM structures of complex I from mouse heart mitochondria in two biochemically defined states. Nat. Struct. Mol. Biol. 25 (7), 548–556. doi: 10.1038/s41594-018-0073-1
Agip A. A., Blaza J. N., Fedor J. G., Hirst J. (2019). Mammalian respiratory complex I through the lens of cryo-EM. Annu. Rev. Biophys. 48, 165–184. doi: 10.1146/annurev-biophys-052118-115704
Alout H., Berthomieu A., Cui F., Tan Y., Berticat C., Qiao C., et al. (2007a). A new amino-acid substitution in acetylcholinesterase 1 confers insecticide resistance to culex pipiens mosquitoes from Cyprus. Insect Biochem. Mol. Biol. 37 (1), 41–47. doi: 10.1016/j.ibmb.2006.10.001
Alout H., Berthomieu A., Hadjivassilis A., Weill M. (2007b). Different amino-acid substitutions confer insecticide resistance through acetylcholinesterase 1 insensitivity in culex vishnui and culex tritaeniorhynchus (Diptera: culicidae) from China. J. Med. Entomol. 44 (3), 463–469. doi: 10.1093/jmedent/44.3.463
Brand A. H., Perrimon N. (1993). Targeted gene expression as a means of altering cell fates and generating dominant phenotypes. Development 118 (2), 401–415. doi: 10.1242/dev.118.2.401
Brengues C., Hawkes N. J., Chandre F., McCarroll L., Duchon S., Guillet P., et al. (2003). Pyrethroid and DDT cross-resistance in aedes aegypti is correlated with novel mutations in the voltage-gated sodium channel gene. Med. Vet. Entomol. 17 (1), 87–94. doi: 10.1046/j.1365-2915.2003.00412.x
Brown F., Paton D. G., Catteruccia F., Ranson H., Ingham V. A. (2020). A steroid hormone agonist reduces female fitness in insecticide-resistant anopheles populations. Insect Biochem. Mol. Biol. 121, 103372. doi: 10.1016/j.ibmb.2020.103372
Bryant B., Raikhel A. S. (2011). Programmed autophagy in the fat body of aedes aegypti is required to maintain egg maturation cycles. PloS One 6 (11), e25502. doi: 10.1371/journal.pone.0025502
Chandor-Proust A., Bibby J., Régent-Kloeckner M., Roux J., Guittard-Crilat E., Poupardin R., et al. (2013). The central role of mosquito cytochrome P450 CYP6Zs in insecticide detoxification revealed by functional expression and structural modelling. Biochem. J. 455 (1), 75–85. doi: 10.1042/BJ20130577
Chang C., Shen W. K., Wang T. T., Lin Y. H., Hsu E. L., Dai S. M. (2009). A novel amino acid substitution in a voltage-gated sodium channel is associated with knockdown resistance to permethrin in aedes aegypti. Insect Biochem. Mol. Biol. 39 (4), 272–278. doi: 10.1016/j.ibmb.2009.01.001
Charli A., Jin H., Anantharam V., Kanthasamy A., Kanthasamy A. G. (2016). Alterations in mitochondrial dynamics induced by tebufenpyrad and pyridaben in a dopaminergic neuronal cell culture model. Neurotoxicology 53, 302–313. doi: 10.1016/j.neuro.2015.06.007
Childs L. M., Cai F. Y., Kakani E. G., Mitchell S. N., Paton D., Gabrieli P., et al. (2016). Disrupting mosquito reproduction and parasite development for malaria control. PloS Pathog. 12 (12), e1006060. doi: 10.1371/journal.ppat.1006060
Clark A. G., Shamaan N. A. (1984). Evidence that DDT-dehydrochlorinase from the house fly is a glutathione s-transferase. Pesticide Biochem. Physiol. 22 (3), 249–261. doi: 10.1016/0048-3575(84)90018-X
Djogbénou L., Akogbéto M., Chandre F. (2008). Presence of insensitive acetylcholinesterase in wild populations of culex pipiens quinquefasciatus from Benin. Acta Trop. 107 (3), 272–274. doi: 10.1016/j.actatropica.2008.06.004
Du W., Awolola T. S., Howell P., Koekemoer L. L., Brooke B. D., Benedict M. Q., et al. (2005). Independent mutations in the rdl locus confer dieldrin resistance to anopheles gambiae and an. arabiensis. Insect Mol. Biol. 14 (2), 179–183. doi: 10.1111/j.1365-2583.2005.00544.x
Dusfour I., Vontas J., David J. P., Weetman D., Fonseca D. M., Corbel V., et al. (2019). Management of insecticide resistance in the major aedes vectors of arboviruses: advances and challenges. PloS Negl. Trop. Dis. 13 (10), e0007615. doi: 10.1371/journal.pntd.0007615
Ekoka E., Maharaj S., Nardini L., Dahan-Moss Y., Koekemoer L. L. (2021). 20-hydroxyecdysone (20E) signaling as a promising target for the chemical control of malaria vectors. Parasit Vectors 14 (1), 86. doi: 10.1186/s13071-020-04558-5
Enayati A. A., Vatandoost H., Ladonni H., Townson H., Hemingway J. (2003). Molecular evidence for a kdr-like pyrethroid resistance mechanism in the malaria vector mosquito anopheles stephensi. Med. Vet. Entomol. 17 (2), 138–144. doi: 10.1046/j.1365-2915.2003.00418.x
Enayati A. A., Ranson H., Hemingway J. (2005). Insect glutathione transferases and insecticide resistance. Insect Mol. Biol. 14 (1), 3–8. doi: 10.1111/j.1365-2583.2004.00529.x
Fiedorczuk K., Letts J. A., Degliesposti G., Kaszuba K., Skehel M., Sazanov L. A., et al. (2016). Atomic structure of the entire mammalian mitochondrial complex I. Nature 538 (7625), 406–410. doi: 10.1038/nature19794
Fischer J. A., Giniger E., Maniatis T., Ptashne M. (1988). GAL4 activates transcription in drosophila. Nature 332 (6167), 853–856. doi: 10.1038/332853a0
Formosa L. E., Dibley M. G., Stroud D. A., Ryan M. T. (2018). Building a complex complex: assembly of mitochondrial respiratory chain complex I. Semin. Cell Dev. Biol. 76, 154–162. doi: 10.1016/j.semcdb.2017.08.011
Formosa L. E., Muellner-Wong L., Reljic B., Sharpe A. J., Jackson T. D., Beilharz T. H., et al. (2020). Dissecting the roles of mitochondrial complex I intermediate assembly complex factors in the biogenesis of complex I. Cell Rep. 31 (3), 107541. doi: 10.1016/j.celrep.2020.107541
Fournier D., Mutero A. (1994). Modification of acetylcholinesterase as a mechanism of resistance eo insecticides. Comp. Biochem. Physiol. C-Pharmacology Toxicol. Endocrinol. 108 (1), 19–31. doi: 10.1016/1367-8280(94)90084-1
Garcia C. J., Khajeh J., Coulanges E., Chen E. I., Owusu-Ansah E. (2017). Regulation of mitochondrial complex I biogenesis in drosophila flight muscles. Cell Rep. 20 (1), 264–278. doi: 10.1016/j.celrep.2017.06.015
García-Reynaga P., Zhao C., Sarpong R., Casida J. E. (2013). New GABA/glutamate receptor target for [³H]isoxazoline insecticide. Chem. Res. Toxicol. 26 (4), 514–516. doi: 10.1021/tx400055p
Gleave K., Lissenden N., Chaplin M., Choi L., Ranson H. (2021). Piperonyl butoxide (PBO) combined with pyrethroids in insecticide-treated nets to prevent malaria in Africa. Cochrane Database Syst. Rev. 5 (5), CD012776. doi: 10.1002/14651858.CD012776.pub3
Guerrero-Castillo S., Baertling F., Kownatzki D., Wessels H. J., Arnold S., Brandt U., et al. (2017). The assembly pathway of mitochondrial respiratory chain complex I. Cell Metab. 25 (1), 128–139. doi: 10.1016/j.cmet.2016.09.002
Habig W. H., Pabst M. J., Jakoby W. B. (1974). Glutathione s-transferases. the first enzymatic step in mercapturic acid formation. J. Biol. Chem. 249 (22), 7130–7139. doi: 10.1016/S0021-9258(19)42083-8
Hemingway J., Hawkes N. J., McCarroll L., Ranson H. (2004). The molecular basis of insecticide resistance in mosquitoes. Insect Biochem. Mol. Biol. 34 (7), 653–665. doi: 10.1016/j.ibmb.2004.03.018
Huang Y., Guo Q., Sun X., Zhang C., Xu N., Xu Y., et al. (2018). Culex pipiens pallens cuticular protein CPLCG5 participates in pyrethroid resistance by forming a rigid matrix. Parasit. Vectors 11 (1), 6. doi: 10.1186/s13071-017-2567-9
Kaduskar B., Kushwah R. B. S., Auradkar A., Guichard A., Li M., Bennett J. B., et al. (2022). Reversing insecticide resistance with allelic-drive in drosophila melanogaster. Nat. Commun. 13 (1), 291. doi: 10.1038/s41467-021-27654-1
Ke Y. T., Hsu H. J. (2019). Generation of inducible gene-switched GAL4 expressed in the. G3 (Bethesda) 9 (6), 2007–2016. doi: 10.1534/g3.119.400246
Kim J. J., Hibbs R. E. (2021). Direct structural insights into GABA(A) receptor pharmacology. Trends Biochem. Sci. 46 (6), 502–517. doi: 10.1016/j.tibs.2021.01.011
Lees R., Praulins G., Davies R., Brown F., Parsons G., White A., et al. (2019). A testing cascade to identify repurposed insecticides for next-generation vector control tools: screening a panel of chemistries with novel modes of action against a malaria vector. Gates Open Res. 3, 1464. doi: 10.12688/gatesopenres.12957.2
Lees R. S., Ismail H. M., Logan R. A. E., Malone D., Davies R., Anthousi A., et al. (2020). New insecticide screening platforms indicate that mitochondrial complex I inhibitors are susceptible to cross-resistance by mosquito P450s that metabolise pyrethroids. Sci. Rep. 10 (1), 16232. doi: 10.1038/s41598-020-73267-x
Li T., Liu L., Zhang L., Liu N. (2014). Role of G-protein-coupled receptor-related genes in insecticide resistance of the mosquito, culex quinquefasciatus. Sci. Rep. 4, 6474. doi: 10.1038/srep06474
Lin W. H., Wright D. E., Muraro N. I., Baines R. A. (2009). Alternative splicing in the voltage-gated sodium channel DmNav regulates activation, inactivation, and persistent current. J. Neurophysiol. 102 (3), 1994–2006. doi: 10.1152/jn.00613.2009
Lin W. H., Günay C., Marley R., Prinz A. A., Baines R. A. (2012). Activity-dependent alternative splicing increases persistent sodium current and promotes seizure. J. Neurosci. 32 (21), 7267–7277. doi: 10.1523/JNEUROSCI.6042-11.2012
Liu N. (2015). Insecticide resistance in mosquitoes: impact, mechanisms, and research directions. Annu. Rev. Entomol. 60, 537–559. doi: 10.1146/annurev-ento-010814-020828
Liu H., Cupp E. W., Micher K. M., Guo A., Liu N. (2004). Insecticide resistance and cross-resistance in Alabama and Florida strains of culex quinquefasciatus [correction]. J. Med. Entomol. 41 (3), 408–413. doi: 10.1603/0022-2585-41.3.408
Liu N., Liu H., Zhu F., Zhang L. (2007). Differential expression of genes in pyrethroid resistant and susceptible mosquitoes, culex quinquefasciatus (S.). Gene 394 (1-2), 61–68. doi: 10.1016/j.gene.2007.01.032
Liu F., Wang Q., Xu P., Andreazza F., Valbon W. R., Bandason E., et al. (2021). A dual-target molecular mechanism of pyrethrum repellency against mosquitoes. Nat. Commun. 12 (1), 2553. doi: 10.1038/s41467-021-22847-0
Maharaj S., Ekoka E., Erlank E., Nardini L., Reader J., Birkholtz L. M., et al. (2022). The ecdysone receptor regulates several key physiological factors in anopheles funestus. Malar J. 21 (1), 97. doi: 10.1186/s12936-022-04123-8
Martinez-Torres D., Chandre F., Williamson M. S., Darriet F., Bergé J. B., Devonshire A. L., et al. (1998). Molecular characterization of pyrethroid knockdown resistance (kdr) in the major malaria vector anopheles gambiae s.s. Insect Mol. Biol. 7 (2), 179–184. doi: 10.1046/j.1365-2583.1998.72062.x
McGuire S. E., Mao Z., Davis R. L. (2004). Spatiotemporal gene expression targeting with the TARGET and gene-switch systems in drosophila. Sci. STKE 2004 (220), pl6. doi: 10.1126/stke.2202004pl6
Mpangala K. R., Halasa-Rappel Y. A., Mohamed M. S., Mnzava R. C., Mkuza K. J., Mangesho P. E., et al. (2021). On the cost-effectiveness of insecticide-treated wall liner and indoor residual spraying as additions to insecticide treated bed nets to prevent malaria: findings from cluster randomized trials in Tanzania. BMC Public Health 21 (1), 1666. doi: 10.1186/s12889-021-11671-2
Murari A., Rhooms S. K., Goparaju N. S., Villanueva M., Owusu-Ansah E. (2020). An antibody toolbox to track complex I assembly defines AIF’s mitochondrial function. J. Cell Biol. 219 (10), e202001071. doi: 10.1083/jcb.202001071
Murari A., Rhooms S. K., Garcia C., Liu T., Li H., Mishra B., et al. (2021). Dissecting the concordant and disparate roles of NDUFAF3 and NDUFAF4 in mitochondrial complex I biogenesis. iScience 24 (8), 102869. doi: 10.1016/j.isci.2021.102869
Murari A., Goparaju N. S. V., Rhooms S. K., Hossain K. F. B., Liang F. G., Garcia C. J., et al. (2022). IDH2-mediated regulation of the biogenesis of the oxidative phosphorylation system. Sci. Adv. 8 (19), eabl8716. doi: 10.1126/sciadv.abl8716.
Nicholson L., Singh G. K., Osterwalder T., Roman G. W., Davis R. L., Keshishian H. (2008). Spatial and temporal control of gene expression in drosophila using the inducible GeneSwitch GAL4 system. i. screen for larval nervous system drivers. Genetics 178 (1), 215–234. doi: 10.1534/genetics.107.081968
Nkya T. E., Poupardin R., Laporte F., Akhouayri I., Mosha F., Magesa S., et al. (2014). Impact of agriculture on the selection of insecticide resistance in the malaria vector anopheles gambiae: a multigenerational study in controlled conditions. Parasit. Vectors 7, 480. doi: 10.1186/s13071-014-0480-z
O’Reilly A. O., Khambay B. P., Williamson M. S., Field L. M., Wallace B. A., Davies T. G., et al. (2006). Modelling insecticide-binding sites in the voltage-gated sodium channel. Biochem. J. 396 (2), 255–263. doi: 10.1042/BJ20051925
Ortelli F., Rossiter L. C., Vontas J., Ranson H., Hemingway J. (2003). Heterologous expression of four glutathione transferase genes genetically linked to a major insecticide-resistance locus from the malaria vector anopheles gambiae. Biochem. J. 373 (Pt 3), 957–963. doi: 10.1042/bj20030169
Owusu-Ansah E., Banerjee U. (2009). Reactive oxygen species prime drosophila haematopoietic progenitors for differentiation. Nature 461 (7263), 537–541. doi: 10.1038/nature08313.
Owusu-Ansah E., Yavari A., Mandal S., Banerjee U. (2008). Distinct mitochondrial retrograde signals control the G1-s cell cycle checkpoint. Nat. Genet. 40 (3), 356–361. doi: 10.1038/ng.2007.50
Owusu-Ansah E., Song W., Perrimon N. (2013). Muscle mitohormesis promotes longevity via systemic repression of insulin signaling. Cell 155 (3), 699–712. doi: 10.1016/j.cell.2013.09.021
Pan C., Zhou Y., Mo J. (2009). The clone of laccase gene and its potential function in cuticular penetration resistance of culex pipiens pallens to fenvalerate. Pesticide Biochem. Physiol. 93 (3), 105–111. doi: 10.1016/j.pestbp.2008.12.003
Pavlidi N., Monastirioti M., Daborn P., Livadaras I., Van Leeuwen T., Vontas J. (2012). Transgenic expression of the aedes aegypti CYP9J28 confers pyrethroid resistance in drosophila melanogaster. Pesticide Biochem. Physiol. 104 (2), 132–135. doi: 10.1016/j.pestbp.2012.07.003
Ravenscroft T. A., Janssens J., Lee P. T., Tepe B., Marcogliese P. C., Makhzami S., et al. (2020). Voltage-gated sodium channels are only expressed in active neurons and are localized to distal axonal initial segment-like domains. J. Neurosci. 40 (42), 7999–8024. doi: 10.1523/JNEUROSCI.0142-20.2020
Reddy M. R., Overgaard H. J., Abaga S., Reddy V. P., Caccone A., Kiszewski A. E., et al. (2011). Outdoor host seeking behaviour of anopheles gambiae mosquitoes following initiation of malaria vector control on bioko island, equatorial Guinea. Malar. J. 10, 184. doi: 10.1186/1475-2875-10-184
Rhooms S. K., Murari A., Goparaju N. S. V., Vilanueva M., Owusu-Ansah E. (2019). Insights from drosophila on mitochondrial complex I. Cell Mol. Life Sci 77, 607–618. doi: 10.1007/s00018-019-03293-0.
Riveron J. M., Irving H., Ndula M., Barnes K. G., Ibrahim S. S., Paine M. J., et al. (2013). Directionally selected cytochrome P450 alleles are driving the spread of pyrethroid resistance in the major malaria vector anopheles funestus. Proc. Natl. Acad. Sci. USA 110 (1), 252–257. doi: 10.1073/pnas.1216705110
Roman G., Endo K., Zong L., Davis R. L. (2001). P[Switch], a system for spatial and temporal control of gene expression in drosophila melanogaster. Proc. Natl. Acad. Sci. USA 98 (22), 12602–12607. doi: 10.1073/pnas.221303998
Russell T. L., Govella N. J., Azizi S., Drakeley C. J., Kachur S. P., Killeen G. F. (2011). Increased proportions of outdoor feeding among residual malaria vector populations following increased use of insecticide-treated nets in rural Tanzania. Malar. J. 10, 80. doi: 10.1186/1475-2875-10-80
Salinas A. E., Wong M. G. (1999). Glutathione s-transferases–a review. Curr. Med. Chem. 6 (4), 279–309. doi: 10.2174/0929867306666220208213032
Sattelle D. B., Cordova D., Cheek T. R. (2008). Insect ryanodine receptors: molecular targets for novel pest control chemicals. Invert Neurosci. 8 (3), 107–119. doi: 10.1007/s10158-008-0076-4
Shaw W. R., Catteruccia F. (2019). Vector biology meets disease control: using basic research to fight vector-borne diseases. Nat. Microbiol. 4 (1), 20–34. doi: 10.1038/s41564-018-0214-7
Stockwell B. R., Friedmann Angeli J. P., Bayir H., Bush A. I., Conrad M., Dixon S. J., et al. (2017). Ferroptosis: a regulated cell death nexus linking metabolism, redox biology, and disease. Cell 171 (2), 273–285. doi: 10.1016/j.cell.2017.09.021
Stroud D. A., Surgenor E. E., Formosa L. E., Reljic B., Frazier A. E., Dibley M. G., et al. (2016). Accessory subunits are integral for assembly and function of human mitochondrial complex I. Nature 538 (7623), 123–126. doi: 10.1038/nature19754
Suh P. F., Elanga-Ndille E., Tchouakui M., Sandeu M. M., Tagne D., Wondji C., et al. (2023). Impact of insecticide resistance on malaria vector competence: a literature review. Malar. J. 22 (1), 19. doi: 10.1186/s12936-023-04444-2
Sun X., Guo J., Ye W., Guo Q., Huang Y., Ma L., et al. (2017). Cuticle genes CpCPR63 and CpCPR47 may confer resistance to deltamethrin in culex pipiens pallens. Parasitol. Res. 116 (8), 2175–2179. doi: 10.1007/s00436-017-5521-z
Susanna D., Pratiwi D. (2021). Current status of insecticide resistance in malaria vectors in the Asian countries: a systematic review. F1000Res 10, 200. doi: 10.12688/f1000research.46883.1
Szczepanowska K., Senft K., Heidler J., Herholz M., Kukat A., Höhne M. N., et al. (2020). A salvage pathway maintains highly functional respiratory complex I. Nat. Commun. 11 (1), 1643. doi: 10.1038/s41467-020-15467-7
Vannini L., Reed T. W., Willis J. H. (2014). Temporal and spatial expression of cuticular proteins of anopheles gambiae implicated in insecticide resistance or differentiation of M/S incipient species. Parasit. Vectors 7, 24. doi: 10.1186/1756-3305-7-24
Vartak R. S., Semwal M. K., Bai Y. (2014). An update on complex I assembly: the assembly of players. J. Bioenerg Biomembr. 46 (4), 323–328. doi: 10.1007/s10863-014-9564-x
Vontas J., Blass C., Koutsos A. C., David J. P., Kafatos F. C., Louis C., et al. (2005). Gene expression in insecticide resistant and susceptible anopheles gambiae strains constitutively or after insecticide exposure. Insect Mol. Biol. 14 (5), 509–521. doi: 10.1111/j.1365-2583.2005.00582.x
Vulule J. M., Beach R. F., Atieli F. K., McAllister J. C., Brogdon W. G., Roberts J. M., et al. (1999). Elevated oxidase and esterase levels associated with permethrin tolerance in anopheles gambiae from Kenyan villages using permethrin-impregnated nets. Med. Vet. Entomol. 13 (3), 239–244. doi: 10.1046/j.1365-2915.1999.00177.x
Weill M., Fort P., Berthomieu A., Dubois M. P., Pasteur N., Raymond M. (2002). A novel acetylcholinesterase gene in mosquitoes codes for the insecticide target and is non-homologous to the ace gene in drosophila. Proc. Biol. Sci. 269 (1504), 2007–2016. doi: 10.1098/rspb.2002.2122
Weill M., Lutfalla G., Mogensen K., Chandre F., Berthomieu A., Berticat C., et al. (2003). Comparative genomics: insecticide resistance in mosquito vectors. Nature 423 (6936), 136–137. doi: 10.1038/423136b
Werling K., Shaw W. R., Itoe M. A., Westervelt K. A., Marcenac P., Paton D. G., et al. (2019). Steroid hormone function controls non-competitive plasmodium development in anopheles. Cell 177 (2), 315–325.e14. doi: 10.1016/j.cell.2019.02.036
Wondji C. S., Dabire R. K., Tukur Z., Irving H., Djouaka R., Morgan J. C. (2011). Identification and distribution of a GABA receptor mutation conferring dieldrin resistance in the malaria vector anopheles funestus in Africa. Insect Biochem. Mol. Biol. 41 (7), 484–491. doi: 10.1016/j.ibmb.2011.03.012
Xu Y., Xu J., Zhou Y., Li X., Meng Y., Ma L., et al. (2022). CPR63 promotes pyrethroid resistance by increasing cuticle thickness in culex pipiens pallens. Parasit. Vectors 15 (1), 54. doi: 10.1186/s13071-022-05175-0
Yahouédo G. A., Chandre F., Rossignol M., Ginibre C., Balabanidou V., Mendez N. G. A., et al. (2017). Contributions of cuticle permeability and enzyme detoxification to pyrethroid resistance in the major malaria vector anopheles gambiae. Sci. Rep. 7 (1), 11091.
Zalucki M. P., Furlong M. J. (2017). Behavior as a mechanism of insecticide resistance: evaluation of the evidence. Curr. Opin. Insect Sci. 21, 19–25. doi: 10.1016/j.cois.2017.05.006
Zhou D., Duan B, Sun Y., Ma L., Zhu C., Shen B. (2017). Preliminary characterization of putative structural cuticular proteins in the malaria vector anopheles sinensis. Pest Manag. Sci. 73 (12), 2519–2528. doi: 10.1002/ps.4649
Keywords: malaria, insecticide resistance, insect vectors, mosquitoes, fruit flies, Drosophila melanogaster
Citation: Hossain KFB, Vimal D and Owusu-Ansah E (2023) Using fruit flies to delve into mosquito insecticide resistance. Front. Malar. 1:1073761. doi: 10.3389/fmala.2023.1073761
Received: 18 October 2022; Accepted: 05 May 2023;
Published: 20 June 2023.
Edited by:
Louisa Alexandra Messenger, University of Nevada, Las Vegas, United StatesReviewed by:
Elis Batista, Federal University of Minas Gerais, BrazilRichard Oxborough, Consultant, Henderson, NV, United States
Matthew Meiselman, University of Nevada, Las Vegas, United States
Copyright © 2023 Hossain, Vimal and Owusu-Ansah. This is an open-access article distributed under the terms of the Creative Commons Attribution License (CC BY). The use, distribution or reproduction in other forums is permitted, provided the original author(s) and the copyright owner(s) are credited and that the original publication in this journal is cited, in accordance with accepted academic practice. No use, distribution or reproduction is permitted which does not comply with these terms.
*Correspondence: Edward Owusu-Ansah, ZW8yMzY0QGN1bWMuY29sdW1iaWEuZWR1